- 1College of Animal Science and Technology, Hunan Agricultural University, Changsha, China
- 2Chemistry Department, University of Liverpool, Liverpool, United Kingdom
Guanidinoacetic acid is the direct precursor of creatine and its phosphorylated derivative phosphocreatine in the body. It is a safe nutritional supplement that can be used to promote muscle growth and development. Improving the growth performance of livestock and poultry and meat quality is the eternal goal of the animal husbandry, and it is also the common demand of today's society and consumers. A large number of experimental studies have shown that guanidinoacetic acid could improve the growth performance of animals, promote muscle development and improve the health of animals. However, the mechanism of how it affects muscle development needs to be further elucidated. This article discusses the physical and chemical properties of guanidinoacetic acid and its synthesis pathway, explores its mechanism of how it promotes muscle development and growth, and also classifies and summarizes the impact of its application in animal husbandry, providing a scientific basis for this application. In addition, this article also proposes future directions for the development of this substance.
Introduction
The body composition of mammals includes skin, muscle, fat and bones, among which the proportion of skeletal muscle is more than 40%, making it the largest organ in the body. In addition to maintaining exercise capacity, body balance and respiratory function, skeletal muscle also acts as an endocrine organ to secrete a variety of cytokines to mediate the interaction between cells and perform diverse biological functions (1, 2).
The basic functional unit of skeletal muscle is myofiber, and its development is closely related to the identification and differentiation of myoblasts (3). The amount of protein consisted in myofibers is about 50–75% of the total amount of protein in the animal body. Under normal physiological conditions, the protein synthesis and degradation rates of myofibers are in a relatively balanced state. When the state is out of balance, the efficiency of protein synthesis is higher than degradation, an overall outcome of increased muscle mass will be shown. No matter humans or animals, there are many factors that may disturb the equilibrium of the state, including nutrition regulation, exogenous nutrient intervention, and regular exercise. The method of exogenous nutrient intervention has gradually grabbed increasingly attention in this field (4).
It is well-known that guanidinoacetic acid (GAA) could be used as a nutritional supplement to promote muscle development and increase the body's energy reserves. Human dietary supplementation with GAA helps to increase muscle strength and endurance, and enhance athletic performance and release fatigue (5). Currently, creatine (Cr) and its precursor GAA have also been used in medical research for the treatment of muscle atrophy and neurodegenerative diseases (6). Furthermore, with the continuous improvement of people's living standards, the dietary structure has undergone significant changes, and the demand for meat product shifted from being quantity-satisfying to quality-ensuring. Therefore, improving the quality of meat products is an important task of today's animal husbandry (7, 8). The addition of GAA in animal diets helps to delay the rate of glycolysis on the basis of increasing muscle production so that the meat quality could be improved (9, 10). However, at present, the mechanism of GAA in promoting myofiber development and growth has not been fully elucidated. This article reviews the physical and chemical properties, mode of action, application effects and prospective development of GAA. It is expected to provide a theoretical basis for the application of GAA in human health and animal husbandry production.
Properties of Guanidinoacetic Acid
GAA is an immediate precursor substance of Cr and its phosphorylated derivative (phosphocreatine, Cr-P) synthesized in the body of animals (11). It was first isolated from the urine of humans and dogs. Cr accepts pyrophosphate from adenosine triphosphate (ATP) and reversibly form Cr-P. Cr and Cr-P form a creatine pool together, which plays a key role in the process of energy storage and utilization. As an energy transporter, Cr has higher mobility than ATP (12, 13). About 70 years ago, medical scientists managed to use GAA to treat human metabolic disorders and improve the working ability of manual workers, which directly proved that GAA has the effect in assisting the treatment of certain diseases (14).
With the progress of research, the physico-chemical properties of GAA have been analyzed in detail. Industrial GAA usually appears as white or off-white crystalline powder without pungent odor. The chemical formula is C3H7N3O2 (relative molecular mass = 117.11 g/mol), and the structural formula is shown in Figure 1. When the sample temperature reaches 190°C, the chemical structure of GAA undergoes thermal decomposition, and the crystal melts as the temperature rises above 284°C. GAA has a high degree of stability in water, and the shelf life can be as long as 2 years, which also makes GAA more widely used than Cr (15).
The safety of GAA and Cr have been verified, and they have entered the public eyes as health care products and are favored by bodybuilders (16). Short or long-term intake of Cr will not harm kidney function or increase the risk of getting kidney disease, but exogenous intake of Cr supplementation is not recommended to somebody who has already been diagnosed with kidney disease (17). Other studies have also shown that Cr supplementation can weaken the excitatory of parasympathetic nerve of the heart, and the autonomic dysfunction may arise in even severe cases (18). However, in the process of livestock and poultry breeding, these substances are used to improve animal-growth performance and facilitate their muscle development and accumulation.
Synthesis and Metabolism of Guanidinoacetic Acid
GAA is a precursor of Cr, an important compound in high-energy phosphate bioenergetics. Its synthesis in the body mainly occurs in the kidneys, it is also synthesized in other tissues such as the pancreas, liver, and muscle (19). The latest research hypothesized that the bacterial flora in the healthy gut can accelerate the synthesis of GAA by secreting enzyme guanidinoacetase (synthesis and hydrolysis occur simultaneously, synthesis > hydrolysis). If this hypothesis is verified, then there's a valid connection between the synthesis of GAA and microorganisms biological functions (20).
Generally speaking, the synthesis of GAA requires the presence of glycine and L-arginine. Under the catalysis of L-arginine:glycine amidinotransferase (AGAT), the two undergo amidino transfer to produce L-ornithine and GAA. GAA travels to the liver by the blood circulation through the portal vein, which sets the basis for the further formation of Cr. The source of GAA in the body comes from endogenous synthesis and food supplementation (negligible, 10 mg/kg of meat), while the consumption of GAA is caused by the synthesis of Cr and excretion by urine. The aim is to keep the GAA content stable at 2.6 ± 0.8 umol/L, which also constituted a theoretical model of GAA homeostasis (21). In the next step, GAA and S-adenosyl-methionine (SAM) catalyzed by guanidinoacetate N-methyltransferase (GAMT), in which GAA combined with a methyl group to generate Cr, and released S-adenosyl-homocysteine (SAH) at meantime. This process mainly occurred in the liver, but also in the skeletal muscle, spleen, brain and genital organs (22). Finally, Cr is released into the blood circulation and entered the cell through a specific transporter SLC6A8 (a Na+/Cl− creatine co-transporter) located on the cell membrane (23). Since Cr can regulate the expression of AGAT through a counter-regulatory mechanism but cannot counter-regulate the expression of GAMT, the synthesis order of GAA and Cr cannot be reversed (24). In cells, Cr and ATP undergo a reversible reaction with the catalysis of creatine kinase (CK) to generate Cr-P and adenosine diphosphate (ADP) for energy storage (25). The body continues to metabolize Cr and Cr-p, and about 1.7% of them are metabolized into the final product—creatinine, which is transported from the blood to the kidneys and is completely filtered by the glomerulus, and is excreted in the urine. It is an important indicator of kidney function testing and is also commonly used to diagnosis of certain kidney diseases (26). The SAH produced during Cr production can be reversibly hydrolyzed to homocysteine and adenosine. Homocysteine is further decomposed into cysteine, which undergo the methylation reaction with its own methyl donors such as vitamin B. The methionine was formed by methylation, and then participates in the synthesis of Cr again (19).
