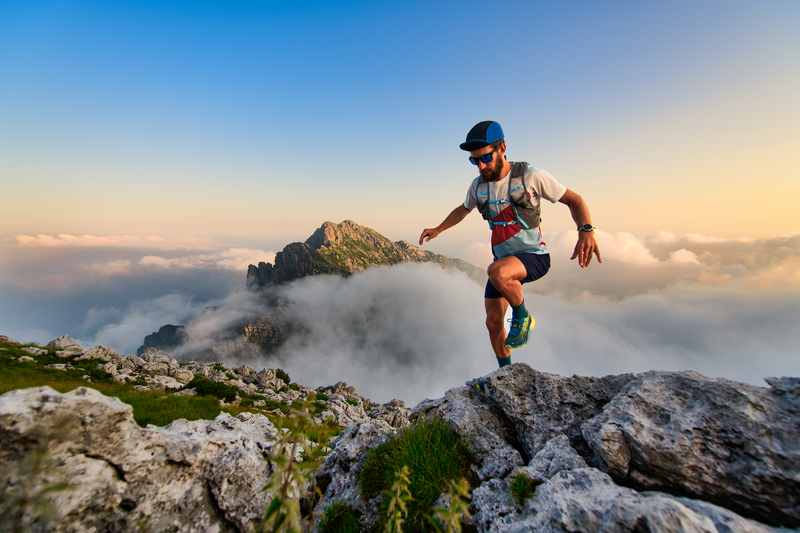
95% of researchers rate our articles as excellent or good
Learn more about the work of our research integrity team to safeguard the quality of each article we publish.
Find out more
MINI REVIEW article
Front. Nutr. , 24 April 2019
Sec. Nutrition and Food Science Technology
Volume 6 - 2019 | https://doi.org/10.3389/fnut.2019.00050
This article is part of the Research Topic Dietary Carbohydrate Digestibility and Metabolic Effects in Human Health View all 11 articles
Among the many bioactive components in human milk, the free oligosaccharides (OS) have been intensely studied in recent decades due to their unique ability to selectively modulate the infant gut microbiota, in addition to providing numerous other health benefits. In light of the demonstrated value of these compounds, recent studies have set out to characterize the structures and properties of the similar and more widely-available OS in the dairy industry. This mini review gives a brief overview of the common analytical techniques used to characterize bovine milk OS and highlights several recent, key studies that have identified valuable physiological and metabolic effects of these molecules in vivo. Although traditionally considered indigestible by human enzymes, evidence now suggests that milk OS are partially absorbed in the intestines and likely contribute to the development of molecular structures in the brain. Furthermore, aside from their prebiotic effects, these compounds show promise as therapeutics that could alleviate numerous metabolic abnormalities, including undernutrition, obesity, and excessive intestinal permeability. The need for novel treatments to address these and related health issues is motivating the development of scalable techniques to produce large quantities of milk OS for use as food ingredients. The safety and tolerability of high dosages of bovine milk OS have been demonstrated in two independent human studies, which potentially opens the door for further research aiming to utilize these molecules to alleviate common metabolic health issues.
Milk harbors a suite of bioactive compounds, including free oligosaccharide (OS) structures that are well-characterized as selective prebiotics and that play an important role in infant health and development (1, 2). Research in the last few decades has made immense progress in characterizing the beneficial biological functions of OS and uncovering the mechanistic pathways by which they are exerted. The majority of studies on milk OS functionality initially focused on the highly-concentrated OS in human milk. Human milk OS have been extensively profiled, with as many as 200 structures being identified in comprehensive studies (3, 4), and the ability of gut-associated bacteria to consume many of these structures is well-documented (5–7). Several gut bacterial species, including select species of Lactobacillus and Bifidobacteria, are highly desirable due to their ability to down-regulate an over-active immune system and reduce inflammatory response (8). This research field has now expanded to identify the similar structures and bioactivities of milk OS from other mammalian species. In particular, industrial production of bovine milk has prompted studies into the therapeutic value of bovine milk oligosaccharides (BMOs) and the dairy industry's relatively underutilized BMO-containing side streams. This mini-review highlights recent studies that demonstrate novel bioactivities of BMOs, with a particular focus on their digestibility and metabolic effects. The paper also provides an overview of the current analytical tools used in OS characterization and the development of industrial-scale processes for BMO production. Considering the wide availability of BMOs in dairy streams and the current need for therapeutics with BMO-like functionalities, these molecules show promise as a solution to epidemic metabolic and digestive illnesses.
Oligosaccharides in bovine milk are assembled in the mammary gland by combining the monosaccharides glucose (Glc), galactose (Gal), N-acetylglucosamine (GlcNAc), N-acetylgalactosamine, fucose, and the sialic acids N-acetylneuraminic acid and N-glycolylneuraminic acid (9, 10). The OS structures contain either lactose (Gal(β1-4)Glc) or N-acetyllactosamine (Gal(β1-4)GlcNAc) at their reducing end, with additional monosaccharide residues branching off from the non-reducing galactose (9, 10). In some cases, the BMOs possess lacto-N-biose (Gal(β1-3)GlcNAc) or N-acetyllactosamine units linked to the lactose core, which are defining features of the type 1 and type 2 OS structures contained within many human milk OS (Figure 1C) (1, 9). The collection of BMOs found in milk and colostrum has been extensively profiled by our research group, with 30–50 structures typically being identified in comprehensive studies (9, 12–14). Although bovine milk contains fewer OS structures than human milk, the two share at least 10 common structures (Figure 1C), including the acidic 3′-sialyllactose and 6′-sialyllactose. These two OS comprise a large percentage of the BMO pool (9, 11). Complete or partial structures are known for many BMOs (9); however, there remains a large proportion of BMOs for which only monosaccharide compositions are known. For example, recent studies have identified several large fucosylated OS in bovine milk, but to date only the monosaccharide compositions have been determined (9, 15, 16). A more complete structural characterization of the entire BMO pool could improve our understanding of their bioactivities and digestibilities by microbes. In many cases, linkage types influence functionality, as exemplified by in vivo studies showing the ability of α1-2–linked fucosyloligosaccharides to prevent Campylobacter jejuni infection (17).
