- Department of Nutrition, University of California, Davis, Davis, CA, United States
The gut microbiome is a key factor in determining inter-individual variability in response to diet. Thus, far, research in this area has focused on metabolic health outcomes such as obesity and type 2 diabetes. However, understanding the role of the gut microbiome in determining response to diet may also lead to improved personalization of sports nutrition for athletic performance. The gut microbiome has been shown to modify the effect of both diet and exercise, making it relevant to the athlete's pursuit of optimal performance. This area of research can benefit from recent developments in the general field of personalized nutrition and has the potential to expand our knowledge of the nexus between the gut microbiome, lifestyle, and individual physiology.
Introduction
The gut microbiome has been implicated in the modulation of human health and metabolism (1, 2). This microbial “organ” has been linked to nutrition-related chronic diseases such as obesity and diabetes (3–6) and has also been shown to influence systemic functions including immunity (7, 8) and brain function (9, 10). The gut microbiome may influence health via mechanisms such as the production of metabolites (2, 11) [e.g., short-chain fatty acids (SCFAs)] that can influence a wide array of host systems and metabolic pathways (12, 13).
However, the gut microbiome is not a fixed trait, but instead responds to environmental stimuli and is a malleable part of the human supraorganism (14) (Figure 1). Much of microbiome research has focused on the effect of lifestyle factors, such as diet (15–17) and exercise (18, 19), on the gut microbiota. Variability in the composition and function of the gut microbiome (20, 21) has also fueled research on the relationship between features of the gut microbiota, such as diversity or the presence, absence, or amount of certain taxa, and host health. Precision nutrition studies are now investigating how to predict individual differences in glycemic response, triglycerides, cholesterol levels, and other indicators of health as a way to personalize nutrition recommendations and prevent diet-related chronic diseases such as obesity and type 2 diabetes. Our previous two-part review (22, 23) explored the effect of the gut microbiome on inter-individual variability in response to diet and how this may contribute to metabolic health.
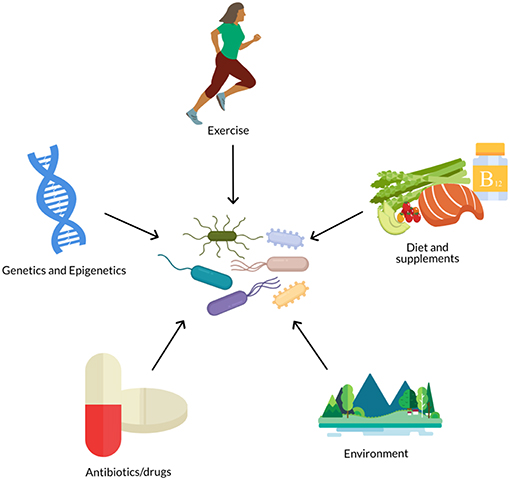
Figure 1. The gut microbiome is influenced by numerous biological and lifestyle factors such as diet, genetics, antibiotics, exercise, and environment (e.g., pollutants, urban vs. rural, etc.).
Alternatively, we may consider the potential effect of the gut microbiome on measures of athletic performance. Successful performance in training, such as a time trial or rep max load, and general metabolic health are two distinct aspects of metabolic response that are not necessarily directly coupled (24). While nutrition is an important part of general health and well-being, it is also an important tool in an athlete's arsenal to optimize performance (25).
Variability in the physiological response to training and nutrition has been attributed to factors such as age, sex, training history, initial training status, psychological factors, and the mode, duration, intensity, and frequency of training (26). Genetics has also become a large topic of research in the area of variability in response to exercise training and potentially ergogenic dietary components (27–38). It is possible that variability in the gut microbiome may also influence gains in performance in response to training and nutrition. Despite the growing interest in the gut microbiome and personalized nutrition, very few studies have combined these fields with that of athletic performance. This is surprising as athletes are extremely motivated to capitalize on any advantage, however small, that could increase their performance. This review focuses on several topics related to the question of whether the gut microbiome may be used to predict performance response to dietary and/or training interventions. This includes topics such as (1) the effect of exercise on the gut microbiome, (2) the effect of dietary components or patterns relevant to athletic nutrition on the gut microbiome, and (3) the effect of the gut microbiome on performance response to diet and exercise. Each of these related topics will be discussed, as will gaps in the research and future directions.
Methods
Numerous reviews have been published highlighting the effect of exercise on the gut microbiota (39–56). However, the primary focus of these reviews has been the implications for aspects of host health, such as the immune system and risk of chronic diseases. Only a few have discussed the implications for athletic performance (41, 48, 52). This review aims to provide a more in-depth discussion of the interactive effect between the gut microbiota and diet on athletic performance and highlight the need for further research in this area. A literature search in PubMed and Google Scholar, including combinations of key words “gut microbiota”, “exercise”, “performance,” “variability,” and “effect,” was used to identify relevant studies. References were also obtained from the above review articles.
The earliest study found was published in 2008 by Matsumoto et al. (57) but was followed by a host of studies aiming to identify the effects of exercise on the gut microbiota (19, 58–83). The majority of these studies have investigated the effect of exercise on the gut microbiota in rodents (57–75), though some have studied humans in intervention trials (18, 76–78, 84) and in cross-sectional or observational comparisons of athletes or active individuals and sedentary individuals (19, 79–83, 85, 86).
Results
The Effect of Exercise on the Gut Microbiome
Microbiota Features Affected by Exercise
Table 1 summarizes the studies listed above and their findings of the effect of exercise on the gut microbiota.
Although there are similarities in microbial factors shown to be affected by exercise within the literature, directions of the effects are inconsistent, and some studies show contradictory results. For example, while some studies show a reduction in Firmicutes and/or an increase in Bacteroidetes as a result of exercise (58, 64, 66, 70, 73, 74, 81, 84), others show the opposite effect (19, 60–62, 68, 71, 81, 86), and others show no effect (69, 76, 82).
Findings on the effect of exercise on measures of diversity are also highly variable, some showing increases in α-diversity (19, 60, 64, 68, 70, 71, 75, 79, 86), some showing decreases (58, 59, 66), and others reporting no difference (18, 59, 63, 65, 69, 76–78, 82, 83). Brandt et al. (73) also found that exercise attenuated the decrease in α-diversity that occurred when mice were fed a high-fat diet.
Bacterial taxa commonly shown to respond to exercise training include Lactobacillus (typically increased) (58, 60, 62, 68), Bifidobacterium (typically increased) (58, 62, 76, 82), Proteobacteria (typically decreased) (58, 65, 66, 68, 76), Akkermansia (typically increased) (19, 75, 76, 79, 82), Streptococcus (variable effects) (61, 68, 69), Clostridium (variable effects) (58, 62, 63, 67, 77), Turicibacter (typically decreased) (59, 64), and Rikenellaceae (typically decreased) (63, 66, 69) as well as measures of α- and β-diversity (variable effects) (19, 58, 60, 64, 66, 68, 70, 76, 80).
However, in some studies, changes in taxa are dependent on other factors such as changes in weight, body fat, and blood glucose (64, 76). This suggests, that the associated metabolic effects of the exercise regime may be the proximal cause, while exercise is the ultimate cause. Related variables, SCFA production and butyrate-producing taxa, have been consistently shown to increase in response to exercise (18, 57, 79) and have also been positively correlated to changes in lean muscle mass, also suggesting that SCFAs may play an important role in mediating the effects of exercise and the gut microbiome on host response (18). Table 2 summarizes additional effects of exercise on microbial metabolites, host health, and dietary interactions.
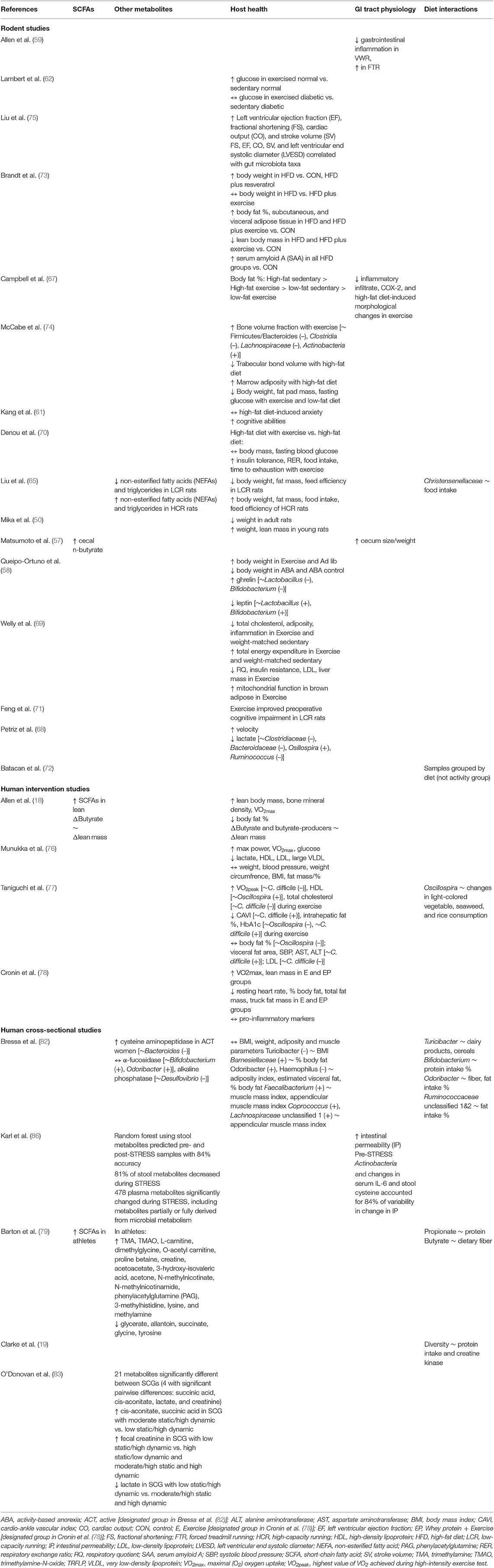
Table 2. Summary of effect of exercise on the microbial metabolites, host health, and dietary interactions.
Potential Causes of Discrepancies Between Studies
Potential reasons for the disparate results of these studies include study design factors as well as analytic methods (Figure 2). Study design factors include the choice of model (e.g., humans, mice, rats), the strain of mouse/rat (e.g., C57BL/6J, Zucker, Wistar, Sprague Dawley, etc.) (87), choice of diet (72), health or disease status (75, 81), age (66), gender, and the mode, duration, and intensity of training (59, 63, 72, 83, 84) as well as analytic methods such as DNA extraction and PCR primer biases (88–90), choice of microbiome sequencing methods (e.g., 16S rRNA gene sequencing, qPCR, metagenomics, etc.) (64), bioinformatic pipelines (90), and choice of diversity metrics (e.g., Shannon, Chao1, Simpson, etc.). For example, mouse models often use forced treadmill running or voluntary wheel running as modes of training. However, forced treadmill exercise often uses aversive motivation, such as shocks, which could induce negative stress responses (91–93) that may also affect intestinal permeability and the gut microbiome (59, 94, 95). In humans, exercise or sport is a broad term that can apply to a wide range of modes, durations, and intensities of activity. O'Donovan et al. (83) attempted to determine differential effects of different modes of exercise on the gut metagenome by doing a cross-sectional analysis of professional athletes from different sports with varying degrees of static and dynamic components. In this analysis, O'Donovan etal. found some differences in bacterial taxa and metabolites between sports classification groups (SCGs) that did not correlate with any other metadata (e.g., diet, sex, etc.) (83).
