- 1Foodomics Laboratory, Institute of Food Science Research (CIAL, CSIC), Madrid, Spain
- 2Departamento de Procesos Industriales, Facultad de Ingenieria Agroindustrial, Universidad de Nariño, Pasto, Colombia
- 3High Pressure Laboratory, Departamento de Química, Facultad de Ciencias, Food Chemistry Research Group, Universidad Nacional de Colombia, Bogotá, Colombia
- 4Departamento de Nutrición y Bioquímica, Facultad de Ciencias, Pontificia Universidad Javeriana, Bogotá, Colombia
Tamarillo (Cyphomandra betacea (Cav.) Sendt.), or tree tomato, is a tropical fruit from the Andean region of South America; it is highly rich in vitamins, minerals, and polyphenolic compounds. In this study, extracts from tamarillo epicarp (TE) were obtained by pressurized liquid extraction (PLE), and their in-vitro neuroprotective potential was assessed. A central composite design with response surface methodology was performed to optimize PLE as a function of solvent composition and temperature. Selected response variables were extraction yield, total phenolic content (TPC), total flavonoid content (TFC), total carotenoid content (TCC), antioxidant (ABTS), and anti-inflammatory (LOX) activities, and anti-acetylcholinesterase (AChE) inhibitory capacity. According to the desirability function, the optimal conditions were 100% ethanol and 180°C with a 0.87 desirability value. Next, the anti-butyrylcholinesterase enzyme (BChE), reactive oxygen species (ROS), and reactive nitrogen species (RNS) inhibition as well as cytotoxicity in HK-2, THP-1 monocytes, and SH-5YSY neuroblastoma cell lines were studied for the TE extract obtained under optimized conditions. The optimum TE extract provided the following results: extraction yield (36.25%), TPC (92.09 mg GAE/g extract), TFC (4.4 mg QE/g extract), TCC (107.15 mg CE/g extract), antioxidant capacity (ABTS, IC50 = 6.33 mg/ml extract), LOX (IC50 = 48.3 mg/ml extract), and AChE (IC50 = 97.46 mg/ml extract), and showed no toxicity at concentration up to 120 μg/ml extract for all the tested cell lines. Finally, chemical characterization by liquid chromatography-tandem mass spectrometry (UHPLC-q-TOF-MS/MS) of the optimum TE extract exhibited an important presence of hydroxycinnamic acid derivatives and other phenolic acids as well as quercetin hexoside and rutin, as main metabolites responsible for the observed biological properties. All these results suggested that TE, which represents between 8 and 15% of the total fruit, could become a promising natural by-product with a potential “multitarget” activity against Alzheimer's disease.
Introduction
Tamarillo (Cyphomandra betacea (Cav.) Sendt.), also known as the tree tomato, is a solanaceous fruit growing in subtropical climates of South America, especially in countries that are crossed by the Andes Mountain, such as Bolivia, Chile, Colombia, Ecuador, Perú, and Venezuela, and is being considered as an Andean crop whose commercial production occurs in Colombia, Perú, and Ecuador (1). Tamarillo fruit is a promising bioresource since it is the fourth tropical fruit produced in Colombia, after pineapple, mango, and avocado (2). Because it grows in a wide range of altitudes (from 1,500 to 3,000 m) and easily adapts in frost-free climatic areas, this emerging crop has been introduced in agricultural economies of many countries, such as Argentina, Brazil, New Zealand, Australia, South-east Asia, Italy, Jamaica, Haiti, Mexico, Puerto Rico, Costa Rica, East Africa, Spain, and Portugal (1, 3).
Tamarillo fruit is an elliptical berry with an ovoid shape and between 5–10 cm long and 5–8 cm in diameter. The fruit is covered by a thick and bitter epicarp with purple, red, or yellow tones according to ecotype (4) and represents around 8–15% of waste biomass within industrial processing (5, 6) (see Figures 1A,B). The fruit is rich in minerals such as potassium, calcium, magnesium, iron, zinc, among others; non-starch polysaccharides, dietary fiber, vitamins A, C, B6, E, and K, carotenoids, and polyphenols (3, 4, 7). Recently, Martin et al. conducted a full study by Raman spectroscopy and Fourier transforms infrared (FTIR) in attenuated total reflectance configuration (FTIR-ATR) in order to identify the type and relative amount of metabolites present in tamarillo pulp, epicarp, and seeds as a guide to elucidate its nutraceutical potential (8).
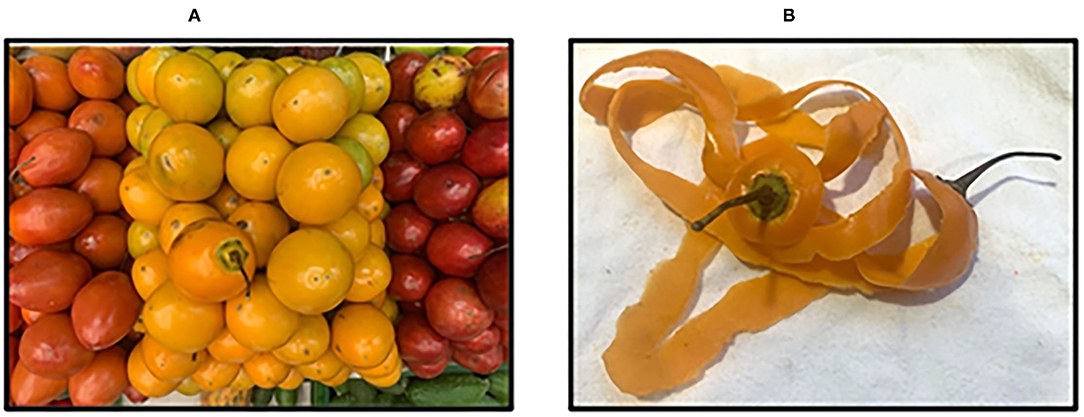
Figure 1. (A) Tamarillo fruit ecotypes (red, yellow, and purple) and (B) tamarillo fruit epicarp (yellow ecotype).
Several studies have reported the agronomical, physical, chemical, and nutritional characteristics of tamarillo (3, 7, 9, 10). Some biological activities have been described for the tamarillo pulp, mainly focused on treating obesity (11), or having antimicrobial (12), anti-inflammatory (11), antioxidant (10, 13–16), and antitumoral (15) properties. Moreover, only one study was focused on the nutritional properties of tamarillo seeds (17), while a few studies can be found related to the activity of tamarillo epicarp (TE) against lipid oxidation (18) and antibacterial potential (19).
At present, data of lifespan (especially in developed countries) have increased, which brings with it a great challenge: healthy aging. However, with elder age, the neurodegenerative process also advances as a natural consequence of brain aging. Manifestations, such as loss of memory, cognitive skills, and language, are exacerbated by the presence of hallmarks, such as oxidative damage, neuroinflammation, and accumulation of toxic amyloid peptides. Among the different neurodegenerative diseases (NDs), Alzheimer's disease (AD) is one of the most frequent; its development is directly related to the presence of extracellular plaques composed of amyloid-beta (Aβ) protein, intraneuronal tau (τ) in its hyperphosphorylated form, significant loss of synaptic connectivity and neuronal death, promoting brain atrophy. Chemical-based therapies have been used to alleviate some symptoms, although no treatment for AD is available yet; as a result, there is an increasing interest in developing new supplements based on natural extracts that can help in slowing down the neurodegeneration process. In this sense, it is worth mentioning the interest in nutraceutical compounds for a “multitarget” therapy of AD, which has been recently recognized as one important possibility for the future control of this disease (20). Some food-related sources and components have been already highlighted as potential anti-AD agents both in vitro and in vivo (21), namely, polyphenols and alkaloids (22), carbohydrates (23), and proteins (24) contained in plants and marine organisms (25). Other interesting sources of bioactive compounds with promising biological activities are food by-products that can be employed as a cheap source of high-added value compounds possessing pharmacological activity against neurodegenerative disorders.
To accomplish the recovery of bioactive compounds from food waste and its application in the food, pharmaceutical, or cosmetic fields, sustainable processes are preferred according to the United Nations Sustainable Development Goals (SDGs). Therefore, eco-friendly processes are intended to comply with the following characteristics: (1) preserving the biological activity of metabolites; (2) being technically efficient (high selectivity and low consumption of energy and solvents); (3) being innocuous (no hazard for the consumer). Among them, pressurized liquid extraction (PLE) is a useful technology to obtain bioactive metabolites from different solid matrices. This technique works at high pressure and temperature, and it can be employed with a wide range of green solvents promoting an important reduction in extraction time and energy consumption, as well as high selectivity, being a suitable green technique for natural sources and by-products.
In summary, the aim of this study was to evaluate the neuroprotective potential of different extracts from TE obtained by PLE and to optimize the operating conditions to attain an extract with potential “multitarget” activity against AD (antioxidant, anti-inflammatory, and anti-cholinergic). In addition, cytotoxicity in HK-2, THP-1 monocytes, and SH-5YSY neuroblastoma cell lines was assessed for the most promising TE extract.
Materials and Methods
Sample Preparation
Tamarillo (Cyphomandra betacea, yellow ecotype) fruit (3 kg) was acquired in a local market in Colombia. The TE was removed manually (600 g) and washed with distilled water. Then, it was dried at room temperature for 72 h, grounded, and sieved through 50 mesh (average particle size: 0.278 ± 0.008 mm); finally, 90 g of dry TE was obtained.
Reagents
Trizma hydrochloride (Tris-HCl), bovine serum albumin (BSA), potassium phosphate monobasic (KH2PO4) ≥ 99%, sodium phosphate dibasic (NaH2PO4) ≥ 99%, potassium persulfate (K2S2O8) ≥ 99%, sodium carbonate (Na2CO3) ≥ 99%, sodium nitroprusside dehydrate (SNP), fluorescein sodium salt, sulfanilamide, naphthylethylene diamine dihydrochloride, phosphoric acid, gallic acid, quercetin, linoleic acid (LA), and formic acid 99% were purchased from VWR Chemicals (EC); 7-fluorobenzofurazan-4-sulfonamide (ABD-F) 98% was acquired from Alfa Aesar (Kandel, Germany). Acetylcholinesterase (AChE) from Electrophorus electricus (electric eel) type VI-S, butyrylcholinesterase (BChE) from equine serum, 2,2-azino-bis (3-ethylbenzothiazoline-6-sulphonic acid) (ABTS·+), 3-(4,5-dimethylthiazol-2-yl)-2,5-diphenyltetrazolium bromide (MTT), phorbol 12-myristate 13-acetate (PMA), and β-mercaptoethanol were purchased from Sigma-Aldrich (Madrid, Spain). Acetylthiocholine iodide (ATCI) ≥ 99%, butyrylthiocoline iodide (BTCI) ≥ 99%, lipoxidase from glycine max (soybean), type 1-B and (±)-6-hydroxy-2,5,7,8-tetramethylchromane-2-carboxylic acid (Trolox) > 97%, were obtained from Sigma-Aldrich (Madrid, Spain). Folin-Ciocalteu's phenol reagent and aluminum chloride hexahydrate were purchased from Merck (Darmstadt, Germany). Galantamine hydrobromide, purity > 98%, was purchased from TCI Chemicals (Tokyo, Japan). HPLC-grade such as methanol, ethanol, and acetonitrile were purchased from VWR Chemicals (Barcelona, Spain), and ethyl acetate was purchased from Scharlau (Barcelona, Spain). Dichloromethane was purchased from Fluka AG (Buchs, Switzerland). MS grade acetonitrile and water from LabScan (Dublin, Ireland) were employed for UHPLC-q-TOF-MS. Ultrapure water was obtained from a Millipore system (Billerica, MA, United States). All the 96-well microplate assays were performed in a spectrophotometer and fluorescent reader (Synergy HT, BioTek Instruments, Winooski, VT, United States).