Figure 1. Overview of common analytical methodologies used to study milk oligosaccharides. Following a suitable extraction procedure, bovine milk is often analyzed by liquid chromatography-mass spectrometry to identify the oligosaccharides present in a sample and measure their abundances (A). Quantification can be conducted using peak heights or areas (label-free quantification) or by isotopic labeling strategies (B). Through analysis of tandem-MS data and comparison with analytical standards, specific oligosaccharide structures present in a sample can be deduced. This panel represents a selection of oligosaccharides with fully-elucidated structures that are found in both bovine and human milk (C) (11). Hex, hexose; NeuAc, N-acetylneuraminic acid. – glucose;
– galactose;
– N-acetylglucosamine;
– N-acetylneuraminic acid;
– fucose.
Identification of the individual OS structures in milk has come with numerous analytical challenges, many of which have been resolved in the past two decades. The free OS in milk are frequently analyzed by liquid chromatography-mass spectrometry (LC-MS, Figure 1A) (12, 16, 18). This technique is utilized for initial discovery and profiling of the entire collection of OS in a sample. It conveniently provides relative compound abundances and monosaccharide compositions for a multitude of OS within a single experiment. Common LC-MS strategies for OS analysis have been recently reviewed in greater detail elsewhere (19). However, the potential for branching within an OS structure, as well as the numerous possible linkages between neighboring monosaccharides, often requires further experiments to achieve complete structural elucidation.
In some cases complementary techniques are used along with MS for in-depth characterization of OS structures. For example, Aldredge et al. (9) fractionated the bovine milk OS pool by high performance liquid chromatography, incubated each bovine milk OS with glycosidases of known specificity, and analyzed the changes produced with LC-MS. This labor-intensive approach determined a variety of glycosidic linkages and specific monosaccharide types for numerous OS (9). A similar approach was used by Wu et al. in the determination of human milk OS structures (3, 20). Alternatively, more rapid approaches that do not rely on pre-fractionation use strategic derivatization and subsequent analysis of the hydrolyzed monosaccharides to provide at least partial linkage information. Galermo et al. have recently published a method by which the monosaccharides and linkage types present in oligo- and polysaccharides can be determined in a high-throughput manner using a pair of derivatization strategies and LC-MS (21).
Not surprisingly, the multitude of potential glycosidic linkages and the efforts required to deduce complete OS structures has hindered chemical synthesis of larger OS structures for use as analytical standards. However, several standards are now available for the smaller bovine milk OS, allowing absolute quantification of a subset of the OS ensemble (18, 22). When analytical standards are unavailable, OS abundances are often measured in relative terms. This can be done using measures such as mass spectral peak height or chromatographic peak area of OS to compare abundances among samples, known as a “label free” relative quantification. Alternatively, several derivatization strategies have been developed that allow relative abundances of isotopically-labeled carbohydrates to be compared on the basis of the intensity of their unique mass spectral peaks. Some examples include reducing-end derivatization of glycan sample pairs with a heavy/light label pair (23, 24) or with a series of isobaric reagents (Figure 1B) (25, 26). Further details of these relative quantification techniques and derivatization strategies have been described in a recent book chapter by Orlando (27) and in a review by Dong et al. (28), Robinson (29).
Although analytical standards for quantification do not yet exist for many BMOs, the total OS concentration in bovine milk is estimated at approximately 1–2 g/L in colostrum and 100 mg/L in mature milk (18, 30, 31). Despite the current difficulties in quantifying some OS structures, analytical studies have deduced a wealth of relevant information on OS production in cows. During the first week of lactation, BMO abundances drop relatively quickly and decline somewhat further as cows transition to mature milk (14, 18). Bovine colostrum is a particularly rich source of these OS, and processing streams within the dairy industry have the potential to serve as a raw material for BMO isolation. During cheese production, non-casein proteins and polar molecules such as salts, lactose, and BMOs are eliminated from the cheese as whey. Purification of the whey proteins produces a liquid byproduct known as whey permeate, a stream that contains BMOs (32). Although uses for whey permeate have been identified, it is often considered a waste stream (33), and dried whey permeate and its byproducts are typically sold at low prices (34). Therefore, recovery of BMOs from whey permeate could add value to this stream and improve dairy industry sustainability. Pilot-scale techniques to isolate OS from this dairy stream have been developed using membrane filtration (35, 36). The wide availability of dairy side streams that contain BMOs could allow these processing techniques to feasibly produce isolated milk OS for functional testing and therapeutic applications (Figure 2). As described in the following sections, isolated BMOs have demonstrated beneficial health effects in a variety of in vivo studies.
Figure 2. A pictorial representation of the progress made in milk oligosaccharide functional testing and isolation strategies over the past 20 years. Previously, functional testing was generally limited to in vitro studies conducted with oligosaccharides isolated from milk at lab scale. Synthetic and pilot-scale isolation can now generate these compounds in larger quantities, and oligosaccharides from both sources have been used in in vivo studies and clinical trials (37).