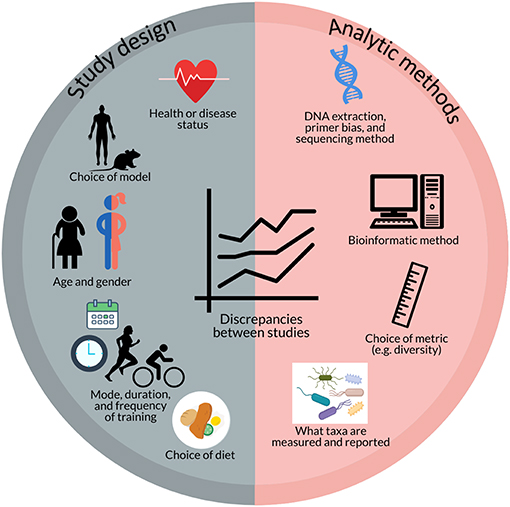
Figure 2. Potential factors contributing to discrepancies between studies investigating the effect of exercise on the gut microbiome include aspects of study design (e.g., health or disease status; choice of model; age and gender; mode, duration, and frequency of training; and choice of diet) and analytic methods (e.g., DNA extraction, primer bias, and sequencing method; bioinformatic method; choice of metrics; and what taxa are measured and reported).
In addition to differences in how results are obtained or measured, there is also a great deal of heterogeneity in what results are measured, or reported, that make it difficult to determine the full extent of variability in response between studies. In order to gain better insight into the potential effects and pathways by which exercise exerts its effect on the gut microbiome, it would be beneficial for studies to report effects on at least a certain standard set of microbiota variables that have already been shown to be relevant by multiple studies such as Firmicutes, Bacteroidetes, Lactobacillus, Bifidobacterium, Akkermansia, Clostridium, and Proteobacteria as well as diversity (though a standard metric has yet to be determined), butyrate-producing taxa (96), and SCFA production, even if the result is no change/difference. Munukka et al. (76) reported a lack of consistent effects due to inter-individual variability in response of the gut microbiota to exercise. This too is an important finding that should be reported and explored to determine factors that contribute to this variability in response and whether these differences in microbial response translate to differences in physiological response. These reporting standards would allow for better comparison between studies and potentially enable researchers to determine how different methods impact the results and elucidate factors that may contribute to variability in response.
Effect of Dietary Components Relevant to Exercise Nutrition on the Gut Microbiome
Confounding Effects of Diet
Diet is also a major factor that influences and shapes the gut microbiome (15–17). Kang et al. report that diet and exercise both cause shifts in the gut microbiome but that these changes are orthogonal (61). However, some of the studies above reported that dietary factors influenced the gut microbiota independently of, or in combination with, exercise. Dietary factors found in the studies presented here to associate with gut microbiome differences or changes include dairy products (82), light-colored vegetables (77), seaweed (77), rice (77), cereals (82), sucrose (76), fiber (76, 79, 82), protein intake (19, 79, 82), fat intake (82), and total food intake (65, 76) (Figure 3). Some differences or changes in the gut microbiota that seem to be associated with exercise may therefore be due to differences or changes in dietary intake, especially plants and carbohydrates, rather than exercise itself. There is therefore a need for studies investigating the link between the gut microbiome and exercise that control and standardize the dietary intake of participants.
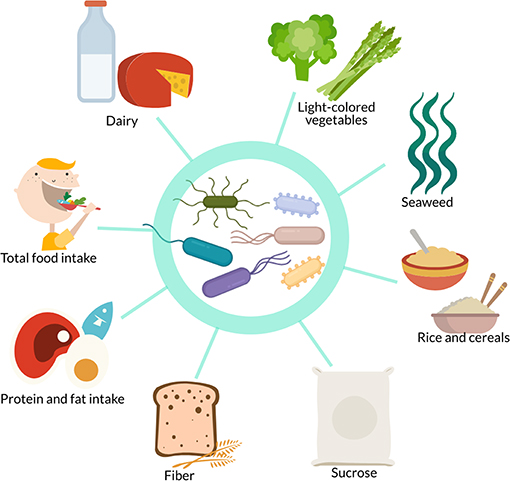
Figure 3. In studies investigating the effect of exercise on the gut microbiome, confounding dietary factors include dairy, light-colored vegetables, seaweed, rice, cereals, sucrose, fiber, protein intake, fat intake, and total food intake.
Effects of Supplements and Dietary Patterns on the Gut Microbiome
Although studies have shown some dietary interactions with the gut microbiome in athletes, it is unclear the extent to which the gut microbiome might be affected by supplements or dietary patterns commonly used by athletes, and the potential effects of this on the host. A review by Kårlund et al. (97) comprehensively discusses the topic of protein supplementation in athletes and the potential unknown effects on the gut microbiome. Excess protein may be fermented in the large intestine by various species from the genera Clostridium, Bacteroides, and others from the Proteobacteria phylum (98, 99), resulting in end products such as ammonia, amines, phenols, and sulfides as well as some SCFAs that may have systemic and metabolic effects on the host (100, 101). Different protein types have been shown to have differential effects on the gut microbiome (102–104) and plant-based vs. animal-based diets have also been shown to induce differences in the gut microbiome composition in humans (16). Additionally, different protein types have been assessed in the context of the anabolic response to exercise (105). However, there are no studies evaluating the impact of different types of protein supplements or whole-food protein sources on the gut microbiome and amino acid fermentation in athletes (97). As protein and protein supplements are widely advertised and recommended to athletes, this is an important gap in the research that should be addressed. Future research should also be sure to compare the effects of different protein sources as both isolated supplements as well as in their whole-food form as the matrix of the whole food has been shown to play an important role in the anabolic response to exercise and may alter effects based on factors such as the type and amount of fat (106, 107). Additionally, it would be interesting to know whether supplementation of plant-based proteins with amino acids such as leucine, lysine, and methionine, which is a strategy that has been shown by a couple studies to augment the anabolic effect of plant proteins (105), alters the effect of these proteins on the gut microbiome.
Carbohydrate is a primary fuel source for exercise and is therefore also a primary focus of athletes' dietary intake (108, 109). In addition to whole food forms of carbohydrates, such as bread, pasta, fruit, and potatoes, there is also a wide array of carbohydrate supplements that may be used before, during, or after exercise to enhance performance and recovery (110). The effects of whole food carbohydrates on the gut microbiome differs widely as a function of fiber content and type (111–113), though generally fiber tends to increase SCFA producing bacteria such as Bacteroidetes and Actinobacteria and decrease Firmicutes (114). However, the effects of frequent use of carbohydrate supplements, which are typically high in sugar and low in microbiota-accessible carbohydrates, on the gut microbiome is unknown. It is may therefore be of interest to develop carbohydrate supplements that also target the gut microbiome.
Though carbohydrates and protein are made the primary focus of athlete nutrition, fat is also an important fuel source during prolonged exercise and the popularity of high-fat diets such as the ketogenic diet has prompted athletes and scientists to investigate its potential for sports performance (115). However, evidence suggests that a high-fat diet does not improve exercise performance more than or as much as a high-carbohydrate diet (116). Additionally, the lack of microbiota-accessible carbohydrates on the ketogenic diet makes it questionable whether or how it would benefit the gut microbiome, though there has been little research in this area and none of it has been in athletes (117, 118).
Caffeine is also a widely used ergogenic aid among athletes. Coffee, one of the primary dietary sources of caffeine, has been linked to increases in Bifidobacterium and protection against high-fat diet-induced decreases in Lactobacillus, though these effects may be due to other bioactive compounds present in coffee such as chlorogenic acid (119). The effect of these aspects of dietary intake on the gut microbiome in athletes is only one half of the story. The other is the effect of the gut microbiome on the overall response, in terms of performance and training adaptation, of the athlete.
Personalized Sports Nutrition and the Potential Effect of the Gut Microbiome on Response to Diet and Exercise
The Gut Microbiome in Personalized Sports Nutrition
As discussed in Hughes et al. (22, 23), the gut microbiome is a potential predictor of response to diet. However, that review focused on predictors of response relevant to general health and prevention of chronic disease. Here, the evidence that the gut microbiome may be a predictor of athletic performance is reviewed. Personalized sports nutrition has incorporated the type of sport or activity, the training status of the individual, the athlete's goals, the time of the competitive season, and the athlete's food preferences (120, 121) as well as biological traits such as genetic polymorphisms, RNA expression, and epigenetic modifications (28–30, 34–38) in the attempt to optimize athletic performance and response to training programs.
The gut microbiome should be incorporated into this system as it modulates metabolism of diet and dietary supplements, and therefore has the potential to contribute to variability in response. Inter-individual variability among athletes in response to dietary supplements, such as caffeine and antioxidants, has been attributed to genetic polymorphisms and baseline antioxidant concentrations (35, 122). However, the gut microbiome has been identified as an important factor in the bioavailability and metabolism of antioxidants (123–125) and may be involved in caffeine metabolism via mechanisms such as modulation of the expression of the N-acetyltransferase 2 (NAT2) gene (126). Variability in the gut microbiome has been linked to variability in serum carotenoid concentrations (127), which suggests that the gut microbiome does indeed play a role in modulating antioxidant metabolism. In short, the gut microbiome may affect the metabolism of dietary components, supplements, and dietary patterns marketed to and used by athletes, but this is an area of research that has not yet been adequately explored.
The Effect of the Gut Microbiome on Performance
Though the gut microbiome has been shown to modulate metabolism of relevant dietary components, as discussed above, the implications of this for performance are still unclear.
Mostly cross-sectional, studies have examined the correlation between measures of fitness, such as VO2max and VO2peak, and the gut microbiota (18, 77, 85, 128–130) (Table 3). Butyrate-producing bacteria have been shown in both Allen et al. (18) and Estaki et al. (129) to correlate positively with VO2max and VO2peak, respectively. Bacteroides and the Firmicutes/Bacteroidetes ratio have also been shown to correlate with VO2max (85, 128, 130), although studies have shown contrasting results. Durk et al. (128) found the Firmicutes/Bacteroidetes ratio to correlate positively with VO2max. Conversely, Yu et al. (85) found a lower F/B ratio in elderly adults with higher exercise capacity and Yang et al. (130) found that the high VO2max group had lower Eubacterium rectale-Clostridium coccoides (Erec), which are members of the Firmicutes phylum, and higher Bacteroides. Yu et al. (85) also identified several other taxa that were correlated with VO2peak in their elderly population such as Lactobacillales, Blautia, Ruminococcus, E. coli, and Alcaligenaceae. Taniguchi et al. (77) found an inverse correlation between Clostridium difficile and changes in VO2peak in elderly Japanese men during a cycling intervention.
