- 1Division of Maternal and Child Health, Department of Translational Medicine, Research Branch, Sidra Medicine, Doha, Qatar
- 2College of Health and Life Sciences, Hamad Bin Khalifa University, Ar-Rayyan, Qatar
A mother is gifted with breast milk, the natural source of nutrition for her infant. In addition to the wealth of macro and micro-nutrients, human milk also contains many microorganisms, few of which originate from the mother, while others are acquired from the mouth of the infant and the surroundings. Among these microbes, the most commonly residing bacteria are Staphylococci, Streptococci, Lactobacilli and Bifidobacteria. These microorganisms initiate and help the development of the milk microbiota as well as the microbiota of the gastrointestinal tract in infants, and contribute to developing immune regulatory factors such as cytokines, growth factors, lactoferrin among others. These factors play an important role in reducing the risk of developing chronic diseases like type 2 diabetes, asthma and others later in life. In this review, we will summarize the known benefits of breastfeeding and highlight the role of the breast milk microbiota and its cross-talk with the immune system in breastfed babies during the early years of life.
Introduction
Breast milk (BM) is the normative source of nutrition for infants in the first six months of life (1). It is considered an essential source of nutrients containing water (87%), fat (3.8%), proteins (1.0%), and lactose (7%), with both lactose and fat providing 40 and 50% of the total energy received from milk (2). BM also contains immune cells, microRNAs, hormones and bioactive compounds with anti-inflammatory, anti-infective properties (3). These include cytokines, chemokines, immunoglobulins, hormones, growth factors, oligosaccharides and antimicrobial peptides such as bacteriocin and lactoferrin (4). Studies have shown that the composition of BM varies depending on maternal and environmental factors, and is tailored to the baby's complex nutritional requirements (5).
Our understanding of the origin of milk microbiota and its role in seeding the infant's gut is still in its infancy and needs further research (6). The delivery mode appears to be an important factor in the development of the infant's gut microbiota (7, 8). Babies born via Caesarian section (C-section) are colonized by microbial communities similar to their mothers' skin microbiota, opposed to the vaginally-delivered babies whose microbiota is closer to their mothers' vaginal microbes (9). Furthermore, it is known that the rupture of the membranes during labor contributes to the early microbial seeding of the newborn (10). This transfer of microbes from the mother to her baby, during delivery, is like a “good starter kit” that will help expand the infant's microbiota.
Studies have shown that breastfed infants have higher levels of Bifidobacterium species in their gut, which has been attributed to the human milk oligosaccharides (HMOs) known to preferentially feed this bacterium (11, 12). This is in contrast to the formula-fed infants where the gut is inhabited by Bacteroides, Firmicutes, Eubacterium and Veillonella (13). When solid food is supplemented after 6 months of age and BM is no longer considered the major source of nutrients, levels of Bifidobacterium in the gutdrop dramatically, while levels of Lachnospiraceace and Ruminoccoceae increase (13). During this formative stage, the child's gut microbiota continues to grow in number and diversity of its microbial communities. At around 14 months old, when most children stop receiving BM, the toddler's gut microbiota enters a transitional stage during which the microbial communities of the gut are considered unstable and can be disrupted by various factors such as antibiotics use, diet and other environmental factors (14). However, as the child grows older, the microbial diversity of the gut becomes more stable and mainly dominated by Firmicutes (Lachnospiraceae and Ruminococcaceae), Bacteroidetes (Bacteroidaceae, Prevotellaceae, and Rikenellaceae), and Actinobacteria (Bifidobacteriaceae and Coriobacteriaceae) (15).
Similar to the gradual changes observed in the gut microbiota composition, the immune system of the infant goes through different phases of maturation in early life, in response to microbial exposures that primarily take place at the mucosa of the respiratory and gastrointestinal tracts (16). Babies are born with a naive immune system making it difficult to fight infectious pathogens directly after birth (17). During lactation, BM provides a source rich with IgA, anti-inflammatory factors and immunologically active cells needed to induce both tolerance to non-harmful antigens (food antigens or beneficial commensal microbes) and to develop a robust immune defense against pathogenic organisms (18).
Currently a comprehensive understanding of the diverse composition of the BM microbiota including prokaryotes (bacteria, archaea) and eukaryotes (fungi) is lacking. In this article, we aim to review the current knowledge about the BM microbiota, its composition and role in the development of the infant's immune system.
Methods
A search of the medical literature was performed using PubMed database for articles published from database commencement until May 2021. Initial search was carried out using the general search terms: Infant, gut, microbiota, BM, breast milk, immune system, immunity, chronic diseases, vaginally delivered, C-section. Only articles published in English were included. No restrictions were placed on the study design or the type of article. References of the included articles were also reviewed for additional relevant articles.
Results
Human Milk Nutrients
Up to 50% of infections-related death in children aged 6–23 months is thought to be caused by the lack of adequate breastfeeding (19). BM contains sufficient nutrients and bioactive compounds to provide complete nutrition for the developing infant (20). The benefits of BM are numerous and superior psychologically, economically, ecologically and nutritionally (21). WHO and UNICEF recommends exclusive breastfeeding for infants for the first six months to achieve optimal growth and development (22).
followed by continued breastfeeding up to age 2 years and beyond, for prevention of childhood malnutrition and other diseases (Figure 1) (23). The nutritional components of BM are derived from three main sources, some from synthesis in the lactocytes, some from dietary origins, and the rest originate from maternal stores (24).
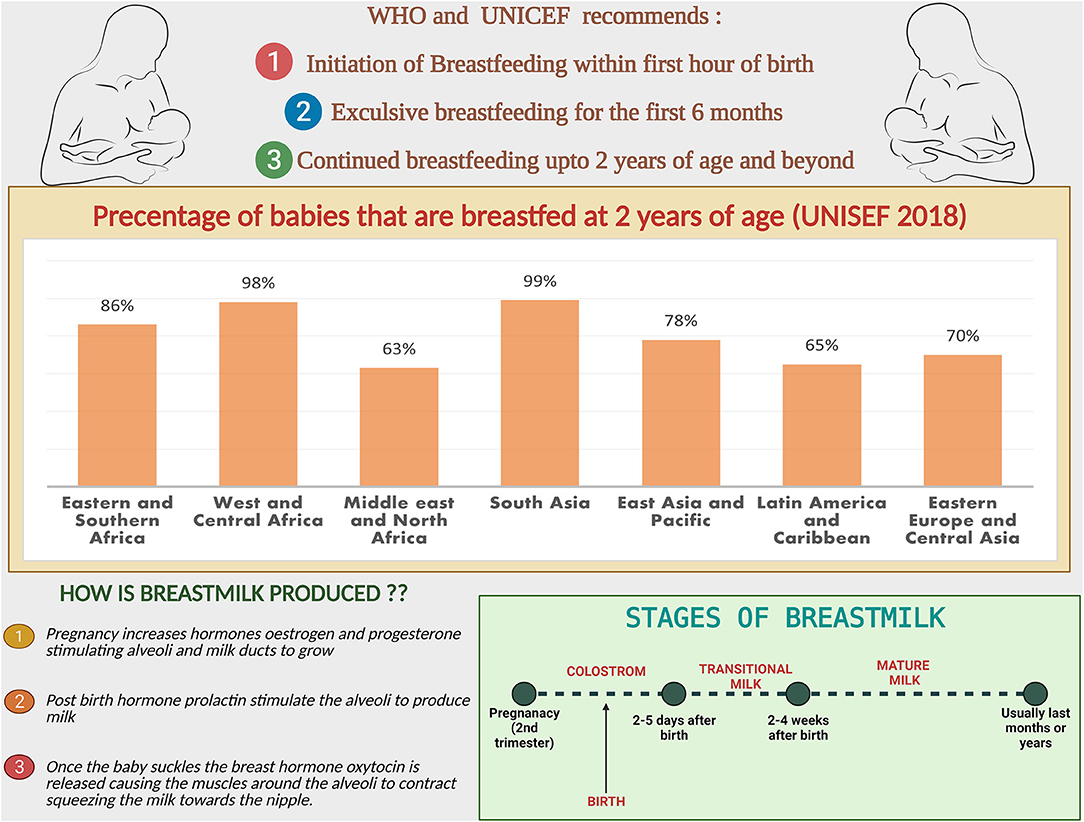
Figure 1. Breastfeeding facts and figures: the recommendations from WHO and UNICEF, comparison of the percentage of babies breastfed at 2 years region wise. Steps and stages of breastmilk production.
Lipids, proteins and carbohydrates are the significant macronutrients found in the BM (25). Macronutrients composition varies with lactation stage, possibly due to adaptation of milk composition to the increased energy demand of the growing infant (26). When compared with mature BM, pre-colostrum milk contains more minerals and protein, but less sugar and fat (27, 28). Another study looked at the macronutrient content of pre-colostrum milk and showed that it has 3.7 g of proteins, 2.9 g of fat and 5.3 g of lactose (29). In comparison to the mature BM macronutrient composition, proteins are estimated to be 0.9 to 1.2 g/dL, with fat at 3.2 to 3.6 g/dL and lactose at 6.7 to 7.8 g/dL (24).
The main source of energy in the BM is fat, and BM fatty acids are known not only as a major energy source, but also as important regulators of development, immune function, and metabolism (30, 31). It is recommended that the mother consumes a well-balanced diet to ensure adequate polyunsaturated fat transfer to human milk.
Lactose is the primary carbohydrate found in milk, accounting for 40% of the baby's energy, it is relatively constant in mature milk and aids minerals and calcium absorption (32, 33). HMOs are lactose-based unconjugated carbohydrates that are considered the third most abundant component of BM after lactose and lipids (34). Oligosaccharides are a group of complex glycans found in the milk of most mammals, but the human milk oligosaccharides profile is thought to be the most diverse with over 200 distinct HMO structures identified in the human BM thus far (35, 36). Given their inhibitory properties, HMOs play an important role in preventing the adhesion of microorganisms to the intestinal mucosa, thereby protecting against the disease-causing organisms in the baby's gastrointestinal tract (4, 37). Some HMOs exhibit antimicrobial and antibiofilm properties against Group B Streptococcus (GBS) (38). GBS is one of the most common pathogens responsible for neonatal infections in full-term newborn infants during the first week of life (39). Humans rely primarily on their gut bacteria, including Bifidobacterium, to digest HMOs due to the lack of enzymes (37, 40). The monosaccharides used as building blocks for HMOs are glucose (Glc), galactose (Gal), N-Acetyl-Glucosamine (GlcNAc), fucose (Fuc), and sialic acid (Neu5Ac) (41). The lactose HMO backbone can also be fucosylated or sialylated to form trisaccharide HMO structures, termed 2′ or 3′-fucosyllactose (2′or and 3′ or 6′-sialyllactose (3′ or 6′SL) respectively (11). HMOs modulate neonatal immunity in the infant gut by binding to the cell surface receptors expressed on epithelial and immune cells (42). HMOs have the potential to mimic viral receptors and block adherence to target cells, thus preventing infection (43).
The protein content of BM is low but highly bioavailable, and it appears to play an important role in the growth of the infant (44). Protein gain in an infant's body is greatest in the first months of life, when protein concentrations in BM are higher than in later stages of lactation (45). In the BM, there are two classes of protein including casein and whey, casein is known to form clots or curds in the stomach; whereas whey remains liquid and is easier to digest (46). Whey accounts for 50 to 80 percent of the protein content in BM, depending on the stage of production (24). There are over 20 different amino acids found in breast milk, such as free amino acids (FAAs) (47). These amino acids play a role in infant immune development. Glutamate and glutamine are major component of the FAAs and support growth of the nervous tissue and intestines (48) followed by Taurine that combines with bile acids to aid in the development of the brain and the eye (48). Additionally BM contain an array of native proteases and protease inhibitors such as carboxypeptidase B2, plasmin, kallikrein, elastase, thrombin, cathepsin D etc. most of which are active and lead to hydrolysis of the milk proteins to release peptides that are relevant to the developing infant (49, 50).
Lactoferrin (Lf) is a multifunctional protein that is found in high concentrations in human milk (51). Lf is an iron-binding glycoprotein that has been linked to a variety of biological functions, including the promotion of cellular proliferation and differentiation, as well as antimicrobial, anti-inflammatory, immunomodulatory, and prebiotic properties (51). Various factors may affect its concentration in BM, such as the stage of lactation, ethnicity, and diet (51). Lactoferrin concentration in BM range from 7 mg/ml in colostrum to 1 mg/ml in mature milk (52). LF binds free iron which is an essential nutrient for the growth of beneficial bacteria such as Lactobacillus and Bifidobacterium (53). This protein has antimicrobial, anti-inflammatory, and immunomodulatory properties that help to maintain homeostasis and control life-threatening diseases in the intestine of neonates (54). Milk is an enriched source of Lf and it also contains lactoferricin (Lfcin), a peptide derived from the Lf N-terminus after gastric digestion that has antimicrobial activity against pathogens (55). Lf and Lfcin inhibit the growth of gut-beneficial microorganisms, but interestingly, both Lf and Lfcin promote the growth of specific probiotic strains such as bifidobacteria and lactobacilli (56). Summary of the main immunological componenets of breastmilk and thei functions have been listed in Table 1.