Pressurized Liquid Extraction (PLE)
Tamarillo epicarp extracts were obtained by a PLE process using a Dionex accelerated solvent extractor (ASE 200; Sunnyvale, CA, United States) at 1,500 ± 200 psi and 20 min as extraction time, as described in our previous study (26). Extraction conditions (temperature and solvent composition) were optimized according to a central composite design (CCD) and as described in the next section. Mixtures of water:ethanol, including a fixed percentage of formic acid (2%), were selected as previously suggested (27).
Near 200 mg d.w. of TE (for experiments T11-T13, when no EtOH was employed) and near 1,000 mg d.w. of TE (for the rest of experiments, including EtOH in the solvent composition) were subjected to PLE under different conditions obtaining nine extracts (T11-T13, T21-T23, T31-T33); five replicates of extract T22 were made to evaluate yield reproducibility. Under selected extraction conditions, two phases were obtained, a soluble phase and an insoluble phase (pectin), except when EtOH-formic acid 98:2 was used at 60°C (extract T31); under these conditions, only one phase was found (pectin was not extracted). Precipitates were separated by centrifugation (Eppendorf centrifuge 5804R; Eppendorf, Hamburg, Germany) at 10,000 rpm, 10°C, 30 min, and the supernatant was saved (S1); the remaining solid was washed with EtOH (5 ml) and centrifugated, obtaining a second supernatant (S2) that was mixed with S1, constituting each of the obtained extracts (T11 to T33). Samples extracted were first dried under N2 and then freeze-dried in a freeze-dryer (Lyobeta; Telstar, Terrassa, Spain).
Total yield was calculated as the ratio between the mass of extract at dry basis (x) and the mass of the dry sample was fed into the extraction cell (y), according to Equation 1:
Response Surface Methodology
The optimization of the extraction of bioactive compounds from TE by PLE was carried out using a CCD with response surface methodology (RSM). Two factors were considered at three levels: solvent composition (percentage of water in the mixture H2O/EtOH: 0, 50, and 100% v/v; considering a fixed 2% of formic acid) and temperature (60, 120, and 180°C). Selected response variables were extraction yield, total phenolic content (TPC), total flavonoid content (TFC), total carotenoid content (TCC), antioxidant (ABTS) and anti-inflammatory (LOX) activities, and anti-cholinergic (AChE) inhibitory capacity. The experimental data were fitted to a quadratic model for each response variable, Yi:
where SC is the solvent composition, T is the temperature, β0 is the intercept, β1, and β2 are the linear coefficients, β1,2 is the linear-by-linear interaction coefficient, β1,1 and β2,2 are the quadratic coefficients, and error is the error variable. STATISTICA 12 (Stat Soft, Inc., Tulsa, OK 74104, United States) was used for experimental design, data analysis, and multiple response optimization. The adequacy of the models was determined by the coefficient of regression (R2) and the F-test value (F-value) obtained from the analysis of variance (ANOVA). In addition, multiple response optimization was performed by the combination of experimental factors, looking to maximize the desirability function.
In vitro Assays
Total Phenolic Content (TPC)
Total phenolic content determination was performed by Folin–Ciocalteu method according to García Martinez et al. (28), with some modifications. A calibration curve was constructed using gallic acid (0–1,000 μg gallic acid per ml EtOH) as a chemical standard. An aliquot of 10 μl of TE extracts solution at a concentration range of 5–12 mg/ml in EtOH was mixed with 600 μl of H2O and 50 μl of Folin–Ciocalteu. After 1 min of agitation, 150 μl of Na2CO3 (20% w/v) was added, and the volume was completed to 1 ml with 190 μl of H2O. After 120 min of incubation at room temperature, 300 μl of each mixture was placed in a 96-well microplate spectrophotometer reader and the absorbance at 760 nm was measured. TPCs were expressed as gallic acid equivalents per gram extract (mg GAE/g of extract). All measurements were done in triplicate.
Total Flavonoid Content (TFC)
Total flavonoid determination was performed according to Kaushal et al. (29). A calibration curve from 0 to 14 μg/ml of a standard solution of quercetin (24 μg/ml) was constructed in order to measure the total flavonoids (or quercetin), expressed in milligram quercetin per gram extract (mg QE/g extract); 100 μl of TE extracts (with a concentration range of 5 to 12 mg extract/ml EtOH), 140 μl of MeOH, and 60 μl of anhydrous aluminum chloride (8 mM) were placed in each well and the absorbance was measured by spectrophotometry using a 96-well plate reader after 30 min at a wavelength 425 nm. All the measurements were done in triplicate.
Total Carotenoid Content (TCC)
Total carotenoid determination was performed according to Gilbert-López et al. (30). For this purpose, a standard solution of β-carotene as a reference carotenoid present in tamarillo was used as a chemical standard. A calibration curve at a concentration range of 0.172–22 μg/ml with R2 = 0.9935 was constructed in order to calculate the TTC expressed as milligram carotenoids per gram extract (mg CE/g extract). The TE extracts were dissolved in EtOH at a concentration range from 5 to 12 mg extract/ml EtOH, and then 300 μl was placed in each well and the absorbance was recorded by spectrophotometry using a 96-well plate reader at a wavelength of 470 nm. All the measurements were performed in triplicate.
In vitro Biological Activities Associated With AD
A total of nine TE extracts were tested using a battery of in vitro assays associated with neurodegenerative processes, such as antioxidant (ABTS·+) scavenging capacity, anti-inflammatory activity (LOX), and inhibition of acetylcholinesterase enzyme (AChE). For the most promising extract (T33), scavenging capacity against ROS and RNS species was measured by oxygen radical absorbance capacity (ORAC) and nitric oxide (NO·) radical, respectively. Moreover, butyrylcholinesterase enzyme (BChE) inhibition capacity and cell toxicity using the MTT assay were also performed for the T33 extract. All the assays were developed with a methodology previously described by Suárez Montenegro et al. (31).
Antioxidant Activity
• ABTS Scavenging Activity
ABTS·+ radical scavenging assay was performed according to Re et al. (32), with modifications. TE at six different concentrations of each sample [from 2.9 to 29 μg/ml EtOH/H2O (1:1, v/v)] was used, giving a linear response between 20 and 80% of the control absorbance. All the measurements were performed in triplicate. Equation (3) represents the percentage of ABTS·+ inhibition due to TE extracts and Trolox compared with the ABTS control.
where A ABTScontrol is absorbance of radical at t = 0 min; Asample, represents the mean of absorbance readings of TE samples, and ASampleblank is the absorbance of the sample without ABTS. The capacity of free radical scavenging was expressed by IC50 value, and the results represent the mean inhibitory concentration (in μg/ml) of TE against ABTS·+ radical.
• ROS Scavenging Capacity
Reactive oxygen species scavenging capacity was carried out by means of the ORAC method, according to Ou et al. (33) for the T33 sample. In this case, a concentration range of extract from 0.62 to 6.2 μg/ml in EtOH/H2O (1:9, v/v) was measured. The capacity of the T33 extract to scavenge peroxyl radicals generated by spontaneous decomposition of 2,20-azobis (2-amidinopropane) dihydrochloride (AAPH) was calculated through the inhibition percentage of the difference between the area under the curve (AUC) of fluorescence decay in the presence (AUCsample) or absence (AUCcontrol) of the sample by Equation (4). The measurements were carried out in triplicate. Trolox and rosemary extracts were used as chemical and natural positive controls, respectively.
AUC was calculated by means of Equation (5).
where f0 and fi are fluorescence at t = 0 and every 5 min, respectively.
• RNS Scavenging Capacity
Reactive nitrogen species scavenging capacity was measured following the NO· radical scavenging assay according to Ho et al. (34) and based on the Griess reaction. A concentration range from 70 to 700 μg/ml of the T33 extract in EtOH/H2O (1:3, v/v) was tested. Each measurement was carried out in triplicate, and ascorbic acid and rosemary extract were used as chemical and natural positive controls, respectively. The NO scavenging capacity of the extract was expressed through inhibition % calculated as described in Equation (3).
Anti-inflammatory Activity
Anti-inflammatory activity was measured by lipoxidase (LOX) inhibitory capacity enzyme by means of the fluorescence-based enzyme kinetic method, using fluorescein as a probe according to Whent et al. (35). In this case, TE at seven different concentrations of each sample (from 21.4 to 214 μg/ml) in EtOH/H2O (0.25:1) was measured. Equation (6) represents the percentage of inhibition of the sample compared with the negative control, where V1 and V0 are mean velocities obtained for LOX in the presence and absence of inhibitors. Quercetin and rosemary extract were used as chemical and natural positive controls, respectively. Each measurement was carried out in triplicate, and the results are expressed as mean ± standard deviation. IC50 value represents the concentration of quercetin or TE extract that produced 50% of enzyme inhibition capacity as compared with control (without inhibitors).
Anti-cholinergic Activity: AChE and BChE
The anti-cholinergic activity was evaluated by means of AChE and BChE inhibitory capacity enzymes of TE extracts, based on Ellman's method (1961) (36), modified by fluorescent enzyme kinetics study using ABD-F as a fluorescent probe (37). Seven different concentrations (17–167 μg/ml for AChE, and 37 to 370 for BChE) in EtOH/H2O (1:1, v/v) were measured. Equation (6) represents the percentage of inhibition of sample compared with negative control, where V1 and V0 are mean velocities obtained for AChE (or BChE) in the presence and absence of inhibitors. Each measurement was carried out in triplicate, and the results are expressed as mean ± standard deviation. IC50 value represents the concentration (in μg/ml) of galantamine or TE extract that produced 50% of cholinergic enzyme inhibition capacity as compared with control (without inhibitors), so it means lower IC50 concentrations exhibit a major inhibitory potency compared with higher IC50 values.
Cell Culture Conditions and Toxicity Assays
The in vitro toxicity evaluation of the selected TE extract (T33) was tested on three different cell lines: human proximal tubular epithelial cells (HK-2), human THP-1 monocytes, and human SH-5YSY neuroblastoma cells (all purchased from ATCC®, Rockville, MD, USA). Cell culture medium Dulbecco's Modified Eagle Medium Nutrient Mixture (DMEM/Ham's F12) and Roswell Park Memorial Institute (RPMI 1640) were acquired from Thermo Fisher Scientific (Grand Island, NY, United States). Also, fetal bovine serum (FBS), L-glutamine, penicillin, streptomycin, antibiotic-antimycotic solution, insulin-transferrine-selenium (ITS), and β-mercaptoethanol were purchased from Thermo Fisher Scientific (Grand Island, NY, United States). Phorbol 12-myristate 13-acetate (PMA) was acquired from Sigma-Aldrich (Madrid, Spain). Retinoic acid (RA) was purchased from Glentham Life Science (Corsham, United Kindgdom), whereas brain-derived neurotrophic factor (BDNF) was acquired from Bachem AG (Bubendorf, Switzerland).
HK-2 cells were grown according to the methodology previously reported in Suárez Montenegro et al. (31). For this purpose, cells were plated in 96-well plates at a density of 5 × 103 cells/well and, after 24 h, for proper attachment, different concentrations of the tamarillo extract (7.5 to 120 μg/ml) were added to the cells, which were then incubated for 24 h. The viability of the cells was then determined by MTT assay (38). Briefly, after 24 h of exposition to the T33 extract, the culture medium was removed, and the cells were incubated with 0.5 mg/ml MTT for 3 h at 37°C. Dimethyl sulfoxide (DMSO) was added to solubilize formazan crystals, and absorbance was measured at 570 nm in a plate reader.
Human THP-1 monocytes were cultured and maintained as described by Villalva et al. (39), with modifications reported by our group (31). For toxicity assay, cells were seeded in 24-well plates at a density of 5 × 105 cells/ml, and monocytes were differentiated to macrophages by maintaining the cells with 100 ng/ml of PMA for 48 h. Then, theT33 extract was added to the wells at different concentrations (60 and 120 μg/ml), and the viability of the cells was evaluated by using the MTT assay as described above.
Furthermore, human SH-SY5Y cells were grown, maintained, and differentiated following the protocol proposed by de Medeiros et al. (40), with some modifications. Briefly, cells were maintained in DMEM/Ham's F12 1:1 supplemented with 100 U/ml penicillin, 100 μg/ml streptomycin, and 250 ng/ml antimycotic at 37°C in a humidified atmosphere of 5% of CO2. Only attached cells were maintained, and floating cells were discarded.