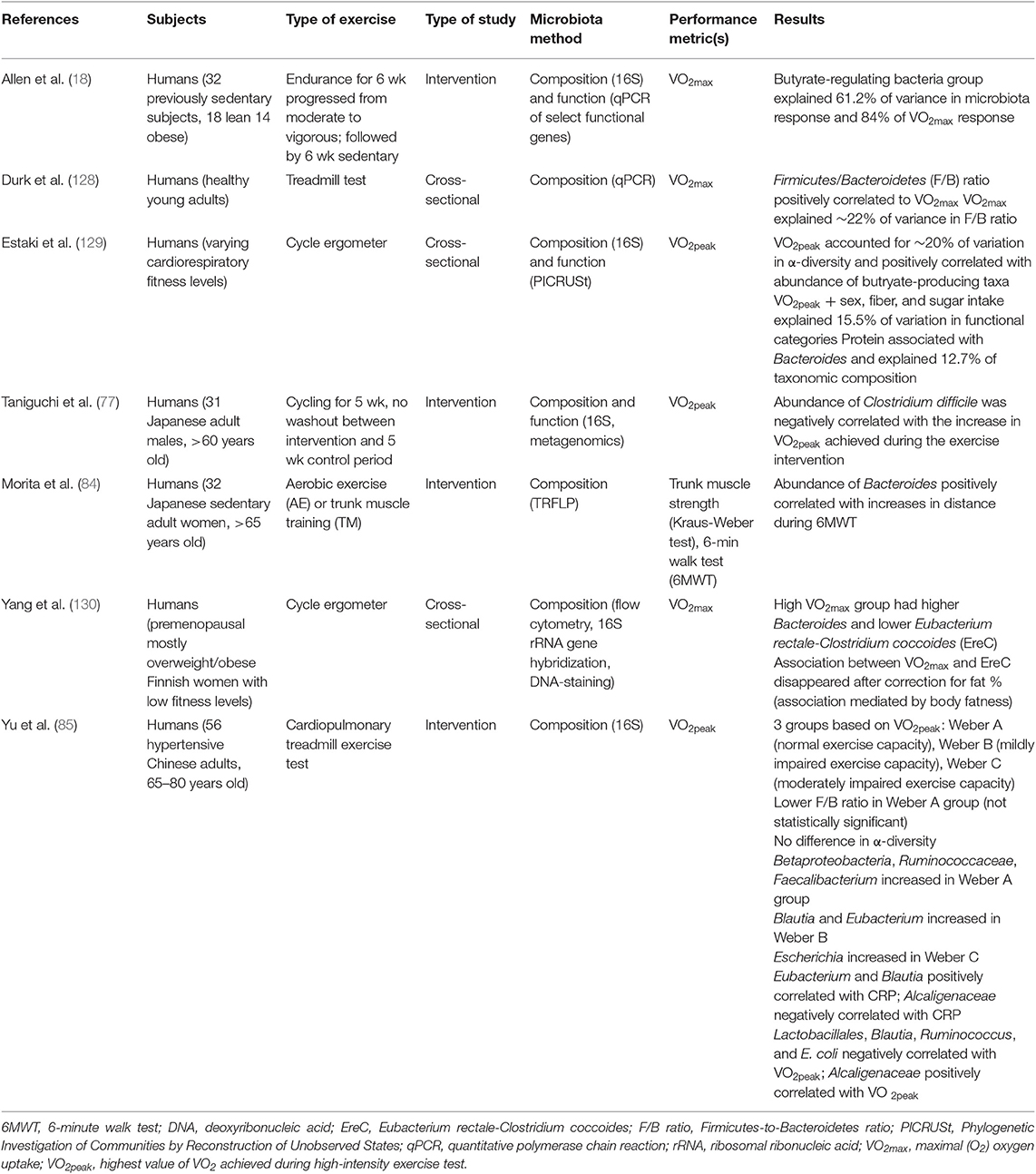
Table 3. Summary of studies investigating the correlation between gut microbiota composition and measures of fitness.
Few studies have directly investigated the effect of the gut microbiome on athletic performance (Table 4). Hsu et al. (131) and Huang et al. (132) both used germ free (GF) mice (C57BL/6JNarl) and compared these to mice colonized with bacteria to determine potential effects of the presence of the microbiome as well as specific bacteria on physical performance. Specific pathogen free (SPF) mice were found to have the highest exercise capacity and germ-free mice the lowest (131, 132). Mice colonized with individual bacterial taxa showed improvements in exercise capacity compared to their GF counterparts (131), though not all bacteria showed the same degree of impact (132). Hsu et al. (131), compared germ free (GF) mice, gnotobiotic mice colonized with Bacteroides fragilis (BF), and specific pathogen free (SPF) mice in a test of endurance swimming. In a similar study, Huang et al. (132) compared germ-free mice to gnotobiotic mice monocolonized with either Eubacterium rectale, Clostridium coccoides, or Lactobacillus plantarum TWK10 on performance in a swim-to-exhaustion test. In Hsu et al. (131), swim-to-exhaustion time was significantly different among all groups, with SPF mice having the greatest endurance, followed by BF mice, with GF mice having the least endurance capacity. In Huang et al. (132), gnotobiotic mice colonized with E. rectale showed significantly higher performance, both with and without aerobic training, than the GF mice as well as the mice colonized with C. coccoides and L. plantarum.
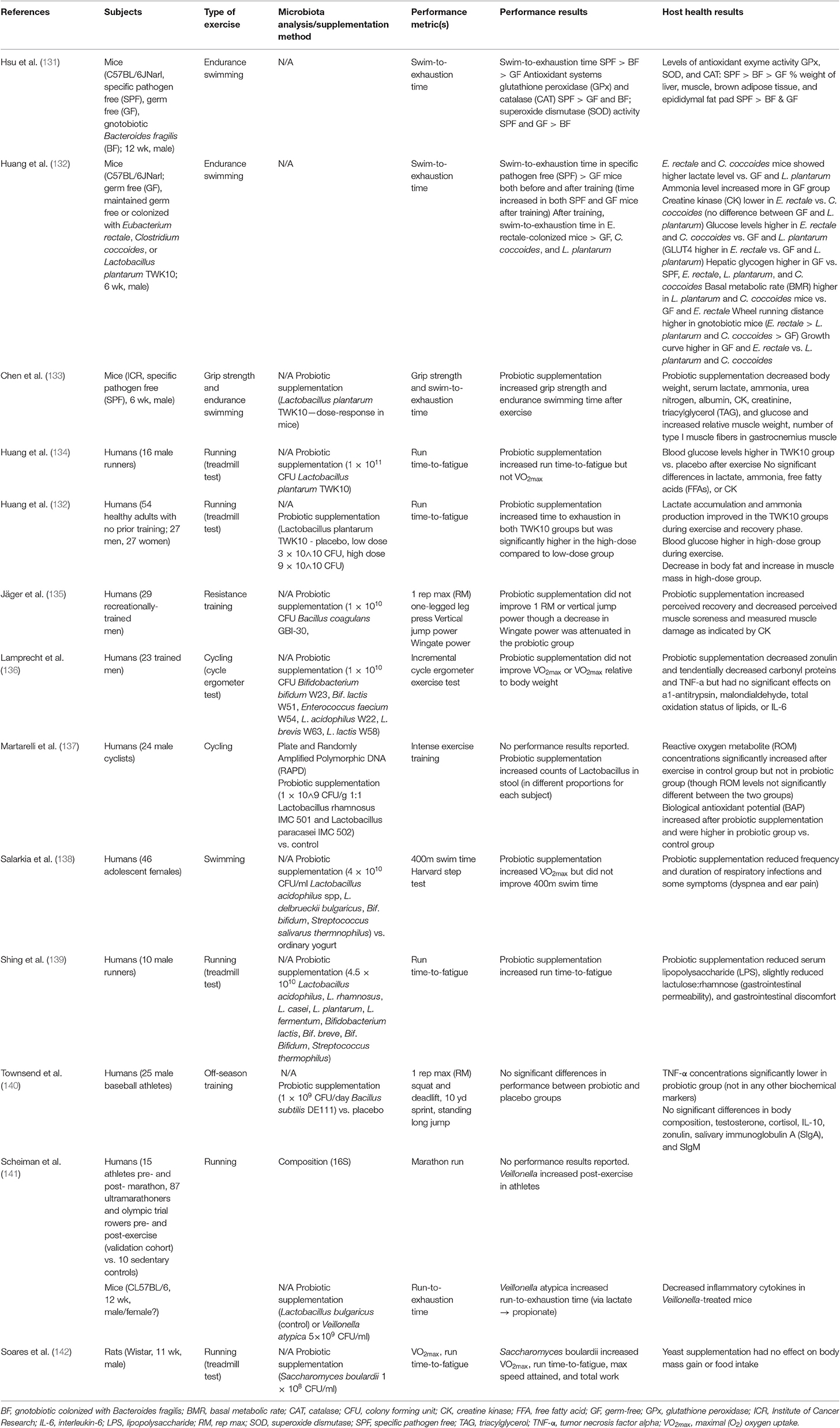
Table 4. Summary of studies investigating the effect of the gut microbiota or probiotic supplementation on exercise performance.
The potential mechanisms of these effects differed between the two studies. Differences in endurance capacity in Hsu et al. were accompanied by differences in antioxidant enzyme systems, with SPF mice showing greater serum and hepatic antioxidant enzyme activity, and physiological metrics, such as weight of muscle and brown adipose tissue (131). The gut microbiome modulates adipose tissue thermogenic pathways, including browning of white adipose and activity of brown adipose, via potential mechanisms such as bile acids and the endocannabinoid system (143). The gut microbiome may also modulate skeletal muscle anabolism and function via SCFA production and alteration of the availability of intramuscular fuels (55). Mice colonized with E. rectale and C. coccoides in Huang et al. showed higher lactate levels and higher glucose levels while mice colonized with E. rectale showed a lower creatine kinase (CK), a marker of muscular stress, and higher wheel running distance compared to both GF mice and the other gnotobiotic mice (132).
It is worth noting, however, in Huang et al. (132) that L. plantarum and C. coccoides did not colonize stably in the mice, fecal analysis showed no significant increases of these microbes, while E. rectale did colonize and increase over time. Therefore, it is unclear whether the ergogenic effect was due to the presence of E. rectale specifically, or simply due to the successful colonization by a microbe. These studies suggest that the gut microbiome may influence performance. They also indicate that a more diverse microbiome may be more beneficial as SPF mice performed better than monocolonized BF mice (131). While Huang et al. (132) suggests that individual taxa such as E. rectale may be partially responsible for performance effects, further research is needed to determine exactly what aspects or taxa contribute to this ergogenic effect. These studies also did not investigate responses to a dietary or training regimen, leaving room for further research on the potential of the gut microbiome to mediate or modify exercise performance response to diet.
The Effect of Probiotic Supplementation on Athlete Health and Performance
While there are a number of studies on probiotic supplementation in animals and human athletes, most focus on effects such as frequency of respiratory and gastrointestinal illness or biomarkers of inflammation and immune function (137, 144–146). Supplementation of probiotic bacteria to boost the abundance or activity of potentially beneficial taxa may also serve as a potential method of modifying performance response to training. Our review of the literature found eleven studies investigating the ergogenic effect of probiotic supplementation (133–142, 147) (Table 4). Common probiotic bacteria used were strains of Lactobacillus or Bifidobacterium (133, 134, 136–139, 147). Additional strains tested included those belonging to species Bacillus subtillis (140) or Bacillus coagulans (135), Veillonella atypica (141), or even yeast Saccharomyces boulardii (142).
The majority of studies investigated the effect of probiotic supplementation on aerobic exercise performance measures such as run time-to-fatigue, VO2max, max speed attained, 10-yard sprint, or 400-meter swim time (132–134, 136, 138, 139, 141, 142). However, some studies also investigated strength and anaerobic outcomes such as grip strength, vertical jump power, standing long jump, Wingate power, or 1 rep max (RM) lifts (133, 135, 140).
Effects on performance variables were highly mixed between studies, though a number of studies found beneficial effects on performance parameters such as time-to-fatigue (132–134, 139, 141, 142). However, some studies found no effects of probiotic supplementation on performance metrics (136, 140) while other studies found mixed effects, with probiotic supplementation improving some performance measures, but not others (134, 135, 138). For example, Huang et al. (134) found that probiotic supplementation with Lactobacillus plantarum TWK10 increased run time-to-fatigue but not VO2max. Thus, studies reporting effects of probiotic supplementation on only one performance outcome may not be providing a complete picture of the ergogenicity of probiotic bacteria. Additionally, all but one study (137) of probiotic supplementation of humans lacked confirmation of probiotic colonization and this study acknowledged that individuals showed different levels of colonization by the probiotic bacteria. It is important that future studies investigating probiotic supplementation also collect fecal samples from participants before and after the intervention to determine whether differences in probiotic colonization may contribute to inter-individual variability in the ergogenic effect of probiotic supplementation.