Nutrients in the BM adapt to needs of the growing baby. In the first few days thick, honey-textured colostrum also called as the “liquid gold” is packed with immunological components that protect the newborn and serves as “baby's first vaccination” (61). The secretory immunoglobulin A (SIgA), present in colostrum coats the internal organs and lining of the digestive tracts preventing it from pathogens. After supercharging the baby's immune-system in the first two or three days, BM increases in volume and changes to transitional milk lasting roughly three to seven days, characyerized by higher levels of proteins, fat as well as increased lactose to provide energy (61). By the end of the first two weeks, mature milk begins to appear bluish is thinner and watery (90% of it is water necessary to keep the infant hydrated), 10% is comprised of carbohydrates, proteins, and fats which are necessary for both growth and energy (61) (Figure 2). It is also worth noting that BM content changes in term and preterm deliveries (62). Preterm BM contains significantly higher concentrations of protein, sodium, chloride, magnesium and iron (63), in addition to higher levels of fat (64–66).
The Breast Milk Microbiota
While initially considered a sterile fluid, several studies concluded that BM is home to diverse microbes known as the human milk microbiome (HMM) (57, 59, 60). According to the Developmental Origins of Health and Disease (DOHaD) hypothesis, early-life environmental exposures can alter fetal and infant programming, resulting in changes in health status (67). Early life microbiota is recognized as a key participant in the DOHaD hypothesis contributing to infant health status in the short and long term (67). Due to the evolving scientific interest in the benefits of the HMM, it is critical to investigate its origin and detailed composition.
Origin and Sources of the Human Milk Microbiota
BM is a source of microbes necessary for the establishment of the oral and gut microbiota in breastfed infants (68). Many hypotheses have been proposed to explain the origin of these microbes in BM, Fernandez et al. proposed “retrograde transfer” of external bacteria and the “entero-mammary pathway” for internal bacterial translocation (69). Recent research has also suggested “oro-mammary translocation” (70, 71).
External bacteria could enter the mammary gland via “retrograde transfer” from sources such as areola skin, infant oral cavity, and/or breast pumps. The reverse flow of milk back into the breast during breastfeeding and pumping may allow microbes to enter and establish in the milk ducts from the infant's mouth (69). The fact that women who use a breast pump and have cracked and/or sore nipples are more likely to develop lactational mastitis (72) supports the retrograde transfer of exogenous microbes.
The “entero-mammary pathway,” on the other hand, occurs when bacteria from the mother's gastrointestinal tract are translocated to the mammary glands via immune cells and colonize the available niche (69). Altered tight junction in the intestinal tract, particularly in the late stages of pregnancy, can predispose to bacterial translocation (73). Vertical transmission of microbes such as bacteria and viruses through breastfeeding (74), the presence of a distinct colostrum microbial community before the start of infant feeding (75), and the isolation of orally administered probiotic strains such as lactobacilli from the BM (76) all support the entero-mammary pathway. A recent study with a single mother-infant pair found intriguing evidence for microbial exchange via the entero-mammary pathway, with the same strain of Bifidobacterium breve found in the mother's gut, her BM, and in the infant's gut (6). This infant was delivered via C-section limiting the possibility of maternal microbe colonization during delivery (6). The authors showed that, while Bifidobacterium breve constituted less than 1% of the maternal rectal sample, it made up to 28% of the BM microbial composition and 68% of the infant's gut microbiota (6). Similarly, comparison of BM and infant stool microbiota in 10 mother–infant pairs from birth to three months of age found 12 predominating 12 core genera: Pseudomonas, Staphylococcus, Streptococcus, Elizabethkingia, Variovorax, Bifidobacterium, Flavobacterium, Lactobacillus, Stenotrophomonas, Brevundimonas, Chryseobacterium, and Enterobacter. The fact that the genera shared by infant feces and human milk samples accounted for 70–88 percent of the total relative abundance in infant fecal samples lends support to vertical transfer of bacteria from BM to human gut (77). Finally, it is possible that microbes in the mother's oral cavity will also translocate to the mammary gland. Several studies have found similarities between maternal oral and milk microbiota, supporting the “oro-mammary translocation” pathway. More research is needed to confirm the extent to which each of the mechanisms described above influences the microbiota load and composition of milk (78).
Composition
Culture-dependent techniques have traditionally been used to confirm the presence of microbes in BM. Next-generation sequencing and omics technology advancements (79) has opened up new avenues for studying the HMM from microbiological and immunological perspectives. This section summarizes the main advances on our understanding of the HMM composition made possible by traditional and refined tools, as well as opportunities to close existing knowledge gaps.
Bacteriome
Exploration of milk microbiota has been made possible through the use of both culture-dependent and culture-independent methods. Culture-dependent methods only target cultivable bacteria, and isolates are highly dependent on the media used, sample storage conditions, and growth conditions. On the other hand, molecular techniques such as denaturing/temperature gradient gel electrophoresis (DGGE), single-strand conformation polymorphism (SSCP), restriction fragment length polymorphism (RFLP), and quantitative polymerase chain reaction (q-PCR) rely on direct extraction of bacterial DNA. High-throughput 16S targeted sequencing or whole genome metagenomics detect DNA from all bacteria in a sample.
Streptococci were isolated from human milk in early 1924 (80) and it was thought that they were colonized through the infant's mouth during suckling (81, 82). McCarthy et al. found S. salivarius to be a common mouth isolate in infants (82). Numerous studies on the microbiology and biochemistry of BM revealed the presence of rapidly growing culturable microorganisms, including Gram-positive species (Staphylococcus, Streptococcus, Corynebacterium, and Propionibacterium) (83, 84). Carroll et al. performed aerobic cultures of 207 samples of drip breast milk collected from 70 mothers, and showed that 82% contained only Staphylococci and Streptococcus viridans, while 17% grew potential pathogens such as Staphylococcus aureus, Enterobacteria or group B Streptococci (85). Other bacterial groups found in BM may require specific conditions or specialized media. For example, Lactobacillus, Lactococcusm and Bifidobacteria have only been isolated from milk after using specific growth media and anaerobic incubations (86–89). It has been reported that over 590 different genera and 1300 bacterial species have been found in BM using various techniques to date (3), but the number of cultivable bacterial species in a given individual at a given time point ranges from 2 to 18 (90).
Noncultural analysis of BM began in the early 2000s with the use of DEGG and 16S rRNA clone library analysis, as well as q-PCR, which confirmed the general composition described by culture studies while highlighting a much broader array of microorganism diversity. These methods have revealed a dominance of Staphylococci, Streptococci, Propionibacteria and Bifidobacteria. Moreover, DNA from other bacterial groups, such as Weissella, Clostridium and Serratia, was also detected (91, 92).
Hunt et al. performed the first deep sequencing analysis on breast milk collected from 16 healthy women (20–40 years of age) nursing infants (93) revealing Streptococcus, Staphylococcus, Serratia, and Corynebacterium as the most abundant genera. The use of next generation sequencing technology has revealed an even more diverse bacterial population, as well as a better understanding of the factors that may influence its composition. A study that used a combination of culture-dependent and culture-independent methods (Sanger sequencing and 454-pyrosequencing) to examine the microbiota of BM from seven lactating women at three different time points, and found Staphylococcus, Streptococcus, Bifidobacterium, Balutia, Brevundimonas, Corynebacterium, Flavobacterium, Propionibacterium, Pseudomonas, Ralstonia, Rothia, and Burkholderia as the most abundant genera (94). Chen et al. discovered five genera of microbiota in milk samples collected from 33 women: Staphylococcus, Streptococcus, Enhydrobacter, Enterococcus, and Rothia (95). Despite the fact that the majority of the above studies sought to identify the BM microbiota of healthy women following birth, differences in the core genera between these studies are evident. Shotgun metagenomics and 454 pyrosequencing have also been used in studies to compare the metagenomes of human milk samples provided by healthy and mastitis-suffering women (96). The healthy core microbiota included the genera Staphylococcus, Streptococcus, Bacteroides, Faecalibacterium, Ruminococcus, Lactobacillus, and Propionibacterium (96). On the other hand, Staphylococcus aureus and Staphylococcus epidermidis dominated the microbiota in the samples collected from the women with acute and subacute mastitis respectively (96). Fungal and protozoa-related reads, as well as archaea and viral related reads, were also detected (96). It is also worth noting that there are significant inter-individual, inter-population, and inter-study variations, thus standardization of samples collection, processing and analysis is required. Table 2 summarizes the list of bacterial species identified in BM using various techniques.
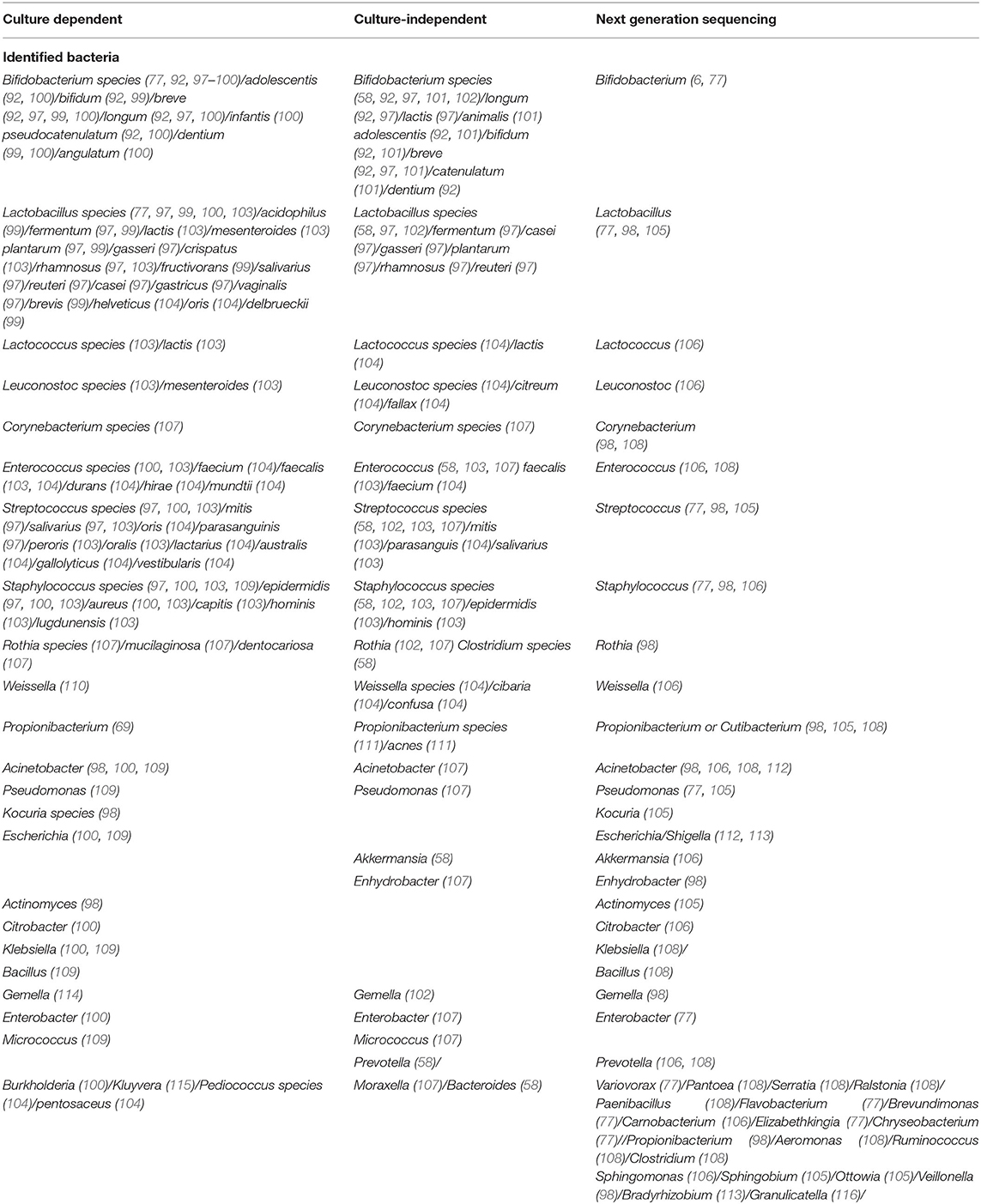
Table 2. Bacterial populations detected in raw human milk using culture-dependent, culture-independent, and next-generation DNA sequencing methods.
