- 1Jaypee Institute of Information Technology, Noida, India
- 2Department of Medical Biotechnology, Yeungnam University, Gyeongsan, South Korea
- 3Research Institute of Cell Culture, Yeungnam University, Gyeongsan, South Korea
Diabetic cardiomyopathy (DCM) is an outcome of disturbances in metabolic activities through oxidative stress, local inflammation, and fibrosis, as well as a prime cause of fatality worldwide. Cardiovascular disorders in diabetic individuals have become a challenge in diagnosis and formulation of treatment prototype. It is necessary to have a better understanding of cellular pathophysiology that reveal the therapeutic targets and prevent the progression of cardiovascular diseases due to hyperglycemia. Critical changes in levels of collagen and integrin have been observed in the extracellular matrix of heart, which was responsible for cardiac remodeling in diabetic patients. This review explored the understanding of the mechanisms of how the phytochemicals provide cardioprotection under diabetes along with the caveats and provide future perspectives on these agents as prototypes for the development of drugs for managing DCM. Thus, here we summarized the effect of various plant extracts and natural polyphenols tested in preclinical and cell culture models of diabetic cardiomyopathy. Further, the potential use of selected polyphenols that improved the therapeutic efficacy against diabetic cardiomyopathy is also illustrated.
Introduction
Diabetes, a group of metabolic diseases associated with damage and dysfunction of various organs, especially the heart, and lead to cardiovascular diseases. The current burden of diabetes has become a major threat to the health of populations, which reflects the cumulative effects of risk factors over the life span of people. Present western lifestyle and environmental factors promote the progression of these pathological conditions and are held responsible for rising rate of diabetic cardiomyopathies (1, 2).
Persistent hyperglycemia causes the structural and molecular changes in cardiomyocytes, by increased production of advanced glycation end products (AGEs) in diabetes due to constant oxidative stress (3, 4). AGEs accumulate in various tissues and can link with other proteins such as collagen type IV, laminin, and fibronectin, resulting in impaired cardiac function and enhanced myocardial stiffness. A significant increase in AGEs and its specific receptors (RAGEs) on cells trigger oxidative stress and activate protein kinase C, finally disrupting cellular and molecular functions (5, 6). Oxidative stress induces the breaks in DNA, resulting in increased activity of poly ADP ribose polymerase (PARP) enzyme, which further promote the progression of various cardiovascular diseases (7).
The preponderance of diabetes will be accelerated by 50–60% in the population from 2015 to 2030, and also annual mortality rate attributing diabetes will be increased by 38% in the US population. Global healthcare expenditures were found to be higher to treat and prevent diabetes and its associated complications (~376 billion US Dollars (USD) in 2010). This cost is projected to exceed ~622 billion USD by 2030 (8, 9). However, the outcome of such expenditures is not satisfactory and people of low-income countries are still devoid of the treatments. Hence, an affordable therapy is required against diabetes, especially in the poorer socio-economic sections of country worldwide.
An alternative therapy by using plant polyphenols may have a great choice to end the cardiovascular complications developed by DCM. In this review, we discusses the pathophysiology and clinical features of DCM and the possible role of polyphenol in relation to the DCM therapy has been explored.
Pathophysiology of Diabetic Cardiomyopathy
In the following sections, we have described the physiological mechanisms associated with progression of diabetic cardiomyopathy (Figure 1).
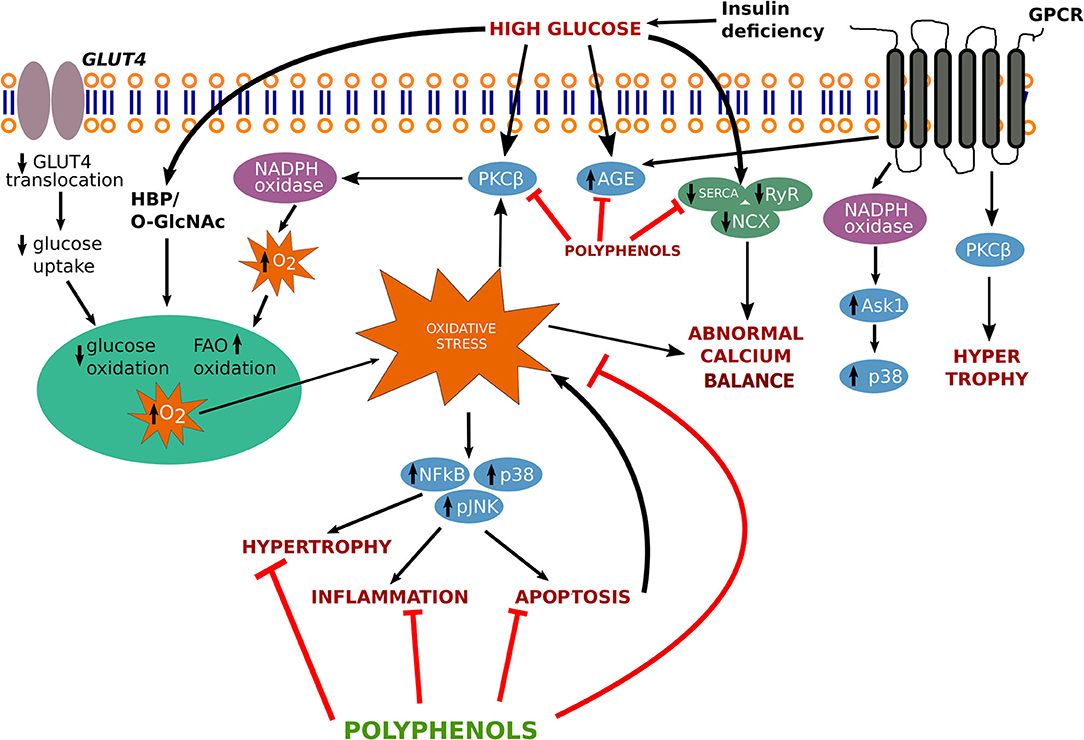
Figure 1. Signaling pathway involved in the progression of diabetic cardiomyopathy. AGEs, advanced glycation end-products; ASK1, apoptotic signal regulating kinase-1; GLUT-4, glucose transporter-4; GPCR, G protein coupled receptor; HBP, hexosamine biosynthesis pathway; JNK, c-Jun N-terminal kinase; NADPH, nicotinamide adenine dinucleotide phosphate; NCX, sodium–calcium exchanger; O-GlcNAc, O-linked beta-N-acetylglucosamine; p38, p38 MAPK; PKCβ, protein kinase C-β; RyR, ryanodine receptor SERCA, sarcoplasmic reticulum Ca2+ ATPase; NF-κB, nuclear factor- κB.