In addition to performance variables, many of these studies investigated effects on body composition and inflammation. Again, results were mixed, with some studies reporting significant effects of supplementation on outcomes such as fat mass and muscle mass (132, 133) or inflammatory markers (133, 137, 139, 141, 147), though results were often mixed with some biochemical markers showing no significant effect of probiotic treatment and some studies showing no significant effects at all on these outcomes (134, 142). However, as none of these variables were analyzed, further research is necessary to determine the mechanism of the effects as well as whether the same effects are seen in humans.
The Effect of Antibiotic Treatment on Exercise Performance
Conversely to the use of probiotics to determine the potential effect of the gut microbiome on athletic performance, the use of antibiotics in mouse models has recently been explored to determine the potential effects of a lack of gut microbes and their metabolites on exercise capacity and muscle function (148, 149). Table 5 displays the findings of these recent studies. In both studies, antibiotic treatment decreased the exercise capacity of the mice, tested using forced treadmill running. Additionally, this phenotype could be rescued by either natural reseeding (148) or acetate infusion (149). Nay et al. also found reduced gene expression of SCFA receptor G-protein coupled receptor 41 (GPR41) and sodium/glucose cotransporter 1 (SGLT1) as well as reduced muscle glycogen in antibiotic-treated mice, suggesting that the reduced exercise capacity in these mice may have been mediated by muscle glycogen availability (148). Okamoto et al. concluded that the reduced exercise capacity of antibiotic-treated mice was due to the lack of acetate available for use as a substrate during exercise as acetyl-CoA (149). With regards to changes in the gut microbial community, Okamoto et al. reported that relative abundance of Firmicutes was increased in antibiotic-treated mice while Bacteroidetes, α-diversity, and fecal bacterial DNA concentration was reduced (149). Nay et al. found that fecal bacterial DNA was reduced in antibiotic-treated mice but only reported differences in composition between control mice and mice treated with antibiotics but naturally reseeded, which showed no significant differences in α- and β-diversity, Bacteroides, and Firmicutes (148).
Okamoto et al. additionally tested the effect of a low microbiota-available carbohydrate diet (LMC) vs. a high microbiota-available carbohydrate (HMC) diet to determine with substrate availability for the gut microbiome altered exercise capacity. In these treatment groups, treadmill running time was decreased in the LMC mice, concomitant with a decrease in muscle mass, fecal SCFA, and plasma acetate and proprionate as well as an increase in Firmicutes and decrease in Bacteroidetes and other SCFA producing bacterial taxa (149). This reduced exercise capacity phenotype was rescued when mice were given a fecal microbiota transplant (FMT) from HMC mice and a dose of inulin prior to exercise. The increased exercise capacity in LMC+FMT+inulin mice was not accompanied by changes in body mass or muscle mass but there was an increase in fecal SCFAs, again suggesting that SCFA concentration may act as a direct substrate or mediate substrate availability in such a way as to influence exercise capacity.
Summary of Findings and Putative Mechanisms of the Effect of the Gut Microbiome on Athletic Performance
These studies suggest that there may be an effect of the gut microbiome on exercise performance via mechanisms such as SCFA availability, muscle glycogen content, antioxidant enzyme activity, gastrointestinal permeability, and lactate metabolism (Figure 4). Additional speculative mechanisms may involve alterations in substrate utilization (142) as well as glycogen metabolism and storage (150), changes in neural function (142), as well as immune modulation (142) or cross-talk between the gut microbiome and mitochondria in energy production and inflammation (45). The gut microbiota has been hypothesized to impact skeletal muscle physiology and function via metabolites such as SCFAs, folate, tryptophan, glycine betaine, vitamins B2 and B12, and urolithins that may act through various pathways such as stimulation of insulin-like growth factor-1 (IGF-1), prevention of oxidative stress or inflammation, and promotion of mitochondrial biogenesis (151). However, these data also indicate that improvements in these functions do not always translate to improvements in performance. Further research is needed to investigate the effects of different probiotic strains, the interaction with dietary composition (e.g., differences of effect in athletes who have different overall dietary patterns), the use of dietary supplements, and in different modes of exercise, such as strength training.
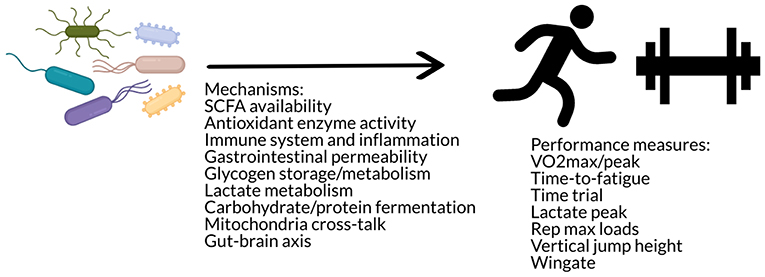
Figure 4. The gut microbiome may influence performance via mechanisms such as antioxidant enzyme activity, immune modulation, gastrointestinal permeability, substrate utilization and storage, mitochondria cross-talk, and/or the gut-brain axis.
Conclusions and Future Directions
The gut microbiome represents an open field of study in the realm of personalized sports nutrition. High interindividual variability in response to training and physical activity is regularly reported (152) and the gut microbiome may contribute to this variability by impacting individual metabolism of food components and/or adaptation to the homeostatic stress, or training load, of the exercise stimulus (153). More research is needed to determine whether the gut microbiome could be an important predictor of athletic performance in response to dietary and exercise interventions. Researchers should refer to guides such as Ross et al. (36), Hecksteden et al. (152), Mann et al. (153), Swinton et al. (154), and Hopkins et al. (155) for statistical frameworks to interpret inter-individual variability in response and identify factors that contribute to this variability.
Specific questions that could be addressed are the role of specific bacterial taxa or groups of taxa involved in gains in athletic performance in response to certain dietary factors (e.g., protein sources such as whey, casein, soy, etc.; macronutrient distribution; or supplements such as caffeine, beta-alanine, antioxidants) or exercise stimuli. This could be investigated by using a combined dietary-exercise intervention, measuring both baseline and final microbiome and performance variables, and using predictive machine learning algorithsms such as random forests (156) to determine whether baseline abundance or changes in certain bacterial taxa can predict an individual's physical performance response. Another question is whether different taxa are involved in different responses (e.g., VO2max, time-to-fatigue, rep max loads, etc.) and the mechanisms of these effects (e.g., SCFA production, antioxidant enzyme activity, muscle protein synthesis, glycogen formation, energy harvest and fuel utilization, inflammation, etc.). While the first question could be addressed by a study measuring multiple exercise performance outcomes within the same population, determining the mechanisms of these effects would require in vitro or animal models and measurement of potential mediating metabolites, such as SCFAs, and physiological variables, such as muscle mass or muscle glycogen content. Furthermore, larger and longer studies are needed to address whether effects or responses differ between demographics (e.g., gender, age, ethnicity, etc.) and whether modulation of the gut microbiome via probiotics and/or prebiotics or modulation of the dietary or exercise stimulus (e.g., amount or type of supplement; mode, duration, intensity of exercise) may serve to increase positive response to the stimulus, decreasing the number of “non-responders.” For example, a study using the same participants and measuring microbiome and performance responses to stepwise increases in the duration and/or intensity of exercise may serve to elucidate what type of exercise may be optimal for certain individuals and their microbiomes. Additionally, studies that have measured changes in performance in response to probiotic supplementation have not looked at individual's gut microbiota composition directly. This is a limitation of these studies as different strains of probiotic bacteria show differing rates of survival through the gastrointestinal tract (157) and the composition of an individual's gut microbiota may also influence the persistence and function of probiotic bacteria in the gut (158–160). Therefore, not all probiotic strains may survive in sufficient quantities to make it down to the gut microbiome and, even if the probiotic bacteria reach the gut microbiome, it may not last as long or have the same effect in each individual.
Additional challenges and limitations in the area of research are numerous and must be taken into consideration when making claims about the exercise-microbiome connection. While the effects of diet and exercise have been shown to be orthogonal to one another (61), diet can still be a confounding factor within and between studies. Thus, conclusive effects of exercise on the gut microbiome must standardize the diet of participants, which has not yet been done. In addition to diet, variables such as genetics (32, 161), epigenetics (162), sleep behavior (163, 164), gender (165, 166), age (66, 167), and a host of other factors contribute to variability in the gut microbiome as well as performance response. This variability makes it extremely difficult to draw concrete conclusions about the effects of the gut microbiome and should always be considered when designing or interpreting studies on the interaction of the gut microbiome and host.
A related body of research has developed investigating the “gut-muscle axis” as it relates to age-related changes in muscle mass (i.e., sarcopenia) and physical frailty (151, 168–174) as well as its potential role in the “muscle-gut-brain” axis and neurodegenerative diseases in aging (175, 176). This field of research has the potential to inform the research in the field of the gut microbiome and exercise performance. Though this research focuses on preservation of muscle mass rather than physical or athletic performance, it is extremely relevant to identifying the pathways that connect these systems and how they can be modulated. Taxa, such as Faecalibacterium prausnitzii (151), or supplementation with prebiotics (177), butyrate (178), or other microbial metabolites such as urolithin A (179, 180) have shown beneficial associations or effects on muscle function and protection against aging-related atrophy. It has also been postulated that the aging gut microbiome may play a role in the phenomenon of anabolic resistance, not by altering protein metabolism per se, but by mechanisms such as gut barrier function, inflammation, and mitochondrial dysfunction (168, 170). Thus, by looking at how age-related changes in the gut microbiome may contribute to sarcopenia and decreases in muscle function, we may better understand how to modify or supplement this community to both maintain health as well as potentially increase performance.
In conclusion, there are several different fields of research that have touched on the question of the role of the gut microbiome in exercise and athletic performance. However, there are many gaps and limitations in the research thus far that must still be addressed. While there have not yet been any conclusive findings, further research and collaboration among disciplines may help shed light on the connection between exercise and the gut microbiome and the potential implications on athletic performance.
Author Contributions
RH performed the literature review, conceived, and composed the manuscript.
Funding
Partial funding for this manuscript was provided by the UC Davis Open Access Fund.
Conflict of Interest
The author declares that the research was conducted in the absence of any commercial or financial relationships that could be construed as a potential conflict of interest.
Acknowledgments
Thank you to James P. Hughes and Nancy L. Keim who assisted in proofing this manuscript. Figures were generated using Piktochart.