Figure 2 describes the synthesis and metabolism of GAA in detail.
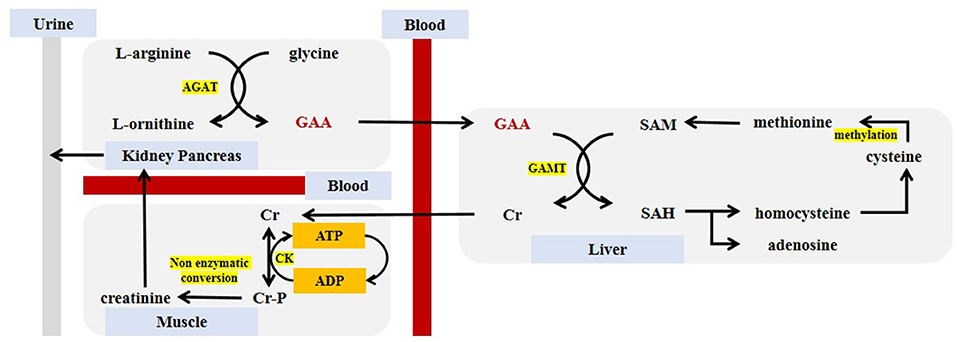
Figure 2. Guanidinoacetic acid synthesis and metabolism. GAA, guanidinoacetic acid; Cr, creatine; Cr-P, phosphocreatine; SAM, S-adenosyl-methionine; SAH, S-adenosyl-homocysteine; AGAT, L-arginine:glycine amidinotransferase; GAMT, guanidinoacetate N-methyltransferase; CK, creatine kinase; ATP, adenosine triphosphate; ADP, adenosine diphosphate.
The Regulation of Guanidinoacetic Acid on Skeletal Muscle Development
Skeletal Muscle Classification and Structure
Taking the difference in biological function and structural composition as the classification basis, muscle tissue can be divided into two types: striated muscle and smooth muscle (27). Smooth muscle is mainly distributed in blood vessel walls, respiratory tract, digestive tract and other internal organs. It is an uncontrollable type of muscle regulated by autonomic nerves (28). The striated muscle is composed of skeletal muscle and myocardium. It is named because of the light and dark stripes that can be observed under the microscope, and the striated muscle are dually innervated by consciousness and the nervous system (29).
Skeletal muscles are also divided into fast muscles and slow muscles, and this classification is not limited to vertebrates. These two types of skeletal muscles are also found in invertebrates such as octopuses and crabs (30). Myofibers are cell units that make up muscle tissues and gather into hundles, while multiple muscle bundles gather to form muscles. In addition, the composition of myofibrils includes thin actin filaments and thick myosin filaments which are composed of specific proteins (responsible for muscle contraction) and arranged regularly in the sarcoplasm (3). Based on the metabolic capacity and contractility of myofibers, it can be summarized as aerobic metabolism myofibers (type I myofibers) and glycolytic metabolism myofibers (type II myofibers, including IIa, IIb, IIx). Type I myofibers are rich in myoglobin and cytochromes showing a brighter red color but have a slow contraction speed, while type II myofibers contract fast and appear white, among all types of type II myofibers, the type IIb has the fastest contraction speed (31).
Skeletal Muscle Development
A large number of studies have shown that the development of skeletal muscle includes the following four processes: the differentiation of amniotic mesoderm stem cells (32) to generate myoblasts, the differentiation and fusion of myoblasts to generate myotubes, the formation of myofibers, and the maturation of myofibers (33). The number of myofibers in humans and animals has been determined before birth, but the expansion of their volume and the transformation of the types of myofiber depends on acquired comprehensive factors (34). In the process of skeletal muscle formation, the primary and secondary myotubes occur at different developmental stages (35). Primary myotubes are formed during the embryonic period and the number is closely related to genetic factors. The secondary myotubes begin to develop in the fetal period, and the nutritional regulation during maternal pregnancy can significantly affect the development of muscle fibers (36–38). In addition, since the secondary myotube grows around the primary myotube, the larger the diameter of the primary myotube, the more secondary myotubes can grow around it (39). Some research have shown that the development of primary myotubes eventually generated slow-twitch fibers, but the secondary myotubes generated fast-twitch fibers (40).
After the fetus is born, the myofibers gradually mature. At this time, muscle growth only depends on the changes in the volume of muscle fiber and the transformation between different types of muscle fiber (41). The reason for the increase in muscle fiber volume is related to the proliferation and differentiation of muscle satellite cells, which are muscle-derived stem cells that are normally in a resting state but have differentiation potential (42). The reason for the increase in muscle fiber volume is related to the proliferation and differentiation of muscle satellite cells, which are muscle-derived stem cells that are normally in a resting state but have differentiation potential (42). The muscle satellite cells exist widely between the muscle cell membrane and the matrix membrane, and have the biological function of promoting myomuscle regeneration after being activated (43, 44). Moreover, myofiber are a type of multinucleated cells (nuclei can reach several hundred), and each nucleus only controls certain part of the cytoplasm, referred to as myonucleus area (each cell nucleus and controlled cytoplasm are called a DNA unit). Satellite cells also have the ability to increase the number of myofiber nuclei to maintain the balance of the ratio between nucleus and cytoplasm (45).
Guanidinoacetic Acid Regulates Genes Involved in Skeletal Muscle Development
In eukaryotes, differences in gene expression are the root cause of differences between individuals, gene expression is controlled at different stages of individual development and is affected by many factors (46). There are many genes that regulate the development of skeletal muscle, among which the myogenic regulatory factors (MRFs) plays an important role in the growth of skeletal muscle. It mainly includes four specific transcription factors, myogenic determining factor (MyoD), myogenic factor-5 (Myf5), myogenin (Myog), and muscle regulatory factor-4 (MRF4 or Myf6). The expression of MyoD and Myf5 contributed to the directed differentiation of myogenic cells, while Myog and MRF4 performed their functions in the differentiation process of myoblasts. It shows that different genes are expressed in the sequence of time during muscle development (47, 48). There are few molecular studies of GAA on muscle growth. We can explain the effect of GAA on muscle growth from its metabolite Cr.