BM can also serve as a vehicle for pathogenic bacteria, several outbreaks and case reports of neonatal diseases such as Group B Streptococcus (GBS) (117–120), Bacillus cerus (121, 122) Staphylococcus aureus (MRSA) (123), have been related to tainted breast milk (74).
GBS is a leading cause of neonatal sepsis and meningitis in developed countries (124) with two distinct syndromes: early-onset disease and late-onset disease (125). Several studies have found a link between GBS colonization in breast milk and late-onset sepsis (LOS), but the causality of the link is unclear (126). Heavy colonization and recurrent infection have been observed in newborns whose GBS late-onset sickness is presumed to be caused by breast milk (127). A total of 59 cases have been identified of infants in which contaminated BM was associated with GBS LOD out of these infants 49% were term and 51% preterm (127). GBS strains were detected in 30 infant-mother pairs in the above cases, where the infant's strain was identical to that found in the mother's breast milk (127). In this review we had earlier discussed different possible mechanisum of the origin of breast milk microbiome via retrograde transmission from the infant: similarly GBS colonization of the infant during delivery or after birth can lead to contamination of the maternal mammary ducts, GBS can then multiply in the mammary ducts, resulting in an increase in bacterial concentration in the milk, which re-exposes infants during breastfeeding, resulting in persistent exposure/colonization of both the infant and the mother (117, 128, 129). Another route proposed for GBS contamination of breast milk is transfer from the maternal gastrointestinal system to the mammary glands via lymphatics (129). Septicemia, respiratory tract infection, enterocolitis, hepatitis, endocarditis, endophthalmitis, and encephalitis with brain abscess are other severe diseases that B. cereus can cause, especially in children (130). In two very low birth weight neonates, a cluster of severe intestinal infections due to B. cereus was reported, with pooled breast milk suspected as a source of contamination (131) and BM was also suspected as a possible source of B. cereus infection in three premature neonates admitted to intensive care units in two hospitals in Île-de-France (132). Furthermore, the environment in which milk is expressed, collected, transported, stored, and handled could introduce B. cereus into milk. Contaminated pumps have been discovered as bacterial contamination reservoirs, particularly after being used by numerous mothers and not being cleaned properly. MRSA, on the other hand, has been linked to mastitis (breast infection) and breast abscesses in breastfeeding moms, and it can also be transferred from mother to preterm newborn via contaminated breast milk, even if the mother is not infected (123). Thus adoption of more strict measures such as appropriate handling and bacteriological screening of milk to identify possible pathogens is crutial to control transmission of infection.
Virome
Human milk viruses, including eukaryotic viruses, bacterium-infecting viruses known as bacteriophages, and other viral particles, have been found to be transmitted from mother to infant via breastfeeding (133). Human breastmilk milk viruses play an important role in shaping the infant gut virome and microbiota because bacteriophages can kill bacteria or provide them with potentially beneficial gene functions (133). Breastmilk modulates and supports stepwise assembly of baby viromes beginning at one month, according to a recent study, and breastfeeding was linked to less human viruses in infants' guts than formula-feeding alone (134). Components of breastmilk such as HMOs, lactoferrin, and maternal antibodies also negatively influence the internalization of pathogenic viruses (Figure 3). The majority of the viruses detected in breast milk were bacteriophages from the Myoviridae, Siphoviridae, and Podoviridae families, according to a study of healthy women in the United States (135). Bacteriophages have been the most extensively studied component of the human virome to date, they account for the vast majority (95 percent) of viruses found in human milk and infant stool (135). Evidence suggest that the mother-to-infant virome transmission occurs, as BM and stool viromes from mother-infant pairs shared a significant homology of bacteriophages (135, 136). Recent research on the role of immunosuppression on the bacteriome and virome of breast milk in HIV-positive women discovered that bacterial and viral communities are resilient in breast milk despite immunosuppression (137). There is a definite need for more longitudinal paired mother- infant studies designed to capture the dynamic nature of the milk and infant virome. Additionally, studies are needed to examine effects of breastfeeding duration, maternal health, age, geographic variation, and other factors on the milk and infant virome. Although virus transmission through breast milk is uncommon, viral pathogens such as hepatitis B virus (HBV), hepatitis C virus (HCV), cytomegalovirus (CMV), West Nile virus, human T-cell lymphotropic virus (HTLV), and HIV have been found in breast milk (74). CMV is the most prevalent congenital infection in the United States (138) and it is found in the breast milk of CMV-positive women at rates ranging from 13 to 50% (139–141). Mothers who were CMV-seronegative did not shed virus in their BM. CMV transmission was only identified in infants of seropositive women who excreted CMV and breast-fed their children, according to Vochem et al. (139). For the first time, potential West Nile virus transmission via human milk was reported in September 2002. The Centers for Disease Control and Prevention has been collecting reports of West Nile virus infection in mothers or infants during the nursing period since 2003 (142). Similarly, possible Zika virus transmission through human lactation was investigated, and the World Health Organization concluded that “the benefits of breastfeeding for the infant and mother outweigh any potential risk of Zika virus transmission through breast milk (143).” HBsAg transmission in breast milk of chronically infected mothers was confirmed in 1970 (144). Other studies later discovered not just HBsAg in breast milk, but also HBeAg and HBV DNA. Furthermore, colostral HBsAg and HBeAg titers are positively correlated with the corresponding amount in maternal blood (145). The World Health Organization, on the other hand, believes that a mother's chronic Hepatitis virus infection is not a reason to stop breastfeeding. When a woman is exposed to infectious diseases, pathogenic agents can be transmitted through human milk, and the healthcare professional must make an informed decision about whether or not to stop breastfeeding in each case.
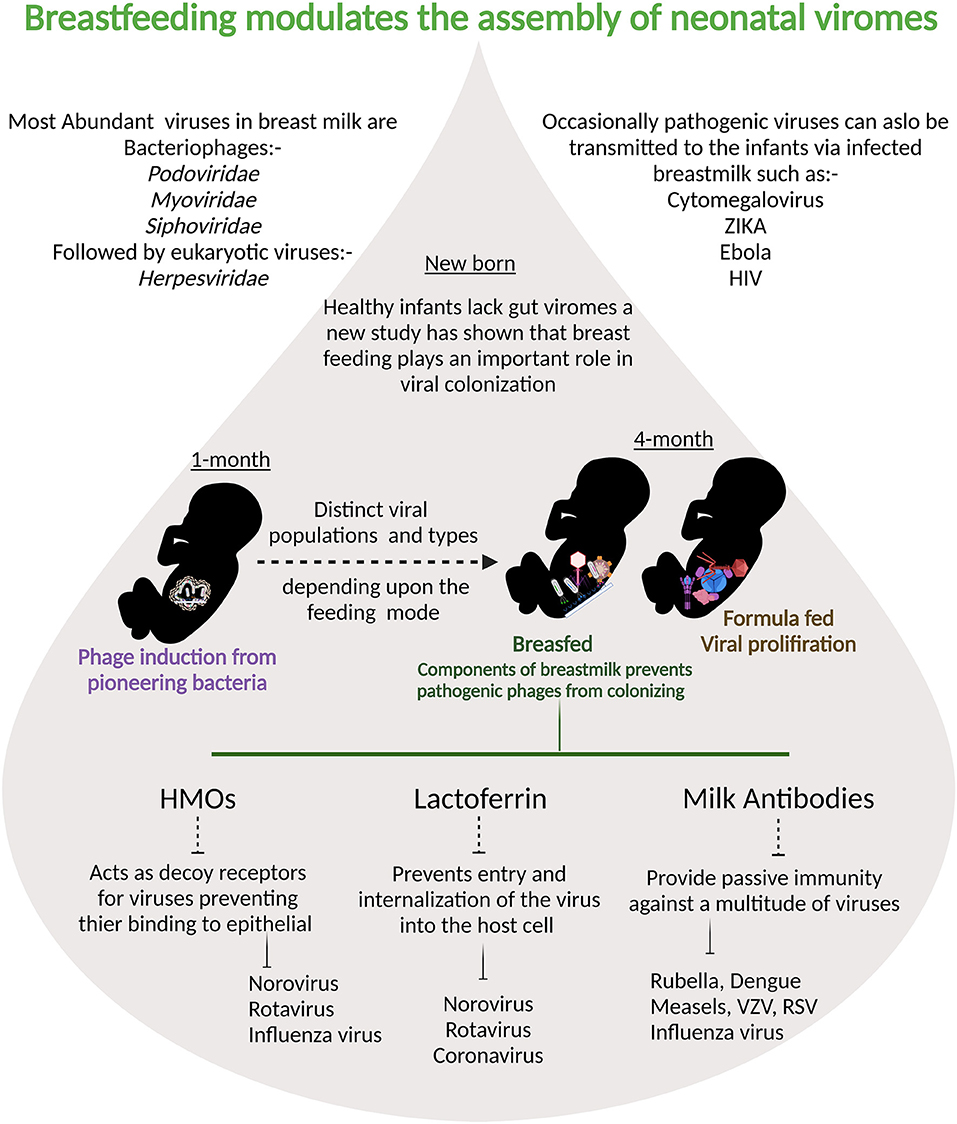
Figure 3. Breastmilk ensures that fewer pathogenic viruses colonize infant intestine: at one month prophages are induced from the pioneering bacteria providing the first population of virus-like particles. By four months of life, multiple human viruses are abundantly detected in stool samples from babies.Viral populations differ depending on the feeding mode and components of breast milk are protective against viral infections.
Mycobiome
Fungi are an important but often overlooked component of the human microbiota (146). Despite the fact that fungal and bacterial colonization occur concurrently during early life (147), most infant microbiota studies have overlooked fungi. Only few studies have have assessed and confirmed the presence of potentially viable fungi in human milk (96, 148–151). According to one metagenomics study of human milk, fungi make up 0.5–2% of the milk microbial community (96). The presence of a variety of fungal species in BM has been investigated using high-throughput sequencing, microscopy and other culture-independent techniques, which revealed the presence of Malassezia, Candida and Saccharomyces as the most common genera (148). In a subsequent study, the authors analyzed BM samples from healthy adult women in South Africa, Finland, China, and Spain with normal weight (20 women per country) to investigate the potential influence of geographical location and mode of delivery on the presence of mycobiota and its composition. They discovered that Malassezia and Davidiella were the most abundant genera across the four countries, regardless of geographical area or mode of delivery (149). Furthermore, BM samples from all participants shared a core mycobiota consisting of Malassezia, Davidiella, Sistotrema, and Penicillium (149). Another study of mother-infant dyads discovered that maternal age, blood type, antibiotics, vaginal delivery, and infant gender were all linked to Candida colonization of the infant, and in a subset, maternal vaginal and rectal samples were identified as potential sources of this taxon (152).
Archeome
Because of advances in sequencing technologies, the human archaeome has recently gained a foothold in microbiota research (153). Methanogenic archaea Methanobrevibacter smithii, is thought to be the main component of human-archaeal-bacterial mutualism, in which it improves energy harvest by consuming end products of microbial fermentation via methanogenesis (154, 155). Vertical transmission of methanogenic Archaea for metabolic phenotype inheritance is a possibility. Until recently, cultivating methanogenic archaea was a laborious, expensive, and time-consuming process. Recent research, however, has used a new antioxidant-based culture system, as well as genome sequencing, to investigate the presence of methanogenic archaea in human colostrum and milk (156). Interestingly, the study found methanogenic archaea in colostrum and human milk. Two species of Methanobrevibacter namely, M. smithii and M. oralis, have been identified by culture and confirmed by genome sequencing (156). These findings pave the way for future research into the mechanisms underlying the transmission of methanogenic archaea as critical commensals to infants via breastfeeding.
Factors Influencing the Composition of Human Milk Microbiota
BM contains the nutritional and immunological elements required for the development of the infant. Aside from these elements, this fluid contains a community of microorganisms known as the microbiota, which is dominated by Staphylococcus, Streptococcus, Lactobacillus, Pseudomonas, Bifidobacterium, Corynebacterium, and Enterococcus (3). The BM microbiota, like many other components, is highly dynamic, and its composition is influenced by both intrinsic and extrinsic factors such as the stage of lactation, the mother's diet, and others.
Stage of Lactation
The composition of the BM microbiota may change during the various stages of lactation. Initially, the bacterial composition of colostrum is more abundant, particularly for the lactic acid bacteria Weisella and Leuconostoc (106) whereas the abundance of Bifidobacterium though low in colostrum increses in the transient milk (106). Several studies suggest that some of these bacteria are essential for the colonization of the infant's gut and the subsequent establishment of its microbiota, which prevents infectious diseases and aids in the maturation of the immune system (3). After ten days, the relative abundance of Methylobacterium, Rothia, and Granulicatella in BM increases (157). In the following months, the lactic acid bacteria remain as the most abundant; however, other taxa suffer variations. Between the first and sixth month, the oropharynx and gut-associated bacteria, Veillonella, Leptotrichia, Prevotella, and Pseudomonas dominate the BM (106).