For neuronal differentiation, cells were treated with retinoic acid (RA) and brain-derived neurotrophic factor (human) (BDNF) for 7 days, as follows: first, the cells were plated and after 4 days, the cell culture medium was changed with a fresh medium with 10% FBS (day 0 of differentiation). Then, after 24 h (day 1), the cell culture medium was replaced with a fresh medium supplemented with 1% FBS and 10 μM of RA. After 3 days (day 4), the cell culture medium was replaced with a fresh medium with 1% FBS, 10 μM of RA, and 50 ng/ml BDNF. Finally, 3 days after (day 7), differentiated cells were seeded in 24-well plates at a density of 8 × 105 cells/ml for 24 h. To evaluate the neurotoxic effect, the cells were incubated with different concentrations (60 and 120 μg/ml) of the T33 extract for 24 h, and the viability of the cells was tested by using the MTT assay as described above.
In all cases, the toxicity of the extracts is shown as relative cell viability, which is expressed as the percentage of living cells compared with controls (DMSO-treated). DMSO, used for diluting the extract, did not exceed a concentration of 0.4% (v/v). All the experiments were performed in triplicate.
Liquid Chromatography-Tandem Mass Spectrometry (UHPLC-q-TOF-MS/MS) Analysis of Tamarillo Epicarp Extract
A liquid chromatography (Agilent 1290 UHPLC system; Agilent Technologies, Sta. Clara, CA, United States) coupled to a quadrupole-time-of-flight mass spectrometer (Agilent 6540 q-TOF MS; Agilent Technologies, Sta. Clara, CA, United States) by an orthogonal ESI source was used for the tentative identification of compounds present in the most active extract (T33). A 5-μl aliquot of the sample was injected at a flow rate of 0.5 ml/min in a Zorbax Eclipse Plus C18 column (2.1 × 100 mm, 1.8 μm particle diameter; Agilent Technologies, Santa Clara, CA, United States) at 30 °C, and the compounds were separated using a mobile phase consisting of A (water 0.01% formic acid) and B (acetonitrile 0.01% formic acid) in a gradient elution: 0 min, 0% B; 7 min, 30% B; 9 min, 80% B; 11 min, 100% B; 13 min, 100% B; 14 min, 0% B. The mass spectrometer was operated in negative mode, and MS and MS/MS analyses were performed for the structural analysis of all the compounds. MS parameters were the following: capillary voltage, 4000 V; nebulizer pressure, 40 psi; drying gas flow rate, 10 L/min; gas temperature, 350°C; skimmer voltage, 45 V; fragmentor voltage, 110 V. The MS and auto MS/MS modes were set to acquire m/z values ranging between 50 and 1,100 and 50 and 800, respectively, at a scan rate of 5 spectra per second. The Agilent Mass Hunter Qualitative Analysis software (B.08.00) was used for post-acquisition data processing, making use of filtering tools by fragments features based on diagnostic product ions (DPIs) and/or neutral loss of interest, or compound search by MS or MS/MS features that greatly enhance data interpretation. Peak identification in the TE extracts was performed by generation of the molecular formula with a mass accuracy of 5 ppm, and the characterization strategy was based on ESI-MS (accurate mass and isotopic distribution) and ESI-MS/MS (fragmentation pattern) data, together with information previously reported in the literature and databases, such as METLIN Metabolite Database (http://metlin.scripps.edu), Reaxys (https://www.reaxys.com), and PubChem (https://pubchem.ncbi.nlm.nih.gov/).
Statistical Analysis
Experimental data results are given as mean ± SD. They were analyzed by ANOVA, and means were compared by Tukey's honestly significant difference (HSD) test (SPSS statics V15 IBM, New York, United States) at a 95% confidence level. Means labeled with different alphabetical letters in the same column of the table are considered statistically different at p < 0.05. Besides, a statistical test of correlation was performed in order to establish the degree of influence of the independent factors on the response variables and the interaction between them.
Results and Discussion
Pressurized Liquid Extraction Optimization
Considering the scarce information found in the literature about TE bioactivities and composition, the first goal of this study was to optimize PLE conditions using an RSM to test its efficiency toward the extraction of bioactive compounds. Based on results from Martin et al. (8), TE comprised high amounts of phenolic constituents and carotenoids. Wang and Zhu's review referred to the most relevant biological activities of tamarillo fruits and their by-products (7), and a mild AChE activity was reported by Hassan and Bakar for a methanolic extract from TE obtained by solid-liquid extraction (41).
Bearing this composition in mind, an experimental CCD was proposed in order to study the effect of the two main parameters involved in the extraction by PLE, i.e., extraction temperature (60–180°C) and green solvent composition (water/ethanol mixtures containing 2% formic acid), on the ability to obtain extracts with multitarget bioactivities. Selection of the range of the factors to be optimized was done according to previous results obtained by our research group targeting phenolic compounds (26). Moreover, 2% formic acid was employed in all solvent compositions as suggested by Brazdauskas et al. (27). A CCD at three levels was set up, as shown in Table 1, which also includes the different response variables studied. In this sense, as previously mentioned, AD is related to the accumulation of toxic amyloid peptides, oxidative stress, and neuroinflammation; therefore, those biological activities were selected as response variables and tested by using in vitro assays such as ABTS, LOX, and AChE. Moreover, other responses were also chosen to measure TPC, TFC, and TCC. All the responses were statistically analyzed, and a regression analysis was performed on the experimental data. The coefficients of the model(s) were evaluated for significance by using ANOVA for the different response variables (Supplementary Table 1).
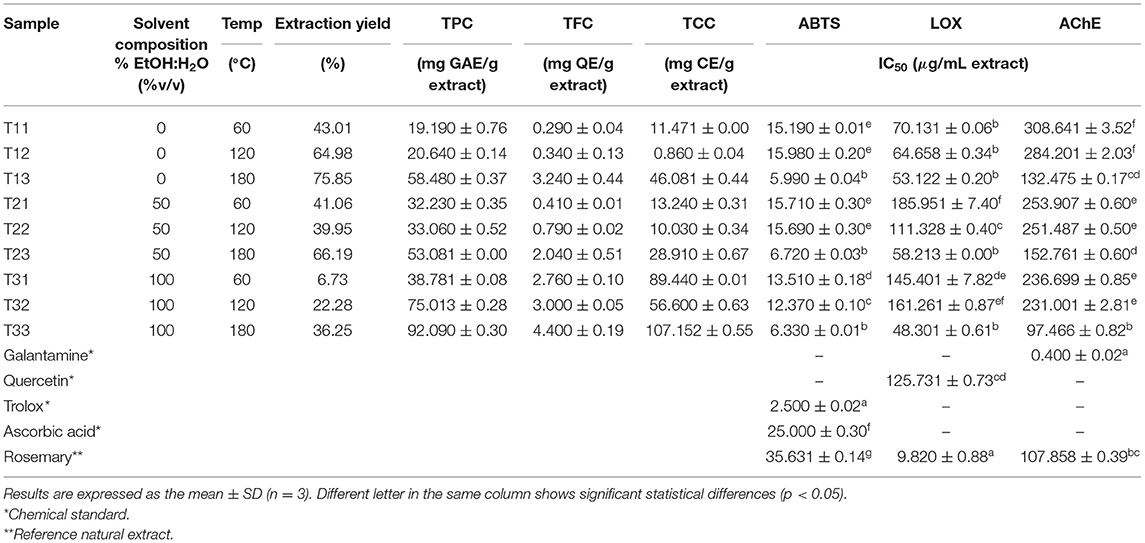
Table 1. Experimental design and results of response variables [yield, total phenolic content (TPC), total flavonoid content (TFC), total carotenoid content (TCC), antioxidant (ABTS), anti-inflammatory (LOX), and anti-acetylcholinesterase (AChE) activities] for TE extracts obtained by pressurized liquid extraction (PLE).
As can be seen in Table 1, the response variables show different behavior depending on the extraction conditions. Considering their interest not only as a global composition but also in terms of “multitarget” bioactivity against AD, the responses will be individually analyzed and discussed in terms of the correlation observed among them and with the total content of phenolics, flavonoids, and carotenoids.
Effect of Extraction Conditions on Yield and Total Bioactive Compound Content
Pearson's correlation model shows high (−0.78) and moderate (0.57) correlations between solvent composition, extraction temperature, and total yield, respectively, and as shown in Figure 2. Thus, the highest global yields of the PLE extracts were achieved with 100% water (98% water, 2% formic acid) and with water:ethanol (50:50) mixtures as extraction solvent. As a general trend, experimental data showed an increase in the global extraction yield by raising the extraction temperature from 60 to 180°C; this result was expected, considering that an increase in temperature improves mass transfer from the sample to the extraction solvent, increasing the solubility of the compounds and reducing solvent viscosity (thus favoring penetration of the solvent into the matrix).
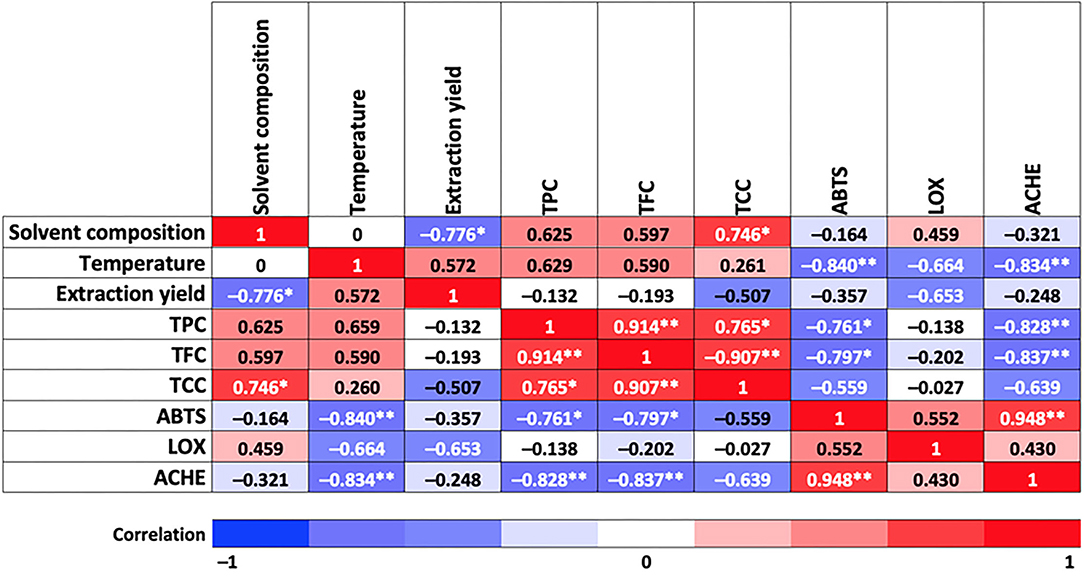
Figure 2. Correlation between independent factors and response variables involved in this study. Asterisks indicate a correlation at 0.05 significance level (*) and at 0.01 significance level (**).
As for TPC and TFC, responses follow the same trend as total yield, that is, increasing their value by increasing temperature within the same extraction solvent, although in this case, higher TPC and TFC were obtained using 100% EtOH (values equal to 92.09 mg GAE/g extract and 4.4 mg QE/g extract, respectively). As for TCC, the highest values were achieved at the highest and lowest temperatures for all the solvents tested, being also 100% ethanol the one providing the highest TCC (107.15 mg CE/g extract). A high (0.75) and moderate (0.63 and 0.60) correlations between TCC, TPC, and TFC, respectively, with solvent composition, were observed in Figure 2. Moreover, TPC and TFC showed a moderate correlation with extraction temperature (0.66 and 0.59, respectively). It is also interesting to observe correlations among the bioactive compounds measured; for instance, between TPC and TCC (high correlation, 0.77) and TPC and TCC with TFC (very high correlation, 0.91). A Pareto diagram for the effect of solvent composition and temperature on total bioactive compound content, and response surface plots are shown in Supplementary Figures 1, 2.