Several studies have explored variations in milk OS abundances within dairy cattle in order to characterize industrial BMO availability and to elucidate factors that influence OS production. The Holstein-Friesian and Jersey breeds are commonly used for milk production, and several studies have examined differences in OS production among these and related breeds (14, 16, 30). Most recently, we have profiled milk OS abundances in a total of 634 samples collected from these breeds and have measured greater amounts of most OS in milk from the Jersey breed (38). The Jersey cows, however, also showed much greater cow-to-cow variability. In light of the fact that environmental sources of variation were controlled, these results may reflect an underlying genetic influence on OS production. A recent study by Liu et al. examined the OS content of genotyped Australian Holstein cows and measured high heritabilities for many OS, indicating that the variation in OS abundances between cows were substantially influenced by genetics (39). The study also identified numerous quantitative trait loci, or regions of the genome which likely influence OS abundances (39). Further studies on this topic will be integral to complete elucidation of the pathways responsible for BMO synthesis. While it is suspected that free milk OS are synthesized by some of the same enzymatic pathways that are used in protein-linked glycan synthesis, investigating the genetic influence on OS production should provide more concrete proof of this possibility. This knowledge could also enable implementation of selective breeding strategies to increase the levels of BMOs in milk without requiring genetically modified organism-based approaches.
Free milk OS are typically considered indigestible by human enzymes (40–42). Nonetheless, there are several reports of human milk OS existing in infant blood (43) and urine (44–46), indicating that a portion of these molecules are absorbed and circulate in the body. The degree of absorption appears to vary substantially by structure (44), and the biological implications of this absorption have yet to be fully elucidated. It has been hypothesized that absorbed OS can prevent urinary tract infections in infants (47), and recent in vivo evidence demonstrates that consumption of 3′-sialyllactose and 6′-sialyllactose increases brain ganglioside-bound sialic acid content in piglets (48). Dietary supplementation with various forms of sialic acid (free or bound to milk OS or protein-linked glycans) has improved learning and increased brain sialic acid content in animal studies (49–51), suggesting that these carbohydrates make an important contribution to brain development.
Milk OS that are not absorbed are available for consumption by the gut microbiota. Human milk has long been known to influence the development of the infant gut microbiota in ways that confer health benefits to the infant, and more recent studies have determined that the milk OS are key to providing this prebiotic functionality (1, 5, 7, 11, 52). These OS selectively feed specific bacterial species that possess the enzymes necessary to metabolize the wide variety of glycosidic linkages found in OS (7, 11, 53). Several of these prebiotic OS from human milk, including lacto-N-tetraose and the sialyllactose isomer pair, are also found in bovine milk (9, 40), and the ability of the BMO ensemble to modulate the gut microbiota in vivo has recently been demonstrated (54, 55). Considering the wide availability of dairy side streams from which these OS can be isolated, BMOs show promise as future therapeutics that could be used to provide human milk OS-associated health benefits to infants and adults at a large scale. Initial studies utilizing these OS from dairy streams have revealed a variety of metabolic benefits resulting from BMO consumption, which are reviewed in the following section.
A well-studied metabolic impact of prebiotic carbohydrates is the ability to indirectly influence short-chain fatty acid (SCFA) production in the intestine by promoting the growth of SCFA-generating bacteria (56, 57). SCFAs are products of anaerobic bacterial fermentation that occurs in the gastrointestinal tract. The major SCFAs produced by the gut microbiota are acetate, butyrate, and propionate. These bacterial metabolites are used as substrates for a variety of host processes, including cholesterol synthesis and gluconeogenesis in the liver, as well as serving as a key energy source for colonocytes (58). The bacterial genera Bifidobacterium and Bacteroides, each of which contain well-characterized milk OS consumers (5, 7, 59), are contributors to SCFA production (56, 60). Recently, our research group has shown that BMO supplementation alone significantly increased the expression of butyrate-generating bacterial genes in western diet-fed mouse models (55). Aside from being the preferred energy source for colonocytes (58), butyrate can have anti-inflammatory effects in the liver and colon (61).
Isolation of milk OS from dairy streams has enabled experiments identifying novel metabolic effects of OS in vivo. A study by Charbonneau et al. used animal models of infant undernutrition to show that dietary supplementation with BMOs provides a microbially-mediated increase in lean body mass and bone growth, and generates metabolite profiles indicative of improved nutrient utilization (62). These results were characterized in both gnotobiotic mice and piglets, and they provide striking evidence that milk OS, in combination with the gut microbiota, play a substantial role in development and regulation of metabolic pathways. Although other non-milk carbohydrate polymers, such as inulin, share some of the properties of milk OS, this study revealed that the metabolic changes induced by milk OS were not duplicated with inulin supplementation (62). Therefore, the unique functionalities of milk OS may be imparted by their higher diversity of monosaccharide types and linkages compared to the less structurally diverse prebiotic polymers.