Oxidative Stress: Critical Contributor to Diabetic Cardiomyopathy
Free radicals are generated due to the continuous production of the oxygen, and considered as “reactive oxygen species” (ROS) (10). Stress inducing agents stimulate the drastic release of these oxidants and create an imbalance in the equilibrium of ROS production and antioxidant capability (11, 12).
Endothelial nitric oxide synthase (eNOS), a critical enzyme of endothelial cells, produces NO· and gets altered due to high glucose stress, which ultimately leads to vascular endothelial cell dysfunction. NO· further produces ONOO–, a cytotoxic free radical responsible for disturbing cardiovascular function (13). Oxidative stress is determined by the overproduction of ROS and RNS molecules. The unstable configuration leads to the breakdown of lipids, inactivation of enzymes, cell membranes, and DNA damage.
Calcium Homeostasis
Intracellular calcium (Ca2+) is a significant marker of cardiac contraction. Hyperglycemic stress enhances Ca2+ accumulation in cardiac myocytes, which imposes impairment in the ionotropic response in the heart. Ca2+ influx activates its release after binding to troponin C and generates tension by activating the sliding of thin and thick filaments, which further resulting in cardiac contraction (14). Intranuclear Ca2+ alteration may also change various kinases activities namely (extracellular-signal-regulated kinase) ERK, (microtubule-associated protein kinase) (MAPK) and Janus kinase (JNK). MAPKs are also involved in the transcription of c-fos and c-jun, which activate phospholipase A2, resulting in the plasma membrane and intracellular membranes permeabilization, further leading to cell death (15) (Figure 1).
Rennin-Angiotensin System
In diabetic cardiomyopathy, rennin-angiotensin (RAAS) system is considered a life-saving system. RAAS is a complex pathway whose activation triggers a cascade of events leading to cardiovascular disease. Studies show that infusing Angiotensin-II (AngII) leads to stimulation of ACE/AngII/AT1R complex accelerating atherosclerosis and blocking of RAAS protect against cardiac damage (16). RAAS can be activated by hyperglycemia, leading to production of Ang-II. It has been known that the Ang-II can produce ROS through NADH/NADPH oxidase system. RAAS's role in NADH/NADPH bound oxidase is further supported by studies showing the effectiveness of ramipril (and ACE inhibitor) in preventing upregulation of p47phox, p22phox, and reduced NADH driven oxide production (17). This led to reduced fibrosis and hypertrophic gene expression. Blocking of Ang-II also showed reduced expression of p22phox, NADH-oxidases and HG-induced p47phox (18). These studies show that the RAAS activity in diabetes supports an interaction between Ang-II and NADPH-oxidases in cardiomyocyte.
Extracellular (ECM) Remodeling in Diabetic Cardiomyopathy
The extracellular matrix is a complex meshwork of fibers comprised of proteins, polysaccharides, and provides structural as well as functional support to the surrounding cells, which are important for the cell-to-cell communication and adhesion. Alteration in the extracellular matrix components contributes to diabetic cardiomyopathy, which enhances stress in the diabetic heart which involves changes in mass, shape, and volume of the left ventricle, leading to ischemia, and pressure overload (19, 20). Changes in physiological conditions due to stress stimulus can trigger various proteases activities such as serine proteases and matrix metalloproteinases (MMPs) that cause alteration in the expression of collagen, fibronectin, and ultimately leads to ECM remodeling (21).
Collagen fibrils are the fundamental blocks of extracellular matrix and give mechanical strength, stiffness, and toughness to the vasculature (22). The extracellular collagen matrix of the myocardium has a major function in maintaining cardiac organization. An excessive accumulation of fibrillar collagen in the myocardium was reported in hypertrophied heart (23). In absence of secondary risk factors such as hypertension or coronary artery disease, cardiac dysfunction in diabetic patients, increased collagen I, III, and IV deposition has been found to results in fibrosis and poor LV function (24). There is a delicate balance between continual degradation and synthesis of collagen in ECM. Specific collagen degrading MMPs enzymes as well as their inhibitors (tissue inhibitors of metalloproteinases, TIMPs), are essential in collagen remodeling (25).
Matrix Metalloproteinases: Key Enzymes for ECM Modulation
MMPs have several conserved domains with different substrate specificity and inducibility. They play a major function in wound healing, tissue repair, and remodeling in various diseases. MMPs are of two types, membrane-bound and secretory. Membrane-type MMPs (MT-MMPs) work in close proximity with the cell, whereas the secreted MMPs act within the matrix, away from the cells from which they are synthesized. MMP-2 (Gelatinase A) and MMP-9 (Gelatinase B) are the most abundant secreted proteases, which degrade gelatin. They are categorized in 6 main classes such as- gelatinases, collagenases, stromelysins, matrilysins, membrane-type MMPs (MT-MMPs), and other MMPs (26).
MMPs are regulated in expression or activity and cellular inhibition by endogenous tissue inhibitors of metalloproteinases (TIMPs) (27). The MMP-TIMP balance maintains the integrity of ECM by regulating the debasement rate of ECM proteins and tissue remodeling. Most of MMP inhibitors have wide-spectrum actions on other MMPs and could cause adverse effects. Usage of synthetic MMP inhibitors in experimental animal models against upregulated activities of MMPs are failed in clinical trials, except FDA approved doxycyclin. The synthesis and design of new generation biological and synthetic MMP inhibitors are required.