Effect of Extraction Conditions on ABTS, LOX, and AChE Activities. Correlation With TPC, TFC, and TCC
Antioxidant Activity
The analysis of variance test applied to determine the behavior of different extraction treatments in response to ABTS variable showed significant statistical differences compared with Trolox, ascorbic acid chemical standard, and rosemary natural standard, at p < 0.05 (see Table 1). However, it is remarkable that all the samples exhibited lower IC50 than ascorbic acid and rosemary, which means a high antioxidant activity of the TE extracts. Moreover, Figure 2 shows a very high correlation between ABTS and extraction temperature (−0.84) and almost no effect of the extraction solvent (−0.16). Therefore, higher antioxidant activity is expected at the highest extraction temperature (obtained extracts at 180°C exhibited near half IC50 values compared with those obtained at lower extraction temperatures), independently of the extraction solvent used. The behavior of the antioxidant activity as response variable as a function of temperature and solvent composition is presented in Supplementary Figures 3, 4.
In terms of chemical composition, a high correlation was observed between ABTS-TPC (−0.76) and ABTS-TFC (−0.8) (see Figure 2), which means that a lower IC50 value is achieved with a higher content of phenolic and flavonoid compounds. In fact, Table 1 shows that higher ABTS scavenging capacity was reached by the T13, T23, and T33 sample groups (IC50 = 5.99 ± 0.04, 6.72 ± 0.03, and 6.33 ± 0.01 μg/ml, respectively), and also reports the highest content of phenolic (58.48, 53.08, and 92.09 mg GAE/g extract) and flavonoid compounds (3.24, 2.04, and 4.4 mg QE/g extract). Skinner et al. (42) mentioned that tamarillo skin is the highest contributor to the phenolic content (by weight) compared with pulp and seeds, being hydroxycinnamic acid (HCA) derivatives the major compounds. The observed antioxidant activity could be attributed mainly to phenolic acids identified in this study, such as HCA group, quinic acid, caffeoyl glucoside, chlorogenic acids (CGAs), and rosmarinic acids, among others, which is in agreement with previously reported results (6, 7, 43).
In the case of flavonoids, a higher amount of these bioactive metabolites has been reported in tamarillo peel (3.36 mg rutin eq/g d.w.) compared with pulp (2.41 mg rutin eq/g d.w.) by Mutalib et al. (44). These authors also stated that flavonoid content is 3-fold larger in ethanolic extracts than in water extracts. This is in agreement with our results in which a higher flavonoid content was achieved in the T31, T32, and T33 samples (2.76, 3, and 4.4 mg QE/g extract, respectively), extracted with 100% EtOH. The antioxidant activity of flavonoids is attributed to the presence of several -OH groups in the molecule; in this sense, some studies reported the strong antioxidant and radical scavenging capacity of these phytochemical compounds (45–47). According to Nijveldt et al., flavonoids exhibit strong cell protection against ROS through different working mechanisms including an increase in the function of endogenous antioxidants (46). First, flavonoids can work as direct radical scavengers being oxidized by radicals because of the high reactivity of the OH group of the flavonoids; hence a radical molecule becomes more stable and inactive; an example of these are rutin (48), luteolin, and quercetin (49, 50); all of them are found in this study. Second, NO· plays a significant role in molecular events involved in neurodegenerative diseases, promoting neuronal death through oxidative damage on cellular lipids, proteins, and ADN (51); therefore, flavonoids are able to remove the overconcentration of NO· radicals (46, 52, 53). Third, other antioxidant pathways attributed to flavonoids are inhibition or xanthine-oxidase (source of highly toxic hydroxyl radicals) enzymes or iron-chelating capacity. The key role of quercetin (one of the most powerful bioflavonoids) as an inhibitor of NO synthase (53), xanthine oxidase (54), and iron-chelator (55) is noticeable.
Total carotenoid content exhibited a moderate correlation with ABTS (−0.56) (Figure 2). In this study, an important content of total carotenoids (89.44, 56.6, and 107.15 mg CE/g extract) was achieved in the ethanolic extracts (T31, T32, and T33) at 60, 120, and 180°C, respectively, being T33 the extract with the highest TCC (107.15 ± 2.55 mg CE/g extract) and the highest ABTS scavenging capacity (IC50 = 6.33 ± 0.01 μg/ml, see Table 1). In fact, tamarillo fruits are classified according to skin color, which is related to the presence of carotenoids. According to this study, a higher amount of carotenoids was found in TE than in the results reported by Ghosh et al. (56) for peels from orange, mango, and pomegranate grape. Likewise, the presence of vitamin C (ascorbic acid) in tamarillo is also important not only for its recognized contribution to antioxidant activity but also when synergic interaction within the metabolic pathways of other vitamins is considered, despite its smaller proportion in the peel than pulp (15–30% lower) according to results reported by Diep et al. (57).
Furthermore, vitamin E (α-tocopherol form) is another relevant antioxidant present in TE with scavenger capacity of lipid peroxyl radicals and potent agent against oxidative damage involving chronic diseases, such as cardiovascular diseases, cancer, and AD (31, 37, 58–60). According to Diep et al. (57), a higher content of α-tocopherol was found in the peel than in the pulp of all studied tamarillo varieties, which undoubtedly contributes to an increase in important results in terms of the antioxidant potential of tamarillo epicarp extracts.
Anti-inflammatory Activity
Neuroinflammation is the mechanism of the central nervous system that occurs in response to trauma, infections, or neurodegenerative diseases. Considering the importance of anti-inflammatory activity on the multitargeted approach of this study, this bioactivity was measured in all the extracts through the enzymatic activity of LOX (data shown in Table 1).
The ANOVA showed no significant differences (p- < 0.05) among the T11, T12, T13, T23, and T33 samples. Nevertheless, there is a statistically significant difference between the mentioned samples and quercetin (employed as chemical standard) (IC50 = 125.73 μg/ml) having, on average, an IC50 twice the one obtained for T11, T12, T13, T23, and T33 (70.13, 64.65, 53.12, 58.21, and 48.3 μg/ml, respectively), suggesting a high anti-inflammatory potential of the TE extracts. Regarding correlations between factors and responses involved in LOX, a medium-high correlation of LOX with extraction yield and extraction temperature (−0.65 in both cases) and with extraction solvent (0.46) was observed (Figure 2). Notwithstanding, the best LOX values (lowest IC50) were achieved at the highest temperature (180°C) for all the extracting solvents studied, with IC50 of 53.12 ± 2.2, 58.21 ± 3, and 48.3 ± 1.61 μg/ml the for T13, T23, and T33 extracts, respectively.
A moderate correlation (0.55) with ABTS is also observed (see Figure 2). It means that the TE extracts that showed a potential antioxidant activity could also have a potential anti-inflammatory response, as can be seen in T13, T23, and T33.
The release of arachidonic acid is a starting point for a general inflammatory response (46). Hence, certain flavonoids act by decreasing the level of the arachidonic acid pathway, prostaglandins, leukotrienes, and NO, recognized mediators in inflammation through the inhibition of enzymes involved in the process, such as phospholipase A2 (PLA2), cycloxygenase (COX), LOX, and NO synthase (45, 51). As a consequence, the better potential of LOX inhibitory capacity was achieved for the T13, T23, and T33 extracts characterized by the higher content of flavonoids with recognized anti-enzymatic effect (47, 61). In this sense, quercetin, a flavonol used in this study, is the standard generally employed in anti-inflammatory assays.
Our results are in agreement with those recently published by Li and Li (61) who evaluated the inhibitory activity of tamarillo peel extracts against inflammatory markers (PGE-2, IL-1β, and TNF-α) on LPS-induced macrophages (RAW 264.7 cell line). The authors concluded that low concentrations of tamarillo skin extracts (12.5 and 25 μg/ml) exerted anti-inflammatory activity by reducing these markers, attributing this activity to the presence of high content of flavones, isoflavones, and flavonols.
Anti-cholinergic Activity
As shown in Table 1, the IC50 values represent the concentration (μg/ml) of the TE extract capable of producing half inhibition of AChE enzyme activity; thus, lower IC50 means greater enzymatic inhibition capacity. Through the analysis of variance (Supplementary Table 1), the behavior of the different treatments applied to TE on the response variable AChE was studied. As can be seen, ANOVA and Tukey post hoc multiple comparisons determined that all the analyzed extracts showed significant differences compared with galantamine as chemical standard, and T13, T23, and T33 exert a significant influence on the AChE response variable, with IC50 values of 132.47 ± 5.17, 152.76 ± 10.6, and 97.46 ± 6.82 μg/ml extract, respectively. These values allow them to be classified as within moderate potency AChE inhibitors, with a range of 20 < IC50 <200 μg/ml, according to data reported by Dos Santos et al. (62) in the general classification of natural extracts efficacy.
As a summary, these results suggested that when the TE (yellow ecotype) is subjected to the highest tested extraction temperature (180 °C) by PLE, it is possible to obtain extracts rich in flavonoid, phenolic, and carotenoid-type compounds, showing promising anti-AChE, anti-inflammatory, and antioxidant activities.
Effect of Extraction Conditions on Total Bioactive Constituent Contents and Biological Activities. Multiple Response Optimization
Due to the promising results obtained for the TE extracts under PLE conditions, an optimization of the operating conditions considering all responses studied was performed to achieve an extract with potential for a “multitarget” therapy of AD (antioxidant, anti-inflammatory, and anti-cholinergic); to do this, a desirability function was calculated. Profiles for predicted values estimated by desirability function are shown in Figure 3.
The optimal conditions were 100% EtOH and 180°C with a 0.87 desirability value, corresponding to the extraction conditions of the T33 extract. The desirability value was close to 1, and the predicted values obtained by the global desirability function under optimum conditions were compared with experiment T33 (Table 2). The observed and predicted data were within the confidence intervals (Figure 3) and, as can be seen, the proximity between predicted and experimental data confirmed that the selected RSM model was successfully applied for obtaining a TE extract with anti-neurodegenerative potential.
As a summary, conditions selected for obtaining the T33 extract provided a medium yield (36.25%), and the highest total content of phenolics (92.09 mg GAE/g extract), flavonoids (4.4 mg QE/g extract), and carotenoids (107.15 mg CE/g extract), and the lowest IC50 values (ABTS: 6.33 μg/ml; LOX: 48.3 μg/ml; AChE: 97.46 μg/ml). In order to deepen the knowledge of the T33 extract and its ability as a multitarget extract against AD, complementary biological activities (ROS, RNS, BChE) and chemical characterization by UHPLC-q-TOF-MS/MS were performed.
ROS, RNS, and BChE Activities
Table 3 shows the results obtained on the complementary biological activities related to neuroprotective potential tested on the T33 extract. Considering that one of the main symptoms of neurodegenerative disorders is oxidative and nitrosative stress caused by an imbalance between the production of ROS/RNS and response capacity with antioxidant molecules (generating as a consequence neuronal damage and cell death), ROS and NRS activities were measured for the T33 extract. As can be seen in Table 3, the T33 extract exhibited an IC50 value lower than those given by rosemary extract (IC50 = 2.54 ± 0.14 μg/mL against IC50= 4.51 ± 0.23, respectively), requiring almost half concentration than rosemary extract to reach the same antioxidant capacity, demonstrating a higher ROS scavenging property but lower than Trolox standard (IC50= 1.29 ± 0.09 μg/ml). In terms of RNS, the IC50 value of the T33 extract improved almost twice compared with ascorbic acid standard (IC50 = 599 ± 5.92 μg/ml against IC50 = 1,100.9 ± 13.96 μg/ml, respectively), but it was less effective than the rosemary standard (IC50 = 95.35 ± 6.45 μg/ml). As mentioned previously, the presence of phenolic and flavonoid-type metabolites with free radical scavenging and inhibitory enzymatic properties, such as quercetin, luteolin, and rutin, exerts powerful protection against ROS and RNS. As for anti-BChE inhibitory activity, the T33 extract exhibited significant differences (p < 0.05) compared with both standards studied (galantamine and rosemary). However, better IC50 (85.462 ± 0.68 μg/ml) was achieved by the TE extract than those reached by the orange by-product ethanolic extract obtained by maceration (IC50 = 494 ± 68 μg/ml) and reported by Sánchez-Martínez et al. (36).
