- 1Clore Laboratory, University of Buckingham, Buckingham, United Kingdom
- 2Obesity Biology Unit, University of Liverpool, Liverpool, United Kingdom
Gaseous oxygen is essential for all aerobic animals, without which mitochondrial respiration and oxidative phosphorylation cannot take place. It is not, however, regarded as a “nutrient” by nutritionists and does not feature as such within the discipline of nutritional science. This is primarily a consequence of the route by which O2 enters the body, which is via the nose and lungs in terrestrial animals as opposed to the mouth and gastrointestinal tract for what are customarily considered as nutrients. It is argued that the route of entry should not be the critical factor in defining whether a substance is, or is not, a nutrient. Indeed, O2 unambiguously meets the standard dictionary definitions of a nutrient, such as “a substance that provides nourishment for the maintenance of life and for growth” (Oxford English Dictionary). O2 is generally available in abundance, but deficiency occurs at high altitude and during deep sea dives, as well as in lung diseases. These impact on the provision at a whole-body level, but a low pO2 is characteristic of specific tissues includings the retina and brain, while deficiency, or overt hypoxia, is evident in certain conditions such as ischaemic disease and in tumours - and in white adipose tissue in obesity. Hypoxia results in a switch from oxidative metabolism to increased glucose utilisation through anaerobic glycolysis, and there are extensive changes in the expression of multiple genes in O2-deficient cells. These changes are driven by hypoxia-sensitive transcription factors, particularly hypoxia-inducible factor-1 (HIF-1). O2 deficiency at a whole-body level can be treated by therapy or supplementation, but O2 is also toxic through the generation of reactive oxygen species. It is concluded that O2 is a critical, but overlooked, nutrient which should be considered as part of the landscape of nutritional science.
Introduction
There is a general understanding of what constitutes the field of nutritional science. At its core, the discipline encompasses the provision of macro- and micro-nutrients, how they are processed in order to meet the requirements of the individual (whether humans or other species) for maintenance—and at certain points in the life cycle, for growth and development. From a biomedical perspective, the focus is with the consequences of under– or over-provision with regard to health and the prevention of disease. Most nutritional scientists understand macronutrients to mean proteins, carbohydrates, and lipids, each of which include multiple molecular entities. Alcohol may also be classified as a macronutrient, and in some cultures makes a significant contribution to total energy intake.
There are, however, two major nutrients which are essentially, or entirely, ignored in nutrition, not least in textbooks of the subject, and water is the most obvious example. Water is, of course, contained within most foods and is released during digestion; it is also obtained as a by-product of multiple metabolic processes. There is nonetheless, a distinct requirement for additional water, particularly in hot environments and in situations such as during extreme exertion when the losses are high. Indeed, the provision of (clean) water for direct consumption is a critical determinant of the health and survival of man and other animals. Water is consumed as such both during and between meals, and enters the body in a manner similar to that of other nutrients.
The other forgotten, though quantitatively major nutrient, is diatomic oxygen. O2 has, however, rarely been considered as a nutrient in the customary sense of the term, certainly in the case of humans and other higher animals; this is despite earlier arguments that it should be so considered, though the proponents were equivocal (1). Indeed, its classification as a nutrient would be regarded as controversial by many nutritionists. In the present article, the proposition that O2 is unambiguously a nutrient (1, 2) and should be included within the landscape of nutritional science is developed.
Definitions—What Is a Nutrient?
Whether O2, a gas, is a macro (in the sense of quantity) nutrient and part of the remit of nutrition depends, of course, on the meaning of the word “nutrient”. There is a commonplace sense of what constitutes a nutrient—as something that is eaten within food and which is necessary for bodily maintenance and good health. However, dictionaries have, as would be expected, clear definitions of the term. In the Oxford Dictionary of English, 2nd edition (2005)—the Oxford dictionaries being the “bible” of the English language—a nutrient is defined as “a substance that provides nourishment for the maintenance of life and for growth”. Its American equivalent, Webster's, is perhaps even more precise in its definition, a nutrient being “any substance or matter that is needed for the life and growth of living things”. Other dictionaries offer similar definitions (e.g., Collins English Dictionary: “any substance that nourishes an animal”), although the word “food” is sometimes included by those that are medical in focus.