Method of BM Expression
The methods of BM expression, whether direct from the breast or indirect from other sources, influence the microbial composition of the BM. Direct breastfeeding is associated with a higher abundance of oral cavity-associated genera Gemellaceae, Vogesella, and Nocardioides (68). In contrast, indirect breastfeeding is associated with increased prevalence of potential opportunistic pathogenic families such as Enterobacteriaceae, Stenotrophomonas, and Pseudomonadaceae, while levels of Bifidobacterium decrease (68). Another study found a higher abundance of Bifidobacterium, Ralstonia, and Lactobacillus and a lower prevalence of Staphylococcus and Escherichia/Shigella in women's milk obtained by direct breastfeeding (158). Overall, bacterial diversity and richness decrease, regardless of the milk expression method. In conclusion, direct breastfeeding might allow the acquisition of oral associated microorganisms, whereas indirect breastfeeding could favor the colonization with environment-related microorganisms that could compromise the infant's immune system and increase the risk of some diseases (68).
Lifestyle and Diet of the Mother
Lifestyle factors, particularly diet and antibiotic use, are thought to be important in modulating the composition of the human microbiota. When taken during labor, it has been shown to increase the microbial alpha diversity and richness in BM while decreasing the presence of Bifidobacterium and Lactobacillus (3, 106). On other hand women receiving anti-cancer chemotherapy during breastfeeding showed reduction in Bifidobacterium, Eubacterium, Staphylococcus and Cloacibacterium (159). Furthermore, diet and antibiotic consumption may vary by geographical location, resulting in differences in breast milk microbiota profiles (93, 160).
Women in the United States have lower levels of Lactobacillus and Bifidobacterium than women in Europe (93). Instead, Spanish women have a higher abundance of Bacteroidetes, Propionibacterium, and Pseudomonas, whereas Finnish women have higher levels of Firmicutes and lower levels of Proteobacteria (160). Meanwhile, samples collected from South African and Chinese women have higher levels of Proteobacteria, and Actinobacteria respectively (160). Some studies show that the mother's diet has an effect on the BM microbiota via the entero-mammary pathway (6). During pregnancy and lactation, bacteria from the maternal gastrointestinal tract translocate into the mammary gland, altering the mammary and BM microbiota (6). Thus, as the ingestion of certain nutrients influences the gut microbiota composition, this could also indirectly impact the microbial composition of the BM microbiota (78). A higher intake of fatty acids correlates with a positive relative abundance of Proteobacteria and with a negative relative abundance of Corynebacterium (157). Additionally, the consumption of polyunsaturated/linoleic fatty acids is linked to Bifidobacterium (161). Total carbohydrate and lactose intake inversely correlate with the relative abundance of Firmicutes (157). Certain vitamins and minerals also have a significant impact on the BM microbiota. Vitamin C consumption is associated with the presence of Staphylococcus, whereas thiamine, riboflavin, and folate consumption is associated with the presence of Enterococcus (161). Riboflavin and calcium intake are positively related to Veillonella relative abundance, whereas thiamine, niacin, folate, vitamin B-6, and chromium intake is negatively related to Veillonella relative abundance (157). Probiotic use during pregnancy and lactation also influences the BM microbiota, promoting higher bacterial loads of Lactobacilli and Bifidobacteria, and lower bacterial loads of Staphylococci (162). Prebiotics, such as fructooligosaccharides and galactooligosaccharides, on the other hand, do not cause significant changes in the richness and alpha diversity of the BM microbiota (163).
Maternal Factors
There is a link between BM microbiota changes and women's body mass index (BMI) (106, 157). Mothers with a high BMI have a more homogeneous bacterial composition in the BM. Notably, one study discovered a correlation between having a high BMI and having a higher load of Lactobacillus in colostrum, a greater number of Staphylococcus, and a lower load of Bifidobacterium in mature milk (106). Another study found higher levels of the genera Staphylococcus aureus and lower abundance of Bifidobacterium in BM samples collected from overweight and obese women (106, 157). Several maternal factors are important in the microbial composition of milk. Several changes occur in the BM microbiota during lactation, particularly in the first six months. The method of milk expression is also important; indirect breastfeeding results in lower richness and diversity, as well as the acquisition of potentially opportunistic bacteria. The entero-mammary pathway shapes the microbial composition of BM based on the mother's lifestyle, geographic location, and diet. Furthermore, the body mass index influences the microbial composition of BM.Other major maternal and environmental factors influencing the composition of breastmilk are summarized in Figure 4.
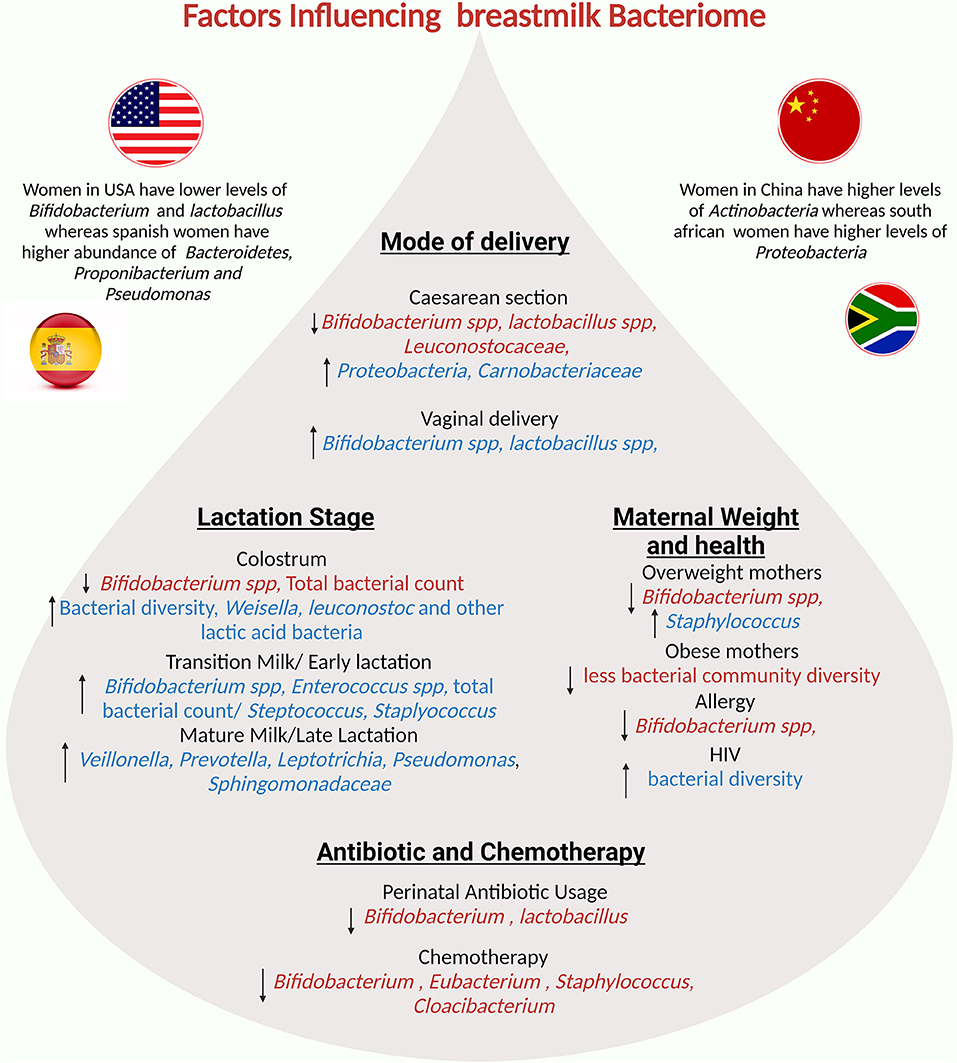
Figure 4. Potential factors influencing the breastmilk bacteriome: mode of delivery, maternal factors, lactation stage, and geographical region influence human milk composition.
Breast Milk as an Immune Educator
The fetus' immune system is immature, and the newborn baby's immune system is considered “naïve” (164). Maternal immunoglobulin (Ig)G crosses the placental barrier to compensate and provide some protection (165, 166). The infant's immune system has limited anti-inflammatory properties after birth, and the passive immunity provided by maternal antibodies begins to fade in the first 6 to 12 months (167). Breastfed infants benefit from additional maternal protection from BM, which supplements the infant's innate immunity (57).
BM is a component of the maternal-mucosal immune system that aids in the development and regulation of both the infant's innate and adaptive immunity (168–172). BM contains various immune-regulatory components including anti-infectious agents [Lf, Lysozymes, secretory IgA (sIgA), CCl28, mucin, beta defensins, etc], anti-inflammatory agents (prostaglandins, cortisol, Interleukin (IL) 10, Tumor growth factor B1 and various antioxidants), immunomodulators 1, 2 (Il-7, IL-2, IL-18, IL-12, IL-4, IL-8 RANTES, erythropoietin, etc), and activated leukocytes (neutrophils, macrophages, and T cells) (169, 171, 172). An inverse relationship exists between the production of many proteins by the mammary gland and those in the infant (173).
Lactoferrin has been shown in animal models to have immunomodulatory properties (174, 175). Lf supplementation has been used in preterm babies to reduce the late onset of sepsis and necrotizing enterocolitis, as well as to protect against fungal infections (176). BM contains a high concentration of sIgA, which can aid the neonate in fighting potential pathogens. It has been demonstrated that sIgA binds pathogens, prevents them from contacting the intestinal epithelial layer, and traps them within the mucin layers without inducing an inflammatory response (177). Aside from sIgA, BM contains IgM, which causes pathogen agglutination, and IgG, which is known to activate phagocytosis and antigen transport to the lamina propria, resulting in B cell activation and thus promoting the infant's adaptive immunity (57).
Lactocytes, mammary stem cells, epithelial cells, and blood cells with approximately 5 × 106 leukocytes are among the cells found in BM. The majority of these leukocytes are neutrophils and macrophages, which aid in the fight against microbial agents. It is now known that breast feeding for 6 months or longer reduces middle ear, lower respiratory tract infections, and allergies (24, 178–180). Toll like receptors (TLRs) such as TLR2, TLR3, TLR5, as well as soluble CD14 and human defensins, which function as pattern recognition receptors, are also found in BM (181). Furthermore, BM contains a population of highly activated switched memory cells primed to secrete antibodies (182).
HMO's absorbed during breast feeding may also play a direct role in postnatal maturation of newborn's immune system in the (183). Infants' guts contain trace amounts of sialylated oligosaccharides (184). These molecules bind to sialic acid-dependent pathogens and inhibit their adhesion to the epithelial cells of newborns and infants (185). Recent reserch has also shown that HMOS may contain tolerogenic factors influencing human DCs and thereby modulating the development of the neonatal immune system (186).
In addition to complementing innate immunity, BM contributes to adaptive immunity by shaping the thymus and promoting T-cell development (187, 188). More than 20 years ago, researchers discovered a link between breast feeding and thymic size, and another group discovered that CD8 and CD4 levels decreased in infants who stopped breastfeeding (164, 187). Another intriguing component of BM is microRNA (miRNA), a highly conserved RNA packaged in exosomes that is known to play a role in regulating immune cell development at the post-transcriptional level (189). They inhibit in vitro production of IL-2 and interferon-γ by stimulated T cells and increase the production of T regulatory cells (190).
Long-Term Benefits of Breast Milk
Breastfeeding has been shown to protect against chronic non-communicable diseases (NCDs) like diabetes mellitus (DM) and cardiovascular disease (191). Breastfeeding has been shown to influence high-density lipoprotein (HDL) and cholesterol levels in adults (192). Several studies suggest that breastfeeding during the early stages of type II diabetes may improve the condition later in life, though the lack of evidence makes this difficult to conclude (193). Breastfeeding can also lower blood pressure by affecting systolic and diastolic blood pressure (194) and children who were breastfed for at least 6 months had lower blood pressure than those who were never breastfed or were breastfed for less than 6 months (192). Furthermore, epidemiological research suggest that prolonged breastfeeding reduces the risk of developing type 1 diabetes mellitus (T1DM), T1DM protection in the mouse model was attributed in part to the expansion of regulatory T cells, which may be dependent on IL-10 and transforming growth factor present in BM (195). Further breastfeeding lowers the risk of obesity allergies, asthma, eczema, Celiac Disease, Type II diabetes, among others later in the life (196).