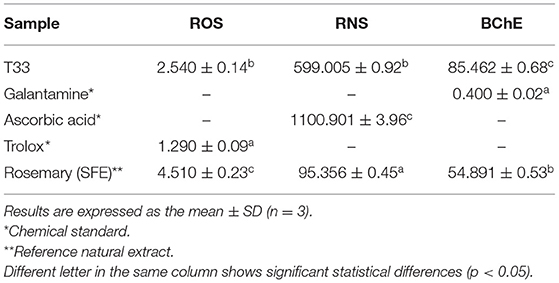
Table 3. IC50 values (μg/ml) of reactive oxygen species (ROS)-oxygen radical absorbance capacity (ORAC), reactive nitrogen species (RNS), and anti-butyrylcholinesterase enzyme (BChE) assays for T33 tamarillo epicarp extract.
Therefore, results on the complementary biological activities (ROS, RNS, and BChE) obtained for the T33 extract allowed to confirm its important neuroprotective potential.
UHPLC-q-TOF-MS/MS Analysis
The chromatographic profile of the T33 extract is shown in Figure 4 and the tentatively identified compounds are summarized in Table 4, namely, the retention time (min), molecular formula, experimental and theoretical m/z, error (ppm), and main MS/MS fragments. A total of 30 compounds were tentatively identified belonging to different chemical classes, namely, hydroxybenzoic acids and derivatives, HCAs, CGAs and derivatives, acetyl quinic acid derivatives, and flavonoids, among others. According to previous studies, neutral losses of H2O (18 Da) or CO2 (44 Da) molecules were frequently found for phenolic acids and derivatives, such as compounds 1 (quinic acid), 7 (hydroxybenzoic acid), 8 (gallic acid), 14 (caffeic acid), 26 (dihydrocaffeic acid), 23 (sinapic acid), and 13 (syringaldehyde) (63, 67, 69, 76). However, compound 9 showed a different fragmentation pattern including the main ion fragment at m/z 93 because of loss of C3H2O2 (70 Da); hence, the structure was tentatively assigned to p-coumaric acid. Phenolic glycosides were detected by the characteristic direct loss of the hexose moiety, such as syringic acid hexoside (compounds 2 and 3) and caffeoyl hexoside (compound 6), as well as by the fragmentation of these compounds yielding aglycone ions (64, 66). CGAs were distinguished on the basis of their typical fragmentation patterns, owing to the loss of phenolic acid residues; these compounds included caffeoylquinic acid (compounds 5, 10, and 12) and caffeoylshikimic acid (compounds 15 and 17). Caffeoylquinic acid isomers showed a fragment ion at m/z 191 because of loss of caffeoyl moiety, and 173 caused by consecutive loss of H2O; also, fragment ions at m/z 179 and 161 were observed because of loss of quinoyl moiety and subsequent H2O loss (63). Similarly, caffeoylshikimic acid isomers showed fragments at m/z 179, 161, and 135 corresponding to the losses of shykimoyl, shykimoyl, and H2O, and shykimoyl and CO2 moieties, respectively (64). Compound 25 was tentatively identified as rosmarinic acid since the MS/MS fragmentation of its pseudomolecular peak ([M – H]−ion at m/z 359) led to three peaks at m/z 197, 179, and 161 corresponding to the deprotonated form of 3-(3,4-dihydroxy-phenyl) lactic and caffeic acids and their dehydrated forms, respectively, which have been previously described (72, 73). Quinic acid derivatives were also observed in the T33 extract. Compounds 11, 16, and 19 were tentatively identified as acetyl quinic acid and acetyl quinic acid derivatives 1 and 2, respectively, because of the loss of acetyl moiety resulting in the quinic acid group observed at m/z 191 in MS/MS experiments (68). Compounds 16 and 19 were the most intense peaks in the chromatogram along with 4 and 30 (see Figure 4). Compound 4 was tentatively assigned to ethyl citrate, which gave a [M – H]−ion at m/z 219 with fragment ion at m/z 111 in the MS/MS experiment caused by the loss of ethyl acetyl and two water groups (65). Compound 30 was identified as phytuberin, a sesquiterpenoid found in other Solanaceae species (77), which showed a [M – H]−ion at m/z 293 in the ESI-MS mode and daughter MS/MS ions at m/z 236, 221, and 192, indicating the losses of acetate, acetate, and CH3, and isopropyl acetate groups, respectively, as described by Serralheiro et al. (75). Methyl esters of CGAs and methyl quinates were also found in the T33 extract. These compounds are widely distributed in plant materials and frequently appear as extraction artifacts in these samples (70). Compounds 18, 22, 24, and 27 were tentatively assigned to methyl caffeoyl quinate, methyl feruloyl quinate isomer 1, methyl feruloyl quinate isomer 2, and methyl feruloyl hexoside, respectively. The fragmentation behavior of methyl esters of CGAs and methyl quinates showed the loss of methyl quinate moiety and subsequent losses of CH3, H2O, or CO2, as can be seen for the explained MS/MS product ions in Table 4. In the case of methyl feruloyl hexoside, direct loss of the hexose (fragment ion at m/z 207) allowed identification. Compound 29 was identified as ethyl caffeate, which gave a characteristic fragment ion at m/z 161 because of the loss of ethanol. Ethyl caffeate is presumably a product of trans-esterification of caffeic acid esters and ethanol, formed during the PLE extraction process. Barth et al. (74) have explained this phenomenon considering that esterification is a reaction that occurs between a carboxylic acid and an alcohol, being favored by the increase in temperature, through nucleophilic substitution on acyl carbon in which the nucleophile (ethanol) attacks the carbon of carbonyl group (caffeic acid), leading to the formation of the ester. Regarding flavonoids, compounds 20 and 21 were tentatively assigned to rutin and quercetin hexoside, respectively, which were distinguished on the basis of their typical loss of sugar moiety (rutinose or hexose) resulting in the fragment ions observed at m/z 301 (71). HCA and CGA derivatives, as well as flavonoids, have been described as the major types of phenolic compounds in the golden-yellow tamarillo fruit (7). Orqueda et al. (16) identified several caffeic acid derivatives (i.e., caffeoyl hexoside, caffeoylquinic acid isomers, among others) and related phenolics, rosmarinic acid derivatives, and flavonoids (i.e., quercetin hexoside, runtin, among others) by HPLC–ESI-MS/MS analysis on different segments of tamarillo fruit (Argentinian cultivar), such as the peel. Espin et al. (78) also identified and quantified some phenolic compounds from tamarillo fruit (Ecuatorian cultivar) finding a concentration that ranged between 1.27 and 42.73 mg caffeoylquinic acid/100 g dw and 12.22 and 32.85 mg rosmarinic acid/100 g dw. Loizzo et al. (79) reported the phenolic content in an ethanolic extract of tamarillo peel fruit (Colombian cultivar): chlorogenic acid (253.8 mg/kg extract), p-coumaric acid (0.2 mg/kg extract), sinapic acid (10.3 mg/kg extract), and syringaldehyde (0.7 mg/kg extract).
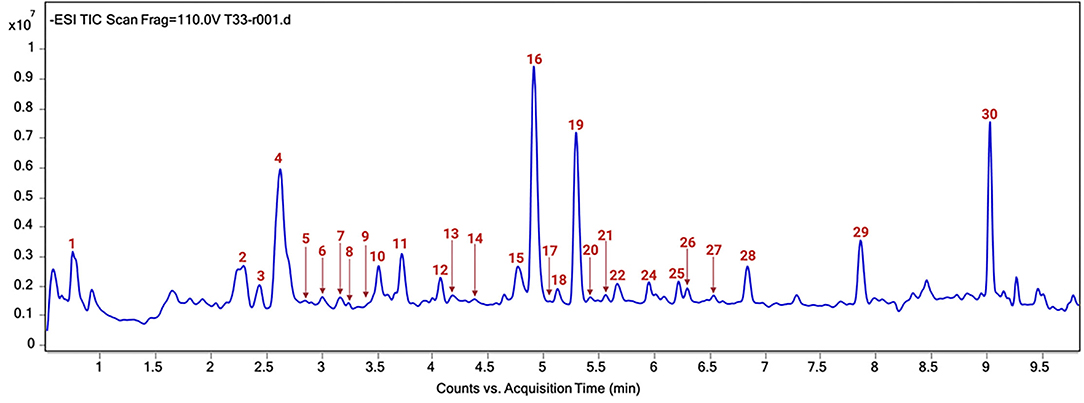
Figure 4. Total ion current (TIC) chromatogram of T33 extract. Peak identification can be found in Table 4.
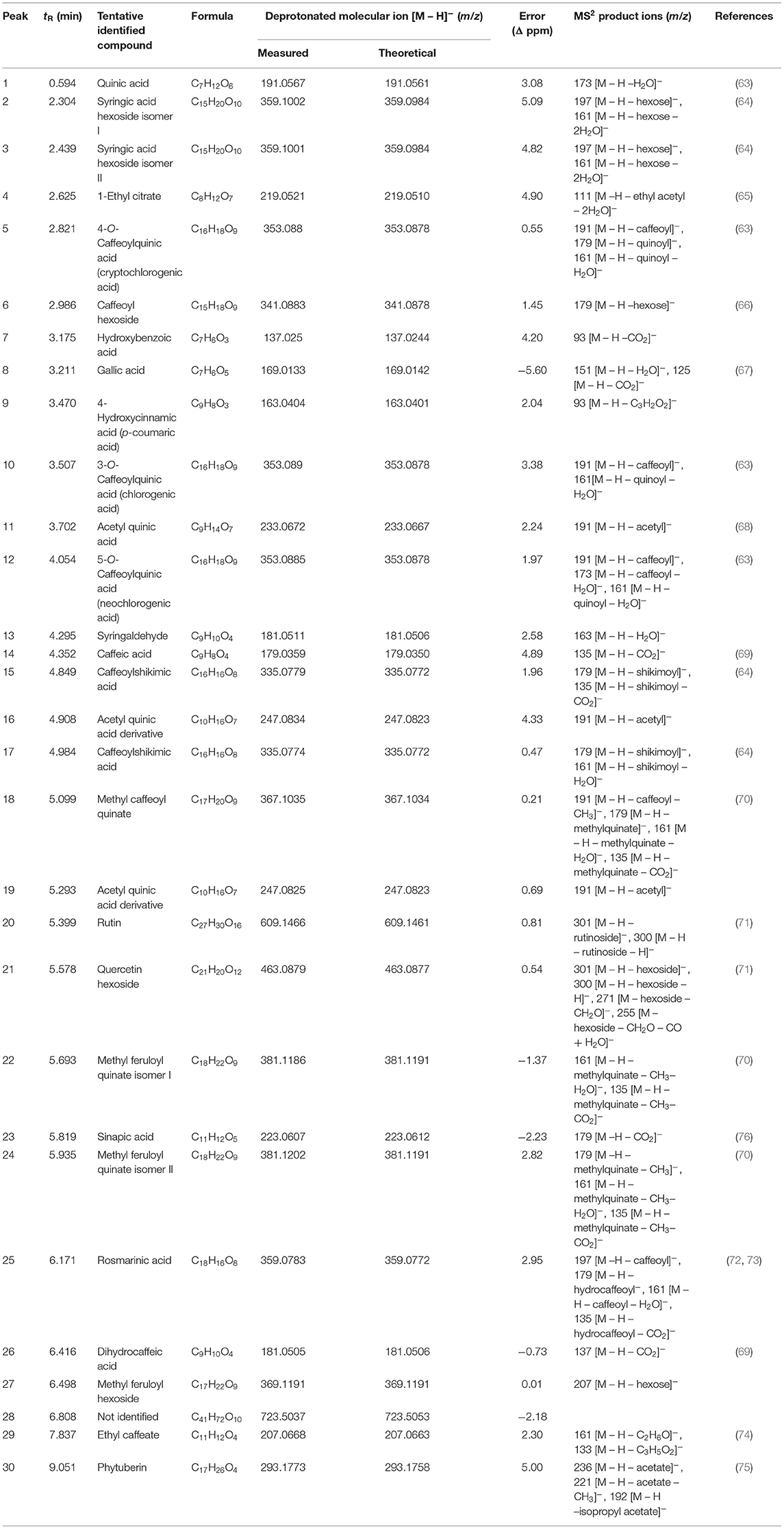
Table 4. Identification of chemical constituents in the T33 extract by liquid chromatography-tandem mass spectrometry (UHPLC-q-TOF-MS/MS).