CR supplementation can improve the expression of MRFs, especially in young individuals, but this effect gradually decreases with the increase of age (49). After treating C2C12 myoblasts with Cr, it was found that although the degree of influences on the expression of MyoD, Myf5, Myog, and MRF4 genes were different, they were all positive (50). Moreover, some studies have shown that Myf5, MyoD, or MRF4 inactivation can produce viable mice, but the absence of Myog causes the mice to die after birth (51).
Pax gene family is involved in all stages of muscle cell growth and differentiation, mainly including Pax3 and Pax7 (52). Pax gene family is involved in various stages of muscle cell growth and differentiation, including Pax3 and Pax7. The main functions of Pax include regulating the behavior of myogenic progenitor cells and the formation of skeletal muscle. Pax3 plays a dominant role in the above processes. The lack of Pax3 has caused the early embryonic development to be restricted, and impaired muscle regeneration in the later period (53, 54). And Pax3 can directly regulate the expression of MyoD and Myf5, and indirectly act in the differentiation process of myogenic cells (55, 56). In contrast, Pax7-missing led to slower muscle development, reduction of the amount of muscle tissue, whereas there is no pathological change in the structure (57).
Myostatin (MSTN) is an important member of transforming growth factor superfamily, also known as growth differentiation factor-8 (GDF-8), which is mainly manifested in the negative regulation of muscle growth and strength increase (58). MSTN can activate receptor function by binding to ALK4/5 and ActR2A/B type receptors on the surface of muscle cells, and lead to the function of promoting protein degradation and inhibiting protein synthesis in muscle (59). Specifically, the mature MSTN fragment first binds to type II receptor (mainly ActRIIB) and starts the signal cascade in muscle cells, which makes ActRIIB autophosphorylate and bind to type I receptor (ALK4 and ALK5) with low affinity to enhance the transcription process of target gene (60). In addition, MSTN can also inhibit the expression of protein kinase B (AKT) and the transcriptional activity of MyoD, and may lead to muscle atrophy and other diseases (61). However, the missing of MSTN or gene homozygous mutation result in abnormal accumulation of muscle mass and proliferation of myofibers (62). In the research field of skeletal muscle growth and development, MSTN is recognized as a negative regulator with important physiological functions (63). GAA and its metabolite Cr have the effect of down-regulating the expression of MSTN and eliminating its inhibitory effect on muscle growth (64).
Myocyte enhancer factor 2 (MEF2) gene family is another gene family in the body that can directly regulate skeletal muscle development in addition to the MRFs gene family. It is composed of four genes (65), MEF2a, MEF2b, MEF2c, and MEF2d, and is highly expressed in myoblasts. It functions mainly by recognizing a conserved A/T-rich elements in genes (66). MEF2c and Myog co-stimulate MyoD expression to activate the differentiation process of myoblasts (67, 68). Exogenous Cr supplementation helps the expression of MEF2 to improve muscle growth (69).
In addition, some scholars have found that peroxisome proliferator-activated receptor gamma coactivator 1α (PGC-1α) can increase the accumulation of skeletal muscle during exercise and regulate the transcription of some target genes (70).
MicroRNA (miRNA) is a very conservative non-protein coding RNA that can directly degrade target gene mRNA or inhibit its translation. It plays a key role as a post-transcriptional regulator in myogenesis (71). GAA induced the activation of AKT/mTOR/S6K signaling pathway through miR-133a-3p and miR-1a-3p to promote myoblast differentiation (72).
Figure 3 describes the pathways regulated by GAA/Cr during their skeletal muscle development and growth.
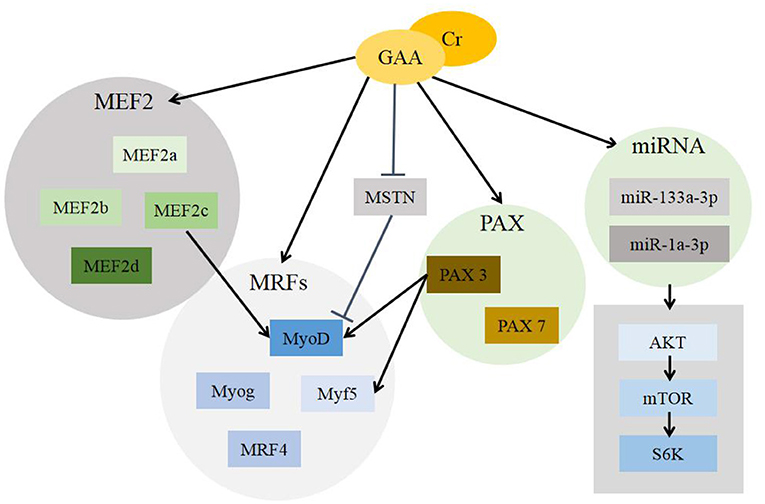
Figure 3. Mechanisms of GAA/Cr promoting skeletal muscle development and growth. GAA, guanidinoacetic acid; Cr, creatine; MEF2, myocyte enhancer factor 2 (includes MEF2a, MEF2b, MEF2c and MEF2d); MRFs, myogenic regulatory factors (includes MyoD, Myog, Myf5, MRF4); MSTN, Myostatin; PAX, Pax gene family (includes Pax3 and Pax7); miRNA, MicroRNA (includes miR-133a-3p and miR-1a-3p); AKT, protein kinase B; mTOR, mammalian target of rapamycin; S6K, S6 kinase.
Application of Guanidinoacetic Acid in Animal Models
Since researchers recognized the importance of Cr for muscle development, many studies have been carried out to evaluate the effects of different amino acids and other substances on endogenous Cr metabolism. Researches added 1 g of GAA to the diet of the young rats in 1930s, and they found that the Cr level was increased by nearly 50% which reached the peak after 17–24 h. This effect was even higher than adding the equivalent amount of Cr (73). Vivo experiments proved that when the mouse body lacks Cr, GAA can provide energy for the body under the catalysis of CK. It also shows that CK can use GAA and go through the phosphorylation pathway to fight against energy damage (24, 74). And the main reason why Cr contributes to the synthesis of muscle tissue protein, improves muscle energy reserves and muscle strength is that it's synthesized with the precursor substance GAA. As a supplement to the aforementioned regulation of genes related to skeletal muscle development, GAA and Cr also functions by promoting the secretion of insulin-like growth factor-1 (IGF-1) and growth hormone (GH) in the body (both IGF-1 and GH are anabolic hormones that can increase muscle growth) (64). Vitro experiments have shown that GAA can promote the expression of MyoD and MyoG mRNA and increase the fusion rate of myotubes in C2C12 myoblasts. It can also affect the level of total myosin heavy chain (MyHC) protein to increase myotube thickness and gastrocnemius cross-section area (72). Another in vitro experiment demonstrated that Cr can activate the differentiation process of C2C12 myoblasts by activating the P38 and Akt/PKB cell pathways, which is manifested by the expression of myosin heavy chain type II, the increase in the number of nuclei in the myotube, and promote the occurrence of the cell fusion process (75).