Conclusion
The BM is a dynamic and complex microecosystem that contains a microbial signature that is transmitted to the newborn baby and is essential for immune system development and education (summarized in Figure 5). The interaction between BM, the intestinal microbiota, and the infant immune system is a developing field, and more research is needed to understand how milk immune factors promote the seeding of beneficial commensals in the gut microbiota and the development of mucosal immunity. HMOs influence mucosal immunity by promoting the growth of commensal enteric bacteria, which compete with enteric bacterial pathogens; however, this interaction may be more complex, requiring a more in-depth understanding. A complete understanding of the BM's multi-directional interaction with those players, as well as its effects on the infant, has yet to be discovered. This will entail the formation of a multidisciplinary team comprised of physicians, lactation consultants, epidemiologists, and research scientists. A multi-omics system biology approach to evaluating BM samples is the way to go.
Author Contributions
AD and SAK: conceptualization. PS and SAK: figures and tables. All authors contributed to the article, writing, review, editing, and approved the submitted version.
Funding
This work was supported by Sidra Medicine Internal Research Fund (No. SDR400075).
Conflict of Interest
The authors declare that the research was conducted in the absence of any commercial or financial relationships that could be construed as a potential conflict of interest.
Publisher's Note
All claims expressed in this article are solely those of the authors and do not necessarily represent those of their affiliated organizations, or those of the publisher, the editors and the reviewers. Any product that may be evaluated in this article, or claim that may be made by its manufacturer, is not guaranteed or endorsed by the publisher.
References
1. Nutrition EC, Agostoni C, Braegger C, Decsi T, Kolacek S, Koletzko B, et al. Breast-feeding: a commentary by the ESPGHAN committee on nutrition. J Pediatr Gastroenterol Nutr. (2009) 49:112–25. doi: 10.1097/MPG.0b013e31819f1e05
2. Guo M. Human Milk Biochemistry and Infant Formula Manufacturing Technology. 1st ed: Woodhead Publishing. (2014). doi: 10.1533/9780857099150.1
3. Zimmermann P, Curtis N. Breast milk microbiota: A review of the factors that influence composition. J Infect. (2020) 81:17–47. doi: 10.1016/j.jinf.2020.01.023
4. Gura T. Nature's first functional food. Science. (2014) 345:747–9. doi: 10.1126/science.345.6198.747
5. Gomez-Gallego C, Kumar H, Garcia-Mantrana I, du Toit E, Suomela JP, Linderborg KM, et al. Breast milk polyamines and microbiota interactions: impact of mode of delivery and geographical location. Ann Nutr Metab. (2017) 70:184–90. doi: 10.1159/000457134
6. Kordy K, Gaufin T, Mwangi M, Li F, Cerini C, Lee DJ, et al. Contributions to human breast milk microbiome and enteromammary transfer of Bifidobacterium breve. PLoS ONE. (2020) 15:e0219633. doi: 10.1371/journal.pone.0219633
7. Penders J, Thijs C, Vink C, Stelma FF, Snijders B, Kummeling I, et al. Factors influencing the composition of the intestinal microbiota in early infancy. Pediatrics. (2006) 118:511–21. doi: 10.1542/peds.2005-2824
8. Gregory KE. Microbiome aspects of perinatal and neonatal health. J Perinat Neonatal Nurs. (2011) 25:158–62. doi: 10.1097/JPN.0b013e3182169346
9. Dominguez-Bello MG, Costello EK, Contreras M, Magris M, Hidalgo G, Fierer N, et al. Delivery mode shapes the acquisition and structure of the initial microbiota across multiple body habitats in newborns. Proc Natl Acad Sci U S A. (2010) 107:11971–5. doi: 10.1073/pnas.1002601107
10. Dunn AB, Jordan S, Baker BJ, Carlson NS. The maternal infant microbiome: considerations for labor and birth. MCN Am J Matern Child Nurs. (2017) 42:318–25. doi: 10.1097/NMC.0000000000000373
11. Thomson P, Medina DA, Garrido D. Human milk oligosaccharides and infant gut bifidobacteria: molecular strategies for their utilization. Food Microbiol. (2018) 75:37–46. doi: 10.1016/j.fm.2017.09.001
12. Thongaram T, Hoeflinger JL, Chow J, Miller MJ. Human milk oligosaccharide consumption by probiotic and human-associated bifidobacteria and lactobacilli. J Dairy Sci. (2017) 100:7825–33. doi: 10.3168/jds.2017-12753
13. Fallani M, Amarri S, Uusijarvi A, Adam R, Khanna S, Aguilera M, et al. Determinants of the human infant intestinal microbiota after the introduction of first complementary foods in infant samples from five European centres. Microbiology. (2011) 157:1385–92. doi: 10.1099/mic.0.042143-0
14. Tamburini S, Shen N, Wu HC, Clemente JC. The microbiome in early life: implications for health outcomes. Nat Med. (2016) 22:713–22. doi: 10.1038/nm.4142
15. Rodriguez JM, Murphy K, Stanton C, Ross RP, Kober OI, Juge N, et al. The composition of the gut microbiota throughout life, with an emphasis on early life. Microb Ecol Health Dis. (2015) 26:26050. doi: 10.3402/mehd.v26.26050
16. Gervassi AL, Horton H. Is infant immunity actively suppressed or immature? Virology. (2014) 2014:1–9. doi: 10.4137/VRT.S12248
17. Edwards KM. Maternal antibodies and infant immune responses to vaccines. Vaccine. (2015) 33:6469–72. doi: 10.1016/j.vaccine.2015.07.085
18. Palmeira P, Carneiro-Sampaio M. Immunology of breast milk. Rev Assoc Med Bras. (2016) 62:584–93. doi: 10.1590/1806-9282.62.06.584
19. Victora CG, Bahl R, Barros AJ, França GV, Horton S, Krasevec J, et al. Breastfeeding in the 21st century: epidemiology, mechanisms, and lifelong effect. Lancet. (2016) 387:475–90. doi: 10.1016/S0140-6736(15)01024-7
20. Lyons KE, Ryan CA, Dempsey EM, Ross RP, Stanton C. Breast Milk, a Source of Beneficial Microbes and Associated Benefits for Infant Health. Nutrients. (2020) 12:1039. doi: 10.3390/nu12041039
21. Erick M. Breast milk is conditionally perfect. Med Hypotheses. (2018) 111:82–9. doi: 10.1016/j.mehy.2017.12.020
22. WHO and UNICEF issue new guidance to promote breastfeeding in health facilities globally [press release]. (2018).
23. Breastfeeding acts as a baby's first vaccine, providing critical protection from diseases and death” - [press release]. (2021).
24. Ballard O, Morrow AL. Human milk composition: nutrients and bioactive factors. Pediatr Clin North Am. (2013) 60:49–74. doi: 10.1016/j.pcl.2012.10.002
25. Samuel TM, Zhou Q, Giuffrida F, Munblit D, Verhasselt V, Thakkar SK. Nutritional and non-nutritional composition of human milk is modulated by maternal, infant, and methodological factors. Front Nutr. (2020) 7:576133. doi: 10.3389/fnut.2020.576133
26. Czosnykowska-Łukacka M, Królak-Olejnik B, Orczyk-Pawiłowicz M. Breast milk macronutrient components in prolonged lactation. Nutrients. (2018) 10:1893. doi: 10.3390/nu10121893
27. Ruiz L, Bacigalupe R, García-Carral C, Boix-Amoros A, Argüello H, Silva CB, et al. Microbiota of human precolostrum and its potential role as a source of bacteria to the infant mouth. Sci Rep. (2019) 9:8435. doi: 10.1038/s41598-019-42514-1
29. Godhia DML, Patel N. Colostrum – its composition, benefits as a nutraceutical – a review. Curr Res Nutr Food Sci J. (2013) 1:37–47. doi: 10.12944/CRNFSJ.1.1.04
30. Demmelmair H, Koletzko B. Lipids in human milk. Best Pract Res Clin Endocrinol Metab. (2018) 32:57–68. doi: 10.1016/j.beem.2017.11.002
31. Ramiro-Cortijo D, Singh P, Liu Y, Medina-Morales E, Yakah W, Freedman SD, et al. Breast milk lipids and fatty acids in regulating neonatal intestinal development and protecting against intestinal injury. Nutrients. (2020) 12:534. doi: 10.3390/nu12020534
32. Gambelli L. Milk and its sugar-lactose: a picture of evaluation methodologies. Beverages. (2017) 3:35. doi: 10.3390/beverages3030035
33. Martin CR, Ling P-R, Blackburn GL. Review of infant feeding: key features of breast milk and infant formula. Nutrients. (2016) 8:279. doi: 10.3390/nu8050279
34. Hegar B, Wibowo Y, Basrowi RW, Ranuh RG, Sudarmo SM, Munasir Z, et al. The role of two human milk oligosaccharides, 2'-fucosyllactose and lacto-N-neotetraose, in infant nutrition. Pediatr Gastroenterol Hepatol Nutr. (2019) 22:330–40. doi: 10.5223/pghn.2019.22.4.330
35. Ogra PL. Summary on immunology of milk and lactation. Nestle Nutr Inst Workshop Ser. (2020) 94:59–64. doi: 10.1159/000505425
36. Ogra PL. Immunology of human milk and lactation: historical overview. Nestle Nutr Inst Workshop Ser. (2020) 94:11–26. doi: 10.1159/000505211
37. Plaza-Díaz J, Fontana L, Gil A. Human milk oligosaccharides and immune system development. Nutrients. (2018) 10:1038. doi: 10.3390/nu10081038
38. Ackerman DL, Doster RS, Weitkamp J-H, Aronoff DM, Gaddy JA, Townsend SD. Human milk oligosaccharides exhibit antimicrobial and antibiofilm properties against group B streptococcus. ACS Infect Dis. (2017) 3:595–605. doi: 10.1021/acsinfecdis.7b00064
39. Borghesi A, Stronati M, Fellay J. Neonatal Group B Streptococcal Disease in Otherwise Healthy Infants: Failure of Specific Neonatal Immune Responses. Front Immunol. (2017) 8:215. doi: 10.3389/fimmu.2017.00215
40. Turroni F, Milani C, Duranti S, Mahony J, van Sinderen D, Ventura M. Glycan Utilization and Cross-Feeding Activities by Bifidobacteria. Trends Microbiol. (2018) 26:339–50. doi: 10.1016/j.tim.2017.10.001
41. Ayechu-Muruzabal V, van Stigt AH, Mank M, Willemsen LEM, Stahl B, Garssen J, et al. Diversity of human milk oligosaccharides and effects on early life immune development. Front Pediatr. (2018) 6:239. doi: 10.3389/fped.2018.00239
42. Triantis V, Bode L, van Neerven RJJ. Immunological effects of human milk oligosaccharides. Front Pediatr. (2018) 6:190. doi: 10.3389/fped.2018.00190
43. Morozov V, Hansman G, Hanisch F-G, Schroten H, Kunz C. Human milk oligosaccharides as promising antivirals. Mol Nutr Food Res. (2018) 62:e1700679. doi: 10.1002/mnfr.201700679
44. Luque V, Closa-Monasterolo R, Escribano J, Ferré N. Early programming by protein intake: the effect of protein on adiposity development and the growth and functionality of vital organs. Nutr Metab Insights. (2015) 8:49–56. doi: 10.4137/NMI.S29525
45. Haschke F, Haiden N, Thakkar SK. Nutritive and bioactive proteins in breastmilk. Ann Nutr Metab. (2016) 69:17–26. doi: 10.1159/000452820
46. Roy D, Ye A, Moughan PJ, Singh H. Composition, structure, and digestive dynamics of milk from different species-a review. Front Nutr. (2020) 7:577759. doi: 10.3389/fnut.2020.577759
47. van Sadelhoff JHJ, Wiertsema SP, Garssen J, Hogenkamp A. Free amino acids in human milk: a potential role for glutamine and glutamate in the protection against neonatal allergies and infections. Front Immunol. (2020) 11:1007. doi: 10.3389/fimmu.2020.01007
48. Dror DK, Allen LH. Overview of nutrients in human milk. Adv Nutr. (2018) 9:278S−94S. doi: 10.1093/advances/nmy022
49. Dallas DC, Murray NM, Gan J. Proteolytic systems in milk: perspectives on the evolutionary function within the mammary gland and the infant. J Mammary Gland Biol Neoplasia. (2015) 20:133–47. doi: 10.1007/s10911-015-9334-3