According to Table 4, the UHPLC-q-TOF-MS/MS analysis of the T33 extract revealed an important group of phenolic acids, such as 4-hydroxycinnamic (9), caffeic (14), rosmarinic (25), and chlorogenic isomer (10, 12) acids, as well as quercetin hexoside (21) and rutin (20) into the flavonoid group that could play an important role in AChE inhibition. In fact, the contribution to the enzyme inhibitory activity attributed to rosmarinic acid (25, 80, 81) and the neuroprotective effect exerted by quercetin, among others (45–47) are widely recognized. According to Dos Santos et al. (62) flavonoids and phenolic compounds seem to act as noncompetitive inhibitors that bind to the peripheral anionic active site of AChE, and they highlight the potential inhibitory effect of quercetin. A study by Oboh et al. (82) suggested that the potential cholinergic enzymatic inhibition of phenolic compounds is a function of the number and position of their OH groups, which forms hydrogen bonds with specific amino acids at the active sites of the enzymes. These authors studied the inhibitory enzymatic activity of caffeic acid and CGAs separately, and they found that caffeic acid exerts higher anti-AChE and anti-BChE capacities than CGA. However, the inhibitory capacity of AChE was decreased when both phenolic acids interact together as a result of the antagonistic effect of chlorogenic on caffeic acid due to the presence of quinic acid moiety on the chlorogenic structure, that could affect the interaction of the phenolic acids with the active site of both AChE and BChE, causing a significant reduction in their enzyme inhibitory activity. The differences between the values reported by some authors and those in this study can be attributed mainly to the tamarillo variety studied as well as the technique and extraction parameters applied. Regarding anti-inflammatory activity, ethyl caffeate (29) has been reported as a bioactive compound with anti-inflammatory properties (74), in addition to quercetin and rutin.
Cytotoxicity and Neurotoxicity Study
Among standard cell lines, human kidney 2 (HK-2) cells are widely used as a model to predict in vitro toxicity in humans (83), so HK-2 cells were chosen in this article in order to study the toxicity effect of the T33 extract as a first step. As it can be observed in Figure 5, the T33 extract presented no toxicity at any concentration tested (7.5 to 120 μg/ml) and even showed an increase in cell viability at the highest T33 concentrations.
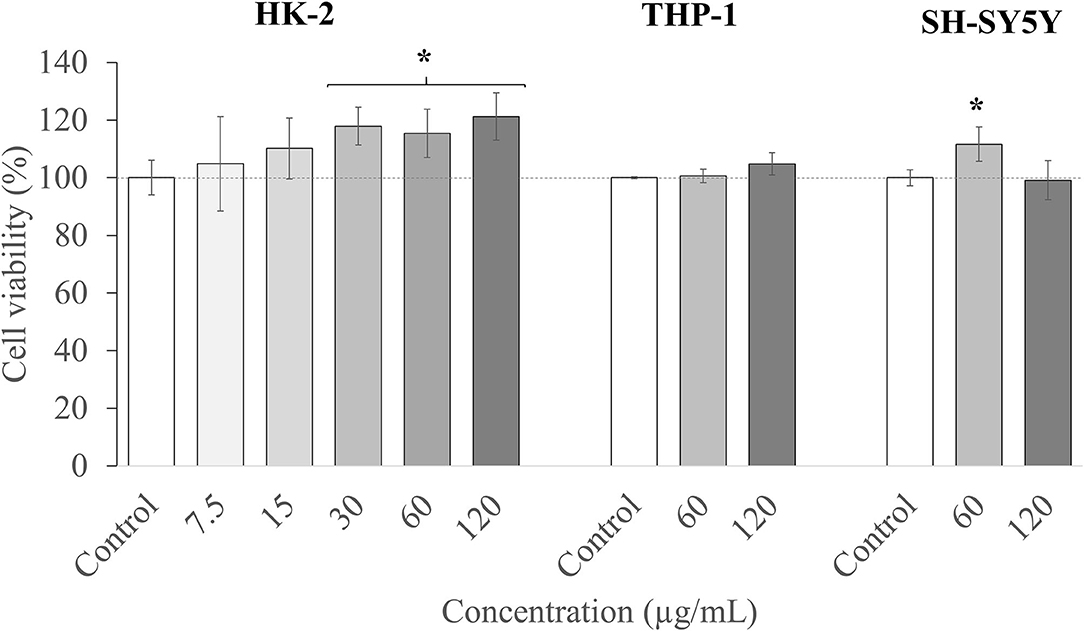
Figure 5. Cell viability in human kidney-2 (HK-2), THP-1, and SH-S5Y5 cell lines upon treatment for 24 h with different concentrations of T33 extract. Error bars are given as the SD of three independent experiments. (*) indicates significant differences between the treated and control samples, p < 0.05.
Thereafter, the two highest nontoxic concentrations (60 and 120 μg/ml) of the extract were selected, and their cytotoxicity was evaluated in two specific cell lines. First, THP-1 cells were used, which are commonly employed to investigate the function and regulation of monocytes and macrophages in the cardiovascular system, and to study functions, mechanisms, and bioactivities of food compounds or pharmacological activities of drugs, among others (84). Besides, and in order to predict any neurotoxic effect of the extract, the neuroblastoma SH-SY5Y cell line was also employed. This model is widely used in in vitro studies of neurological disorders, such as AD, ischemia, or neurotoxicity (85). Moreover, these cells can be differentiated and acquire mature neuron-like features, thus becoming phenotypically closer to primary neurons (40).
As can be observed in Figure 5, the two concentrations tested (60 and 120 μg/ml) maintained the cell viability at the maximum level. To summarize, the T33 extract showed no toxicity (up to 120 μl) for all the tested cell culture lines, and further in vitro and in vivo studies could be performed to investigate its potential bioactivity. It should also be highlighted that the concentrations tested are relatively high compared with other matrices also tested in these models, such as macroalgae Cystoseira usneoides (86), or Thymbra capitata and Thymus Species (87), with toxicity reported in THP-1 cells at concentrations above 8 μg/ml.
Conclusions
This study reported the potential of the tamarillo (C. betacea) epicarp as a promising source of bioactive extracts. TE extracts obtained by PLE have demonstrated, for the first time, their antioxidant, anti-inflammatory, and anti-cholinergic inhibitory activities. These extracts showed high phenolic, flavonoid, and carotenoid contents. The most active extract showed no toxicity in all the tested cell lines. The analysis of the optimum TE extract by UHPLC-q-TOF-MS/MS showed an important presence of hydroxycinnamic acid derivatives and other phenolic acids as well as quercetin hexoside and rutin as bioactive metabolites. Therefore, the results suggest that the TE subjected to PLE could become a promising natural by-product with multitarget activity against AD. This study attempts to approach sustainability and improvement of the quality of life from different perspectives. On one hand, the reduction of food waste through the development of environmentally friendly technologies is able to achieve improved and high added value extracts; on the other hand, the possibility of designing extracts with multitarget activity against important diseases, such as AD. The obtained results corroborate the interest of both, TE as a by-product and PLE as technology, to achieve promising extracts with confirmed bioactivities and composition, and represent a step forward toward the development of therapies based on natural products.
Data Availability Statement
The datasets presented in this study can be found in online repositories. The names of the repository/repositories and accession number(s) can be found below: EBI MetaboLites, accession no: MTBLS3489.
Author Contributions
ZS-M, FP-A, EI, and AC designed the experiments. ZS-M, FP-A, AV, RG, and JS-M performed the experiments. ZS-M and FP-A wrote the original draft. ZS-M, FP-A, DB-V, AV, and RG analyzed the data. EI and AC acquired funding and reviewed and edited the article. All authors contributed to the article and approved the submitted version.
Funding
This research was funded by the Ministry of Economy and Competitiveness (MINECO), Spain, project AGL2017-89417-R and PID2020-113050RB-I00.
Conflict of Interest
The authors declare that the research was conducted in the absence of any commercial or financial relationships that could be construed as a potential conflict of interest.
Publisher's Note
All claims expressed in this article are solely those of the authors and do not necessarily represent those of their affiliated organizations, or those of the publisher, the editors and the reviewers. Any product that may be evaluated in this article, or claim that may be made by its manufacturer, is not guaranteed or endorsed by the publisher.
Acknowledgments
ZS-M would like to acknowledge the Universidad de Nariño for granting commission for doctoral studies. AV would like to acknowledge the Spanish Ministry of Science, Innovation and Universities for his Juan de la Cierva post-doctoral grant (IJC2018-037560-I). JS-M would like to acknowledge the Spanish Ministry of Science and Innovation and Universities for an FPU predoctoral grant FPU17/01876. DB-V and FP-A thank the DIEB at the Universidad Nacional de Colombia for financial support (51274 project).
Supplementary Material
The Supplementary Material for this article can be found online at: https://www.frontiersin.org/articles/10.3389/fnut.2021.769617/full#supplementary-material
References
1. Ramírez F. Kallarackal J. Tree tomato (Solanum betaceum Cav) reproductive physiology: A review. Sci Hortic (Amsterdam). (2019) 248:206–15. doi: 10.1016/j.scienta.2019.01.019
2. Palacio PAE. Lineamientos y estrategias de articulación de ASOHOFRUCOL con la Agroindustria en pro del desarrollo hortifrutícola en Colombia. Asoc Hortofrutícola Colomb. (2017) 60. Available online at: https://sioc.minagricultura.gov.co/DocumentosContexto/S2561-LineamientosASOHOFRUCOL.pdf
3. Prohens J, Nuez F. The tamarillo (Cyphomandra betacea): A review of a promising small fruit crop. Small Fruits Rev. (2001) 1:43–68. doi: 10.1300/J301v01n02_06
4. Moreno-Miranda C, Molina JI, Ortiz J, Peñafiel C, Moreno R. The value chain of tree tomato (Solanum betaceum) network in Ecuador. Agron Mesoamerican. (2020) 31:13–29. doi: 10.15517/AM.V31I1.36887
5. Castro-Vargas HI, Benelli P, Ferreira SRS, Parada-Alfonso F. Supercritical fluid extracts from tamarillo (Solanum betaceum Sendtn) epicarp and its application as protectors against lipid oxidation of cooked beef meat. J Supercrit Fluids. (2013) 76:17–23. doi: 10.1016/j.supflu.2012.10.006
6. Vasco C, Avila J, Ruales J, Svanberg U. Kamal-Eldin A. Physical and chemical characteristics of golden-yellow and purple-red varieties of tamarillo fruit (Solanum betaceum Cav). Int J Food Sci Nutr. (2009) 60:278–88. doi: 10.1080/09637480903099618
7. Wang S, Zhu F. Tamarillo (Solanum betaceum): Chemical composition, biological properties, and product innovation. Trends Food Sci Technol. (2020) 95:45–58. doi: 10.1016/j.tifs.2019.11.004
8. Martin D, Lopes T, Correia S, Canhoto J, Marques MPM, Batista de. Carvalho LAE. Nutraceutical properties of tamarillo fruits: A vibrational study Spectrochim Acta - Part A. Mol Biomol Spectrosc. (2021) 252:119501. doi: 10.1016/j.saa.2021.119501
9. Meza N. Manzano Méndez J. Características del fruto de tomate de árbol (Cyphomandra betaceae [Cav] Sendtn) basadas en la coloración del arilo, en la Zona Andina Venezolana. Rev Cient UDO Agric. (2009) 9:289–94.