Cardiac Hypertrophy in Diabetic Cardiomyopathy
High glucose uptake initiates an imbalance in myocardial energetics and results in myocardial ischemia or hypertrophy. Whenever cells are exposed to high glucose stress, they enlarge and undergo hypertrophy (increase in size, not in number) to combat the excessive stress, resulting in an increase in myocytes length (eccentric hypertrophy), or myocytes width (concentric hypertrophy), which further enhances thickening of the septum and ventricular wall (28).
Cardiac hypertrophy, a phenomenon observed with many forms of human heart disease including diabetic cardiomyopathy, results in an increase in protein synthesis, addition of sarcomeres and fetal genes re-expression such as myosin heavy chain (β-MHC) and GATA-1 and activation of early response genes, such as c-jun, c-fos, and c-myc etc. In hypertrophic conditions, various signaling pathways such as mitogen-activated protein kinases, tyrosine kinase Src, GTP-binding protein Ras, protein kinase C, phosphoinositol 3-kinase are involved (29) (Figure 1). Transforming growth factor β (TGF-β) mediates the production of transcription factors such as nuclear factor kappa B (NF-kB), small mothers against decapentaplegic (SMAD), signal transducer activating protein-1 (AP-1), and activator of transcription (STAT) that takes part in MMPs and TIMPs transcription leading to remodeling of the extracellular matrix.
Cardiac hypertrophy is prevalent in asymptomatic type 2 diabetes patients (30). The following fetal genes are used as an indicator/marker of cardiac hypertrophy under diabetic stress.
Sarcoplasmic Reticulum Ca2+ ATPase 2 (Serca2)
Sarcoplasmic reticulum Ca2+ ATPase 2 (Serca2), a candidate molecule for re-uptake of calcium into the sarcoplasmic reticulum, allows the muscle relaxation. Decreased Serca2 expression level was found in the diabetic heart and undergone diastolic dysfunction in cardiomyopathy (31). The SR Ca2+ loading determines the Ca2+ ion concentration available for next contraction dictating the rate of myocardial relaxation (32). Increases in Serca2 activity tend to enhance myocardial contraction and relaxation.
Myofilament Proteins
Myosin, actin, and titin filament proteins are highly expressed in the fetal heart than that of adult and major signs of cardiac hypertrophy. Myosin-binding protein C, interacts with actin, and changes the myosin cross-bridges (33) Myosin heavy chains (MHC), with integral ATPase activity, is one of the most underlying ways to find changes of MHC composition in the hypertrophied heart. Z-disc MLP–TCAP–titin complex defects can lead to cardiomyopathy and heart failure development.
Peptide Hormones
Atrial and brain natriuretic peptide (ANP and BNP) are small hormones, and their secretion occurs during cardiac stress. For acute heart failures, ANP and BNP can be clinically administered as therapeutic agents. For chronic cases, on the other hand, neprilysin (responsible for the degradation of ANP and BNP) (34) inhibitor is used. Recent studies reported that human proBNP, in bloodstream and its post- translational modification at the N-terminal region could lead to its higher levels in cardiac patients (35).
Transcription Factors and Inflammatory Signals
The main transcription factor, critically involved in hypertrophy is GATA-4, which was found to be highly expressed in the fetal myocardium. It triggers a wide group of heart- specific genes such as α and β-MHC (Myosin heavy chain), MEF-2 (Myocyte enhancer factor-2), SP-1 (Specificity protein-1), and NFκB (Nuclear factor-κappa B) associated with hypertrophy (36).
Natural Polyphenols: Therapeutics of DCM
Natural products have multiple pharmacological actions against pathological conditions and the use of these products is safer than synthetic drugs. The use of plants as a source of natural polyphenols in various forms of traditional medicines from ancient time. Huynh et al. illustrates the pathway involving several cascades involves in the progression of diabetic cardiomyopathy (37). Based on the findings the key findings on disease pathways and treatment options such as targeting antioxidant-signaling pathways, we also proposed a figure that involves in the treatment strategy through various polyphenolics to target several genes and ameliorates the pathological conditions arises by cardiomyopathy (Figure 1).
Herbal remedies are gaining significant attention and that nearly 80% of the total world population uses conventional medicine, comprising 40,000–70,000 medicinal plants (38, 39). In recent years, due to toxicological concerns with the synthetic substances in food and increasing awareness about herbal therapies, the use of natural substances are demanding. Herbal formulations are found to be cost-effective and having low side effects. Plant extracts have recently gained interest due to their antiglycoxidative activities, that defend cells against the free radical attack and have numerous biological consequences (40, 41). The synthesis of different organic solvent extracts may differ in quality and concentration depending upon the difference in the polarity of the solvent used for extraction and the extracted polyphenols (42, 43). Phenolic compounds such as gallic acid, caffeic acid, ferulic acid, trans-resveratrol, quercetin, fisetin have been shown to act as natural antioxidants by neutralizing free radicals (44, 45).