50. Dallas DC, German JB. Enzymes in human milk. Intest Microb. (2017) 88:129–36. doi: 10.1159/000455250
51. Yang Z, Jiang R, Chen Q, Wang J, Duan Y, Pang X, et al. Concentration of lactoferrin in human milk and its variation during lactation in different chinese populations. Nutrients. (2018) 10:1235. doi: 10.3390/nu10091235
52. Szewczyk B, Summers DF. Fluorescent staining of proteins transferred to nitrocellulose allowing for subsequent probing with antisera. Anal Biochem. (1987) 164:303–6. doi: 10.1016/0003-2697(87)90497-0
53. Anghel L, Radulescu A, Erhan RV. Structural aspects of human lactoferrin in the iron-binding process studied by molecular dynamics and small-angle neutron scattering. Eur Phys J E Soft Matter. (2018) 41:109. doi: 10.1140/epje/i2018-11720-x
54. Giansanti F, Panella G, Leboffe L, Antonini G. Lactoferrin from milk: nutraceutical and pharmacological properties. Pharmaceuticals. (2016) 9:61. doi: 10.3390/ph9040061
55. Park YW, Nam MS. Bioactive peptides in milk and dairy products: a review. Korean J Food Sci Anim Resour. (2015) 35:831–40. doi: 10.5851/kosfa.2015.35.6.831
56. Vega-Bautista A, de la Garza M, Carrero JC, Campos-Rodríguez R, Godínez-Victoria M, Drago-Serrano ME. The Impact of Lactoferrin on the Growth of Intestinal Inhabitant Bacteria. Int J Molec Sci. (2019) 20:4707. doi: 10.3390/ijms20194707
57. Cacho NT, Lawrence RM. Innate immunity and breast milk. Front Immunol. (2017) 8:584. doi: 10.3389/fimmu.2017.00584
58. Aakko J, Kumar H, Rautava S, Wise A, Autran C, Bode L, et al. Human milk oligosaccharide categories define the microbiota composition in human colostrum. Benef Microbes. (2017) 8:563–7. doi: 10.3920/BM2016.0185
59. Hill DR, Newburg DS. Clinical applications of bioactive milk components. Nutr Rev. (2015) 73:463–76. doi: 10.1093/nutrit/nuv009
60. Gila-Diaz A, Arribas SM, Algara A, Martín-Cabrejas MA, López de Pablo Á L, Sáenz de Pipaón M, et al. A review of bioactive factors in human breastmilk: a focus on prematurity. Nutrients. (2019) 11:1307. doi: 10.3390/nu11061307
61. Andreas NJ, Kampmann B, Mehring Le-Doare K. Human breast milk: a review on its composition and bioactivity. Early Hum Dev. (2015) 91:629–35. doi: 10.1016/j.earlhumdev.2015.08.013
62. Bhatia J. Human milk and the premature infant. Ann Nutr Metab. (2013) 62:8–14. doi: 10.1159/000351537
63. Lemons JA, Moye L, Hall D, Simmons M. Differences in the composition of preterm and term human milk during early lactation. Pediatr Res. (1982) 16:113–7. doi: 10.1203/00006450-198202000-00007
64. Narang APS, Bains HS, Kansal S, Singh D. Serial composition of human milk in preterm and term mothers. Indian J Clin Biochem. (2006) 21:89. doi: 10.1007/BF02913072
65. Underwood MA. Human milk for the premature infant. Pediatr Clin North Am. (2013) 60:189–207. doi: 10.1016/j.pcl.2012.09.008
66. Bauer J, Gerss J. Longitudinal analysis of macronutrients and minerals in human milk produced by mothers of preterm infants. Clinical Nutrition. (2011) 30:215–20. doi: 10.1016/j.clnu.2010.08.003
67. Sarkar A, Yoo JY, Valeria Ozorio Dutra S, Morgan KH, Groer M. The association between early-life gut microbiota and long-term health and diseases. J Clin Med. (2021) 10:459. doi: 10.3390/jcm10030459
68. Moossavi S, Sepehri S, Robertson B, Bode L, Goruk S, Field CJ, et al. Composition and variation of the human milk microbiota are influenced by maternal and early-life factors. Cell Host Microbe. (2019) 25:324–35e4. doi: 10.1016/j.chom.2019.01.011
69. Fernández L, Langa S, Martín V, Maldonado A, Jiménez E, Martín R, et al. The human milk microbiota: origin and potential roles in health and disease. Pharmacol Res. (2013) 69:1–10. doi: 10.1016/j.phrs.2012.09.001
70. Drell T, Štšepetova J, Simm J, Rull K, Aleksejeva A, Antson A, et al. The influence of different maternal microbial communities on the development of infant gut and oral microbiota. Sci Rep. (2017) 7:9940. doi: 10.1038/s41598-017-09278-y
71. Williams JE, Carrothers JM, Lackey KA, Beatty NF, Brooker SL, Peterson HK, et al. Strong multivariate relations exist among milk, oral, and fecal microbiomes in mother-infant dyads during the first six months postpartum. J Nutr. (2019) 149:902–14. doi: 10.1093/jn/nxy299
72. Johns HM, Forster DA, Amir LH, McLachlan HL. Prevalence and outcomes of breast milk expressing in women with healthy term infants: a systematic review. BMC Pregnancy Childbirth. (2013) 13:212. doi: 10.1186/1471-2393-13-212
73. Miranda J, Martín-Tapia D, Valdespino-Vázquez Y, Alarcón L, Espejel-Nuñez A, Guzmán-Huerta M, et al. Syncytiotrophoblast of placentae from women with zika virus infection has altered tight junction protein expression and increased paracellular permeability. Cells. (2019) 8:1174. doi: 10.3390/cells8101174
74. Lawrence RM, Lawrence RA. Breast milk and infection. Clin Perinatol. (2004) 31:501–28. doi: 10.1016/j.clp.2004.03.019
75. Damaceno QS, Souza JP, Nicoli JR, Paula RL, Assis GB, Figueiredo HC, et al. Evaluation of potential probiotics isolated from human milk and colostrum. Probiot Antimic Proteins. (2017) 9:371–9. doi: 10.1007/s12602-017-9270-1
76. Treven P, Mrak V, Bogovič Matijašić B, Horvat S, Rogelj I. Administration of probiotics Lactobacillus rhamnosus GG and Lactobacillus gasseri K7 during pregnancy and lactation changes mouse mesenteric lymph nodes and mammary gland microbiota. J Dairy Sci. (2015) 98:2114–28. doi: 10.3168/jds.2014-8519
77. Murphy K, Curley D, O'Callaghan TF, O'Shea C-A, Dempsey EM, O'Toole PW, et al. The composition of human milk and infant faecal microbiota over the first three months of life: a pilot study. Sci Rep. (2017) 7:1–10. doi: 10.1038/srep40597
78. Moossavi S, Azad MB. Origins of human milk microbiota: new evidence and arising questions. Gut Microbes. (2020) 12:1667722. doi: 10.1080/19490976.2019.1667722
79. Rawat A, Engelthaler DM, Driebe EM, Keim P, Foster JT. MetaGeniE: characterizing human clinical samples using deep metagenomic sequencing. PLoS ONE. (2014) 9:e110915. doi: 10.1371/journal.pone.0110915
80. Ayers SH, Johnson WT. Jr., Mudge CS. Streptococci of souring milk with special reference to streptococcus lactis*: Studies of the streptococci IX. J Infect Dis. (1924) 34:29–48. doi: 10.1093/infdis/34.1.29
81. Wysham DN, Mulhern ME, Navarre GC, La Veck GD, Kennan AL, Giedt WR. Staphylococcal infections in an obstetric unit. II Epidemiologic studies of puerperal mastitis. N Engl J Med. (1957) 257:304–6. doi: 10.1056/NEJM195708152570702
82. McCarthy C, Snyder ML, Parker rbthe indigenous oral flora of mani, the newborn to the. 1-year-old infant. Arch Oral Biol. (1965) 10:61–70. doi: 10.1016/0003-9969(65)90058-0
83. Chen Z., Pan W-g, Xian W-y, Cheng H, Zheng J-x, Hu Q-h, et al. Identification of infantile diarrhea caused by breast milk-transmitted Staphylococcus aureus infection. Curr Microbiol. (2016) 73:498–502. doi: 10.1007/s00284-016-1088-7
84. Williamson S, Hewitt JH, Finucane E, Gamsu HR. Organisation of bank of raw and pasteurised human milk for neonatal intensive care. Br Med J. (1978) 1:393–6. doi: 10.1136/bmj.1.6110.393
85. Carroll L, Davies DP, Osman M, McNeish AS. Bacteriological criteria for feeding raw breast-milk to babies on neonatal units. Lancet. (1979) 314:732–3. doi: 10.1016/S0140-6736(79)90654-8
86. Martín R, Langa S, Reviriego C, Jimínez E, Marín ML, Xaus J, et al. Human milk is a source of lactic acid bacteria for the infant gut. J Pediatr. (2003) 143:754–8. doi: 10.1016/j.jpeds.2003.09.028
87. Abrahamsson TR, Sinkiewicz G, Jakobsson T, Fredrikson M, Björkstén B. Probiotic lactobacilli in breast milk and infant stool in relation to oral intake during the first year of life. J Pediatr Gastroenterol Nutr. (2009) 49:349–54. doi: 10.1097/MPG.0b013e31818f091b
88. Arboleya S, Ruas-Madiedo P, Margolles A, Solís G, Salminen S, de Los Reyes-Gavilán CG, et al. Characterization and in vitro properties of potentially probiotic Bifidobacterium strains isolated from breast-milk. Int J Food Microbiol. (2011) 149:28–36. doi: 10.1016/j.ijfoodmicro.2010.10.036
89. Arboleya S, Binetti A, Salazar N, Fernández N, Solís G, Hernández-Barranco A, et al. Establishment and development of intestinal microbiota in preterm neonates. FEMS Microbiol Ecol. (2012) 79:763–72. doi: 10.1111/j.1574-6941.2011.01261.x
90. Martín V, Manes-Lazaro R, Rodríguez JM, Maldonado-Barragan A. Streptococcus lactarius sp. nov, isolated from breast milk of healthy women. Int J Syst Evolution Microbiol. (2011) 61:1048–52. doi: 10.1099/ijs.0.021642-0
91. Martin R, Langa S, Reviriego C, Jimenez E, Marin ML, Olivares M, et al. The commensal microflora of human milk: new perspective for food bacteriotherapy and probiotics. Trends Food Sci Technol. (2004) 15:121–7. doi: 10.1016/j.tifs.2003.09.010
92. Martín R, Jiménez E, Heilig H, Fernández L, Marín ML, Zoetendal EG, et al. Isolation of bifidobacteria from breast milk and assessment of the bifidobacterial population by PCR-denaturing gradient gel electrophoresis and quantitative real-time PCR. Appl Environ Microbiol. (2009) 75:965–9. doi: 10.1128/AEM.02063-08
93. Hunt KM, Foster JA, Forney LJ, Schütte UME, Beck DL, Abdo Z, et al. Characterization of the diversity and temporal stability of bacterial communities in human milk. PLoS ONE. (2011) 6:e21313. doi: 10.1371/journal.pone.0021313
94. Jost T, Lacroix C, Braegger C, Chassard C. Assessment of bacterial diversity in breast milk using culture-dependent and culture-independent approaches. Br J Nutr. (2013) 110:1253–62. doi: 10.1017/S0007114513000597
95. Chen PW, Lin YL, Huang MS. Profiles of commensal and opportunistic bacteria in human milk from healthy donors in Taiwan. J Food Drug Anal. (2018) 26:1235–44. doi: 10.1016/j.jfda.2018.03.004
96. Jiménez E, de Andrés J, Manrique M, Pareja-Tobes P, Tobes R, Martínez-Blanch JF, et al. Metagenomic analysis of milk of healthy and mastitis-suffering women. J Hum Lact. (2015) 31:406–15. doi: 10.1177/0890334415585078
97. Soto A, Martín V, Jiménez E, Mader I, Rodríguez JM, Fernández L. Lactobacilli and bifidobacteria in human breast milk: influence of antibiotherapy and other host and clinical factors. J Pediatr Gastroenterol Nutr. (2014) 59:78–88. doi: 10.1097/MPG.0000000000000347
98. Treven P, Mahnič A, Rupnik M, Golob M, Pirš T, Matijašić BB, et al. Evaluation of human milk microbiota by 16S rRNA gene next-generation sequencing (NGS) and Cultivation/MALDI-TOF mass spectrometry identification. Front Microbiol. (2019) 10:2612. doi: 10.3389/fmicb.2019.02612
99. Sallam M, Wali I, Attia A, Mehanna N. Isolation of lactobacilli and bifidobacteria species from human breast milk. Egypt J Med Microbiol. (2015) 24:69–73. doi: 10.12816/0024931
100. Jiménez E, Delgado S, Maldonado A, Arroyo R, Albújar M, García N, et al. Staphylococcus epidermidis: A differential trait of the fecal microbiota of breast-fed infants. BMC Microbiol. (2008) 8:143. doi: 10.1186/1471-2180-8-143