Returning to the perspective of animal husbandry and nutrition. With the development of the global economy and the continuous improvement of consumption levels, human being to pay more and more attention to the nutritional balance of daily diet (76). Due to the demand for high-quality protein, the demand for high-quality meat is also increasing. Therefore, enhancing the amount and speed of muscle accumulation in livestock and poultry is the most crucial section in the development of animal husbandry (77).
In addition to affecting the growth of muscles, the content of Cr in the animal body can also maintain the steady state of ATP and buffer the accumulation of lactic acid in the muscles (78), and improve meat quality. The methods for animals to obtain Cr can be categorized as endogenous and exogenous. The endogenous method mainly generates GAA through arginine and glycine, and then synthesizes Cr with methionine in the liver, but this does not meet the animals' needs (79). Exogenous Cr sources mainly include animal protein raw materials (meat and bone meal) and fish protein raw materials (fish meal) (80), while plant raw materials lack Cr or its precursor. Combined with the analysis of animal feed composition and nutritional level, it can be found that animals cannot get enough Cr. So they can only synthesize Cr at the cost of consuming endogenous amino acids, resulting in the loss of amino acids (81). Especially taking the application of methionine in poultry production as an example, methionine is the first limiting amino acid of poultry and is very beneficial to the growth of muscles and feathers, and the consumption of methionine in the process of Cr synthesis has resulted in increased demand for methionine in poultry (82). Moreover, for young animals, arginine is an essential amino acid with growth promoting effect. Lack of arginine may easily cause the slow growth of chicks, while adding GAA to arginine-deficient diets can significantly improve the growth performance of chicks. It is suggested that GAA can be a good substitute for dietary arginine (83).
Using Cr as feed additive directly has problems of poor stability, high cost and low animal bioavailability. The use of GAA will solve these problems (84). In addition, animals have a very high utilization efficiency of exogenous GAA. After experiments with colon-fistulated broilers, it was found that the digestibility-rate of GAA reached 99% (85). GAA and its metabolite Cr not only improve animal growth performance and promote muscle growth, but also affect meat quality (10). It shows that the drip loss of meat is reduced, the yellowness is increased, and the activity of the free radical metabolism related enzymes and the antioxidant enzymes (reducing lipid peroxidation) are improved (86).
Table 1 summarizes the results of using GAA or Cr in livestock production.
Apart from synthesizing Cr to promote protein deposition and muscle growth in the body, the apply of GAA also has the effect of promoting insulin secretion to control blood glucose (98). Insulin is the only hormone in the body that plays a role in lowering blood glucose, and it is also the only hormone that contributes to glycogen, protein and fat synthesis. It links the regulatory effect of GAA intake on insulin with muscle growth (99, 100). The safety of GAA as a dietary supplement was evaluated in the initial human studies, and subsequent clinical trials have also proved that GAA is a non-toxic and highly tolerable substance. Dietary GAA supplementation in humans leads to an increase in serum creatinine levels without impairment of renal function (15). Some studies also shown that a small number of samples that take GAA will have an enhancement of serum homocysteine. This might be a potential adverse effect, since hyperhomocysteinemia is considered as an independent risk factor for cardiovascular and atherosclerotic diseases (101). Exogenous intake of GAA mainly exhibits antioxidant effects at low doses, while pro-oxidative effects and even oxidative stress appear at high doses (102). GAA is a kind of pro-oxidant (103), but its metabolites such as Cr and arginine all express anti-oxidant effects, so GAA is also regarded as an indirect antioxidant (104). However, the relationship between the level of GAA intake and the pro-oxidant-antioxidant properties needs to be further elucidated.
Perspectives
A large number of animal experiments and human clinical applications have proved that GAA has good effects in improving muscle development and growth. Especially in animal husbandry, it has been widely used, and has the advantages of low cost relative to Cr and arginine, and better growth promoting effect. However, the research on GAA are not very in-depth, directions such as its mechanism of promoting muscle development, the determination of the effective dosage in animal production, and whether GAA has toxic effects or not have not been fully studied. Therefore, more detailed research work are needed.
Author Contributions
ZhaomY, ZhaoyY, and SL are the primary investigator in this study. YY and TY are participated in literature collection and summary. ZhaoyY and QC revised the manuscript. QC designed this study and wrote the manuscript. All authors have read and approved the final manuscript.
Funding
This study was supported by Hunan Province double first-class construction project(kxk201801004).
Conflict of Interest
The authors declare that the research was conducted in the absence of any commercial or financial relationships that could be construed as a potential conflict of interest.
Publisher's Note
All claims expressed in this article are solely those of the authors and do not necessarily represent those of their affiliated organizations, or those of the publisher, the editors and the reviewers. Any product that may be evaluated in this article, or claim that may be made by its manufacturer, is not guaranteed or endorsed by the publisher.
References
1. Frontera WR, Ochala J. Skeletal muscle: a brief review of structure and function. Calcif Tissue Int. (2015) 96:183–95. doi: 10.1007/s00223-014-9915-y
2. Schnyder S, Handschin C. Skeletal muscle as an endocrine organ: PGC-1α, myokines and exercise. Bone. (2015) 80:115–25. doi: 10.1016/j.bone.2015.02.008
3. Grefte S, Kuijpers-Jagtman AM, Torensma R, VondenHoff JW. Skeletal muscle development and regeneration. Stem Cells Dev. (2007) 16:857–68. doi: 10.1089/scd.2007.0058
4. Bongiovanni T, Genovesi F, Nemmer M, Carling C, Alberti G, Howatson G. Nutritional interventions for reducing the signs and symptoms of exercise-induced muscle damage and accelerate recovery in athletes: current knowledge, practical application and future perspectives. Eur J Appl Physiol. (2020) 120:1965–96. doi: 10.1007/s00421-020-04432-3
5. Ostojic SM, Premusz V, Nagy D, Acs P. Guanidinoacetic acid as a novel food for skeletal muscle health. J Funct Foods. (2020) 73:104129. doi: 10.1016/j.jff.2020.104129
6. Padilha CS, Cella PS, Salles LR, Deminice R. Oral creatine supplementation attenuates muscle loss caused by limb immobilization: a systematic review. Fisioterapia em Movimento. (2017) 30:831–8. doi: 10.1590/1980-5918.030.004.ar01
7. Leroy F, De Smet S. Meat in the Human Diet: A Biosocial Perspective. More than Beef, Pork and Chicken-The Production, Processing, and Quality Traits of Other Sources of Meat for Human Diet. Cham: Springer (2019). p. 1–19.