We have summarized below some plant extracts and natural polyphenols, showing doses of polyphenols, experimental models studied, and key findings, which further suggest their beneficial effects against cardiovascular abnormalities (Table 1). In vivo studies indicated the beneficial effects of some polyphenols in DCM such as methanolic extract of S. cumini seeds treated group showed normal morphological cardiac features compared to the diabetic control. This may be due to the presence of quercetin, ellagic acid, rutin, and gallic acid in the extract which contributes to reducing aspartate aminotransferase (AST), creatine kinase-MB (CK-MB), and lactate dehydrogenase (LDH) level up to the normal (46, 47). The combined effect of A. sativum and voluntary exercise has worked as a powerful defense system, which decreases HbA1c and malondialdehyde (MDA) production in cardiomyocytes of diabetic models (48). B. oleracea also found to upregulate Nrf2 activation, a critical marker of DCM, which were found to be decreased in diabetic models (49). Decreasing MDA and AGEs formation levels was observed on the administration of H. sabdariffa (100 mg/Kg) in STZ treated rats, which also improved cardiac function by augmenting mitochondrial antioxidant defense (50). Administration of M. oleifera significantly reduced the lipid peroxidation products and increased the enzymatic as well as non-enzymatic antioxidants in the diabetic rat (51). Administration of A. augusta and A. marmelos could significantly reduce the levels of Interleukin (IL-6) IL-6, IL-1β, and (tumor necrosis factor- α) TNF-α in cardiac tissues of diabetic rats (52, 53), stimulates the antioxidants defense system by increasing catalase (CAT), superoxide dismutase (SOD) activities, and maintained the cardiac integrity. E. oleracea treatment along with exercise reduced leptin, IL-6, and TNF-α serum levels in diabetic models, which may improve the insulin sensitivity (54). P. pinnata significantly diminished the activity of cardiac enzymes such as LDH, CK-MB, AST compared with diabetic rats showing its cardioprotective effects (55). Resveratrol also promotes Nrf2 mediated cardiac protection (56). Treatment of catechins significantly increases SOD, CAT, and GSH activities up to control in diabetic rat hearts, however decreasing the higher levels of cardiac biomarkers CK-MB, AST, LDH, and troponin T, suggesting its cardioprotective effect (57). Curcumin supplementation leads to lowering the level of blood glucose and proinflammatory cytokines in DCM models (58).
Plant polyphenols have been studied extensively through in vitro cell models for the treatment of cardiovascular diseases. In cardiac H9C2 cells, gallic acid was found to suppress hypertrophy and fibrosis by regulating JNK2 signaling and Smad3 binding. In these cells, ferulic acid was also shown to protect cardiomyoblasts from high glucose induced oxidative stress by mediating Ca2+ homeostasis (68). Similarly, epigallocatechin-3-gallate mediated cardioprotection was observed through Akt/GSK-3β/caveolin signaling (69). Quercetin, a flavonoid, inhibits AP-1, and activates the PPAR-γ pathways leading to protection against cardiac hypertrophy (70). Another agents such as, resveratrol and kaempferol have been shown to involve in Sirt-1 dependent pathways to provide protection against ER stress and reoxygenation injuries (71, 72) leading to decrease in cardiomyocyte apoptosis. Administration of curcumin (10 μM) in cardiac cells, combat the glucose induced stress by reducing the overproduction of ROS and apoptosis through PI3K/Akt pathway (67).
Polyphenols are large phytochemicals and only a small fraction of them seem to be absorbed by the gastrointestinal tract in their consumed form (73, 74). It is assumed that a large portion of these is first decomposed by the gut microbiome into lower molecule metabolites that are then absorbed in the gut. The polyphenols could work in two ways: firstly, the polyphenols could be broken down in the gut to produce several different metabolites which are absorbed and enter the system circulation. Secondly, polyphenols can directly affect the gut microbiota leading to health benefits in the patients. This could be through a change in microbiome composition (75), by facilitating the generation of short-chain fatty acids (76) and improving oxygen levels by reacting with ROS and improving the immune system in the gut (77), however, such mechanisms are unavailable to in vitro models, which could be a major limitation of these studies.
Conclusion and Future Perspectives
Hyperglycemia is strongly correlated with the manifestation of cardiac malfunction and heart failure. Preclinical studies also revealed the beneficial effects of antioxidants, anti-inflammatory agents on cardiac dysfunction.
Although DCM has been found in both type-1 and type-2 diabetes, hyperglycemia induced cardiac fibrosis has been mainly observed in type-I DM hearts. Type-2 diabetes is primarily associated with cardiomyocyte hypertrophy and steatosis (78–80). Therefore, the studies showed in this paper are limited for type 1 diabetes.
In vitro studies involving high dosage of anti-oxidants have shown their protective effects. However, larger studies especially in vivo and clinical trials have shown variable results. Large clinical trials with anti-oxidant agents like vitamin C and E have not been able to provide clear evidence of their beneficial effects in diabetic patients. One of the reason anti-oxidant treatments are failed because the overall oxidation levels in cells are strongly regulated. Simply using anti-oxidants concentrates, as supplements may not work, as intended and new techniques need to be developed to improve the efficacy of anti-oxidant treatments. One such recent successful method is by reversing loss of enzyme functions such as treatment with drugs that prevent ROS-induced eNOS uncoupling (81). Another approach could be delivery of anti-oxidant enzymes using viral transfection gene therapy and targeted delivery at cellular or sub-cellular levels (82). Additionally, nanoparticles based drug delivery may lead to an effective treatment against such diabetic cardiomyopathies.
Author Contributions
NA and VR designed the manuscript. NA, DY, VR, and J-OJ wrote the manuscript. NA, DY, and J-OJ edited the manuscript. All authors contributed to the article and approved the submitted version.
Funding
This work was supported by the National Research Foundation of Korea (NRF) funded by the Ministry of Education (NRF-2019R1G1A1008566). Basic Science Research Program through the NRF funded by the Ministry of Education (2020R1A6A1A03044512).
Conflict of Interest
The authors declare that the research was conducted in the absence of any commercial or financial relationships that could be construed as a potential conflict of interest.