101. Gueimonde M, Laitinen K, Salminen S, Isolauri E. Breast milk: a source of bifidobacteria for infant gut development and maturation? Neonatology. (2007) 92:64–6. doi: 10.1159/000100088
102. González R, Mandomando I, Fumadó V, Sacoor C, Macete E, Alonso PL, et al. Breast milk and gut microbiota in african mothers and infants from an area of high HIV prevalence. PLoS ONE. (2013) 8:e80299. doi: 10.1371/journal.pone.0080299
103. Heikkilä MP, Saris PE. Inhibition of Staphylococcus aureus by the commensal bacteria of human milk. J Appl Microbiol. (2003) 95:471–8. doi: 10.1046/j.1365-2672.2003.02002.x
104. Jeurink P., van bergenhenegouwen J, Jiménez E, Knippels L, Fernández L, Garssen J, et al. Human milk: a source of more life than we imagine. Benef Microbes. (2012) 4:17–30. doi: 10.3920/BM2012.0040
105. Gonzalez E, Brereton NJB, Li C, Lopez Leyva L, Solomons NW, Agellon LB, et al. Distinct changes occur in the human breast milk microbiome between early and established lactation in breastfeeding guatemalan mothers. Front Microbiol. (2021) 12:557180. doi: 10.3389/fmicb.2021.557180
106. Cabrera-Rubio R, Collado MC, Laitinen K, Salminen S, Isolauri E, Mira A. The human milk microbiome changes over lactation and is shaped by maternal weight and mode of delivery. Am J Clin Nutr. (2012) 96:544–51. doi: 10.3945/ajcn.112.037382
107. Huang MS, Cheng CC, Tseng SY, Lin YL, Lo HM, Chen PW. Most commensally bacterial strains in human milk of healthy mothers display multiple antibiotic resistance. Microbiologyopen. (2019) 8:e00618. doi: 10.1002/mbo3.618
108. Patel SH, Vaidya YH, Patel RJ, Pandit RJ, Joshi CG, Kunjadiya AP. Culture independent assessment of human milk microbial community in lactational mastitis. Sci Rep. (2017) 7:7804. doi: 10.1038/s41598-017-08451-7
109. Dahaban NM, Romli MF, Roslan NR, Kong SS, Cheah FC. Bacteria in expressed breastmilk from mothers of premature infants and maternal hygienic status. Breastfeed Med. (2013) 8:422–3. doi: 10.1089/bfm.2012.0109
110. Reis NA, Saraiva MA, Duarte EA, de Carvalho EA, Vieira BB, Evangelista-Barreto NS. Probiotic properties of lactic acid bacteria isolated from human milk. J Appl Microbiol. (2016) 121:811–20. doi: 10.1111/jam.13173
111. Martín R, Heilig HG, Zoetendal EG, Jiménez E, Fernández L, Smidt H, et al. Cultivation-independent assessment of the bacterial diversity of breast milk among healthy women. Res Microbiol. (2007) 158:31–7. doi: 10.1016/j.resmic.2006.11.004
112. Corona-Cervantes K, García-González I, Villalobos-Flores LE, Hernández-Quiroz F, Piña-Escobedo A, Hoyo-Vadillo C, et al. Human milk microbiota associated with early colonization of the neonatal gut in Mexican newborns. PeerJ. (2020) 8:e9205. doi: 10.7717/peerj.9205
113. Lopez Leyva L, Brereton NJB, Koski KG. Emerging frontiers in human milk microbiome research and suggested primers for 16S rRNA gene analysis. Comput Struct Biotechnol J. (2021) 19:121–33. doi: 10.1016/j.csbj.2020.11.057
114. Delgado S, Arroyo R, Martin R, Rodríguez J, PCR-DGGE. assessment of the bacterial diversity of breast milk in women with lactational infectious mastitis. BMC Infect Dis. (2008) 8:51. doi: 10.1186/1471-2334-8-51
115. Chen PW, Tseng SY, Huang MS. Antibiotic susceptibility of commensal bacteria from human milk. Curr Microbiol. (2016) 72:113–9. doi: 10.1007/s00284-015-0925-4
116. Simpson MR, Avershina E, Storrø O, Johnsen R, Rudi K, Øien T. Breastfeeding-associated microbiota in human milk following supplementation with Lactobacillus rhamnosus GG, Lactobacillus acidophilus La-5, and Bifidobacterium animalis ssp. lactis Bb-12 J Dairy Sci. (2018) 101:889–99. doi: 10.3168/jds.2017-13411
117. Byrne PA, Miller C, Justus K. Neonatal group B streptococcal infection related to breast milk. Breastfeed Med. (2006) 1:263–70. doi: 10.1089/bfm.2006.1.263
118. Kenny JF, Zedd AJ. Recurrent group B streptococcal disease in an infant associated with the ingestion of infected mother's milk. J Pediatr. (1977) 91:158–9. doi: 10.1016/S0022-3476(77)80473-3
119. Olver WJ, Bond DW, Boswell TC, Watkin SL. Neonatal group B streptococcal disease associated with infected breast milk. Arch Dis Childh Fetal Neonatal Ed. (2000) 83:F48–F9. doi: 10.1136/fn.83.1.F48
120. Wang L-Y, Chen C-T, Liu W-H, Wang Y-H. Recurrent neonatal group B streptococcal disease associated with infected breast milk. Clin Pediatr. (2007) 46:547–9. doi: 10.1177/0009922807299467
121. Lewin A, Delage G, Bernier F, Germain M. Banked human milk and quantitative risk assessment of bacillus cereus infection in premature infants: a simulation study. Canad J Infect Dis Med Microbiol. (2019) 2019:1–8. doi: 10.1155/2019/6348281
122. Cormontagne D, Rigourd V, Vidic J, Rizzotto F, Bille E, Ramarao N. Bacillus cereus induces severe infections in preterm neonates: implication at the hospital and human milk bank level. Toxins. (2021) 13:123. doi: 10.3390/toxins13020123
123. Behari P, Englund J, Alcasid G, Garcia-Houchins S, Weber SG. Transmission of methicillin-resistant Staphylococcus aureus to preterm infants through breast milk. Infect Control Hosp Epidemiol. (2004) 25:778–80. doi: 10.1086/502476
124. Cossey V, Vanhole C, Eerdekens A, Rayyan M, Fieuws S, Schuermans A. Pasteurization of mother's own milk for preterm infants does not reduce the incidence of late-onset sepsis. Neonatology. (2013) 103:170–6. doi: 10.1159/000345419
125. Berardi A, Cattelani C, Creti R, Berner R, Pietrangiolillo Z, Margarit I, et al. Group B streptococcal infections in the newborn infant and the potential value of maternal vaccination. Expert Rev Anti Infect Ther. (2015) 13:1387–99. doi: 10.1586/14787210.2015.1079126
126. Nicolini G, Borellini M, Loizzo V, Creti R, Memo L, Berardi A. Group B streptococcus late-onset disease,contaminated breast milk and mothers persistently GBS negative: report of 3cases. BMC Pediatr. (2018) 18:214. doi: 10.1186/s12887-018-1192-x
127. Zimmermann P, Gwee A, Curtis N. The controversial role of breast milk in GBS late-onset disease. J Infect. (2017) 74:S34–40. doi: 10.1016/S0163-4453(17)30189-5
128. Bingen E, Denamur E, Lambert-Zechovsky N, Aujard Y, Brahimi N, Geslin P, et al. Analysis of DNA restriction fragment length polymorphism extends the evidence for breast milk transmission in Streptococcus agalactiae late-onset neonatal infection. J Infect Dis. (1992) 165:569–73. doi: 10.1093/infdis/165.3.569
129. Bertini G, Dani C. Group B streptococcal late-onset sepsis with submandibular phlegmon in a premature infant after beginning of breast-feeding. J Matern Fetal Neonatal Med. (2008) 21:213–5. doi: 10.1080/14767050801924886
130. Lequin MH, Vermeulen JR, Van Elburg RM, Barkhof F, Kornelisse RF, Swarte R, et al. Bacillus cereus meningoencephalitis in preterm infants: neuroimaging characteristics. Am J Neuroradiol. (2005) 26:2137–43.
131. Decousser J-W, Ramarao N, Duport C, Dorval M, Bourgeois-Nicolaos N, Guinebretière M-H, et al. Bacillus cereus and severe intestinal infections in preterm neonates: putative role of pooled breast milk. Am J Infect Control. (2013) 41:918–21. doi: 10.1016/j.ajic.2013.01.043
132. Fournier S, Faraut-Derouin V, Casetta A, Frange P, Doit C, Fortineau N, et al. Bactériémies à Bacillus cereus en réanimation néonatale à l'ap-hp en 2016. Bull Epidémiol Hebdomadaire-BEH. (2018) 25:536–40.
133. Mohandas S, Pannaraj PS. Beyond the bacterial microbiome: virome of human milk and effects on the developing infant. Nestle Nutr Inst Workshop Ser. (2020) 94:86–93. doi: 10.1159/000504997
134. Liang G, Zhao C, Zhang H, Mattei L, Sherrill-Mix S, Bittinger K, et al. The stepwise assembly of the neonatal virome is modulated by breastfeeding. Nature. (2020) 581:470–4. doi: 10.1038/s41586-020-2192-1
135. Pannaraj P, Ly M, Cerini C, Saavedra M, Aldrovandi G, Saboory A, et al. Shared and distinct features of human milk and infant stool viromes. Front Microbiol. (2018) 9:1162. doi: 10.3389/fmicb.2018.01162
136. Duranti S, Lugli GA, Mancabelli L, Armanini F, Turroni F, James K, et al. Maternal inheritance of bifidobacterial communities and bifidophages in infants through vertical transmission. Microbiome. (2017) 5:1–13. doi: 10.1186/s40168-017-0282-6
137. Maqsood R, Reus JB, Wu LI, Holland LA, Nduati R, Mbori-Ngacha D, et al. Breast milk virome and bacterial microbiome resilience in kenyan women living with HIV. mSystems. (2021) 6:e01079–20. doi: 10.1128/mSystems.01079-20
139. Vochem M, Hamprecht K, Jahn G, Speer CP. Transmission of cytomegalovirus to preterm infants through breast milk. Pediatr Infect Dis J. (1998) 17:53–8. doi: 10.1097/00006454-199801000-00012
140. Hamprecht K, Vochem M, Baumeister A, Boniek M, Speer CP, Jahn G. Detection of cytomegaloviral DNA in human milk cells and cell free milk whey by nested PCR. J Virol Methods. (1998) 70:167–76. doi: 10.1016/S0166-0934(97)00179-1
141. Boeckh M, Boivin G. Quantitation of cytomegalovirus: methodologic aspects and clinical applications. Clin Microbiol Rev. (1998) 11:533–54. doi: 10.1128/CMR.11.3.533
142. Hinckley AF, O'Leary DR, Hayes EB. Transmission of West Nile virus through human breast milk seems to be rare. Pediatrics. (2007) 119:e666–71. doi: 10.1542/peds.2006-2107
143. Sampieri CL, Montero H. Breastfeeding in the time of Zika: a systematic literature review. PeerJ. (2019) 7:e6452-e. doi: 10.7717/peerj.6452
144. Linnemann CC, Goldberg S. Letter: HBAg in breast milk. Lancet. (1974) 2:155. doi: 10.1016/S0140-6736(74)91574-8
145. Lin H-H, Hsu H-Y, Chang M-H, Chen P-J, Chen D-S. Hepatitis B virus in the colostra of HBeAg-positive carrier mothers. J Pediatr Gastroenterol Nutr. (1993) 17:207–10. doi: 10.1097/00005176-199308000-00014
146. Huseyin CE, O'Toole PW, Cotter PD, Scanlan PD. Forgotten fungi—the gut mycobiome in human health and disease. FEMS Microbiol Rev. (2017) 41:479–511. doi: 10.1093/femsre/fuw047
147. Ward TL, Dominguez-Bello MG, Heisel T, Al-Ghalith G, Knights D, Gale CA. Development of the human mycobiome over the first month of life and across body sites. MSystems. (2018) 3:e00140–17. doi: 10.1128/mSystems.00140-17
148. Boix-Amorós A, Martinez-Costa C, Querol A, Collado MC, Mira A. Multiple approaches detect the presence of fungi in human breastmilk samples from healthy mothers. Sci Rep. (2017) 7:1–13. doi: 10.1038/s41598-017-13270-x
149. Boix-Amorós A, Puente-Sánchez F, Du Toit E, Linderborg KM, Zhang Y, Yang B, et al. Mycobiome profiles in breast milk from healthy women depend on mode of delivery, geographic location, and interaction with bacteria. Appl Environ Microbiol. (2019) 85:e02994–18. doi: 10.1128/AEM.02994-18
150. Amir LH, Donath SM, Garland SM, Tabrizi SN, Bennett CM, Cullinane M, et al. Does Candida and/or staphylococcus play a role in nipple and breast pain in lactation? A cohort study in melbourne, Australia. BMJ Open. (2013) 3:e002351. doi: 10.1136/bmjopen-2012-002351