The availability of a pilot-scale supply of milk OS has also led to key experiments demonstrating the beneficial effects of OS on the development of obesity and intestinal permeability. With the prevalence of overweight adults reaching nearly 40% worldwide, and obesity at 13% worldwide (63), novel strategies to combat this unfavorable metabolic state could lead to widespread improvements in health status and reduce healthcare costs arising from obesity-associated illnesses. A growing body of evidence is establishing a causal relationship between gut microbial dysbiosis, intestinal permeability, and the onset of obesity. Weight gain from diet-induced obesity occurs simultaneously with altered intestinal permeability and gut microbial profiles, as well as decreases in anti-inflammatory cytokine expression (64, 65). Furthermore, transplantation of the gut microbiota from an obese individual to germ-free mice can induce elevated weight gain in the mice (66, 67), highlighting the gut environment as a potential target for therapeutic interventions. Though not entirely understood, it is possible that obesity onset is at least partially initiated by increases in circulating bacterial endotoxin as a result of altered intestinal permeability, as outlined in a previous review (68). Therefore, treatments that maintain gut barrier function and/or reduce endotoxin circulation could lead to viable interventions to prevent obesity and its related metabolic conditions. Studies investigating the ability of BMOs to modulate intestinal permeability have shown promising results. Hamilton et al. recently showed that consumption of an ensemble of BMOs can significantly reduce weight gain and reduce the intestinal permeability that is induced in mice consuming a high-fat diet (54). Dietary supplementation with BMOs also increased SCFA abundance in the cecum, an effect that was not replicated by inulin supplementation (54). In a similar study, introduction of BMOs to the diet of high fat-fed mice, in combination with a weekly gavage of the probiotic Bifidobacterium longum subspecies infantis, prevented increases in intestinal permeability otherwise associated with the high-fat diet (69).
The prebiotic effect of milk OS could be another significant factor contributing to obesity prevention. The presence of bifidobacteria in the mouse gastrointestinal tract is correlated with reduced plasma and intestinal endotoxin levels (70, 71). Conversely, the gut microbiota of high fat-fed mice is associated with increased endotoxin levels. Cani et al. have shown that administration of broad-spectrum antibiotics to mice consuming a high fat diet reduces plasma endotoxin levels to that of the control mice, while antibiotic administration to the control mice produced no significant change in plasma endotoxin (65). The presence of bifidobacteria has also been correlated with a reduction in diabetic symptoms, including improved glucose tolerance (70). Modulation of the gut microbiota may therefore be another promising strategy to prevent these prevalent metabolic issues. Furthermore, although prevention of the obese phenotype is a major clinical target, prevention of gut dysbiosis and excessive intestinal permeability will likely have other important health effects. For example, a high-fat diet can induce liver abnormalities, such as steatosis and inflammation (72), and a recent report suggests that these effects can be eliminated via regulating lipid and glucose metabolism through the consumption of BMOs and Bifidobacterium longum subspecies infantis in genetically predisposed animal models (55). Considering the interrelated nature of these and other physiological processes, the above studies may represent only a fraction of the metabolic benefits provided by milk OS.
In light of the promising effects of milk OS consumption, industrial interest in marketing these compounds for therapeutic purposes is building. As of January 2019, over 180 US patents have been filed relating to 2′-fucosyllactose alone, and the inclusion of 2′-fucosyllactose as a food ingredient is now commonplace in infant formula (73, 74). The use of 2′-fucosyllactose as an ingredient has prompted a need for large quantities of this OS to be produced synthetically. A multitude of strategies to produce synthetic OS have been designed, using both genetically engineered microorganisms and enzymatic approaches. These strategies, which have been reviewed extensively elsewhere (75), are promising routes by which individual OS could be made available at large scale. These synthetic approaches, in conjunction with the established membrane filtration strategies described above, will likely grow in application to supply the marketplace with OS that can be used as ingredients for therapeutic foods, extending availability of milk OS and their bioactivities to the general public.
Growing availability of milk OS for in vivo experimentation is uncovering a multitude of unique and previously unknown bioactivities that could be harnessed to alleviate widespread metabolic illnesses. Future studies will likely probe further into the mechanistic details of OS functionalities, as well as evaluate the feasibility of supplementing these molecules into adult diets as therapeutics. The safety and tolerability of isolated milk OS for human consumption were recently evaluated in two independent studies and showed promising results, with even relatively high dosages being well-tolerated (76, 77). These studies could pave the way for the known metabolic impacts of BMOs to be further evaluated in human subjects, including in specialized applications such as infant formula production. Finally, implementing industrial-scale strategies to produce and isolate OS with desired bioactivities will be imperative to the application of OS as therapeutics. Therefore, we should expect continued work to identify factors influencing OS production in dairy cattle, as well as efforts to translate pilot-scale isolation techniques to dairy processing facilities.
The author confirms being the sole contributor of this work and has approved it for publication.
The author acknowledges support from the National Institute of Health award R01AT007079 and from the United States Department of Agriculture CRIS# 8040-51530-056-00D.
The author declares that the research was conducted in the absence of any commercial or financial relationships that could be construed as a potential conflict of interest.
The author would like to thank Dr. Daniela Barile for providing feedback on the article content. A portion of the section entitled Analytical methodologies for milk OS is taken from the author's dissertation (75).
BMO, bovine milk oligosaccharide; Gal, galactose; Glc, glucose; GlcNAc, N-acetylglucosamine; LC-MS, liquid chromatography—mass spectrometry; OS, oligosaccharide.
1. Bode L. Human milk oligosaccharides: every baby needs a sugar mama. Glycobiology. (2012) 22:1147–62. doi: 10.1093/glycob/cws074
2. Morrow AL, Newburg DS. Human milk oligosaccharide. In: Neu J, Poindexter B, Polin RA, editors. Gastroenterology and Nutrition: Neonatology Questions and Controversies. Philadelphia, PA: Elsevier (2019). p. 43–58.