References
1. Gilca G-E, Stefanescu G, Badulescu O, Tanase D-M, Bararu I, Ciocoiu M. Diabetic cardiomyopathy: current approach and potential diagnostic and therapeutic targets. J Diabetes Res. (2017) 2017:1310265. doi: 10.1155/2017/1310265
2. Ormazabal V, Nair S, Elfeky O, Aguayo C, Salomon C, Zuñiga FA. Association between insulin resistance and the development of cardiovascular disease. Cardiovasc Diabetol. (2018) 17:122. doi: 10.1186/s12933-018-0762-4
3. Nowotny K, Jung T, Hohn A, Weber D, Grune T. Advanced glycation end products and oxidative stress in type 2 diabetes mellitus. Biomolecules. (2015) 5:194–222. doi: 10.3390/biom5010194
4. Fishman SL, Sonmez H, Basman C, Singh V, Poretsky L. The role of advanced glycation end-products in the development of coronary artery disease in patients with and without diabetes mellitus: a review. Mol Med. (2018) 24:59. doi: 10.1186/s10020-018-0060-3
5. Giacco F, Brownlee M. Oxidative stress and diabetic complications. Circ Res. (2010) 107:1058–70. doi: 10.1161/CIRCRESAHA.110.223545
6. Singh VP, Bali A, Singh N, Jaggi AS. Advanced glycation end products and diabetic complications. Korean J Physiol Pharmacol. (2014) 18:1–14. doi: 10.4196/kjpp.2014.18.1.1
7. Pacher P, Szabó C. Role of poly (ADP-ribose) polymerase-1 activation in the pathogenesis of diabetic complications: endothelial dysfunction, as a common underlying theme. Antioxidants Redox Signal. (2005) 7:1568–80. doi: 10.1089/ars.2005.7.1568
8. Zhang P, Zhang X, Brown J, Vistisen D, Sicree R, Shaw J, et al. Global healthcare expenditure on diabetes for 2010 and 2030. Diabetes Res Clin Pract. (2010) 87:293–301. doi: 10.1016/j.diabres.2010.01.026
9. Rowley WR, Bezold C, Arikan Y, Byrne E, Krohe S. Diabetes 2030: insights from yesterday, today, and future trends. Popul Health Manag. (2017) 20:6–12. doi: 10.1089/pop.2015.0181
10. Rahal A, Kumar A, Singh V, Yadav B, Tiwari R, Chakraborty S, et al. Oxidative stress, prooxidants, and antioxidants: the interplay. BioMed Res Int. (2014) 2014:761264. doi: 10.1155/2014/761264
11. Kurutas EB. The importance of antioxidants which play the role in cellular response against oxidative/nitrosative stress: current state. Nutr J. (2016) 15:71. doi: 10.1186/s12937-016-0186-5
12. Pizzino G, Irrera N, Cucinotta M, Pallio G, Mannino F, Arcoraci V, et al. Oxidative stress: harms and benefits for human health. Oxidative Med Cell Longevity. (2017) 2017:8416763–8416763. doi: 10.1155/2017/8416763
13. Elahi MM, Kong YX, Matata BM. Oxidative stress as a mediator of cardiovascular disease. Oxidative Med Cell Longevity. (2009) 2:259–69. doi: 10.4161/oxim.2.5.9441
14. Cesario J, Plaks JE, Higgins ET. Automatic social behavior as motivated preparation to interact. J Pers Soc Psychol. (2006) 90:893–910. doi: 10.1037/0022-3514.90.6.893
15. Zhivotovsky B, Orrenius S. Calcium and cell death mechanisms: a perspective from the cell death community. Cell Calcium. (2011) 50:211–21. doi: 10.1016/j.ceca.2011.03.003
16. Wollert KC, Drexler H. The renin–angiotensin system and experimental heart failure. Cardiovas Res. (1999) 43:838–49. doi: 10.1016/S0008-6363(99)00145-5
17. Huynh K, Kiriazis H, Du X-J, Love JE, Gray SP, Jandeleit-Dahm KA, et al. Targeting the upregulation of reactive oxygen species subsequent to hyperglycemia prevents type 1 diabetic cardiomyopathy in mice. Free Radical Biol Med. (2013) 60:307–17. doi: 10.1016/j.freeradbiomed.2013.02.021
18. Fukuda M, Nakamura T, Kataoka K, Nako H, Tokutomi Y, Dong Y-F, et al. Potentiation by candesartan of protective effects of pioglitazone against type 2 diabetic cardiovascular and renal complications in obese mice. J Hypertension. (2010) 28:340–52. doi: 10.1097/HJH.0b013e32833366cd
19. Kehat I, Molkentin JD. Molecular pathways underlying cardiac remodeling during pathophysiological stimulation. Circulation. (2010) 122:2727–35. doi: 10.1161/CIRCULATIONAHA.110.942268
20. Rienks M, Papageorgiou A-P, Frangogiannis NG, Heymans S. Myocardial extracellular matrix: an ever-changing and diverse entity. Circulation Res. (2014) 114:872–88. doi: 10.1161/CIRCRESAHA.114.302533
21. Abedin M, King N. Diverse evolutionary paths to cell adhesion. Trends Cell Biol. (2010) 20:734–42. doi: 10.1016/j.tcb.2010.08.002
22. Martufi G, Gasser TC. Turnover of fibrillar collagen in soft biological tissue with application to the expansion of abdominal aortic aneurysms. J Royal Soc Interface. (2012) 9:3366–77. doi: 10.1098/rsif.2012.0416
23. Lopez Salazar B, Ravassa Albeniz S, Arias Guedon T, Gonzalez Miqueo A, Querejeta R, Diez Martinez J. Altered fibrillar collagen metabolism in hypertensive heart failure. current understanding and future prospects. Rev Esp Cardiol. (2006) 59:1047–57. doi: 10.1157/13093982
24. Aneja A, Tang WW, Bansilal S, Garcia MJ, Farkouh ME. Diabetic cardiomyopathy: insights into pathogenesis, diagnostic challenges, and therapeutic options. Am J Med. (2008) 121:748–57. doi: 10.1016/j.amjmed.2008.03.046