10. Torres A. Caracterización física química y compuestos bioactivos de pulpa madura de tomate de árbol (Cyphomandra betacea) (Cav). Sendtn Arch Latinoam Nutr. (2012) 62:381–8.
11. Abdul Kadir NAA, Rahmat A, Jaafar HZE. Protective effects of tamarillo (cyphomandra betacea) extract against high fat diet induced obesity in sprague-dawley rats. J Obes. (2015) 2015:846041. doi: 10.1155/2015/846041
12. Ordóñez RM, Ordóñez AAL, Sayago JE, Nieva Moreno MI, Isla MI. Antimicrobial activity of glycosidase inhibitory protein isolated from Cyphomandra betacea Sendt. fruit. Peptides. (2006) 27:1187–91. doi: 10.1016/j.peptides.2005.11.016
13. Kou M-C, Yen J-H, Hong J-T, Wang C-L, Lin C-W, Wu M-J. Cyphomandra betacea Sendt. phenolics protect LDL from oxidation and PC12 cells from oxidative stress. (2009) 42:458–63 doi: 10.1016/j.lwt.2008.09.010
14. Stangeland T, Remberg SF, Lye KA. Total antioxidant activity in 35 Ugandan fruits and vegetables. Food Chem. (2009) 113:85–91. doi: 10.1016/j.foodchem.2008.07.026
15. C. SK, M. S, K. R. Microwave-assisted extraction of polysaccharides from Cyphomandra betacea and its biological activities. Int J Biol Macromol. (2016) 92:682–93. doi: 10.1016/j.ijbiomac.2016.07.062
16. Orqueda ME, Rivas M, Zampini IC, Alberto MR, Torres S, Cuello S, et al. Chemical and functional characterization of seed, pulp and skin powder from chilto (Solanum betaceum), an Argentine native fruit. Phenolic fractions affect key enzymes involved in metabolic syndrome and oxidative stress. Food Chem. (2017) 216:70–9. doi: 10.1016/j.foodchem.2016.08.015
17. Dorado Achicanoy D, Hurtado Benavides A, Martínez-Correa HA. Study of supercritical CO2 extraction of tamarillo (Cyphomandra Betacea) seed oil containing high added value compounds. Electrophoresis. (2018) 39:1917–25. doi: 10.1002/elps.201700430
18. Castro-Vargas HI, Parada-Alfonso F. Avaliação do efeito protetor contra oxidação lipídica de frações obtidas a partir da casca de tomate da árvore (Solanum betaceum Sendtn). Rev Colomb Quim. (2017) 46:17–23. doi: 10.15446/rev.colomb.quim.v46n2.62988
19. Santos TRJ, de Aquino Santana LCL. Antimicrobial potential of exotic fruits residues. South African J Bot. (2019) 124:338–44. doi: 10.1016/j.sajb.2019.05.031
20. Guzman-Martinez L, Calfío C, Farias GA, Vilches C, Prieto R, Maccioni RB. New Frontiers in the Prevention, Diagnosis, and Treatment of Alzheimer's Disease. J Alzheimer's Dis. (2021) 1–13. doi: 10.3233/jad-201059
21. Atlante A, Amadoro G, Bobba A, Latina V. Functional foods: an approach to modulate molecular mechanisms of Alzheimer's disease. Cells. (2020) 9:2347. doi: 10.3390/cells9112347
22. Pérez-Hernández J, Zaldívar-Machorro VJ, Villanueva-Porras D, Vega-Ávila E, Chavarría A. A Potential Alternative against Neurodegenerative Diseases: Phytodrugs. Oxid Med Cell Longev. (2016) 2016:8378613. doi: 10.1155/2016/8378613
23. Kerch G. The potential of chitosan and its derivatives in prevention and treatment of Age-related diseases. Mar Drugs. (2015) 13:2158–82. doi: 10.3390/md13042158
24. Rafiquzzaman SM, Kim EY, Lee JM, Mohibbullah M, Alam MB, Soo Moon I, et al. Anti-Alzheimers and anti-inflammatory activities of a glycoprotein purified from the edible brown alga Undaria pinnatifida. Food Res Int. (2015) 77:118–24. doi: 10.1016/j.foodres.2015.08.021
25. Sangha JS, Wally O, Banskota AH, Stefanova R, Hafting JT, Critchley AT, et al. cultivated form of a red seaweed (Chondrus crispus), suppresses β-amyloid-induced paralysis in caenorhabditis elegans. Mar Drugs. (2015) 13:6407–24. doi: 10.3390/md13106407
26. Sánchez-Camargo AP, Mendiola JA, Valdés A, Castro-Puyana M, García-Cañas V, Cifuentes A, et al. Supercritical antisolvent fractionation of rosemary extracts obtained by pressurized liquid extraction to enhance their antiproliferative activity. J Supercrit Fluids. (2016) 107:581–9. doi: 10.1016/j.supflu.2015.07.019
27. Brazdauskas T, Montero L, Venskutonis PR, Ibañez E, Herrero M. Downstream valorization and comprehensive two-dimensional liquid chromatography-based chemical characterization of bioactives from black chokeberries (Aronia melanocarpa) pomace. J Chromatogr A. (2016) 1468:126–35. doi: 10.1016/j.chroma.2016.09.033
28. García Martínez E, Fernández Segovia I, Fuentes López A. Determinación de polifenoles totales por el método de Folin- Ciocalteu. Etsiamn. (2015).
29. García Martínez E, Fernández Segovia I, Fuentes López A. Determinación de polifenoles totales por el método de Folin- Ciocalteu. Etsiamn. (2015) 9.
30. Gilbert-López B, Mendiola JA, Fontecha J, Van Den Broek LAM, Sijtsma L, Cifuentes A, et al. Downstream processing of Isochrysis galbana: a step towards microalgal biorefinery. Green Chem. (2015) 17:4599–609. doi: 10.1039/c5gc01256b
31. Suárez Montenegro ZJ, Álvarez-Rivera G, Sánchez-Martínez JD, Gallego R, Valdés A, Bueno M, et al. Neuroprotective effect of terpenoids recovered from olive oil by-products. Foods. (2021) 10:1507. doi: 10.3390/foods10071507
32. Re R, Pellegrini N, Proteggente A, Pannala A, Yang M, Rice-Evans C. Antioxidant activity applying an improved ABTS radical cation decolorization assay. Free Radic Biol Med. (1999) 26:1231–7. doi: 10.1016/S0891-5849(98)00315-3
33. Ou B, Hampsch-Woodill M, Prior RL. Development and validation of an improved oxygen radical absorbance capacity assay using fluorescein as the fluorescent probe. J Agric Food Chem. (2001) 49:4619–26. doi: 10.1021/jf010586o
34. Ho SC, Tang YL, Lin SM, Liew YF. Evaluation of peroxynitrite-scavenging capacities of several commonly used fresh spices. Food Chem. (2010) 119:1102–7. doi: 10.1016/j.foodchem.2009.08.020
35. Whent M, Ping T, Kenworthy W, Liangli A, Yu L. High-throughput assay for detection of soybean lipoxygenase-1. J Agric Food Chem. (2010) 58:12602–7. doi: 10.1021/jf1028784
36. Worek F, Eyer P, Thiermann H. Determination of acetylcholinesterase activity by the Ellman assay: A versatile tool for in vitro research on medical countermeasures against organophosphate poisoning. Drug Test Anal. (2012) 4:282–91. doi: 10.1002/dta.337
37. Sánchez-Martínez JD, Bueno M, Alvarez-Rivera G, Tudela J, Ibañez E, Cifuentes A. In vitro neuroprotective potential of terpenes from industrial orange juice by-products. Food Funct. (2021) 12:302–14. doi: 10.1039/d0fo02809f
38. Mosmann T. Rapid colorimetric assay for cellular growth and survival: Application to proliferation and cytotoxicity assays. J Immunol Methods. (1983) 65:55–63. doi: 10.1016/0022-1759(83)90303-4
39. Villalva M, Jaime L, Aguado E, Nieto JA, Reglero G, Santoyo S. Anti-inflammatory and antioxidant activities from the basolateral fraction of caco-2 cells exposed to a rosmarinic acid enriched extract. J Agric Food Chem. (2018) 66:1167–74. doi: 10.1021/acs.jafc.7b06008
40. de Medeiros LM, De Bastiani MA, Rico EP, Schonhofen P, Pfaffenseller B, Wollenhaupt-Aguiar B, et al. Cholinergic differentiation of human neuroblastoma SH-SY5Y cell line and its potential use as an in vitro model for Alzheimer's disease studies. Mol Neurobiol. (2019) 56:7355–67. doi: 10.1007/s12035-019-1605-3
41. Hassan SHA, Bakar MFA. Antioxidative and anticholinesterase activity of cyphomandra betacea fruit. Sci World J. (2013) 2013:278071. doi: 10.1155/2013/278071
42. Skinner SJM, Hunter D, Cho S, Skinner M. The Potential Health Benefits of the Subtropical Fruits Kiwifruit, Feijoa and Tamarillo. In: Bioactives in Fruit. John Wiley & Sons, Ltd; Wiley Blackwell. (2013). p. 169–195. doi: 10.1002/9781118635551.ch8
43. Diep TT, Pook C. Yoo MJY. Physicochemical properties and proximate composition of tamarillo (Solanum betaceum Cav) fruits from New Zealand. J Food Compos Anal. (2020) 92:103563. doi: 10.1016/j.jfca.2020.103563
44. Abdul Mutalib M, Rahmat A, Ali F, Othman F, Ramasamy R. Nutritional compositions and antiproliferative activities of different solvent fractions from ethanol extract of cyphomandra betacea (Tamarillo) fruit. Malaysian J Med Sci. (2017) 24:19–32. doi: 10.21315/mjms2017.24.5.3
45. Kim HP, Ho Son K, Chang HW, Kang SS. Anti-inflammatory Plant flavonoids and cellular action mechanisms. J Pharmacol Sci. (2004) 96:229–45. doi: 10.1254/jphs.CRJ04003X
46. Nijveldt RJ, Van Nood E, Van Hoorn DEC, Boelens PG, Van Norren K, Van Leeuwen PAM. Flavonoids: A review of probable mechanisms of action and potential applications. Am J Clin Nutr. (2001) 74:418–25. doi: 10.1093/ajcn/74.4.418
47. Mutha RE, Tatiya AU, Surana SJ. Flavonoids as natural phenolic compounds and their role in therapeutics: an overview. Futur J Pharm Sci. (2021) 7:1–13. doi: 10.1186/s43094-020-00161-8
48. Negahdari R, Bohlouli S, Sharifi S, Maleki Dizaj S, Rahbar Saadat Y, Khezri K, et al. Therapeutic benefits of rutin and its nanoformulations. Phyther Res. (2021) 35:1719–38. doi: 10.1002/ptr.6904
49. Halevas E, Mavroidi B, Pelecanou M, Hatzidimitriou AG. Structurally characterized zinc complexes of flavonoids chrysin and quercetin with antioxidant potential. Inorganica Chim Acta. (2021) 523:120407. doi: 10.1016/j.ica.2021.120407
50. de Lacerda Alexandre JV, Viana YIP, David CEB, Cunha PLO, Albuquerque AC, Varela ALN, et al. Quercetin treatment increases H2O2 removal by restoration of endogenous antioxidant activity and blocks isoproterenol-induced cardiac hypertrophy. Naunyn Schmiedebergs. Arch Pharmacol. (2021) 394:217–26. doi: 10.1007/s00210-020-01953-8
51. Aquilano K, Baldelli S, Rotilio G, Ciriolo MR. Role of nitric oxide synthases in Parkinson's disease: A review on the antioxidant and anti-inflammatory activity of polyphenols. Neurochem Res. (2008) 33:2416–26. doi: 10.1007/s11064-008-9697-6
52. Ramassamy C. Emerging role of polyphenolic compounds in the treatment of neurodegenerative diseases: A review of their intracellular targets. Eur J Pharmacol. (2006) 545:51–64. doi: 10.1016/j.ejphar.2006.06.025
53. Tian C, Liu X, Chang Y, Wang R, Lv T, Cui C, et al. Investigation of the anti-inflammatory and antioxidant activities of luteolin, kaempferol, apigenin and quercetin. South African J Bot. (2021) 137:257–64. doi: 10.1016/j.sajb.2020.10.022
54. Shoskes D, Lapierre C, Cruz-Corerra M, Muruve N, Rosario R, Fromkin B, et al. Beneficial effects of the bioflavonoids curcumin and quercetin on early function in cadaveric renal transplantation: A randomized placebo controlled trial. Transplantation. (2005) 80:1556–9. doi: 10.1097/01.tp.0000183290.64309.21
55. Lomozová Z, Catapano MC, Hrubša M, Karlíčková J, Macáková K, Kučera R, et al. Chelation of iron and copper by quercetin b-ring methyl metabolites, isorhamnetin and tamarixetin, and their effect on metal-based fenton chemistry. J Agric Food Chem. (2021) 69:5926–37. doi: 10.1021/acs.jafc.1c01729
56. Ghosh S, Chatterjee JK, Chalkroborty B, Kundu P. Estimation of beta carotene from fruit peel wastes by high performance thin layer chromatography. J Pharmacogn Phytochem. (2019) 8:2598–600. Available at: https://www.researchgate.net/publication/333449044
57. Diep TT, Pook C, Rush EC. Yoo MJY. Quantification of carotenoids, α-tocopherol, and ascorbic acid in amber, mulligan, and laird's large cultivars of New Zealand tamarillos (Solanum betaceum Cav). Foods. (2020) 9:1–16. doi: 10.3390/foods9060769
58. De Lucas A, Martinez De La Ossa E, Rincó N A J, Blanco MA, Gracia I. Supercritical fluid extraction of tocopherol concentrates from olive tree leaves. (2002). Available oonline at: www.elsevier.com/locate/supflu (accessed October 31, 2020).