3. Wu S, Tao N, German JB, Grimm R, Lebrilla CB. Development of an annotated library of neutral human milk oligosaccharides. J Proteome Res. (2010) 9:4138–51. doi: 10.1021/pr100362f
4. Ninonuevo MR, Park Y, Yin H, Zhang J, Ward RE, Clowers BH, et al. A strategy for annotating the human milk glycome. J Agric Food Chem. (2006) 54:7471–80. doi: 10.1021/jf0615810
5. LoCascio RG, Ninonuevo MR, Freeman SL, Sela DA, Grimm R, Lebrilla CB, et al. Glycoprofiling of bifidobacterial consumption of human milk oligosaccharides demonstrates strain specific, preferential consumption of small chain glycans secreted in early human lactation. J Agric Food Chem. (2007) 55:8914–9. doi: 10.1021/jf0710480
6. Marcobal A, Barboza M, Froehlich JW, Block DE, German JB, Lebrilla CB, et al. Consumption of human milk oligosaccharides by gut-related microbes. J Agric Food Chem. (2010) 58:5334–40. doi: 10.1021/jf9044205
7. Yu Z-T, Chen C, Newburg DS. Utilization of major fucosylated and sialylated human milk oligosaccharides by isolated human gut microbes. Glycobiology. (2013) 23:1281–92. doi: 10.1093/glycob/cwt065
8. Hardy H, Harris J, Lyon E, Beal J, Foey AD. Probiotics, prebiotics and immunomodulation of gut mucosal defences: homeostasis and immunopathology. Nutrients. (2013) 5:1869–912. doi: 10.3390/nu5061869
9. Aldredge DL, Geronimo MR, Hua S, Nwosu CC, Lebrilla CB, Barile D. Annotation and structural elucidation of bovine milk oligosaccharides and determination of novel fucosylated structures. Glycobiology. (2013) 23:664–76. doi: 10.1093/glycob/cwt007
10. Gopal PK, Gill HS. Oligosaccharides and glycoconjugates in bovine milk and colostrum. Br J Nutr. (2000) 84:69–74. doi: 10.1017/S0007114500002270
11. Kirmiz N, Robinson RC, Shah IM, Barile D, Mills DA. Milk glycans and their interaction with the infant gut microbiota. Annu Rev Food Sci Technol. (2018) 9:429–50. doi: 10.1146/annurev-food-030216-030207
12. Tao N, DePeters EJ, Freeman S, German JB, Grimm R, Lebrilla CB. Bovine milk glycome. J Dairy Sci. (2008) 91:3768–78. doi: 10.3168/jds.2008-1305
13. Barile D, Marotta M, Chu C, Mehra R, Grimm R, Lebrilla CB, et al. Neutral and acidic oligosaccharides in Holstein-Friesian colostrum during the first 3 days of lactation measured by high performance liquid chromatography on a microfluidic chip and time-of-flight mass spectrometry. J Dairy Sci. (2010) 93:3940–9. doi: 10.3168/jds.2010-3156
14. Tao N, DePeters EJ, German JB, Grimm R, Lebrilla CB. Variations in bovine milk oligosaccharides during early and middle lactation stages analyzed by high-performance liquid chromatography-chip/mass spectrometry. J Dairy Sci. (2009) 92:2991–3001. doi: 10.3168/jds.2008-1642
15. Mehra R, Barile D, Marotta M, Lebrilla CB, Chu C, German JB. Novel high-molecular weight fucosylated milk oligosaccharides identified in dairy streams. PLoS ONE. (2014) 9:e96040. doi: 10.1371/journal.pone.0096040
16. Sundekilde UK, Barile D, Meyrand M, Poulsen NA, Larsen LB, Lebrilla CB, et al. Natural variability in bovine milk oligosaccharides from Danish Jersey and Holstein-Friesian breeds. J Agric Food Chem. (2012) 60:6188–96. doi: 10.1021/jf300015j
17. Ruiz-Palacios GM, Cervantes LE, Ramos P, Chavez-Munguia B, Newburg DS. Campylobacter jejuni binds intestinal H (O) antigen (Fucα1, 2Galβ1, 4GlcNAc), and fucosyloligosaccharides of human milk inhibit its binding and infection. J Biol Chem. (2003) 278:14112–20. doi: 10.1074/jbc.M207744200
18. Fong B, Ma K, McJarrow P. Quantification of bovine milk oligosaccharides using liquid chromatography–selected reaction monitoring–mass spectrometry. J Agric Food Chem. (2011) 59:9788–95. doi: 10.1021/jf202035m
19. Kailemia MJ, Ruhaak LR, Lebrilla CB, Amster IJ. Oligosaccharide analysis by mass spectrometry: a review of recent developments. Anal Chem. (2014) 86:196–212. doi: 10.1021/ac403969n
20. Wu S, Grimm R, German JB, Lebrilla CB. Annotation and structural analysis of sialylated human milk oligosaccharides. J Proteome Res. (2011) 10:856–68. doi: 10.1021/pr101006u
21. Galermo AG, Nandita E, Barboza M, Amicucci MJ, Vo T-TT, Lebrilla CB. Liquid chromatography–tandem mass spectrometry approach for determining glycosidic linkages. Anal Chem. (2018) 90:13073–80 doi: 10.1021/acs.analchem.8b04124
22. Lee H, de MeloSilva VL, Liu Y, Barile D. Short communication: Quantification of carbohydrates in whey permeate products using high-performance anion-exchange chromatography with pulsed amperometric detection. J Dairy Sci. (2015) 98:7644–9. doi: 10.3168/jds.2015-9882
23. Zhang P, Zhang Y, Xue X, Wang C, Wang Z, Huang L. Relative quantitation of glycans using stable isotopic labels 1-(d0/d5) phenyl-3-methyl-5-pyrazolone by mass spectrometry. Anal Biochem. (2011) 418:1–9. doi: 10.1016/j.ab.2011.07.006
24. Walker SH, Budhathoki-Uprety J, Novak BM, Muddiman DC. Stable-isotope labeled hydrophobic hydrazide reagents for the relative quantification of N-linked glycans by electrospray ionization mass spectrometry. Anal Chem. (2011) 83:6738–45. doi: 10.1021/ac201376q
25. Hahne H, Neubert P, Kuhn K, Etienne C, Bomgarden R, Rogers JC, et al. Carbonyl-reactive tandem mass tags for the proteome-wide quantification of N-linked glycans. Anal Chem. (2012) 84:3716–24. doi: 10.1021/ac300197c
26. Robinson RC, Poulsen NA, Barile D. Multiplexed bovine milk oligosaccharide analysis with aminoxy tandem mass tags. PLoS ONE. (2018) 13:e0196513. doi: 10.1371/journal.pone.0196513
27. Orlando R. Quantitative analysis of glycoprotein glycans. In: Kohler JJ, Patrie SM, editors. Mass Spectrometry of Glycoproteins: Methods and Protocols Methods in Molecular Biology. Totowa, NJ: Humana Press (2013). p. 197–215.