8. Yang C, Jiang X, Du H, Li Q, Zhang Z, Qiu M, et al. A review: achievements and new obstacles in china's food security revealed by grain and animal meat production. IOP Conf Series Earth Environ Sci. (2021) 705:012025. doi: 10.1088/1755-1315/705/1/012025
9. Zhang L, Li JL, Wang XF, Zhu XD, Gao F, Zhou GH. Attenuating effects of guanidinoacetic acid on preslaughter transport-induced muscle energy expenditure and rapid glycolysis of broilers. Poult Sci. (2019) 98:3223–32. doi: 10.3382/ps/pez052
10. Michiels J, Maertens L, Buyse J, Lemme A, Rademacher M, Dierick NA, et al. Supplementation of guanidinoacetic acid to broiler diets: effects on performance, carcass characteristics, meat quality, and energy metabolism. Poult Sci. (2012) 91:402–12. doi: 10.3382/ps.2011-01585
11. Bloch K, Schoenheimer R. The biological origin of the amidine group in creatine. J Biol Chem. (1940) 134:785–6. doi: 10.1016/S0021-9258(18)73239-0
12. Chen H, Zhang YHPJ. Enzymatic regeneration and conservation of ATP: challenges and opportunities. Crit Rev Biotechnol. (2021) 41:16–33. doi: 10.1080/07388551.2020.1826403
13. Ben-Sahra I, Puissant A. HER2 signaling hijacks the creatine shuttle to fuel breast cancer cell growth. Cell Metab. (2018) 28:805–7. doi: 10.1016/j.cmet.2018.11.009
14. Borsook ME, Borsook H. Treatment of cardiac decompensation with betaine and glycocyamine. Ann West Med Surg. (1951) 5:830–55.
15. Ostojic SM. Guanidinoacetic acid as a performance-enhancing agent. Amino Acids. (2016) 48:1867–75. doi: 10.1007/s00726-015-2106-y
16. Smith RN, Agharkar AS, Gonzales EB. A review of creatine supplementation in age-related diseases: more than a supplement for athletes. F1000Research. (2014) 3:222. doi: 10.12688/f1000research.5218.1
17. Davani-Davari D, Karimzadeh I, Ezzatzadegan-Jahromi S, Sagheb MM. Potential adverse effects of Creatine supplement on the kidney in athletes and bodybuilders. Iran J Kidney Dis. (2018) 12:253–60.
18. Mert K U, Ilgüy S, Dural M, Mert O, Ozakin E. Effects of creatine supplementation on cardiac autonomic functions in bodybuilders. Pacing Clin Electrophysiol. (2017) 40:721–7. doi: 10.1111/pace.13096
19. Edison EE, Brosnan ME, Meyer C, Brosnan JT. Creatine synthesis: production of guanidinoacetate by the rat and human kidney in vivo. Am J Physiol Renal Physiol. (2007) 293:F1799–804. doi: 10.1152/ajprenal.00356.2007
20. Ostojic SM. Human gut microbiota as a source of guanidinoacetic acid. Med Hypotheses. (2020) 142:109745. doi: 10.1016/j.mehy.2020.109745
21. Ostojic SM, Ratgeber L, Olah A, Betlehem J, Acs P. Guanidinoacetic acid deficiency: a new entity in clinical medicine?. Int J Med Sci. (2020) 17:2544. doi: 10.7150/ijms.47757
22. Nabuurs CI, Choe CU, Veltien A, Kan HE, van Loon LJC, Rodenburg RJT, et al. Disturbed energy metabolism and muscular dystrophy caused by pure creatine deficiency are reversible by creatine intake. J Physiol. (2013) 591:571–92. doi: 10.1113/jphysiol.2012.241760
23. Shi K, Zhao H, Xu S, Han H, Li W. Treatment efficacy of high-dose creatine supplementation in a child with creatine transporter (SLC6A8) deficiency. Mol Genet Genomic Med. (2021) 9:e1640. doi: 10.1002/mgg3.1640
24. Balestrino M, Adriano E. Presence of guanidinoacetate may compensate creatine absence and account for less statin-induced muscle damage in gamt-deficient compared to agat-deficient mice. Amino Acids. (2020) 52:667–9. doi: 10.1007/s00726-020-02838-z
25. Marie, Joncquel-Chevalier, Curt, Pia-Manuela, Voicu, Monique, et al. Creatine biosynthesis and transport in health and disease. Biochimie. (2015) 119:146–65. doi: 10.1016/j.biochi.2015.10.022
26. Post A, Tsikas D, Bakker S. Creatine is a conditionally essential nutrient in chronic kidney disease: a hypothesis and narrative literature review. Nutrients. (2019) 11:1044. doi: 10.3390/nu11051044
27. Steinmetz PRH, Kraus JEM, Larroux C, Hammel JU, Amon-Hassenzahl A, Houliston E, et al. Independent evolution of striated muscles in cnidarians and bilaterians. Nature. (2012) 487:231–4. doi: 10.1038/nature11180
28. Sanders KM, Ward SM, Koh SD. Interstitial cells: regulators of smooth muscle function. Physiol Rev. (2014) 94:859–907. doi: 10.1152/physrev.00037.2013
29. Shadrin IY, Khodabukus A, Bursac N. Striated muscle function, regeneration, and repair. Cell Mol Life Sci. (2016) 73:4175–202. doi: 10.1007/s00018-016-2285-z
30. Ochiai Y, Ozawa H. Biochemical and physicochemical characteristics of the major muscle proteins from fish and shellfish. Fisheries Sci. (2020) 86:729–40. doi: 10.1007/s12562-020-01444-y
31. He J, Watkins S, Kelley DE. Skeletal muscle lipid content and oxidative enzyme activity in relation to muscle fiber type in type 2 diabetes and obesity. Diabetes. (2001) 50:817–23. doi: 10.2337/diabetes.50.4.817
32. Bryson-Richardson RJ, Currie PD. The genetics of vertebrate myogenesis. Nat Rev Genet. (2008) 9:632–46. doi: 10.1038/nrg2369
33. Song T, Sadayappan S. Featured characteristics and pivotal roles of satellite cells in skeletal muscle regeneration. J Muscle Res Cell Motil. (2019) 41:341–53. doi: 10.1007/s10974-019-09553-7