151. Derakhshani H, Plaizier JC, De Buck J, Barkema HW, Khafipour E. Association of bovine major histocompatibility complex (BoLA) gene polymorphism with colostrum and milk microbiota of dairy cows during the first week of lactation. Microbiome. (2018) 6:1–18. doi: 10.1186/s40168-018-0586-1
152. Bliss JM, Basavegowda KP, Watson WJ, Sheikh AU, Ryan RM. Vertical and horizontal transmission of Candida albicans in very low birth weight infants using DNA fingerprinting techniques. Pediatr Infect Dis J. (2008) 27:231–5. doi: 10.1097/INF.0b013e31815bb69d
153. Pausan MR, Csorba C, Singer G, Till H, Schöpf V, Santigli E, et al. Exploring the archaeome: detection of archaeal signatures in the human body. Front Microbiol. (2019) 10:2796. doi: 10.3389/fmicb.2019.02796
154. Samuel BS, Hansen EE, Manchester JK, Coutinho PM, Henrissat B, Fulton R, et al. Genomic and metabolic adaptations of Methanobrevibacter smithii to the human gut. Proc Natl Acad Sci U S A. (2007) 104:10643–8. doi: 10.1073/pnas.0704189104
155. Samuel BS, Gordon JI, A. humanized gnotobiotic mouse model of host-archaeal-bacterial mutualism. Proc Natl Acad Sci U S A. (2006) 103:10011–6. doi: 10.1073/pnas.0602187103
156. Togo AH, Grine G, Khelaifia S, des Robert C, Brevaut V, Caputo A, et al. Culture of methanogenic archaea from human colostrum and milk. Scientific Reports. (2019) 9:18653. doi: 10.1038/s41598-019-54759-x
157. Williams JE, Carrothers JM, Lackey KA, Beatty NF, York MA, Brooker SL, et al. Human milk microbial community structure is relatively stable and related to variations in macronutrient and micronutrient intakes in healthy lactating women. J Nutr. (2017) 147:1739–48. doi: 10.3945/jn.117.248864
158. Cortes-Macias E, Selma-Royo M, Martinez-Costa C, Collado MC. Breastfeeding practices influence the breast milk microbiota depending on pre-gestational maternal bmi and weight gain over pregnancy. Nutrients. (2021) 13:1518. doi: 10.3390/nu13051518
159. Urbaniak C, McMillan A, Angelini M, Gloor GB, Sumarah M, Burton JP, et al. Effect of chemotherapy on the microbiota and metabolome of human milk, a case report. Microbiome. (2014) 2:24. doi: 10.1186/2049-2618-2-24
160. Kumar H, du Toit E, Kulkarni A, Aakko J, Linderborg KM, Zhang Y, et al. Distinct patterns in human milk microbiota and fatty acid profiles across specific geographic locations. Front Microbiol. (2016) 7:1619. doi: 10.3389/fmicb.2016.01619
161. Padilha M, Danneskiold-Samsoe NB, Brejnrod A, Hoffmann C, Cabral VP, Iaucci JM, et al. The human milk microbiota is modulated by maternal diet. Microorganisms. (2019) 7:502. doi: 10.3390/microorganisms7110502
162. Zaidi AZ, Moore SE, Okala SG. Impact of maternal nutritional supplementation during pregnancy and lactation on the infant gut or breastmilk microbiota: a systematic review. Nutrients. (2021) 13:1137. doi: 10.3390/nu13041137
163. Padilha M, Brejnrod A, Danneskiold-Samsoe NB, Hoffmann C, de Melo Iaucci J, Cabral VP, et al. Response of the human milk microbiota to a maternal prebiotic intervention is individual and influenced by maternal age. Nutrients. (2020) 12:1081. doi: 10.3390/nu12041081
164. Jeppesen DL, Hasselbalch H, Lisse IM, Ersbøll AK, Engelmann MDM. T-lymphocyte subsets, thymic size and breastfeeding in infancy. Pediat Allergy Immunol. (2004) 15:127–32. doi: 10.1111/j.1399-3038.2004.00032.x
165. Kloc M, Ghobrial RM, Kuchar E, Lewicki S, Kubiak JZ. Development of child immunity in the context of COVID-19 pandemic. Clin Immunol. (2020) 217:108510. doi: 10.1016/j.clim.2020.108510
166. Martinez DR, Fong Y, Li SH, Yang F, Jennewein MF, Weiner JA, et al. Fc Characteristics mediate selective placental transfer of IgG in HIV-infected women. Cell. (2019) 178:190–201.e11. doi: 10.1016/j.cell.2019.05.046
167. Henrick BM, Yao X-D, Nasser L, Roozrogousheh A, Rosenthal KL. Breastfeeding behaviors and the innate immune system of human milk: working together to protect infants against inflammation, HIV-1, and other infections. Front Immunol. (2017) 8:1631. doi: 10.3389/fimmu.2017.01631
168. Iwasaki A, Medzhitov R. Control of adaptive immunity by the innate immune system. Nat Immunol. (2015) 16:343–53. doi: 10.1038/ni.3123
169. McGuire MK, McGuire MA. Human milk: mother nature's prototypical probiotic food? Adv Nutr. (2015) 6:112–23. doi: 10.3945/an.114.007435
170. Hanson LA. Session 1: Feeding and infant development breast-feeding and immune function. Proc Nutr Soc. (2007) 66:384–96. doi: 10.1017/S0029665107005654
171. Pacheco AR, Barile D, Underwood MA, Mills DA. The impact of the milk glycobiome on the neonate gut microbiota. Annu Rev Anim Biosci. (2015) 3:419–45. doi: 10.1146/annurev-animal-022114-111112
172. Alexander KL, Targan SR, Elson CO. Microbiota activation and regulation of innate and adaptive immunity. Immunol Rev. (2014) 260:206–20. doi: 10.1111/imr.12180
173. Goldman AS, Chheda S, Keeney SE, Schmalstieg FC. Fetal and Neonatal Physiology. Richard AP, David HR, William EB, William WF, (eds.). (2017).
174. Welsh KJ, Hwang SA, Boyd S, Kruzel ML, Hunter RL, Actor JK. Influence of oral lactoferrin on Mycobacterium tuberculosis induced immunopathology. Tuberculosis. (2011) 91:S105–13. doi: 10.1016/j.tube.2011.10.019
175. Liu KY, Comstock SS, Shunk JM, Monaco MH, Donovan SM. Natural killer cell populations and cytotoxic activity in pigs fed mother's milk, formula, or formula supplemented with bovine lactoferrin. Pediatr Res. (2013) 74:402–7. doi: 10.1038/pr.2013.125
176. Pammi M, Suresh G. Enteral lactoferrin supplementation for prevention of sepsis and necrotizing enterocolitis in preterm infants. Cochrane Database Syst Rev. (2017) 6:CD007137. doi: 10.1002/14651858.CD007137.pub5
177. Riskin A, Almog M, Peri R, Halasz K, Srugo I, Kessel A. Changes in immunomodulatory constituents of human milk in response to active infection in the nursing infant. Pediatr Res. (2012) 71:220–5. doi: 10.1038/pr.2011.34
178. Hassiotou F, Geddes DT. Immune cell-mediated protection of the mammary gland and the infant during breastfeeding. Adv Nutr. (2015) 6:267–75. doi: 10.3945/an.114.007377
179. Tromp I., Jong JK-d, Raat H, Jaddoe V, Franco O, Hofman A, et al. Breastfeeding and the risk of respiratory tract infections after infancy: the generation R study. PLoS ONE. (2017) 12:e0172763. doi: 10.1371/journal.pone.0172763
180. Bowatte G, Tham R, Allen KJ, Tan DJ, Lau M, Dai X, et al. Breastfeeding and childhood acute otitis media: a systematic review and meta-analysis. Acta Paediatr. (2015) 104:85–95. doi: 10.1111/apa.13151
181. Chatterton DEW, Nguyen DN, Bering SB, Sangild PT. Anti-inflammatory mechanisms of bioactive milk proteins in the intestine of newborns. Int J Biochem Cell Biol. (2013) 45:1730–47. doi: 10.1016/j.biocel.2013.04.028
182. Tuaillon E, Valea D, Becquart P, Al Tabaa Y, Meda N, Bollore K, et al. Human milk-derived B cells: a highly activated switched memory cell population primed to secrete antibodies. J Immunol. (2009) 182:7155–62. doi: 10.4049/jimmunol.0803107
183. Eiwegger T, Stahl B, Schmitt J, Boehm G, Gerstmayr M, Pichler J, et al. Human milk–derived oligosaccharides and plant-derived oligosaccharides stimulate cytokine production of cord blood T-cells in vitro. Pediatr Res. (2004) 56:536–40. doi: 10.1203/01.PDR.0000139411.35619.B4
184. Ruhaak LR, Stroble C, Underwood MA, Lebrilla CB. Detection of milk oligosaccharides in plasma of infants. Anal Bioanal Chem. (2014) 406:5775–84. doi: 10.1007/s00216-014-8025-z
185. Lis-Kuberka J, Orczyk-Pawilowicz M. Sialylated oligosaccharides and glycoconjugates of human milk. the impact on infant and newborn protection, development and well-being. Nutrients. (2019) 11:306. doi: 10.3390/nu11020306
186. Xiao L, van De Worp WR, Stassen R, van Maastrigt C, Kettelarij N, Stahl B, et al. Human milk oligosaccharides promote immune tolerance via direct interactions with human dendritic cells. Eur J Immunol. (2019) 49:1001–14. doi: 10.1002/eji.201847971
187. Hasselbalch H, Jeppesen DL, Engelmann MD, Michaelsen KF, Nielsen MB. Decreased thymus size in formula-fed infants compared with breastfed infants. Acta Paediatr. (1996) 85:1029–32. doi: 10.1111/j.1651-2227.1996.tb14211.x
188. Hsu PS, Nanan R. Does breast milk nurture T lymphocytes in their cradle? Front Pediatr. (2018) 6:268. doi: 10.3389/fped.2018.00268
189. Melnik BC, John SM, Schmitz G. Milk: an exosomal microRNA transmitter promoting thymic regulatory T cell maturation preventing the development of atopy? J Transl Med. (2014) 12:43. doi: 10.1186/1479-5876-12-43
190. Admyre C, Johansson SM, Qazi KR, Filén J-J, Lahesmaa R, Norman M, et al. Exosomes with immune modulatory features are present in human breast milk. J Immunol. (2007) 179:1969–78. doi: 10.4049/jimmunol.179.3.1969
191. Verduci E, Banderali G, Barberi S, Radaelli G, Lops A, Betti F, et al. Epigenetic effects of human breast milk. Nutrients. (2014) 6:1711–24. doi: 10.3390/nu6041711
192. Lawlor DA, Najman JM, Sterne J, Williams GM, Ebrahim S, Davey Smith G. Associations of parental, birth, and early life characteristics with systolic blood pressure at 5 years of age: findings from the Mater-University study of pregnancy and its outcomes. Circulation. (2004) 110:2417–23. doi: 10.1161/01.CIR.0000145165.80130.B5
193. Cope MB, Allison DB. Critical review of the World Health Organization's (WHO) 2007 report on 'evidence of the long-term effects of breastfeeding: systematic reviews and meta-analysis' with respect to obesity. Obes Rev. (2008) 9:594–605. doi: 10.1111/j.1467-789X.2008.00504.x
194. Mazariegos M, Zea MR. Breastfeeding and non-communicable diseases later in life. Arch Latinoam Nutr. (2015) 65:143–51.
195. Goldman AS. Future research in the immune system of human milk. J Pediatr. (2019) 206:274–9. doi: 10.1016/j.jpeds.2018.11.024
Keywords: breastfeeding, microbiota, delivery, chronic diseases, immune system
Citation: Duale A, Singh P and Al Khodor S (2022) Breast Milk: A Meal Worth Having. Front. Nutr. 8:800927. doi: 10.3389/fnut.2021.800927
Received: 24 October 2021; Accepted: 20 December 2021;
Published: 26 January 2022.
Edited by:
Amber Farooqui, Hospital for Sick Children, CanadaReviewed by:
Veronique Demers-Mathieu, University of California, San Diego, United StatesJaime Salcedo Dominguez, Independent Researcher, Wexford, Ireland
Copyright © 2022 Duale, Singh and Al Khodor. This is an open-access article distributed under the terms of the Creative Commons Attribution License (CC BY). The use, distribution or reproduction in other forums is permitted, provided the original author(s) and the copyright owner(s) are credited and that the original publication in this journal is cited, in accordance with accepted academic practice. No use, distribution or reproduction is permitted which does not comply with these terms.
*Correspondence: Souhaila Al Khodor, salkhodor@sidra.org
†These authors have contributed equally to this work and share first authorship