References
1. Tremaroli V, Backhed F. Functional interactions between the gut microbiota and host metabolism. Nature. (2012) 489:242–9. doi: 10.1038/nature11552
2. Nicholson JK, Holmes E, Kinross J, Burcelin R, Gibson G, Jia W, et al. Host-gut microbiota metabolic interactions. Science. (2012) 336:1262–7. doi: 10.1126/science.1223813
3. Turnbaugh PJ, Ley RE, Mahowald MA, Magrini V, Mardis ER, Gordon JI. An obesity-associated gut microbiome with increased capacity for energy harvest. Nature. (2006) 444:1027–31. doi: 10.1038/nature05414
4. Musso G, Gambino R, Cassader M. Interactions between gut microbiota and host metabolism predisposing to obesity and diabetes. Annu Rev Med. (2011) 62:361–80. doi: 10.1146/annurev-med-012510-175505
5. de Groot PF, Belzer C, Aydin O, Levin E, Levels JH, Aalvink S, et al. Distinct fecal and oral microbiota composition in human type 1 diabetes, an observational study. PLoS ONE. (2017) 12:e0188475. doi: 10.1371/journal.pone.0188475
6. Qin J, Li Y, Cai Z, Li S, Zhu J, Zhang F, et al. A metagenome-wide association study of gut microbiota in type 2 diabetes. Nature. (2012) 490:55–60. doi: 10.1038/nature11450
7. Kau AL, Ahern PP, Griffin NW, Goodman AL, Gordon JI. Human nutrition, the gut microbiome and the immune system. Nature. (2011) 474:327–36. doi: 10.1038/nature10213
8. Hooper LV, Littman DR, Macpherson AJ. Interactions between the microbiota and the immune system. Science. (2012) 336:1268–73. doi: 10.1126/science.1223490
9. Dinan TG, Cryan JF. The microbiome-gut-brain axis in health and disease. Gastroenterol Clin North Am. (2017) 46:77–89. doi: 10.1016/j.gtc.2016.09.007
10. Cryan JF, Dinan TG. Mind-altering microorganisms: the impact of the gut microbiota on brain and behaviour. Nat Rev Neurosci. (2012) 13:701–12. doi: 10.1038/nrn3346
11. Lamichhane S, Sen P, Dickens AM, Oresic M, Bertram HC. Gut metabolome meets microbiome: a methodological perspective to understand the relationship between host and microbe. Methods. (2018) 149:3–12. doi: 10.1016/j.ymeth.2018.04.029
12. Tolhurst G, Heffron H, Lam YS, Parker HE, Habib AM, Diakogiannaki E, et al. Short-chain fatty acids stimulate glucagon-like peptide-1 secretion via the G-protein–coupled receptor FFAR2. Diabetes. (2012) 61:364–71. doi: 10.2337/db11-1019
13. Canfora EE, Jocken JW, Blaak EE. Short-chain fatty acids in control of body weight and insulin sensitivity. Nat Rev Endocrinol. (2015) 11:577–91. doi: 10.1038/nrendo.2015.128
14. Turnbaugh PJ, Ley RE, Hamady M, Fraser-Liggett CM, Knight R, Gordon JI. The human microbiome project. Nature. (2007) 449:804–10. doi: 10.1038/nature06244
15. De Filippo C, Cavalieri D, Di Paola M, Ramazzotti M, Poullet JB, Massart S, et al. Impact of diet in shaping gut microbiota revealed by a comparative study in children from Europe and rural Africa. Proc Natl Acad Sci USA. (2010) 107:14691–6. doi: 10.1073/pnas.1005963107
16. David LA, Maurice CF, Carmody RN, Gootenberg DB, Button JE, Wolfe BE, et al. Diet rapidly and reproducibly alters the human gut microbiome. Nature. (2014) 505:559–63. doi: 10.1038/nature12820
17. Wu GD, Chen J, Hoffmann C, Bittinger K, Chen YY, Keilbaugh SA, et al. Linking long-term dietary patterns with gut microbial enterotypes. Science. (2011) 334:105–8. doi: 10.1126/science.1208344
18. Allen JM, Mailing LJ, Niemiro GM, Moore R, Cook MD, White BA, et al. Exercise alters gut microbiota composition and function in lean and obese humans. Med Sci Sports Exerc. (2018) 50:747–57. doi: 10.1249/MSS.0000000000001495
19. Clarke SF, Murphy EF, O'Sullivan O, Lucey AJ, Humphreys M, Hogan A, et al. Exercise and associated dietary extremes impact on gut microbial diversity. Gut. (2014) 63:1913–20. doi: 10.1136/gutjnl-2013-306541
20. Yatsunenko T, Rey FE, Manary MJ, Trehan I, Dominguez-Bello MG, Contreras M, et al. Human gut microbiome viewed across age and geography. Nature. (2012) 486:222–7. doi: 10.1038/nature11053
21. Koppel N, Balskus EP. Exploring and understanding the biochemical diversity of the human microbiota. Cell Chem Biol. (2016) 23:18–30. doi: 10.1016/j.chembiol.2015.12.008
22. Hughes RL, Kable ME, Marco M, Keim NL. The role of the gut microbiome in predicting response to diet and the development of precision nutrition models. Part II: results. Adv Nutr. (2019) 10:979–98. doi: 10.1093/advances/nmz049
23. Hughes RL, Marco ML, Hughes JP, Keim NL, Kable ME. The role of the gut microbiome in predicting response to diet and the development of precision nutrition models—Part I: overview of current methods. Adv Nutr. (2019) 10:953–78. doi: 10.1093/advances/nmz022
24. Timmons JA. Variability in training-induced skeletal muscle adaptation. J Appl Physiol. (2011) 110:846–53. doi: 10.1152/japplphysiol.00934.2010
25. Thomas D, Erdman K, Burke L American College of Sports Medicine Joint Position Statement. Nutrition and athletic performance. Med Sci Sport Exerc. (2016) 48:543–68. doi: 10.1249/MSS.0000000000000852
26. Borresen J, Lambert MI. The quantification of training load, the training response and the effect on performance. Sports Med. (2009) 39:779–95. doi: 10.2165/11317780-000000000-00000
27. Pickering C, Kiely J. Exercise response efficiency: a novel way to enhance population health? Lifestyle Genom. (2019) 11:129–35. doi: 10.1159/000501206.
28. Jones N, Kiely J, Suraci B, Collins D, De Lorenzo D, Pickering C, et al. A genetic-based algorithm for personalized resistance training. Biol Sport. (2016) 33:117. doi: 10.5604/20831862.1198210
29. Pickering C, Kiely J, Suraci B, Collins D. The magnitude of Yo-Yo test improvements following an aerobic training intervention are associated with total genotype score. PloS ONE. (2018) 13:e0207597. doi: 10.1371/journal.pone.0207597
30. Guest NS, Horne J, Vanderhout SM, El-Sohemy A. Sport nutrigenomics: personalized nutrition for athletic performance. Front Nutr. (2019) 6:8. doi: 10.3389/fnut.2019.00008
31. Bouchard C, An P, Rice T, Skinner JS, Wilmore JH, Gagnon J, Pérusse L, et al. Familial aggregation of Vo2 max response to exercise training: results from the HERITAGE Family Study. J Appl Physiol. (1999) 87:1003–8. doi: 10.1152/jappl.1999.87.3.1003
32. Ahmetov II, Egorova ES, Gabdrakhmanova LJ, Fedotovskaya ON. Genes and athletic performance: an update. Med Sport Sci. (2016) 61:41–54. doi: 10.1159/000445240
33. Bray MS, Hagberg JM, Perusse L, Rankinen T, Roth SM, Wolfarth B, et al. The human gene map for performance and health-related fitness phenotypes: the 2006–2007 update. Med Sci Sport Exer. (2009) 41:34–72. doi: 10.1249/MSS.0b013e3181844179
34. Timmons JA, Knudsen S, Rankinen T, Koch LG, Sarzynski M, Jensen T, et al. Using molecular classification to predict gains in maximal aerobic capacity following endurance exercise training in humans. J Appl Physiol. (2010) 108:1487–96. doi: 10.1152/japplphysiol.01295.2009
35. Pickering C, Kiely J. Are the current guidelines on caffeine use in sport optimal for everyone? Inter-individual variation in caffeine ergogenicity, and a move towards personalised sports nutrition. Sports Med. (2018) 48:7–16. doi: 10.1007/s40279-017-0776-1
36. Ross R, Goodpaster BH, Koch LG, Sarzynski MA, Kohrt WM, Johannsen NM, et al. Precision exercise medicine: understanding exercise response variability. Br J Sports Med. (2019) 53:1141–53. doi: 10.1136/bjsports-2018-100328
37. Ramos-Lopez O, Riezu-Boj JI, Milagro FI, Cuervo M, Goni L, Martinez JA. Genetic and nongenetic factors explaining metabolically healthy and unhealthy phenotypes in participants with excessive adiposity: relevance for personalized nutrition. Ther Adv Endocrinol. (2019) 10:2042018819877303. doi: 10.1177/2042018819877303
38. Danaher J. Metabolic Mechanisms of the Fat Mass and Obesity-Associated (FTO) Gene. Melbourne, VIC: Victoria University (2016).
39. Cook MD, Allen JM, Pence BD, Wallig MA, Gaskins HR, White BA, et al. Exercise and gut immune function: evidence of alterations in colon immune cell homeostasis and microbiome characteristics with exercise training. Immunol Cell Biol. (2016) 94:158–63. doi: 10.1038/icb.2015.108
40. Cronin O, Molloy MG, Shanahan F. Exercise, fitness, and the gut. Curr Opin Gastroenterol. (2016) 32:67–73. doi: 10.1097/MOG.0000000000000240
41. Cronin O, O'Sullivan O, Barton W, Cotter PD, Molloy MG, Shanahan F. Gut microbiota: implications for sports and exercise medicine. Br J Sports Med. (2017) 51:700–1. doi: 10.1136/bjsports-2016-097225
42. Bermon S, Petriz B, Kajeniene A, Prestes J, Castell L, Franco OL. The microbiota: an exercise immunology perspective. Exerc Immunol Rev. (2015) 21:70–9. Available online at: https://www.researchgate.net/profile/Bernardo_Petriz/publication/272480734_The_Microbiota_an_Exercise_Immunology_Perspective/links/54ee51a00cf2e55866f2871a.pdf
43. Cerdá B, Pérez M, Pérez-Santiago JD, Tornero-Aguilera JF, González-Soltero R, Larrosa M. Gut microbiota modification: another piece in the puzzle of the benefits of physical exercise in health? Front Physiol. (2016) 7:51. doi: 10.3389/fphys.2016.00051
44. Chen J, Guo Y, Gui Y, Xu D. Physical exercise, gut, gut microbiota, and atherosclerotic cardiovascular diseases. Lipids Health Dis. (2018) 17:17. doi: 10.1186/s12944-017-0653-9
45. Clark A, Mach N. The crosstalk between the gut microbiota and mitochondria during exercise. Front Physiol. (2017) 8:319. doi: 10.3389/fphys.2017.00319
46. Codella R, Luzi L, Terruzzi I. Exercise has the guts: how physical activity may positively modulate gut microbiota in chronic and immune-based diseases. Dig Liver Dis. (2018) 50:331–41. doi: 10.1016/j.dld.2017.11.016
47. Hamasaki H. Exercise and gut microbiota: clinical implications for the feasibility of Tai Chi. J Integr Med. (2017) 15:270–81. doi: 10.1016/S2095-4964(17)60342-X
48. Mach N, Fuster-Botella D. Endurance exercise and gut microbiota: a review. J Sport Health Sci. (2017) 6:179–97. doi: 10.1016/j.jshs.2016.05.001
49. Mailing LJ, Allen JM, Buford TW, Fields CJ, Woods JA. Exercise and the gut microbiome: a review of the evidence, potential mechanisms, and implications for human health. Exerc Sport Sci Rev. (2019) 47:75–85. doi: 10.1249/JES.0000000000000183
50. Mika A, Fleshner M. Early-life exercise may promote lasting brain and metabolic health through gut bacterial metabolites. Immunol Cell Biol. (2016) 94:151. doi: 10.1038/icb.2015.113
51. O'Sullivan O, Cronin O, Clarke SF, Murphy EF, Molloy MG, Shanahan F, et al. Exercise and the microbiota. Gut Microbes. (2015) 6:131–6. doi: 10.1080/19490976.2015.1011875
52. Rankin A, O'Donovan C, Madigan SM, O'Sullivan O, Cotter PD. 'Microbes in sport' -the potential role of the gut microbiota in athlete health and performance. Br J Sports Med. (2017) 51:698–9. doi: 10.1136/bjsports-2016-097227
53. Monda V, Villano I, Messina A, Valenzano A, Esposito T, Moscatelli F, et al. Exercise modifies the gut microbiota with positive health effects. Oxid Med Cell Longev. (2017) 2017:3831972. doi: 10.1155/2017/3831972
54. Sohail MU, Yassine HM, Sohail A, Al Thani AA. Impact of physical exercise on gut microbiome, inflammation, and the pathobiology of metabolic disorders. Rev Diabet Stud. (2019) 15:35–48. doi: 10.1900/RDS.2019.15.35
55. Hawley JA. Microbiota and muscle highway—two way traffic. Nat Rev Endocrinol. (2019). doi: 10.1038/s41574-019-0291-6. [Epub ahead of print].