28. Dong X, Huang Y, Cho BG, Zhong J, Gautam S, Peng W, et al. Advances in mass spectrometry-based glycomics. Electrophoresis. (2018) 39:3063–81. doi: 10.1002/elps.201800273
29. Robinson RC. Novel Analytical Techniques for Characterization of Health-Promoting Bioactive Peptides and Oligosaccharides in Dairy Products. dissertation. University of California, Davis, Davis, CA. (2019).
30. McJarrow P, van Amelsfort-Schoonbeek J. Bovine sialyl oligosaccharides: seasonal variations in their concentrations in milk, and a comparison of the colostrums of Jersey and Friesian cows. Int Dairy J. (2004) 14:571–9. doi: 10.1016/j.idairyj.2003.11.006
31. Nakamura T, Kawase H, Kimura K, Watanabe Y, Ohtani M, Arai I, et al. Concentrations of sialyloligosaccharides in bovine colostrum and milk during the prepartum and early lactation. J Dairy Sci. (2003) 86:1315–20. doi: 10.3168/jds.S0022-0302(03)73715-1
32. Barile D, Tao N, Lebrilla CB, Coisson J-D, Arlorio M, German JB. Permeate from cheese whey ultrafiltration is a source of milk oligosaccharides. Int Dairy J. (2009) 19:524–30. doi: 10.1016/j.idairyj.2009.03.008
33. Yang S-T. Chapter 1. Bioprocessing - from biotechnology to biorefinery. In: Yang S-T, editor. Bioprocessing for Value-Added Products from Renewable Resources: New Technologies and Applications. Amsterdam: Elsevier (2007). p. 17–8.
34. Paterson AHJ. Lactose and oligosaccharides: Lactose: Production, applications. In: Fuquay JW, Fox PF, McSweeny PLH, editors. Encyclopedia of Dairy Sciences. Boston: Elsevier, (2011). p. 196–201.
35. de Moura Bell JMLN, Cohen JL, de Aquino LFMC, Lee H, de Melo Silva VL, Liu Y, et al. An integrated bioprocess to recover bovine milk oligosaccharides from colostrum whey permeate. J Food Eng. (2018) 216:27–35. doi: 10.1016/j.jfoodeng.2017.07.022
36. Cohen JL, Barile D, Liu Y, de Moura Bell JM. Role of pH in the recovery of bovine milk oligosaccharides from colostrum whey permeate by nanofiltration. Int Dairy J. (2017) 66:68–75. doi: 10.1016/j.idairyj.2016.10.016
37. Factory. Available online at: http://www.publicdomainfiles.com/show_file.php?id=13525908011871. Public domain photo (accessed February 7, 2019).
38. Robinson RC, Poulsen NA, Colet E, Duchene C, Larsen LB, Barile D. Profiling of aminoxyTMT-labeled bovine milk oligosaccharides reveals substantial variation in oligosaccharide abundance between dairy cattle breeds. Sci Rep. (2019) 9:5465. doi: 10.1038/s41598-019-41956-x
39. Liu Z, Wang T, Pryce JE, MacLeod IM, Hayes BJ, Chamberlain AJ, et al. Fine-mapping sequence mutations with a major effect on oligosaccharide content in bovine milk. Sci Rep. (2019) 9:2137. doi: 10.1038/s41598-019-38488-9
40. Kunz C, Rudloff S, Baier W, Klein N, Strobel S. Oligosaccharides in human milk: structural, functional, and metabolic aspects. Annu Rev Nutr. (2000) 20:699–722. doi: 10.1146/annurev.nutr.20.1.699
41. Engfer MB, Stahl B, Finke B, Sawatzki G, Daniel H. Human milk oligosaccharides are resistant to enzymatic hydrolysis in the upper gastrointestinal tract. Am J Clin Nutr. (2000) 71:1589–96. doi: 10.1093/ajcn/71.6.1589
42. Gnoth MJ, Kunz C, Kinne-Saffran E, Rudloff S. Human milk oligosaccharides are minimally digested in vitro. J Nutr. (2000) 130:3014–20. doi: 10.1093/jn/130.12.3014
43. Ruhaak LR, Stroble C, Underwood MA, Lebrilla CB. Detection of milk oligosaccharides in plasma of infants. Anal Bioanal Chem. (2014) 406:5775–84. doi: 10.1007/s00216-014-8025-z
44. Leoz MLAD, Wu S, Strum JS, Niñonuevo MR, Gaerlan SC, Mirmiran M, et al. A quantitative and comprehensive method to analyze human milk oligosaccharide structures in the urine and feces of infants. Anal Bioanal Chem. (2013) 405:4089–105. doi: 10.1007/s00216-013-6817-1
45. Underwood MA, Gaerlan S, De Leoz MLA, Dimapasoc L, Kalanetra KM, Lemay DG, et al. Human milk oligosaccharides in premature infants: absorption, excretion, and influence on the intestinal microbiota. Pediatr Res. (2015) 78:670–7. doi: 10.1038/pr.2015.162
46. Rudloff S, Pohlentz G, Diekmann L, Egge H, Kunz C. Urinary excretion of lactose and oligosaccharides in preterm infants fed human milk or infant formula. Acta Paediatr. (1996) 85:598–603. doi: 10.1111/j.1651-2227.1996.tb14095.x