34. Wigmore PM, Stickland NC. Muscle development in large and small pig fetuses. J Anat. (1983) 137:235–45.
35. Duxson MJ, Usson Y, Harris AJ. The origin of secondary myotubes in mammalian skeletal muscles: ultrastructural studies. Development. (1989) 107:743–50. doi: 10.1242/dev.107.4.743
36. Zhang M, Mclennan IS. During secondary myotube formation, primary myotubes preferentially absorb new nuclei at their ends. Dev Dynam. (2010) 204:168–77. doi: 10.1002/aja.1002040207
37. Maley M, Davies MJ, Grounds MD. Extracellular matrix, growth factors, genetics: their influence on cell proliferation and myotube formation in primary cultures of adult mouse skeletal muscle. Exp Cell Res. (1995) 219:169–79. doi: 10.1006/excr.1995.1217
38. Fiorotto ML, Davis TA. Critical windows for the programming effects of early-life nutrition on skeletal muscle mass. Nestle Nutr Inst Workshop Ser. (2018) 89:25–35. doi: 10.1159/000486490
39. Duxson MJ, Sheard PW. Formation of new myotubes occurs exclusively at the multiple innervation zones of an embryonic large muscle. Dev Dynam. (1995) 204:391–405. doi: 10.1002/aja.1002040406
40. Gros J, Scaal M, Marcelle C. A two-step mechanism for myotome formation in chick. Dev Cell. (2004) 6:875–82. doi: 10.1016/j.devcel.2004.05.006
41. Ren H, Li L, Su H, Xu L, Du L. Histological and transcriptome-wide level characteristics of fetal myofiber hyperplasia during the second half of gestation in Texel and Ujumqin sheep. BMC Genomics. (2011) 12:1–18. doi: 10.1186/1471-2164-12-411
42. Montecino F, González N, Blanco N, Ramírez MJ, Olguín H. c-Abl kinase is required for satellite cell function through Pax7 regulation. Front Cell Dev Biol. (2021) 9:606403. doi: 10.3389/fcell.2021.606403
43. Sambasivan R, Yao R, Kissenpfennig A, Van Wittenberghe L, Paldi A, Gayraud-Morel B, et al. Pax7-expressing satellite cells are indispensable for adult skeletal muscle regeneration. Development. (2011) 138:3647–56. doi: 10.1242/dev.067587
44. Lepper C, Partridge TA, Fan CM. An absolute requirement for Pax7-positive satellite cells in acute injury-induced skeletal muscle regeneration. Development. (2011) 138:3639–46. doi: 10.1242/dev.067595
45. Cramer AAW, Prasad V, Eftestøl E, Song T, Hansson KA, Dugdale HF, et al. Nuclear numbers in syncytial muscle fibers promote size but limit the development of larger myonuclear domains. Nat Commun. (2020) 11:1–14. doi: 10.1038/s41467-020-20058-7
46. Cipriano A, Ballarino M. The ever-evolving concept of the gene: the use of RNA/protein experimental techniques to understand genome functions. Front Mol Biosci. (2018) 5:20. doi: 10.3389/fmolb.2018.00020
47. Kim K, Kim D, Min Y, Jeong D, Son YO, Do K. Myogenic regulatory factors are key players in determining muscle mass and meat quality in Jeju native and Berkshire pigs. Vet Med Sci. (2021) 7:735–45. doi: 10.1002/vms3.418
48. Zammit PS. Function of the myogenic regulatory factors Myf5, MyoD, Myogenin and MRF4 in skeletal muscle, satellite cells and regenerative myogenesis. Semin Cell Dev Biol. (2017) 72:19–32. doi: 10.1016/j.semcdb.2017.11.011
49. Candow DG, Forbes SC, Chilibeck PD, Cornish SM, Antonio J, Kreider RB. Variables influencing the effectiveness of creatine supplementation as a therapeutic intervention for sarcopenia. Front Nutr. (2019) 6:124. doi: 10.3389/fnut.2019.00124
50. Louis M, Van Beneden R, Dehoux M, Thissen JP, Francaux M. Creatine increases IGF-I and myogenic regulatory factor mRNA in C2C12 cells. FEBS Lett. (2004) 557:243–7. doi: 10.1016/S0014-5793(03)01504-7
51. Knapp JR, Davie JK, Myer A, Meadows E, Olson EN, Klein WH. Loss of myogenin in postnatal life leads to normal skeletal muscle but reduced body size. Development. (2006) 133:601–10. doi: 10.1242/dev.02249
52. Thompson B, Davidson EA, Liu W, Nebert DW, Bruford EA, Zhao H, et al. Overview of PAX gene family: analysis of human tissue-specific variant expression and involvement in human disease. Hum Genet. (2020) 140:381–400. doi: 10.1007/s00439-020-02212-9
53. Kim HK, Ankamreddy H, Lee DJ, Kong KA, Ko HW, Kim MH, et al. Pax3 function is required specifically for inner ear structures with melanogenic fates. Biochem Biophys Res Commun. (2014) 445:608–14. doi: 10.1016/j.bbrc.2014.02.047
54. Manandhar D. Methods for Comparative Analysis of Chromatin Accessibility and Gene Expression, with Applications to Cellular Reprogramming. Durham, NC: Duke University (2018).