56. Dalton A, Mermier C, Zuhl M. Exercise influence on the microbiome–gut–brain axis. Gut Microbes. (2019) 10:555–68. doi: 10.1080/19490976.2018.1562268
57. Matsumoto M, Inoue R, Tsukahara T, Ushida K, Chiji H, Matsubara N, et al. Voluntary running exercise alters microbiota composition and increases n-butyrate concentration in the rat cecum. Biosci Biotechnol Biochem. (2008) 72:572–6. doi: 10.1271/bbb.70474
58. Queipo-Ortuno MI, Seoane LM, Murri M, Pardo M, Gomez-Zumaquero JM, Cardona F, et al. Gut microbiota composition in male rat models under different nutritional status and physical activity and its association with serum leptin and ghrelin levels. PLoS ONE. (2013) 8:e65465. doi: 10.1371/journal.pone.0065465
59. Allen JM, Berg Miller ME, Pence BD, Whitlock K, Nehra V, Gaskins HR, et al. Voluntary and forced exercise differentially alters the gut microbiome in C57BL/6J mice. J Appl Physiol. (2015) 118:1059–66. doi: 10.1152/japplphysiol.01077.2014
60. Choi JJ, Eum SY, Rampersaud E, Daunert S, Abreu MT, Toborek M. Exercise attenuates PCB-induced changes in the mouse gut microbiome. Environ Health Perspect. (2013) 121:725–30. doi: 10.1289/ehp.1306534
61. Kang SS, Jeraldo PR, Kurti A, Miller ME, Cook MD, Whitlock K, et al. Diet and exercise orthogonally alter the gut microbiome and reveal independent associations with anxiety and cognition. Mol Neurodegen. (2014) 9:36. doi: 10.1186/1750-1326-9-36
62. Lambert JE, Myslicki JP, Bomhof MR, Belke DD, Shearer J, Reimer RA. Exercise training modifies gut microbiota in normal and diabetic mice. Appl Physiol Nutr Metab. (2015) 40:749–52. doi: 10.1139/apnm-2014-0452
63. Lamoureux EV, Grandy SA, Langille MGI. Moderate exercise has limited but distinguishable effects on the mouse microbiome. mSystems. (2017) 2:e00006-17. doi: 10.1128/mSystems.00006-17
64. Evans CC, LePard KJ, Kwak JW, Stancukas MC, Laskowski S, Dougherty J, et al. Exercise prevents weight gain and alters the gut microbiota in a mouse model of high fat diet-induced obesity. PLoS ONE. (2014) 9:e92193. doi: 10.1371/journal.pone.0092193
65. Liu TW, Park YM, Holscher HD, Padilla J, Scroggins RJ, Welly R, et al. Physical activity differentially affects the cecal microbiota of ovariectomized female rats selectively bred for high and low aerobic capacity. PLoS ONE. (2015) 10:e0136150. doi: 10.1371/journal.pone.0136150
66. Mika A, Van Treuren W, Gonzalez A, Herrera JJ, Knight R, Fleshner M. Exercise is more effective at altering gut microbial composition and producing stable changes in lean mass in juvenile versus adult male F344 rats. PLoS ONE. (2015) 10:e0125889. doi: 10.1371/journal.pone.0125889
67. Campbell SC, Wisniewski PJ, Noji M, McGuinness LR, Häggblom MM, Lightfoot SA, et al. The effect of diet and exercise on intestinal integrity and microbial diversity in mice. PloS ONE. (2016) 11:e0150502. doi: 10.1371/journal.pone.0150502
68. Petriz BA, Castro AP, Almeida JA, Gomes CP, Fernandes GR, Kruger RH, et al. Exercise induction of gut microbiota modifications in obese, non-obese and hypertensive rats. BMC Genomics. (2014) 15:511. doi: 10.1186/1471-2164-15-511
69. Welly RJ, Liu TW, Zidon TM, Rowles JL III, Park YM, Smith TN, et al. Comparison of diet versus exercise on metabolic function and gut microbiota in obese rats. Med Sci Sports Exerc. (2016) 48:1688–98. doi: 10.1249/MSS.0000000000000964
70. Denou E, Marcinko K, Surette MG, Steinberg GR, Schertzer JD. High-intensity exercise training increases the diversity and metabolic capacity of the mouse distal gut microbiota during diet-induced obesity. Am J Physiol-Endoc Metab. (2016) 310:E982–93. doi: 10.1152/ajpendo.00537.2015
71. Feng X, Uchida Y, Koch L, Britton S, Hu J, Lutrin D, et al. Exercise prevents enhanced postoperative neuroinflammation and cognitive decline and rectifies the gut microbiome in a rat model of metabolic syndrome. Front Immunol. (2017) 8:1768. doi: 10.3389/fimmu.2017.01768
72. Batacan R, Fenning A, Dalbo V, Scanlan A, Duncan M, Moore R, et al. A gut reaction: the combined influence of exercise and diet on gastrointestinal microbiota in rats. J Appl Microbiol. (2017) 122:1627–38. doi: 10.1111/jam.13442
73. Brandt N, Kotowska D, Kristensen CM, Olesen J, Lützhøft DO, Halling JF, et al. The impact of exercise training and resveratrol supplementation on gut microbiota composition in high-fat diet fed mice. Physiol Rep. (2018) 6:e13881. doi: 10.14814/phy2.13881
74. McCabe LR, Irwin R, Tekalur A, Evans C, Schepper JD, Parameswaran N, et al. Exercise prevents high fat diet-induced bone loss, marrow adiposity and dysbiosis in male mice. Bone. (2019) 118:20–31. doi: 10.1016/j.bone.2018.03.024
75. Liu Z, Liu HY, Zhou H, Zhan Q, Lai W, Zeng Q, et al. Moderate-intensity exercise affects gut microbiome composition and influences cardiac function in myocardial infarction mice. Front Microbiol. (2017) 8:1687. doi: 10.3389/fmicb.2017.01687
76. Munukka E, Ahtiainen JP, Puigbo P, Jalkanen S, Pahkala K, Keskitalo A, et al. Six-week endurance exercise alters gut metagenome that is not reflected in systemic metabolism in over-weight women. Front Microbiol. (2018) 9:2323. doi: 10.3389/fmicb.2018.02323
77. Taniguchi H, Tanisawa K, Sun X, Kubo T, Hoshino Y, Hosokawa M, et al. Effects of short-term endurance exercise on gut microbiota in elderly men. Physiol Rep. (2018) 6:e13935. doi: 10.14814/phy2.13935
78. Cronin O, Barton W, Skuse P, Penney NC, Garcia-Perez I, Murphy EF, et al. A prospective metagenomic and metabolomic analysis of the impact of exercise and/or whey protein supplementation on the gut microbiome of sedentary adults. MSystems. (2018) 3:e00044–18. doi: 10.1128/mSystems.00044-18
79. Barton W, Penney NC, Cronin O, Garcia-Perez I, Molloy MG, Holmes E, et al. The microbiome of professional athletes differs from that of more sedentary subjects in composition and particularly at the functional metabolic level. Gut. (2018) 67:625–33. doi: 10.1136/gutjnl-2016-313627
80. Petersen LM, Bautista EJ, Nguyen H, Hanson BM, Chen L, Lek SH, et al. Community characteristics of the gut microbiomes of competitive cyclists. Microbiome. (2017) 5:98. doi: 10.1186/s40168-017-0320-4
81. Shukla SK, Cook D, Meyer J, Vernon SD, Le T, Clevidence D, et al. Changes in gut and plasma microbiome following exercise challenge in myalgic encephalomyelitis/chronic fatigue syndrome (ME/CFS). PLoS ONE. (2015) 10:e0145453. doi: 10.1371/journal.pone.0145453
82. Bressa C, Bailén-Andrino M, Pérez-Santiago J, González-Soltero R, Pérez M, Montalvo-Lominchar MG, et al. Differences in gut microbiota profile between women with active lifestyle and sedentary women. PLoS ONE. (2017) 12:e0171352. doi: 10.1371/journal.pone.0171352
83. O'Donovan CM, Madigan SM, Garcia-Perez I, Rankin A, O'Sullivan O, Cotter PD. Distinct microbiome composition and metabolome exists across subgroups of elite Irish athletes. J Sci Med Sport. (2019) 23:63–8. doi: 10.1016/j.jsams.2019.08.290
84. Morita E, Yokoyama H, Imai D, Takeda R, Ota A, Kawai E, et al. Aerobic exercise training with brisk walking increases intestinal bacteroides in healthy elderly women. Nutrients. (2019) 11:868. doi: 10.3390/nu11040868
85. Yu Y, Mao G, Wang J, Zhu L, Lv X, Tong Q, et al. Gut dysbiosis is associated with the reduced exercise capacity of elderly patients with hypertension. Hypertens Res. (2018) 41:1036. doi: 10.1038/s41440-018-0110-9
86. Karl JP, Margolis LM, Madslien EH, Murphy NE, Castellani JW, Gundersen Y, et al. Changes in intestinal microbiota composition and metabolism coincide with increased intestinal permeability in young adults under prolonged physiological stress. Am J Physiol-Gastr Liver Physiol. (2017) 312:G559–71. doi: 10.1152/ajpgi.00066.2017
87. Lerman I, Harrison BC, Freeman K, Hewett TE, Allen DL, Robbins J, et al. Genetic variability in forced and voluntary endurance exercise performance in seven inbred mouse strains. J Appl Physiol. (2002) 92:2245–55. doi: 10.1152/japplphysiol.01045.2001
88. Zielinska S, Radkowski P, Blendowska A, Ludwig-Gałezowska A, Łoś JM, Łoś M. The choice of the DNA extraction method may influence the outcome of the soil microbial community structure analysis. Microbiol Open. (2017) 6:e00453. doi: 10.1002/mbo3.453
89. Aird D, Ross MG, Chen WS, Danielsson M, Fennell T, Russ C, et al. Analyzing and minimizing PCR amplification bias in Illumina sequencing libraries. Genome Biol. (2011) 12:R18. doi: 10.1186/gb-2011-12-2-r18
90. Sinha R, Abu-Ali G, Vogtmann E, Fodor AA, Ren B, Amir A, et al. Assessment of variation in microbial community amplicon sequencing by the Microbiome Quality Control (MBQC) project consortium. Nat Biotechnol. (2017) 35:1077–86. doi: 10.1038/nbt.3981
91. Moraska A, Deak T, Spencer RL, Roth D, Fleshner M. Treadmill running produces both positive and negative physiological adaptations in Sprague-Dawley rats. Am J Physiol Regul Integr Comp Physiol. (2000) 279:R1321–9. doi: 10.1152/ajpregu.2000.279.4.R1321
92. Noble EG, Moraska A, Mazzeo RS, Roth DA, Olsson MC, Moore RL, et al. Differential expression of stress proteins in rat myocardium after free wheel or treadmill run training. J Appl Physiol. (1999) 86:1696–701. doi: 10.1152/jappl.1999.86.5.1696
93. Leasure J, Jones M. Forced and voluntary exercise differentially affect brain and behavior. Neuroscience. (2008) 156:456–65. doi: 10.1016/j.neuroscience.2008.07.041
94. Kelly JR, Kennedy PJ, Cryan JF, Dinan TG, Clarke G, Hyland NP. Breaking down the barriers: the gut microbiome, intestinal permeability and stress-related psychiatric disorders. Front Cell Neurosci. (2015) 9:392. doi: 10.3389/fncel.2015.00392