47. Lin AE, Autran CA, Espanola SD, Bode L, Nizet V. Human milk oligosaccharides protect bladder epithelial cells against uropathogenic Escherichia coli invasion and cytotoxicity. J Infect Dis. (2014) 209:389–98. doi: 10.1093/infdis/jit464
48. Jacobi SK, Yatsunenko T, Li D, Dasgupta S, Robert KY, Berg BM, et al. Dietary isomers of sialyllactose increase ganglioside sialic acid concentrations in the corpus callosum and cerebellum and modulate the colonic microbiota of formula-fed piglets. J Nutr. (2016) 146:200–8. doi: 10.3945/jn.115.220152
49. Wang B, Yu B, Karim M, Hu H, Sun Y, McGreevy P, et al. Dietary sialic acid supplementation improves learning and memory in piglets. Am J Clin Nutr. (2007) 85:561–9. doi: 10.1093/ajcn/85.2.561
50. Morgan BLG, Winick M. Effects of administration of N-acetylneuraminic acid (NANA) on brain NANA content and behavior. J Nutr. (1980) 110:416–24. doi: 10.1093/jn/110.3.416
51. Vázquez E, Barranco A, Ramírez M, Gruart A, Delgado-García JM, Martínez-Lara E, et al. Effects of a human milk oligosaccharide, 2′-fucosyllactose, on hippocampal long-term potentiation and learning capabilities in rodents. J Nutr Biochem. (2015) 26:455–65. doi: 10.1016/j.jnutbio.2014.11.016
52. Ruiz-Moyano S, Totten SM, Garrido DA, Smilowitz JT, German JB, Lebrilla CB, et al. Variation in consumption of human milk oligosaccharides by infant gut-associated strains of Bifidobacterium breve. Appl Environ Microbiol. (2013) 79:6040–9. doi: 10.1128/AEM.01843-13
53. Sela DA, Chapman J, Adeuya A, Kim JH, Chen F, Whitehead TR, et al. The genome sequence of Bifidobacterium longum subsp. infantis reveals adaptations for milk utilization within the infant microbiome. Proc Natl Acad Sci USA. (2008) 105:18964–9. doi: 10.1073/pnas.0809584105
54. Hamilton MK, Ronveaux CC, Rust BM, Newman JW, Hawley M, Barile D, et al. Prebiotic milk oligosaccharides prevent development of obese phenotype, impairment of gut permeability, and microbial dysbiosis in high fat-fed mice. Am J Physiol: Gastrointest Liver Physiol. (2017) 312:G474–87. doi: 10.1152/ajpgi.00427.2016
55. Jena PK, Sheng L, Nagar N, Wu C, Barile D, Mills DA, et al. Synbiotics Bifidobacterium infantis and milk oligosaccharides are effective in reversing cancer-prone nonalcoholic steatohepatitis using western diet-fed FXR knockout mouse models. J Nutr Biochem. (2018) 57:246–54. doi: 10.1016/j.jnutbio.2018.04.007
56. Scott KP, Martin JC, Duncan SH, Flint HJ. Prebiotic stimulation of human colonic butyrate-producing bacteria and bifidobacteria, in vitro. FEMS Microbiol Ecol. (2014) 87:30–40. doi: 10.1111/1574-6941.12186
57. Topping DL, Clifton PM. Short-chain fatty acids and human colonic function: roles of resistant starch and nonstarch polysaccharides. Physiol Rev. (2001) 81:1031–64. doi: 10.1152/physrev.2001.81.3.1031
58. Wong JMW, de Souza R, Kendall CWC, Emam A, Jenkins DJA. Colonic health: fermentation and short chain fatty acids. J Clin Gastroenterol. (2006) 40:235–43. doi: 10.1097/00004836-200603000-00015
59. Marcobal A, Barboza M, Sonnenburg ED, Pudlo N, Martens EC, Desai P, et al. Bacteroides in the infant gut consume milk oligosaccharides via mucus-utilization pathways. Cell Host Microbe. (2011) 10:507–14. doi: 10.1016/j.chom.2011.10.007
60. Samuel BS, Gordon JI. A humanized gnotobiotic mouse model of host-archaeal-bacterial mutualism. Proc Natl Acad Sci USA. (2006) 103:10011–6. doi: 10.1073/pnas.0602187103
61. Sheng L, Jena PK, Hu Y, Liu H-X, Nagar N, Kalanetra KM, et al. Hepatic inflammation caused by dysregulated bile acid synthesis is reversible by butyrate supplementation. J Pathol. (2017) 243:431–41. doi: 10.1002/path.4983
62. Charbonneau MR, O'Donnell D, Blanton LV, Totten SM, Davis JC, Barratt MJ, et al. Sialylated milk oligosaccharides promote microbiota-dependent growth in models of infant undernutrition. Cell. (2016) 164:859–71. doi: 10.1016/j.cell.2016.01.024
63. Obesity and overweight Fact Sheet. World Health Organization (2018). Available online at: https://www.who.int/en/news-room/fact-sheets/detail/obesity-and-overweight (accessed January 15, 2019).