25. Kwak H-B. Aging, exercise, and extracellular matrix in the heart. J Exercise Rehabil. (2013) 9:338–47. doi: 10.12965/jer.130049
26. Benjamin MM, Khalil RA. Matrix metalloproteinase inhibitors as investigative tools in the pathogenesis and management of vascular disease. Exp Supplement. (2012) 103:209–79. doi: 10.1007/978-3-0348-0364-9_7
27. Sun J. Matrix metalloproteinases and tissue inhibitor of metalloproteinases are essential for the inflammatory response in cancer cells. J Signal Transduct. (2010) 2010:985132. doi: 10.1155/2010/985132
28. Bayes-Genis A. Hypertrophy and inflammation: too much for one heart. Eur Heart J. (2007) 28:661–63. doi: 10.1093/eurheartj/ehm008
29. Cambronero F, Marin F, Roldan V, Hernandez-Romero D, Valdes M, Lip GY. Biomarkers of pathophysiology in hypertrophic cardiomyopathy: implications for clinical management and prognosis. Eur Heart J. (2009) 30:139–51. doi: 10.1093/eurheartj/ehn538
30. Somaratne JB, Whalley GA, Poppe KK, Ter Bals MM, Wadams G, Pearl A, et al. Screening for left ventricular hypertrophy in patients with type 2 diabetes mellitus in the community. Cardiovas Diabetol. (2011) 10:29. doi: 10.1186/1475-2840-10-29
31. Nikolajevic Starcevic J, Janic M, Sabovic M. Molecular mechanisms responsible for diastolic dysfunction in diabetes mellitus patients. Int J Mol Sci. (2019) 20:1197. doi: 10.3390/ijms20051197
32. Davis JP, Tikunova SB. Ca2+ exchange with troponin C and cardiac muscle dynamics. Cardiovasc Res. (2008) 77:619–26. doi: 10.1093/cvr/cvm098
33. Waddingham MT, Edgley AJ, Astolfo A, Inagaki T, Fujii Y, Du C-K, et al. Chronic Rho-kinase inhibition improves left ventricular contractile dysfunction in early type-1 diabetes by increasing myosin cross-bridge extension. Cardiovasc Diabetol. (2015) 14:92. doi: 10.1186/s12933-015-0256-6
34. Potter LR. Natriuretic peptide metabolism, clearance and degradation. FEBS J. (2011) 278:1808–17. doi: 10.1111/j.1742-4658.2011.08082.x
35. Sato Y, Ishizaki Y, Aso K, Minakwa A, Toida T, Nishizono R, et al. Characterisation of N-terminal pro-brain natriuretic peptide in dialysis patients and its reduced prognostic significance in the elderly. Sci Rep. (2019) 9:1–9. doi: 10.1038/s41598-019-43253-z
36. Cox EJ, Marsh SA. A systematic review of fetal genes as biomarkers of cardiac hypertrophy in rodent models of diabetes. PLoS ONE. (2014) 9:e92903. doi: 10.1371/journal.pone.0092903
37. Huynh K, Bernardo BC, Mcmullen JR, Ritchie RH. Diabetic cardiomyopathy: mechanisms and new treatment strategies targeting antioxidant signaling pathways. Pharmacol Ther. (2014) 142:375–415. doi: 10.1016/j.pharmthera.2014.01.003
38. Ekor M. The growing use of herbal medicines: issues relating to adverse reactions and challenges in monitoring safety. Front Pharmacol. (2014) 4:177. doi: 10.3389/fphar.2013.00177
39. Yadav D, Cho K-H. Preventive and therapeutic aspects of selected herbal medicines in diabetes mellitus. Prog Nutr. (2017) 19:117–26. doi: 10.23751/pn.v19i2.5976
40. Masibo M, He Q. Major mango polyphenols and their potential significance to human health. Comprehen Rev Food Sci Food Safety. (2008) 7:309–19. doi: 10.1111/j.1541-4337.2008.00047.x
41. Yeh W-J, Hsia S-M, Lee W-H, Wu C-H. Polyphenols with antiglycation activity and mechanisms of action: a review of recent findings. J Food Drug Anal. (2017) 25:84–92. doi: 10.1016/j.jfda.2016.10.017
42. Durling NE, Catchpole OJ, Grey JB, Webby RF, Mitchell KA, Foo LY, et al. Extraction of phenolics and essential oil from dried sage (Salvia officinalis) using ethanol–water mixtures. Food Chem. (2007) 101:1417–24. doi: 10.1016/j.foodchem.2006.03.050
43. Alothman M, Bhat R, Karim A. Antioxidant capacity and phenolic content of selected tropical fruits from Malaysia, extracted with different solvents. Food Chem. (2009) 115:785–8. doi: 10.1016/j.foodchem.2008.12.005
44. Ndhlala AR, Moyo M, Van Staden J. Natural antioxidants: fascinating or mythical biomolecules? Molecules. (2010) 15:6905–30. doi: 10.3390/molecules15106905
45. Singh AK, Patel PK, Choudhary K, Joshi J, Yadav D, Jin JO. Quercetin and coumarin inhibit Dipeptidyl Peptidase-IV and exhibits antioxidant properties: in silico, in vitro, ex vivo. Biomolecules. (2020) 10:207. doi: 10.3390/biom10020207
46. Nahid S, Mazumder K, Rahman Z, Islam S, Rashid MH, Kerr PG. Cardio- and hepato-protective potential of methanolic extract of Syzygium cumini (L.) Skeels seeds: a diabetic rat model study. Asian Pacif J Trop Biomed. (2017) 7:126–33. doi: 10.1016/j.apjtb.2016.11.025
47. Chagas VT, Coelho RMRDS, Gaspar RS, Da Silva SA, Mastrogiovanni M, Mendonça CDJ, et al. Protective effects of a polyphenol-rich extract from Syzygium cumini (L.) skeels leaf on oxidative stress-induced diabetic rats. Oxidative Med Cell Longevity. (2018) 2018:5386079. doi: 10.1155/2018/5386079