95. Clark A, Mach N. Exercise-induced stress behavior, gut-microbiota-brain axis and diet: a systematic review for athletes. J Int Soc Sport Nutr. (2016) 13:43. doi: 10.1186/s12970-016-0155-6
96. Louis P, Flint HJ. Diversity, metabolism and microbial ecology of butyrate-producing bacteria from the human large intestine. FEMS Microbiol Lett. (2009) 294:1–8. doi: 10.1111/j.1574-6968.2009.01514.x
97. Kårlund A, Gómez-Gallego C, Turpeinen AM, Palo-oja OM, El-Nezami H, Kolehmainen M. Protein supplements and their relation with nutrition, microbiota composition and health: is more protein always better for sportspeople? Nutrients. (2019) 11:E829. doi: 10.3390/nu11040829
98. Dallas DC, Sanctuary MR, Qu Y, Khajavi SH, Van Zandt AE, Dyandra M, et al. Personalizing protein nourishment. Crit Rev Food Sci. (2017) 57:3313–31. doi: 10.1080/10408398.2015.1117412
99. Diether NE, Willing BP. Microbial fermentation of dietary protein: an important factor in diet–microbe–host interaction. Microorganisms. (2019) 7:19. doi: 10.3390/microorganisms7010019
100. Windey K, De Preter V, Verbeke K. Relevance of protein fermentation to gut health. Mol Nutr Food Res. (2012) 56:184–96. doi: 10.1002/mnfr.201100542
101. Portune KJ, Beaumont M, Davila AM, Tomé D, Blachier F, Sanz Y. Gut microbiota role in dietary protein metabolism and health-related outcomes: the two sides of the coin. Trends Food Sci Tech. (2016) 57:213–32. doi: 10.1016/j.tifs.2016.08.011
102. Zhu Y, Lin X, Zhao F, Shi X, Li H, Li Y, et al. Meat, dairy and plant proteins alter bacterial composition of rat gut bacteria. Sci Rep. (2015) 5:15220. doi: 10.1038/srep15220
103. Butteiger DN, Hibberd AA, McGraw NJ, Napawan N, Hall-Porter JM, Krul ES. Soy protein compared with milk protein in a western diet increases gut microbial diversity and reduces serum lipids in golden syrian hamsters. J Nutr. (2015) 146:697–705. doi: 10.3945/jn.115.224196
104. An C, Kuda T, Yazaki T, Takahashi H, Kimura B. Caecal fermentation, putrefaction and microbiotas in rats fed milk casein, soy protein or fish meal. Appl Microbiol Biol. (2014) 98:2779–87. doi: 10.1007/s00253-013-5271-5
105. van Vliet S, Burd NA, van Loon LJ. The skeletal muscle anabolic response to plant-versus animal-based protein consumption. J Nutr. (2015) 145:1981–91. doi: 10.3945/jn.114.204305
106. Burd NA, Beals JW, Martinez IG, Salvador AF, Skinner SK. Food-first approach to enhance the regulation of post-exercise skeletal muscle protein synthesis and remodeling. Sports Med. (2019) 49:59–68. doi: 10.1007/s40279-018-1009-y
107. Van Vliet S, Beals JW, Martinez IG, Skinner SK, Burd NA. Achieving optimal post-exercise muscle protein remodeling in physically active adults through whole food consumption. Nutrients. (2018) 10:224. doi: 10.3390/nu10020224
108. Burke LM, Cox GR, Cummings NK, Desbrow B. Guidelines for daily carbohydrate intake. Sports Med. (2001) 31:267–99. doi: 10.2165/00007256-200131040-00003
109. Burke LM, Hawley JA, Wong SH, Jeukendrup AE. Carbohydrates for training and competition. J Sports Sci. (2011) 29(Suppl. 1):S17–27. doi: 10.1080/02640414.2011.585473
110. Stellingwerff T, Cox GR. Systematic review: carbohydrate supplementation on exercise performance or capacity of varying durations. Appl Physiol Nutr Metab. (2014) 39:998–1011. doi: 10.1139/apnm-2014-0027
111. Sonnenburg ED, Sonnenburg JL. Starving our microbial self: the deleterious consequences of a diet deficient in microbiota-accessible carbohydrates. Cell Metab. (2014) 20:779–86. doi: 10.1016/j.cmet.2014.07.003
112. Chassard C, Lacroix C. Carbohydrates and the human gut microbiota. Curr Opin Clin Nutr Metab Care. (2013) 16:453–60. doi: 10.1097/MCO.0b013e3283619e63
113. Hamaker BR, Tuncil YE. A perspective on the complexity of dietary fiber structures and their potential effect on the gut microbiota. J Mol Biol. (2014) 426:3838–50. doi: 10.1016/j.jmb.2014.07.028
114. Sharma P, Bhandari C, Kumar S, Sharma B, Bhadwal P, Agnihotri N. Dietary fibers: a way to a healthy microbiome. Diet Microbiome Health. (2018) 2018:299–345. doi: 10.1016/B978-0-12-811440-7.00011-9
115. Paoli A, Bianco A, Grimaldi KA. The ketogenic diet and sport: a possible marriage? Exerc Sport Sci Rev. (2015) 43:153–62. doi: 10.1249/JES.0000000000000050
116. Fritzen AM, Lundsgaard AM, Kiens B. Dietary fuels in athletic performance. Annu Rev Nutr. (2019) 39:45–73. doi: 10.1146/annurev-nutr-082018-124337
117. Paoli A, Mancin L, Bianco A, Thomas E, Mota JF, Piccini F. Ketogenic diet and microbiota: friends or enemies? Genes. (2019) 10:534. doi: 10.3390/genes10070534
118. Ellerbroek A. The effect of ketogenic diets on the gut microbiota. J Exerc Nutr. (2018) 1:534. Available online at: https://www.journalofexerciseandnutrition.com/ManuscriptUploadsPDF/65.pdf
119. Nishitsuji K, Watanabe S, Xiao J, Nagatomo R, Ogawa H, Tsunematsu T, et al. Effect of coffee or coffee components on gut microbiome and short-chain fatty acids in a mouse model of metabolic syndrome. Sci Rep. (2018) 8:16173. doi: 10.1038/s41598-018-34571-9
120. Casazza GA, Tovar AP, Richardson CE, Cortez AN, Davis BA. Energy availability, macronutrient intake, and nutritional supplementation for improving exercise performance in endurance athletes. Curr Sport Med Rep. (2018) 17:215–23. doi: 10.1249/JSR.0000000000000494
121. Jeukendrup A. A step towards personalized sports nutrition: carbohydrate intake during exercise. Sports Med. (2014) 44(Suppl. 1): S25–33. doi: 10.1007/s40279-014-0148-z
122. Margaritelis NV, Paschalis V, Theodorou AA, Kyparos A, Nikolaidis MG. Antioxidants in personalized nutrition and exercise. Adv Nutr. (2018) 9:813–23. doi: 10.1093/advances/nmy052
123. Goñi I, Serrano J, Saura-Calixto F. Bioaccessibility of β-carotene, lutein, and lycopene from fruits and vegetables. J Agr Food Chem. (2006) 54:5382–7. doi: 10.1021/jf0609835
124. Palafox-Carlos H, Ayala-Zavala JF, González-Aguilar GA. The role of dietary fiber in the bioaccessibility and bioavailability of fruit and vegetable antioxidants. J Food Sci. (2011) 76:R6–15. doi: 10.1111/j.1750-3841.2010.01957.x
125. Selma MV, Espin JC, Tomas-Barberan FA. Interaction between phenolics and gut microbiota: role in human health. J Agric Food Chem. (2009) 57:6485–501. doi: 10.1021/jf902107d
126. Meinl W, Sczesny S, Brigelius-Flohe R, Blaut M, Glatt H. Impact of gut microbiota on intestinal and hepatic levels of phase 2 xenobiotic-metabolizing enzymes in the rat. Drug Metab Dispos. (2009) 37:1179–86. doi: 10.1124/dmd.108.025916
127. Djuric Z, Bassis CM, Plegue MA, Ren J, Chan R, Sidahmed E, et al. Colonic mucosal bacteria are associated with inter-individual variability in serum carotenoid concentrations. J Acad Nutr Diet. (2018) 118:606–16. e3. doi: 10.1016/j.jand.2017.09.013
128. Durk RP, Castillo E, Marquez-Magana L, Grosicki GJ, Bolter ND, Lee CM, et al. Gut microbiota composition is related to cardiorespiratory fitness in healthy young adults. Int J Sport Nutr Exerc Metab. (2018) 29:249–53. doi: 10.1123/ijsnem.2018-0024.
129. Estaki M, Pither J, Baumeister P, Little JP, Gill SK, Ghosh S, et al. Cardiorespiratory fitness as a predictor of intestinal microbial diversity and distinct metagenomic functions. Microbiome. (2016) 4:42. doi: 10.1186/s40168-016-0189-7
130. Yang Y, Shi Y, Wiklund P, Tan X, Wu N, Zhang X, et al. The association between cardiorespiratory fitness and gut microbiota composition in premenopausal women. Nutrients. (2017) 9:792. doi: 10.3390/nu9080792
131. Hsu YJ, Chiu CC, Li YP, Huang WC, Huang YT, Huang CC, et al. Effect of intestinal microbiota on exercise performance in mice. J Strength Cond Res. (2015) 29:552–8. doi: 10.1519/JSC.0000000000000644
132. Huang WC, Chen YH, Chuang HL, Chiu CC, Huang CC. Investigation of the effects of microbiota on exercise physiological adaption, performance, and energy utilization using a gnotobiotic animal model. Front Microbiol. (2019) 10:1906. doi: 10.3389/fmicb.2019.01906
133. Chen YM, Wei L, Chiu YS, Hsu YJ, Tsai TY, Wang MF, et al. Lactobacillus plantarum TWK10 supplementation improves exercise performance and increases muscle mass in mice. Nutrients. (2016) 8:205. doi: 10.3390/nu8040205
134. Huang WC, Hsu YJ, Li H, Kan NW, Chen YM, Lin JS, et al. Effect of Lactobacillus plantarum TWK10 on improving endurance performance in humans. Chin Physiol J. (2018) 61:163–70. doi: 10.4077/CJP.2018.BAH587
135. Jäger R, Shields KA, Lowery RP, De Souza EO, Partl JM, Hollmer C, et al. Probiotic Bacillus coagulans GBI-30, 6086 reduces exercise-induced muscle damage and increases recovery. Peer J. (2016) 4:e2276. doi: 10.7717/peerj.2276
136. Lamprecht M, Bogner S, Schippinger G, Steinbauer K, Fankhauser F, Hallstroem S, et al. Probiotic supplementation affects markers of intestinal barrier, oxidation, and inflammation in trained men; a randomized, double-blinded, placebo-controlled trial. J Int Soc Sport Nutr. (2012) 9:45. doi: 10.1186/1550-2783-9-45
137. Martarelli D, Verdenelli MC, Scuri S, Cocchioni M, Silvi S, Cecchini C, et al. Effect of a probiotic intake on oxidant and antioxidant parameters in plasma of athletes during intense exercise training. Curr Microbiol. (2011) 62:1689–96. doi: 10.1007/s00284-011-9915-3
138. Salarkia N, Ghadamli L, Zaeri F, Rad LS. Effects of probiotic yogurt on performance, respiratory and digestive systems of young adult female endurance swimmers: a randomized controlled trial. Med J Islam Repub Iran. (2013) 27:141–6.