64. Hamilton MK, Boudry G, Lemay DG, Raybould HE. Changes in intestinal barrier function and gut microbiota in high-fat diet-fed rats are dynamic and region dependent. Am J Physiol: Gastrointest Liver Physiol. (2015) 308:G840–51. doi: 10.1152/ajpgi.00029.2015
65. Cani PD, Rodrigo B, Knauf C, Waget A, Neyrinck AM, Delzenne NM, et al. Changes in gut microbiota control metabolic endotoxemia-induced inflammation in high-fat diet-induced obesity and diabetes in mice. Diabetes. (2008) 57:1470–81. doi: 10.2337/db07-1403
66. Ridaura VK, Faith JJ, Rey FE, Cheng J, Duncan AE, Kau AL, et al. Gut microbiota from twins discordant for obesity modulate metabolism in mice. Science. (2013) 341:1241214. doi: 10.1126/science.1241214
67. Turnbaugh PJ, Ley RE, Mahowald MA, Magrini V, Mardis ER, Gordon JI. An obesity-associated gut microbiome with increased capacity for energy harvest. Nature. (2006) 444:1027–31. doi: 10.1038/nature05414
68. Teixeira TFS, Collado MC, Ferreira CLLF, Bressan J, Peluzio M do CG. Potential mechanisms for the emerging link between obesity and increased intestinal permeability. Nutr Res. (2012) 32:637–47. doi: 10.1016/j.nutres.2012.07.003
69. Boudry G, Hamilton MK, Chichlowski M, Wickramasinghe S, Barile D, Kalanetra KM, et al. Bovine milk oligosaccharides decrease gut permeability and improve inflammation and microbial dysbiosis in diet-induced obese mice. J Dairy Sci. (2017) 100:2471–81. doi: 10.3168/jds.2016-11890
70. Cani PD, Neyrinck AM, Fava F, Knauf C, Burcelin RG, Tuohy KM, et al. Selective increases of bifidobacteria in gut microflora improve high-fat-diet-induced diabetes in mice through a mechanism associated with endotoxaemia. Diabetologia. (2007) 50:2374–83. doi: 10.1007/s00125-007-0791-0
71. Griffiths EA, Duffy LC, Schanbacher FL, Qiao H, Dryja D, Leavens A, et al. In vivo effects of bifidobacteria and lactoferrin on gut endotoxin concentration and mucosal immunity in Balb/c mice. Dig Dis Sci. (2004) 49:579–89. doi: 10.1023/B:DDAS.0000026302.92898.ae
72. Jena PK, Sheng L, Liu H-X, Kalanetra KM, Mirsoian A, Murphy WJ, et al. Western diet–induced dysbiosis in farnesoid X receptor knockout mice causes persistent hepatic inflammation after antibiotic treatment. Am J Pathol. (2017) 187:1800–13. doi: 10.1016/j.ajpath.2017.04.019
73. Gerber Good Start Gentle (HMO) Powder Infant Formula. Available online at: https://www.gerber.com/gerber-good-start-gentle-powder-formula (accessed February 5, 2019).
74. Similac Pro-Advance. Available online at: https://similac.com/baby-formula/pro-advance (accessed February 5, 2019).
75. Chen X. Chapter four - Human milk oligosaccharides (HMOS): Structure, function, and enzyme-catalyzed synthesis. In: Baker DC, Horton D, editors. Advances in Carbohydrate Chemistry and Biochemistry. Waltham, MA: Academic Press (2015). p. 113–90.
76. Smilowitz JT, Lemay DG, Kalanetra KM, Chin EL, Zivkovic AM, Breck MA, et al. Tolerability and safety of the intake of bovine milk oligosaccharides extracted from cheese whey in healthy human adults. J Nutr Sci. (2017) 6:e6. doi: 10.1017/jns.2017.2
Keywords: milk, prebiotic, obesity, mass spectrometry, therapeutic
Citation: Robinson RC (2019) Structures and Metabolic Properties of Bovine Milk Oligosaccharides and Their Potential in the Development of Novel Therapeutics. Front. Nutr. 6:50. doi: 10.3389/fnut.2019.00050
Received: 27 February 2019; Accepted: 04 April 2019;
Published: 24 April 2019.
Edited by:
Oswaldo Hernandez-Hernandez, Instituto de Investigación en Ciencias de la Alimentación (CIAL), SpainReviewed by:
Vasiliki Kachrimanidou, University of Reading, United KingdomCopyright © 2019 Robinson. This is an open-access article distributed under the terms of the Creative Commons Attribution License (CC BY). The use, distribution or reproduction in other forums is permitted, provided the original author(s) and the copyright owner(s) are credited and that the original publication in this journal is cited, in accordance with accepted academic practice. No use, distribution or reproduction is permitted which does not comply with these terms.
*Correspondence: Randall C. Robinson, cmNyb2JpbnNvbkB1Y2RhdmlzLmVkdQ==
Disclaimer: All claims expressed in this article are solely those of the authors and do not necessarily represent those of their affiliated organizations, or those of the publisher, the editors and the reviewers. Any product that may be evaluated in this article or claim that may be made by its manufacturer is not guaranteed or endorsed by the publisher.
Research integrity at Frontiers
Learn more about the work of our research integrity team to safeguard the quality of each article we publish.