59. Ferri P, Angelino D, Gennari L, Benedetti S, Ambrogini P, Del Grande P, et al. Enhancement of flavonoid ability to cross the blood-brain barrier of rats by co-administration with α-tocopherol. Food Funct. (2015) 6:394–400. doi: 10.1039/c4fo00817k
60. Suárez Montenegro ZJ, Álvarez-Rivera G, Mendiola JA, Ibáñez E, Cifuentes A. Extraction and mass spectrometric characterization of terpenes recovered from olive leaves using a new adsorbent-assisted supercritical CO2 process. Foods. (2021) 10:1301. doi: 10.3390/foods10061301
61. Li N. Li W. Cytotoxicity and anti-inflammatory activity of tamarillo (Solanum betaceum Cav) peel extract in lipopolysaccharide stimulated RAW 2647 cells. e-GiGi. (2021) 9:92–8. doi: 10.35790/eg.9.1.2021.32847
62. Dos Santos TC, Gomes TM, Pinto BAS, Camara AL, De Andrade Paes AM. Naturally occurring acetylcholinesterase inhibitors and their potential use for Alzheimer's disease therapy. Front Pharmacol. (2018) 9:1–14. doi: 10.3389/fphar.2018.01192
63. Masike K, Mhlongo MI, Mudau SP, Nobela O, Ncube EN, Tugizimana F, George MJ, Madala NE. Highlighting mass spectrometric fragmentation differences and similarities between hydroxycinnamoyl-quinic acids and hydroxycinnamoyl-isocitric acids. Chem Cent J. (2017) 11:1–7. doi: 10.1186/S13065-017-0262-8
64. Sun L, Tao S, Zhang S. Characterization and quantification of polyphenols and triterpenoids in thinned young fruits of ten pear varieties by UPLC-Q TRAP-MS/MS. Molecule. (2019) 24:159. doi: 10.3390/molecules24010159
65. Pierson JT, Monteith GR, Roberts-Thomson SJ, Dietzgen RG, Gidley MJ. Shaw PN. Phytochemical extraction, characterisation and comparative distribution across four mango (Mangifera indica L) fruit varieties. Food Chem. (2014) 149:253–63. doi: 10.1016/j.foodchem.2013.10.108
66. Crupi P, Bleve G, Tufariello M, Corbo F, Clodoveo ML, Tarricone L. Comprehensive identification and quantification of chlorogenic acids in sweet cherry by tandem mass spectrometry techniques. J Food Compos Anal. (2018) 73:103–11. doi: 10.1016/J.JFCA.2018.06.013
67. Singh A, Bajpai V, Kumar S, Sharma KR, Kumar B. Profiling of gallic and ellagic acid derivatives in different plant parts of Terminalia arjuna by HPLC-ESI-QTOF-MS/MS. Nat Prod Commun. (2016) 11:239–44. doi: 10.1177/1934578x1601100227
68. Pacifico S, Galasso S, Piccolella S, Kretschmer N, Pan SP, Nocera P, et al. Winter wild fennel leaves as a source of anti-inflammatory and antioxidant polyphenols. Arab J Chem. (2018) 11:513–24. doi: 10.1016/J.ARABJC.2015.06.026
69. Narváez-Cuenca CE, Vincken JP, Zheng C, Gruppen H. Diversity of (dihydro) hydroxycinnamic acid conjugates in Colombian potato tubers. Food Chem. (2013) 139:1087–97. doi: 10.1016/J.FOODCHEM.2013.02.018
70. Jaiswal R, Kuhnert N. How to identify and discriminate between the methyl quinates of chlorogenic acids by liquid chromatography-tandemmass spectrometry. J Mass Spectrom. (2011) 46:269–81. doi: 10.1002/JMS.1889
71. Kumar S, Singh A, Kumar B. Identification and characterization of phenolics and terpenoids from ethanolic extracts of Phyllanthus species by HPLC-ESI-QTOF-MS/MS. J Pharm Anal. (2017) 7:214–22. doi: 10.1016/J.JPHA.2017.01.005
72. Lecomte J, Giraldo LJL, Laguerre M, Baréa B, Villeneuve P. Synthesis, characterization and free radical scavenging properties of rosmarinic acid fatty esters. JAOCS, J Am Oil Chem Soc. (2010) 87:615–20. doi: 10.1007/S11746-010-1543-8
73. Møller JKS, Catharino RR, Eberlin MN. Electrospray ionization mass spectrometry fingerprinting of essential oils: Spices from the Labiatae family. Food Chem. (2007) 100:1283–8. doi: 10.1016/J.FOODCHEM.2005.10.013
74. Barth C da S, Souza HGT de, Rocha LW, Madeira CR de S, Assis C, Bonomini T, et al. RP-HPLC and LC–MS–MS determination of a bioactive artefact from Ipomoea pes-caprae extract. Rev Bras Farmacogn. (2019) 29:570–7. doi: 10.1016/J.BJP.2019.05.005
75. Serralheiro ML, Guedes R, Fadel SR, Bendif H. Data on identification of primary and secondary metabolites in aqueous extract of Verbascum betonicifolium. Data Br. (2020) 32: 106146. doi: 10.1016/J.DIB.2020.106146
76. Ma C, Whitaker BD, Kennelly EJ. New 5-O-caffeoylquinic acid derivatives in fruit of the wild eggplant relative solanum viarum. J Agric Food Chem. (2010) 58:11036–42. doi: 10.1021/JF102963F
77. Eich E. Terpenoids (Isoprenoids). Solanaceae Convolvulaceae Second Metab. (2008) 343–523. doi: 10.1007/978-3-540-74541-9_7
78. Espin S, Gonzalez-Manzano S, Taco V, Poveda C, Ayuda-Durán B, Gonzalez-Paramas AM. Santos-Buelga C. Phenolic composition and antioxidant capacity of yellow and purple-red Ecuadorian cultivars of tree tomato (Solanum betaceum Cav). Food Chem. (2016) 194:1073–80. doi: 10.1016/j.foodchem.2015.07.131
79. Loizzo MR, Lucci P, Núñez O, Tundis R, Balzano M, Frega NG, et al. Native Colombian fruits and their by-products: Phenolic profile, antioxidant activity and hypoglycaemic potential. Foods. (2019) 8:89. doi: 10.3390/foods8030089
80. Cutillas AB, Carrasco A, Martinez-Gutierrez R, Tomas V, Tudela J. Rosmarinus officinalis L. essential oils from Spain: composition, antioxidant capacity, lipoxygenase and acetylcholinesterase inhibitory capacities, and antimicrobial activities. Plant Biosyst. (2018) 152:1282–92. doi: 10.1080/11263504.2018.1445129
81. Ozarowski M, Mikolajczak PL, Bogacz A, Gryszczynska A, Kujawska M, Jodynis-Liebert J, et al. Rosmarinus officinalis L. leaf extract improves memory impairment and affects acetylcholinesterase and butyrylcholinesterase activities in rat brain. Fitoterapia. (2013) 91:261–71. doi: 10.1016/j.fitote.2013.09.012
82. Oboh G, Agunloye OM, Akinyemi AJ, Ademiluyi AO, Adefegha SA. Comparative study on the inhibitory effect of caffeic and chlorogenic acids on key enzymes linked to Alzheimer's disease and some pro-oxidant induced oxidative stress in rats' brain-in vitro. Neurochem Res. (2013) 38:413–9. doi: 10.1007/s11064-012-0935-6
83. Gunness P, Aleksa K, Kosuge K, Ito S, Koren G. Comparison of the novel HK-2 human renal proximal tubular cell line with the standard LLC-PK1 cell line in studying drug-induced nephrotoxicity. Can J Physiol Pharmacol. (2010) 88:448–55. doi: 10.1139/Y10-023
84. Chanput W, Mes JJ, Wichers HJ. THP-1 cell line: An in vitro cell model for immune modulation approach. Int Immunopharmacol. (2014) 23:37–45. doi: 10.1016/j.intimp.2014.08.002
85. Peng Y, Chu S, Yang Y, Zhang Z, Pang Z, Chen N. Neuroinflammatory in vitro cell culture models and the potential applications for neurological disorders. Front Pharmacol. (2021) 12: 671734. doi: 10.3389/fphar.2021.671734
86. Zbakh H, Zubía E, Reyes C de. los, Calderón-Montaño JM, López-Lázaro M, Motilva V. Meroterpenoids from the brown alga cystoseira usneoides as potential anti-inflammatory and lung anticancer agents. Mar Drugs. (2020) 18:207. doi: 10.3390/md18040207
Keywords: neuroprotective activity, Alzheimer's disease, Cyphomandra betacea, tropical fruit by-product, green extraction, pressurized liquid extraction, polyphenol extracts
Citation: Suárez-Montenegro ZJ, Ballesteros-Vivas D, Gallego R, Valdés A, Sánchez-Martínez JD, Parada-Alfonso F, Ibáñez E and Cifuentes A (2021) Neuroprotective Potential of Tamarillo (Cyphomandra betacea) Epicarp Extracts Obtained by Sustainable Extraction Process. Front. Nutr. 8:769617. doi: 10.3389/fnut.2021.769617
Received: 02 September 2021; Accepted: 28 September 2021;
Published: 15 November 2021.
Edited by:
Maria Fernanda Silva, Universidad Nacional de Cuyo, ArgentinaReviewed by:
Filomena Nazzaro, National Research Council (CNR), ItalyKin Weng Kong, University of Malaya, Malaysia
Copyright © 2021 Suárez-Montenegro, Ballesteros-Vivas, Gallego, Valdés, Sánchez-Martínez, Parada-Alfonso, Ibáñez and Cifuentes. This is an open-access article distributed under the terms of the Creative Commons Attribution License (CC BY). The use, distribution or reproduction in other forums is permitted, provided the original author(s) and the copyright owner(s) are credited and that the original publication in this journal is cited, in accordance with accepted academic practice. No use, distribution or reproduction is permitted which does not comply with these terms.
*Correspondence: Fabián Parada-Alfonso, ZnBhcmFkYWEmI3gwMDA0MDt1bmFsLmVkdS5jbw==; Elena Ibáñez, ZWxlbmEuaWJhbmV6JiN4MDAwNDA7Y3NpYy5lcw==