It is clear from these definitions that O2, as well as water, is unambiguously a nutrient. In the case of water, its inclusion would not be considered controversial, given that it is consumed via the mouth and is absorbed from the gastrointestinal tract similar to those substances that are classically regarded as nutrients. The provision of O2 is, of course, fundamentally different in that it is obtained from the ambient air as a gas and delivered through the nose and lungs (or gills in the case of aquatic animals). It is this route of entry into the body which is the primary reason why O2 is not regarded as a nutrient in humans and other higher animals, and is absent from nutritional discourse. Indeed, reference to O2 in a nutritional context is invariably limited to metabolic rate and energy expenditure with respect to energy balance and RQ (respiratory quotient).
It is argued that the actual route of entry—gastrointestinal tract or lungs/gills—should not be considered a defining characteristic of a substance as a nutrient, but rather the functions and essentiality as delineated in the dictionary definitions above (Table 1). With considerable insight, the Polish alchemist Michael Sendivogius wrote as far back as 1604 that “Man was created of the Earth, and lives by virtue of the air; for there is in the air a secret food of life….” (my italics) (3). This was, of course, well-before the formal discovery of O2, though Sendivogius in effect hypothesised its existence. The discovery of O2 as such in the latter half of the 18th century is variously credited to the English chemist Joseph Priestley, the Swedish apothecary Carl Scheele, and the French chemist Antoine Lavoisier, each contributing in different respects.
The word oxygen is derived from the Greek for “acid-former” (oxys—sharp, sour and genes—producer), which referred to the mistaken view that it was necessary for the formation of acids. The name was first coined by Lavoisier (French, oxygène) who showed that combustion was the reaction of O2 with carbon and other substances.
Oxygen and the Origins of Life
It is an axiom that life on Earth as we know it is dependent on the presence of an atmosphere containing O2. Gaseous oxygen has been detected elsewhere in the universe, its presence in the atmosphere of distant exoplanets providing a potential biomarker for life (4–6). When the Earth first formed 4.54 billion years ago through accretion from the solar nebula, the primordial atmosphere was devoid of O2—there was no free O2 (7, 8). The diatomic oxygen that was present was essentially locked up in rocks and in water, and some 4 billion years ago the atmosphere was thought to have contained O2 at just one part in a million (8, 9). The earliest single celled organisms existed under anoxic conditions and were superseded by cyanobacteria, which appeared up to 3 billion years or more ago—and, importantly, generated O2 through photosynthesis (9). Microorganisms, such as those harboured in deep sea hydrothermal vents, are able to survive, indeed flourish, under anoxic conditions through sulphur respiration—anaerobic respiration with sulphur (10, 11).
Approximately 2.45 billion years ago O2 began to appear in the atmosphere in significant quantities at what is termed the “Great Oxidation Event” (actually a protracted process) (9, 12, 13). O2 levels gradually increased, and probably then fell, until around 700 million years ago when a further sharp increase occurred (12). The level of O2 in the atmosphere is believed to have hovered at around the current 21% since ~600 million years ago (9). While O2 can be toxic in many respects, as discussed in a later section, its rise was critical to the development of multi-cellular organisms and the physiological complexity that this implies. The earliest known fossils of a eukaryote, from which multi-cellular organisms evolved, date from at least 2 billion years ago (14). In eukaryotes and early multi-cellular organisms requiring O2, uptake occurs by direct transfer across the cell membrane in essentially the same manner as other nutrients prior to the development of specialised digestive and respiratory organs.
The ready availability of O2 had a profound effect on the metabolic opportunities for an organism and the consequent systems that developed. The mitochondrion, through respiration and oxidative phosphorylation, is a potent example of a cellular organelle whose evolution resulted in major new metabolic processes. The most widely accepted view on the origin of mitochondria is the endosymbiotic hypothesis which proposes that mitochondria were originally prokaryotic cells (14, 15). These prokaryotes were able to undertake oxidative processes that early eukaryotic cells could not perform, and they subsequently became endosymbionts living within the eukaryote cell structure.