48. Ghyasi R, Mohaddes G, Naderi R. Combination effect of voluntary exercise and garlic (Allium sativum) on oxidative stress, cholesterol level and histopathology of heart tissue in type 1 diabetic rats. J Cardiovas Thoracic Res. (2019) 11:61–7. doi: 10.15171/jcvtr.2019.10
49. Xu Z, Wang S, Ji H, Zhang Z, Chen J, Tan Y, et al. Broccoli sprout extract prevents diabetic cardiomyopathy via Nrf2 activation in db/db T2DM mice. Sci Rep. (2016) 6:30252. doi: 10.1038/srep30252
50. Mohammed Yusof NL, Zainalabidin S, Mohd Fauzi N, Budin SB. Hibiscus sabdariffa (roselle) polyphenol-rich extract averts cardiac functional and structural abnormalities in type 1 diabetic rats. Appl Physiol Nutr Metab. (2018) 43:1224–32. doi: 10.1139/apnm-2018-0084
51. Aju BY, Rajalakshmi R, Mini S. Protective role of Moringa oleifera leaf extract on cardiac antioxidant status and lipid peroxidation in streptozotocin induced diabetic rats. Heliyon. (2019) 5:e02935. doi: 10.1016/j.heliyon.2019.e02935
52. Bhatti R, Sharma S, Singh J, Ishar MPS. Ameliorative effect of Aegle marmelos leaf extract on early stage alloxan-induced diabetic cardiomyopathy in rats. Pharm Biol. (2011) 49:1137–43. doi: 10.3109/13880209.2011.572077
53. Khanra R, Dewanjee S, Dua TK, Sahu R, Gangopadhyay M, De Feo V, et al. Abroma augusta L. (Malvaceae) leaf extract attenuates diabetes induced nephropathy and cardiomyopathy via inhibition of oxidative stress and inflammatory response. J Translat Med. (2015) 13:6. doi: 10.1186/s12967-014-0364-1
54. De Bem GF, Costa CA, Santos IB, Cristino Cordeiro VDS, De Carvalho L, De Souza M, et al. Antidiabetic effect of Euterpe oleracea Mart. (acai) extract and exercise training on high-fat diet and streptozotocin-induced diabetic rats: A positive interaction. PLoS ONE. (2018) 13:e0199207. doi: 10.1371/journal.pone.0199207
55. Badole SL, Chaudhari SM, Jangam GB, Kandhare AD, Bodhankar SL. Cardioprotective activity of Pongamia pinnata in streptozotocin-nicotinamide induced diabetic rats. BioMed Res Internat. (2015) 2015:403291. doi: 10.1155/2015/403291
56. Wang G, Song X, Zhao L, Li Z, Liu B. Resveratrol prevents diabetic cardiomyopathy by increasing Nrf2 expression and transcriptional activity. BioMed Res Int. (2018) 2018:2150218. doi: 10.1155/2018/2150218
57. Othman AI, El-Sawi MR, El-Missiry MA, Abukhalil MH. Epigallocatechin-3-gallate protects against diabetic cardiomyopathy through modulating the cardiometabolic risk factors, oxidative stress, inflammation, cell death and fibrosis in streptozotocin-nicotinamide-induced diabetic rats. Biomed Pharmacother. (2017) 94:362–73. doi: 10.1016/j.biopha.2017.07.129
58. Jain SK, Rains J, Croad J, Larson B, Jones K. Curcumin supplementation lowers TNF-alpha, IL-6, IL-8, and MCP-1 secretion in high glucose-treated cultured monocytes and blood levels of TNF-alpha, IL-6, MCP-1, glucose, and glycosylated hemoglobin in diabetic rats. Antioxidants Redox Signal. (2009) 11:241–9. doi: 10.1089/ars.2008.2140
59. Atale N, Chakraborty M, Mohanty S, Bhattacharya S, Nigam D, Sharma M, et al. Cardioprotective role of Syzygium cumini against glucose-induced oxidative stress in H9C2 cardiac myocytes. Cardiovasc Toxicol. (2013) 13:278–89. doi: 10.1007/s12012-013-9207-1
60. Atale N, Gupta K, Rani V. Protective effect of Syzygium cumini against pesticide-induced cardiotoxicity. Environ Sci Pollut Res. (2014) 21:7956–72. doi: 10.1007/s11356-014-2684-3
61. Khatua TN, Borkar RM, Mohammed SA, Dinda AK, Srinivas R, Banerjee SK. Novel sulfur metabolites of garlic attenuate cardiac hypertrophy and remodeling through induction of Na+/K+-ATPase expression. Front Pharmacol. (2017) 8:18. doi: 10.3389/fphar.2017.00018
62. Yang DK. Cabbage (Brassica oleracea var. capitata) protects against H(2)O(2)-induced oxidative stress by preventing mitochondrial dysfunction in H9c2 cardiomyoblasts. Evid Based Comple Alternat Med. (2018) 2018:2179021. doi: 10.1155/2018/2179021
63. Xu K, Liu XF, Ke ZQ, Yao Q, Guo S, Liu C. Resveratrol modulates apoptosis and autophagy induced by high glucose and palmitate in cardiac cells. Cell Physiol Biochem. (2018) 46:2031–40. doi: 10.1159/000489442
64. Sheng R, Gu ZL, Xie ML, Zhou WX, Guo CY. Epigallocatechin gallate protects H9c2 cardiomyoblasts against hydrogen dioxides- induced apoptosis and telomere attrition. Eur J Pharmacol. (2010) 641:199–206. doi: 10.1016/j.ejphar.2010.05.054
65. Ahuja S, Kohli S, Krishnan S, Dogra D, Sharma D, Rani V. Curcumin: a potential therapeutic polyphenol, prevents noradrenaline-induced hypertrophy in rat cardiac myocytes. J Pharm Pharmacol. (2011) 63:1604–12. doi: 10.1111/j.2042-7158.2011.01363.x
66. Kohli S, Chhabra A, Jaiswal A, Rustagi Y, Sharma M, Rani V. Curcumin suppresses gelatinase B mediated norepinephrine induced stress in H9c2 cardiomyocytes. PLoS ONE. (2013) 8:e76519. doi: 10.1371/journal.pone.0076519