139. Shing CM, Peake JM, Lim CL, Briskey D, Walsh NP, Fortes MB, et al. Effects of probiotics supplementation on gastrointestinal permeability, inflammation and exercise performance in the heat. Eur J Appl Physiol. (2014) 114:93–103. doi: 10.1007/s00421-013-2748-y
140. Townsend J, Bender D, Vantrease W, Sapp P, Toy A, Woods C, et al. Effects of Probiotic (Bacillus subtilis DE111) supplementation on immune function, hormonal status, and physical performance in division I baseball players. Sports. (2018) 6:70. doi: 10.3390/sports6030070
141. Scheiman J, Luber JM, Chavkin TA, MacDonald T, Tung A, Pham LD, et al. Meta-omics analysis of elite athletes identifies a performance-enhancing microbe that functions via lactate metabolism. Nat Med. (2019) 25:1104–9. doi: 10.1038/s41591-019-0485-4
142. Soares ADN, Wanner SP, Morais ESS, Hudson ASR, Martins FS, Cardoso VN. Supplementation with Saccharomyces boulardii increases the maximal oxygen consumption and maximal aerobic speed attained by rats subjected to an incremental-speed exercise. Nutrients. (2019) 11:2352. doi: 10.3390/nu11102352
143. Moreno-Navarrete JM, Fernandez-Real JM. The gut microbiota modulates both browning of white adipose tissue and the activity of brown adipose tissue. Rev Endocr Metab Disord. (2019). doi: 10.1007/s11154-019-09523-x. [Epub ahead of print].
144. Nichols AW. Probiotics and athletic performance: a systematic review. Curr Sport Med Rep. (2007) 6:269–73. doi: 10.1007/s11932-007-0044-5
145. Pyne DB, West NP, Cox AJ, Cripps AW. Probiotics supplementation for athletes - clinical and physiological effects. Eur J Sport Sci. (2015) 15:63–72. doi: 10.1080/17461391.2014.971879
146. Wosinska L, Cotter PD, O'Sullivan O, Guinane C. The potential impact of probiotics on the gut microbiome of athletes. Nutrients. (2019) 11:2270. doi: 10.3390/nu11102270
147. Huang WC, Lee MC, Lee CC, Ng KS, Hsu YJ, Tsai TY, et al. Effect of Lactobacillus plantarum TWK10 on exercise physiological adaptation, performance, and body composition in healthy humans. Nutrients. (2019) 11:2836. doi: 10.3390/nu11112836
148. Nay K, Jollet M, Goustard B, Baati N, Vernus B, Pontones M, et al. Gut bacteria are critical for optimal muscle function: a potential link with glucose homeostasis. Am J Physiol Endocrinol Metab. (2019) 317:E158–71. doi: 10.1152/ajpendo.00521.2018
149. Okamoto T, Morino K, Ugi S, Nakagawa F, Lemecha M, Ida S, et al. Microbiome potentiates endurance exercise through intestinal acetate production. Am J Physiol Endocrinol Metab. (2019) 316:E956–66. doi: 10.1152/ajpendo.00510.2018
150. Kovatcheva-Datchary P, Nilsson A, Akrami R, Lee YS, De Vadder F, Arora T, et al. Dietary fiber-induced improvement in glucose metabolism is associated with increased abundance of prevotella. Cell Metab. (2015) 22:971–82. doi: 10.1016/j.cmet.2015.10.001
151. Ticinesi A, Lauretani F, Milani C, Nouvenne A, Tana C, Del Rio D, et al. Aging gut microbiota at the cross-road between nutrition, physical frailty, and sarcopenia: is there a gut–muscle axis? Nutrients. (2017) 9:1303. doi: 10.3390/nu9121303
152. Hecksteden A, Kraushaar J, Scharhag-Rosenberger F, Theisen D, Senn S, Meyer T. Individual response to exercise training - a statistical perspective. J Appl Physiol. (2015) 118:1450–9. doi: 10.1152/japplphysiol.00714.2014
153. Mann TN, Lamberts RP, Lambert MI. High responders and low responders: factors associated with individual variation in response to standardized training. Sports Med. (2014) 44:1113–24. doi: 10.1007/s40279-014-0197-3
154. Swinton PA, Hemingway BS, Saunders B, Gualano B, Dolan E. A statistical framework to interpret individual response to intervention: paving the way for personalized nutrition and exercise prescription. Front Nutr. (2018) 5:41. doi: 10.3389/fnut.2018.00041
155. Hopkins WG. Individual responses made easy. J Appl Physiol. (2015) 118:1444–6. doi: 10.1152/japplphysiol.00098.2015
157. Bezkorovainy A. Probiotics: determinants of survival and growth in the gut. Am J Clin Nutr. (2001) 73:399s–405s. doi: 10.1093/ajcn/73.2.399s
158. Zmora N, Zilberman-Schapira G, Suez J, Mor U, Dori-Bachash M, Bashiardes S, et al. Personalized gut mucosal colonization resistance to empiric probiotics is associated with unique host and microbiome features. Cell. (2018) 174:1388–405 e21. doi: 10.1016/j.cell.2018.08.041
159. Zhang C, Derrien M, Levenez F, Brazeilles R, Ballal SA, Kim J, et al. Ecological robustness of the gut microbiota in response to ingestion of transient food-borne microbes. ISME J. (2016) 10:2235. doi: 10.1038/ismej.2016.13
160. Senan S, Prajapati JB, Joshi CG, Sreeja V, Gohel MK, Trivedi S, et al. Geriatric respondents and non-respondents to probiotic intervention can be differentiated by inherent gut microbiome composition. Front Microbiol. (2015) 6:25. doi: 10.3389/fmicb.2015.00944
161. Goodrich JK, Waters JL, Poole AC, Sutter JL, Koren O, Blekhman R, et al. Human genetics shape the gut microbiome. Cell. (2014) 159:789–99. doi: 10.1016/j.cell.2014.09.053
162. Cortese R, Lu L, Yu Y, Ruden D, Claud EC. Epigenome-Microbiome crosstalk: a potential new paradigm influencing neonatal susceptibility to disease. Epigenetics. (2016) 11:205–15. doi: 10.1080/15592294.2016.1155011
163. Benedict C, Vogel H, Jonas W, Woting A, Blaut M, Schürmann A, et al. Gut microbiota and glucometabolic alterations in response to recurrent partial sleep deprivation in normal-weight young individuals. Mol Metab. (2016) 5:1175–86. doi: 10.1016/j.molmet.2016.10.003
164. Fullagar HH, Skorski S, Duffield R, Hammes D, Coutts AJ, Meyer T. Sleep and athletic performance: the effects of sleep loss on exercise performance, and physiological and cognitive responses to exercise. Sports Med. (2015) 45:161–86. doi: 10.1007/s40279-014-0260-0
165. Tarnopolsky L, MacDougall J, Atkinson S, Tarnopolsky M, Sutton J. Gender differences in substrate for endurance exercise. J Appl Physiol. (1990) 68:302–8. doi: 10.1152/jappl.1990.68.1.302
166. Haro C, Rangel-Zúñiga OA, Alcalá-Díaz JF, Gómez-Delgado F, Pérez-Martínez P, Delgado-Lista J, et al. Intestinal microbiota is influenced by gender and body mass index. PloS ONE. (2016) 11:e0154090. doi: 10.1371/journal.pone.0154090
167. O'Toole PW, Claesson MJ. Gut microbiota: changes throughout the lifespan from infancy to elderly. Int Dairy J. (2010) 20:281–91. doi: 10.1016/j.idairyj.2009.11.010
168. Ni Lochlainn M, Bowyer R, Steves C. Dietary protein and muscle in aging people: the potential role of the gut microbiome. Nutrients. (2018) 10:929. doi: 10.3390/nu10070929
169. Shin HE, Kwak SE, Lee JH, Zhang D, Bae JH, Song W. Exercise, the gut microbiome, and frailty. Ann Geriatr Med Res. (2019) 23:105–14. doi: 10.4235/agmr.19.0014
170. Grosicki GJ, Fielding RA, Lustgarten MS. Gut microbiota contribute to age-related changes in skeletal muscle size, composition, and function: biological basis for a gut-muscle axis. Calcified Tissue Int. (2018) 102:433–42. doi: 10.1007/s00223-017-0345-5
171. Claesson MJ, Jeffery IB, Conde S, Power SE, O'connor EM, Cusack S, et al. Gut microbiota composition correlates with diet and health in the elderly. Nature. (2012) 488:178. doi: 10.1038/nature11319
172. Ticinesi A, Tana C, Nouvenne A. The intestinal microbiome and its relevance for functionality in older persons. Curr Opin Clin Nutr Metab Care. (2019) 22:4–12. doi: 10.1097/MCO.0000000000000521
173. Siddharth J, Chakrabarti A, Pannerec A, Karaz S, Morin-Rivron D, Masoodi M, et al. Aging and sarcopenia associate with specific interactions between gut microbes, serum biomarkers and host physiology in rats. Aging. (2017) 9:1698. doi: 10.18632/aging.101262
174. Ticinesi A, Lauretani F, Tana C, Nouvenne A, Ridolo E, Meschi T. Exercise and immune system as modulators of intestinal microbiome: implications for the gut-muscle axis hypothesis. Exerc Immunol Rev. (2019) 25:84–95. Available online at: http://eir-isei.de/2019/eir-2019-084-article.pdf
175. Gubert C, Kong G, Renoir T, Hannan AJ. Exercise, diet and stress as modulators of gut microbiota: implications for neurodegenerative diseases. Neurobiol Dis. (2019) 134:104621. doi: 10.1016/j.nbd.2019.104621
176. Schlegel P, Novotny M, Klimova B, Valis M. “Muscle-gut-brain axis”: can physical activity help patients with Alzheimer's disease due to microbiome modulation? J Alzheimers Dis. (2019) 71:861–78. doi: 10.3233/JAD-190460
177. Buigues C, Fernández-Garrido J, Pruimboom L, Hoogland A, Navarro-Martínez R, Martínez-Martínez M, et al. Effect of a prebiotic formulation on frailty syndrome: a randomized, double-blind clinical trial. Int J Mol Sci. (2016) 17:932. doi: 10.3390/ijms17060932
178. Walsh ME, Bhattacharya A, Sataranatarajan K, Qaisar R, Sloane L, Rahman MM, et al. The histone deacetylase inhibitor butyrate improves metabolism and reduces muscle atrophy during aging. Aging Cell. (2015) 14:957–70. doi: 10.1111/acel.12387
179. Ryu D, Mouchiroud L, Andreux PA, Katsyuba E, Moullan N, Nicolet-dit-Félix AA, et al. Urolithin A induces mitophagy and prolongs lifespan in C. elegans and increases muscle function in rodents. Nat Med. (2016) 22:879. doi: 10.1038/nm.4132
Keywords: gut microbiome, exercise, personalized nutrition, sports nutrition, performance, metabolism, athletes, optimization
Citation: Hughes RL (2020) A Review of the Role of the Gut Microbiome in Personalized Sports Nutrition. Front. Nutr. 6:191. doi: 10.3389/fnut.2019.00191
Received: 11 October 2019; Accepted: 12 December 2019;
Published: 10 January 2020.
Edited by:
David Christopher Nieman, Appalachian State University, United StatesReviewed by:
David Michael Bellar, University of North Carolina at Charlotte, United StatesJonathan Peake, Queensland University of Technology, Australia
Copyright © 2020 Hughes. This is an open-access article distributed under the terms of the Creative Commons Attribution License (CC BY). The use, distribution or reproduction in other forums is permitted, provided the original author(s) and the copyright owner(s) are credited and that the original publication in this journal is cited, in accordance with accepted academic practice. No use, distribution or reproduction is permitted which does not comply with these terms.
*Correspondence: Riley L. Hughes, cmxodWdoZXNAdWNkYXZpcy5lZHU=