55. Hernández-Hernández JM, García-González EG, Brun CE, Rudnicki MA. The myogenic regulatory factors, determinants of muscle development, cell identity and regeneration. Semin Cell Dev Biol. (2017) 72:10–8. doi: 10.1016/j.semcdb.2017.11.010
56. Daubas P, Buckingham ME. Direct molecular regulation of the myogenic determination gene Myf5 by Pax3, with modulation by Six1/4 factors, is exemplified by the-111 kb-Myf5 enhancer. Dev Biol. (2013) 76:236–44. doi: 10.1016/j.ydbio.2013.01.028
57. Florkowska A, Meszka I, Zawada M, Legutko D, Proszynski TJ, Janczyk-llach K, et al. Pax7 as molecular switch regulating early and advanced stages of myogenic mouse ESC differentiation in teratomas. Stem Cell Res Ther. (2020) 11:1–18. doi: 10.1186/s13287-020-01742-3
58. Lee SJ. Regulation of muscle mass by myostatin. Annu Rev Cell Dev Biol. (2004) 20:61–86. doi: 10.1146/annurev.cellbio.20.012103.135836
59. Muramatsu H, Kuramochi T, Katada H, Ueyama A, Ruike Y, Ohmine K, et al. Novel myostatin-specific antibody enhances muscle strength in muscle disease models. Sci Rep. (2021) 11:1–16. doi: 10.1038/s41598-021-81669-8
60. Aiello D, Patel K, Lasagna E. The myostatin gene: an overview of mechanisms of action and its relevance to livestock animals. Anim Genet. (2018) 49:505–19. doi: 10.1111/age.12696
61. Lee SJ, Reed LA, Davies MV, Girgenrath S, Goad ME, Tomkinson KN, et al. Regulation of muscle growth by multiple ligands signaling through activin type II receptors. Proc Nat Acad Sci. (2005) 102:18117–22. doi: 10.1073/pnas.0505996102
62. Sharma M, Langley B, Bass J, Kambadur R. Myostatin in muscle growth and repair. Exerc Sport Sci Rev. (2001) 29:155–8. doi: 10.1097/00003677-200110000-00004
63. Ahad WA, Andrabi M, Beigh SA, Bhat RA, Shah RA. Applications of myostatin (MSTN) gene in the livestock animals and humans: a review. Int J Curr Microbiol Appl Sci. (2017) 6:1807–11. doi: 10.20546/ijcmas.2017.609.222
64. Farshidfar FA, Pinder MB, Myrie S. Creatine supplementation and skeletal muscle metabolism for building muscle mass-review of the potential mechanisms of action. Curr Protein Peptide Sci. (2017) 18:1273–87. doi: 10.2174/1389203718666170606105108
65. Chen Z, Wang Q, Zhang H, Ma X, Wu W, Cheng N, et al. Purification, crystallization, and X-ray diffraction analysis of myocyte enhancer factor 2D and DNA complex. Protein Expr Purif. (2021) 179:105788. doi: 10.1016/j.pep.2020.105788
66. Shi Y, Mao X, Cai M, Hu S, Lai X, Chen S, et al. miR-194-5p negatively regulates the proliferation and differentiation of rabbit skeletal muscle satellite cells. Mol Cell Biochem. (2021) 476:425–33. doi: 10.1007/s11010-020-03918-0
67. Mohammadabadi M, Bordbar F, Jensen J, Du M, Guo W. Key genes regulating skeletal muscle development and growth in farm animals. Animals. (2021) 11:835. doi: 10.3390/ani11030835
68. Arnold HH, Winter B. Muscle differentiation: more complexity to the network of myogenic regulators. Curr Opin Genet Dev. (1998) 8:539–44. doi: 10.1016/S0959-437X(98)80008-7
69. Ju JS, Smith JL, Oppelt PJ, Fisher JS. Creatine feeding increases GLUT4 expression in rat skeletal muscle. Am J Physiol Endocrinol Metabol. (2005) 288:E347–52. doi: 10.1152/ajpendo.00238.2004
70. Sugimoto T, Uchitomi R, Hatazawa Y, Miura S, Kamei Y. Metabolomic analysis on blood of transgenic mice overexpressing PGC-1α in skeletal muscle. Biosci Biotechnol Biochem. (2021) 85:579–86. doi: 10.1093/bbb/zbaa059
71. Mok GF, Lozano-Velasco E, Münsterberg A. microRNAs in skeletal muscle development. Semin Cell Dev Biol. (2017) 72:67–76. doi: 10.1016/j.semcdb.2017.10.032
72. Wang Y, Ma J, Qiu W, Zhang J, Feng S, Zhou X, et al. Guanidinoacetic acid regulates myogenic differentiation and muscle growth through miR-133a-3p and miR-1a-3p co-mediated Akt/mTOR/S6K signaling pathway. Int J Mol Sci. (2018) 19:2837. doi: 10.3390/ijms19092837
73. Beard HH, Barnes BO. The influence of feeding proteins, amino acids, and related substances upon creatine-creatinine metabolism. J Biol Chem. (1931) 94:49–69.
74. Lygate CA, Aksentijevic D, Dawson D, Ten Hove M, Phillips D, de Bono JP, et al. Living without creatine: unchanged exercise capacity and response to chronic myocardial infarction in creatine-deficient mice. Circ Res. (2013) 112:945–55. doi: 10.1161/CIRCRESAHA.112.300725
75. Deldicque L, Theisen D, Bertrand L, Hespel P, Hue L, Francaux M. Creatine enhances differentiation of myogenic C2C12 cells by activating both p38 and Akt/PKB pathways. Am J Physiol Cell Physiol. (2007) 293:C1263–71. doi: 10.1152/ajpcell.00162.2007
76. Chang X, DeFries RS, Liu L, Davis K. Understanding dietary and staple food transitions in China from multiple scales. PLoS ONE. (2018) 13:e0195775. doi: 10.1371/journal.pone.0195775
77. Minocha S, Makkar S, Swaminathan S, Thomas T, Webb P, Kurpad AV. Supply and demand of high quality protein foods in India: trends and opportunities. Global Food Security. (2019) 23:139–48. doi: 10.1016/j.gfs.2019.05.004
78. Lee S, Hong G, Park W, Lee J, Kim N, Park H, et al. The effect of short-term creatine intake on blood lactic acid and muscle fatigue measured by accelerometer-based tremor response to acute resistance exercise. Phys Act Nutr. (2020) 24:29–36. doi: 10.20463/pan.2020.0006
79. Negro M, Avanzato I, D'Antona G. Creatine in skeletal muscle physiology. Nonvitamin Nonmin Nutritional Suppl. (2019) 2019:59–68. doi: 10.1016/B978-0-12-812491-8.00008-4
80. Shomrat A, Weinstein Y, Katz A. Effect of creatine feeding on maximal exercise performance in vegetarians. Eur J Appl Physiol. (2000) 82:321–5. doi: 10.1007/s004210000222
81. Fryer C, Rademacher M. Using a creatine source to improve broiler performance: advertorial. AFMA Matrix. (2013) 22:29–31.
82. McBreairty LE, Robinson JL, Furlong KR, Brunton JA, Bertolo RF, et al. Guanidinoacetate is more effective than creatine at enhancing tissue creatine stores while consequently limiting methionine availability in Yucatan miniature pigs. PLoS ONE. (2015) 10:e0131563. doi: 10.1371/journal.pone.0131563
83. Dilger RN, Bryant-Angeloni K, Payne RL, Lemme A, Parsons CM. Dietary guanidino acetic acid is an efficacious replacement for arginine for young chicks. Poult Sci. (2013) 92:171–7. doi: 10.3382/ps.2012-02425
84. Baker DH. Advances in protein-amino acid nutrition of poultry. Amino Acids. (2009) 37:29–41. doi: 10.1007/s00726-008-0198-3
85. Lemme A, Tossenberger J, Ringel J. Digestibility and availability of the creatine source guanidino acetic acid in broilers. J Dairy Sci. (2007) 90:153.