67. Yu W, Zha W, Ke Z, Min Q, Li C, Sun H, et al. Curcumin protects neonatal rat cardiomyocytes against high glucose-induced apoptosis via PI3K/Akt signalling pathway. J Diabetes Res. (2016) 2016:1–11. doi: 10.1155/2016/4158591
68. Salin Raj P, Swapna SU, Raghu KG. High glucose induced calcium overload via impairment of SERCA/PLN pathway and mitochondrial dysfunction leads to oxidative stress in H9c2 cells and amelioration with ferulic acid. Fundamental Clin Pharmacol. (2019) 33:412–25. doi: 10.1111/fcp.12452
69. Hsieh S-R, Hsu C-S, Lu C-H, Chen W-C, Chiu C-H, Liou Y-M. Epigallocatechin-3-gallate-mediated cardioprotection by Akt/GSK-3β/caveolin signalling in H9c2 rat cardiomyoblasts. J Biomed Sci. (2013) 20:86. doi: 10.1186/1423-0127-20-86
70. Yan L, Zhang JD, Wang B, Lv YJ, Jiang H, Liu GL, et al. Quercetin inhibits left ventricular hypertrophy in spontaneously hypertensive rats and inhibits angiotensin II-induced H9C2 cells hypertrophy by enhancing PPAR-γ expression and suppressing AP-1 activity. PLoS ONE. (2013) 8:e72548. doi: 10.1371/journal.pone.0072548
71. Li Y-G, Zhu W, Tao J-P, Xin P, Liu M-Y, Li J-B, et al. Resveratrol protects cardiomyocytes from oxidative stress through SIRT1 and mitochondrial biogenesis signaling pathways. Biochem Biophys Res Commun. (2013) 438:270–6. doi: 10.1016/j.bbrc.2013.07.042
72. Guo Z, Liao Z, Huang L, Liu D, Yin D, He M. Kaempferol protects cardiomyocytes against anoxia/reoxygenation injury via mitochondrial pathway mediated by SIRT1. Europ J Pharmacol. (2015) 761:245–53. doi: 10.1016/j.ejphar.2015.05.056
73. Gowd V, Karim N, Shishir MRI, Xie L, Chen W. Dietary polyphenols to combat the metabolic diseases via altering gut microbiota. Trends Food Sci Technol. (2019) 93:81–93. doi: 10.1016/j.tifs.2019.09.005
74. Catalkaya G, Venema K, Lucini L, Rocchetti G, Delmas D, Daglia M, et al. Interaction of dietary polyphenols and gut microbiota: microbial metabolism of polyphenols, influence on the gut microbiota, and implications on host health. Food Front. (2020) 1:109–33. doi: 10.1002/fft2.25
75. Pistollato F, Sumalla Cano S, Elio I, Masias Vergara M, Giampieri F, Battino M. Role of gut microbiota and nutrients in amyloid formation and pathogenesis of Alzheimer disease. Nutr Rev. (2016) 74:624–34. doi: 10.1093/nutrit/nuw023
76. Parkar SG, Trower TM, Stevenson DE. Fecal microbial metabolism of polyphenols and its effects on human gut microbiota. Anaerobe. (2013) 23:12–9. doi: 10.1016/j.anaerobe.2013.07.009
77. Liso M, De Santis S, Scarano A, Verna G, Dicarlo M, Galleggiante V, et al. A bronze-tomato enriched diet affects the intestinal microbiome under homeostatic and inflammatory conditions. Nutrients. (2018) 10:1862. doi: 10.3390/nu10121862
78. Phillips RA, Krakoff LR, Dunaif A, Finegood DT, Gorlin R, Shimabukuro S. Relation among left ventricular mass, insulin resistance, and blood pressure in nonobese subjects. J Clin Endocrinol Metab. (1998) 83:4284–8. doi: 10.1210/jc.83.12.4284
79. Battiprolu PK, Lopez-Crisosto C, Wang ZV, Nemchenko A, Lavandero S, Hill JA. Diabetic cardiomyopathy and metabolic remodeling of the heart. Life Sci. (2013) 92:609–15. doi: 10.1016/j.lfs.2012.10.011
80. Fuentes-Antrás J, Picatoste B, Ramírez E, Egido J, Tuñón J, Lorenzo Ó. Targeting metabolic disturbance in the diabetic heart. Cardiovasc Diabetol. (2015) 14:17. doi: 10.1186/s12933-015-0173-8
81. Wenzel P, Schulz E, Oelze M, Müller J, Schuhmacher S, Alhamdani MS, et al. AT1-receptor blockade by telmisartan upregulates GTP-cyclohydrolase I and protects eNOS in diabetic rats. Free Radical Biol Med. (2008) 45:619–26. doi: 10.1016/j.freeradbiomed.2008.05.009
Keywords: diabetic cardiomyopathy, oxidative stress, extracellular matrix, cardiac hypertrophy, natural polyphenols
Citation: Atale N, Yadav D, Rani V and Jin J-O (2020) Pathophysiology, Clinical Characteristics of Diabetic Cardiomyopathy: Therapeutic Potential of Natural Polyphenols. Front. Nutr. 7:564352. doi: 10.3389/fnut.2020.564352
Received: 21 May 2020; Accepted: 27 October 2020;
Published: 03 December 2020.
Edited by:
Mohammad Amjad Kamal, King Abdulaziz University, Saudi ArabiaReviewed by:
Oscar Lorenzo, Universidad Autónoma Madrid, SpainAnandh Babu Pon Velayutham, The University of Utah, United States
Copyright © 2020 Atale, Yadav, Rani and Jin. This is an open-access article distributed under the terms of the Creative Commons Attribution License (CC BY). The use, distribution or reproduction in other forums is permitted, provided the original author(s) and the copyright owner(s) are credited and that the original publication in this journal is cited, in accordance with accepted academic practice. No use, distribution or reproduction is permitted which does not comply with these terms.
*Correspondence: Vibha Rani, dmliaGEucmFuaSYjeDAwMDQwO2ppaXQuYWMuaW4=; Jun-O Jin, amluam8mI3gwMDA0MDt5dS5hYy5rcg==
†These authors have contributed equally to this work