86. Wang LS, Shi BM, Shan AS, Zhang YY. Effects of guanidinoacetic acid on growth performance, meat quality and antioxidation in growing-finishing pigs. J Anim Vet Adv. (2012) 11:631–6. doi: 10.3923/javaa.2012.631.636
87. Lu Y, Zou T, Wang Z, Yang J, Li L, Guo X, et al. Dietary guanidinoacetic acid improves the growth performance and skeletal muscle development of finishing pigs through changing myogenic gene expression and myofibre characteristics. J Anim Physiol Anim Nutr. (2020) 104:1875–83. doi: 10.1111/jpn.13351
88. He DT, Gai XR, Yang LB, Li JT, Lai WQ, Sun X, et al. Effects of guanidinoacetic acid on growth performance, creatine and energy metabolism, and carcass characteristics in growing-finishing pigs. J Anim Sci. (2018) 96:3264–73. doi: 10.1093/jas/sky186
89. Li J, Zhang L, Fu Y, Li Y, Jiang Y, Zhou G, et al. Creatine monohydrate and guanidinoacetic acid supplementation affects the growth performance, meat quality, and creatine metabolism of finishing pigs. J Agric Food Chem. (2018) 66:9952–9. doi: 10.1021/acs.jafc.8b02534
90. Liu Y, Li JL, Li YJ, Gao T, Zhang L, Gao F, et al. Effects of dietary supplementation of guanidinoacetic acid and combination of guanidinoacetic acid and betaine on postmortem glycolysis and meat quality of finishing pigs. Anim Feed Sci Technol. (2015) 205:82–9. doi: 10.1016/j.anifeedsci.2015.03.010
91. Bahelka I, Bučko O, Stupka R, Šprysl M, Cítek J. Effects of creatine monohydrate diet on muscle metabolism, quality, sensory and oxidative stability of pork in female, entire and castrated male pigs. J Agric Sci TechnolA. (2020) 10:78–85. doi: 10.17265/2161-6256/2020.02.004
92. Zhao W, Li J, Xing T, Zhang L, Gao F. Effects of guanidinoacetic acid and complex antioxidant supplementation on growth performance, meat quality and antioxidant function of broiler chickens. J Sci Food Agric. (2020) 97:890–900. doi: 10.1002/JSFA.11036
93. Zarghi H, Golian A, Tabatabaei Yazdi F. Effect of dietary sulphur amino acid levels and guanidinoacetic acid supplementation on performance, carcase yield and energetic molecular metabolites in broiler chickens fed wheat-soy diets. Ital J Anim Sci. (2020) 19:951–9. doi: 10.1080/1828051X.2020.1809537
94. Esser AFG, Taniguti TL, da Silva AM, Vanroo E, Kaneko IN., dos Santos TC, et al. Effect of supplementation of guanidinoacetic acid and arginine in vegetable diets for broiler on performance, carcass yield and meat quality. Semina Ciências Agrárias. (2018) 39:1307–18. doi: 10.5433/1679-0359.2018v39n3p1307
95. Zhao MM, Gao T, Zhang L, Li JL, Lv PA, Yu LL, et al. Effects of in ovo feeding of creatine pyruvate on the hatchability, growth performance and energy status in embryos and broiler chickens. Animal. (2017) 11:1689–97. doi: 10.1017/S1751731117000374
96. Amer N, Hatab M, Sabic E. Efficacy of zinc/creatine supplementation on improving growth performance of local balady chicks. Braz J Poultry Sci. (2018) 20:219–30. doi: 10.1590/1806-9061-2017-0562
97. Ibrahim D, El Sayed R, Abdelfattah-Hassan A, Morshedy AM. Creatine or guanidinoacetic acid? Which is more effective at enhancing growth, tissue creatine stores, quality of meat, and genes controlling growth/myogenesis in Mulard ducks. J Appl Anim Res. (2019) 47:159–66. doi: 10.1080/09712119.2019.1590205
98. Alsever RN, Georg RH, Sussman KE. Stimulation of insulin secretion by guanidinoacetic acid and other guanidine derivatives. Endocrinology. (1970) 86:332–6. doi: 10.1210/endo-86-2-332
99. Qaid MM, Abdelrahman MM. Role of insulin and other related hormones in energy metabolism-A review. Cogent Food Agric. (2016) 2:1267691. doi: 10.1080/23311932.2016.1267691
100. Dimitriadis G, Mitrou P, Lambadiari V, Maratou E, Raptis SA. Insulin effects in muscle and adipose tissue. Diabetes Res Clin Pract. (2011) 93:S52–9. doi: 10.1016/S0168-8227(11)70014-6
101. Morris MS. Homocysteine and Alzheimer's disease. Lancet Neurol. (2003) 2:425–8. doi: 10.1016/S1474-4422(03)00438-1
102. Zugno AI, Stefanello FM, Scherer EBS, Mattos C, Pederzolli CD, Andrade VM, et al. Guanidinoacetate decreases antioxidant defenses and total protein sulfhydryl content in striatum of rats. Neurochem Res. (2008) 33:1804–10. doi: 10.1007/s11064-008-9636-6
103. Aziza A, Mahmoud R, Zahran E, Gadalla H. Dietary supplementation of guanidinoacetic acid improves growth, biochemical parameters, antioxidant capacity and cytokine responses in Nile tilapia (Oreochromis niloticus). Fish Shellfish Immunol. (2020) 97:367–74. doi: 10.1016/j.fsi.2019.12.052
Keywords: guanidinoacetic acid, creatine, muscle development, gene regulate, livestock and poultry
Citation: Yan Z, Yan Z, Liu S, Yin Y, Yang T and Chen Q (2021) Regulative Mechanism of Guanidinoacetic Acid on Skeletal Muscle Development and Its Application Prospects in Animal Husbandry: A Review. Front. Nutr. 8:714567. doi: 10.3389/fnut.2021.714567
Received: 25 May 2021; Accepted: 22 July 2021;
Published: 12 August 2021.
Edited by:
Marcello Iriti, University of Milan, ItalyReviewed by:
Giuseppe Annunziata, University of Naples Federico II, ItalyTatiana Carlesso Santos, State University of Maringá, Brazil
Copyright © 2021 Yan, Yan, Liu, Yin, Yang and Chen. This is an open-access article distributed under the terms of the Creative Commons Attribution License (CC BY). The use, distribution or reproduction in other forums is permitted, provided the original author(s) and the copyright owner(s) are credited and that the original publication in this journal is cited, in accordance with accepted academic practice. No use, distribution or reproduction is permitted which does not comply with these terms.
*Correspondence: Qinghua Chen, Y2hxaDMxNCYjeDAwMDQwOzE2My5jb20=