Provision and Delivery of O2
Animals are constant metabolisers, whether they are poikilotherms or homeotherms, and this is so even in those species that undergo periods of hibernation, aestivation or torpor (albeit at a reduced rate of metabolism). However, most nutrients are obtained in higher animals on an intermittent basis, such species being periodic feeders—whether in the form of distinct meals or through frequent foraging. Foods, entering through the mouth, are generally complex structures and the nutrients that they contain are not immediately available. Instead, they require release through digestion and are subsequently absorbed from the gastrointestinal tract, a process that may involve specific transporters.
In simple organisms, O2 is obtained in a manner similar to that of other nutrients—by absorption across the cell membrane—while in complex organisms it is fundamentally different. The evolution of specialised organs has resulted in the development of a respiratory system for the delivery of O2, differentiating it sharply from the route by which all other nutrients are provided through the digestive system (Table 1). In marked contrast to nutrients obtained via the mouth and gastrointestinal tract, the requirement for O2 is virtually continuous in higher animals; as is well-recognised, the absence of O2 results in death within a very few minutes in humans and many other higher species. This reflects both the constant metabolic need for O2 together with the absence of any significant storage. There is some limited storage, however, in skeletal muscle for local use through binding to the iron-containing protein myoglobin, but this is primarily a feature of marine animals such as whales, which experience apnoea during diving and where the haem protein is present in relative abundance (16).
On entering the lungs, O2 passes into the alveoli which as highly vascularised sacs enable the rapid movement of the gas by simple diffusion, first across the alveolar epithelium and then the endothelial cells of the alveolar capillaries. Once in the circulation, O2 binds to haemoglobin in the erythrocytes and is immediately transported to tissues (17). Modifications to this route of entry occur through the presence of gills in aquatic species, while in lower animals simpler systems for obtaining O2 are evident.
The presence of haemoglobin as a specific carrier for O2 has some parallels with the transport and delivery of a number of other nutrients. There are selective carriers for the movement of nutrients from the lumina of the gastrointestinal tract—such as the Na+-dependent glucose transporter (SGLT1) to transfer glucose (18, 19), and several amino acid transporters to transfer various amino acids (19, 20). Once across the gastrointestinal wall, from mucosal to serosal side, nutrients move to their immediate sites of action or to storage organs for subsequent use. Storage occurs particularly in the liver and skeletal muscle for glucose as glycogen, and in white adipose tissue depots for the sequestration of fatty acids as triacylglycerols (19). In some cases, carrier proteins are involved in the transport of nutrients to their storage site, such as transferrin for the transport of iron to the bone marrow (21). Specific carriers, analogous to haemoglobin, also transport a number of nutrients to the tissues where they are required once released from storage, examples including retinol binding protein for retinol (21, 22) and plasma lipoproteins in the case of lipids (19, 23).
Metabolic Function of O2
Despite O2 not being recognised as a nutrient in the context of nutritional science and in relation to whole-body physiology, it is frequently described as such in studies at a cellular level [e.g., (1, 24)]. The central role of O2 as a nutrient is in mitochondrial respiration, acting as an electron acceptor thereby enabling ATP to be formed through oxidative phosphorylation. This process is fundamental to aerobic organisms, with the oxidation of glucose and fatty acids requiring the continuous provision of O2. Several core metabolic pathways are central to mitochondrial oxidative phosphorylation—glycolysis, glycogenolysis, lipolysis, and the tricarboxylic acid (Krebs) cycle (19).
There are differences between cell types in the number of mitochondria that are present, as well as in the relative development of their cristae structure, according to the level of respiration and oxidative phosphorylation that is functionally required. White adipocytes, for example, have moderate numbers of mitochondria which contain limited cristae, with most of the volume of these cells being due to the lipid droplet (25, 26). Brown adipocytes, in marked contrast, contain large numbers of mitochondria with a highly developed and dense cristae structure, especially in rodents adapted to cold environments when maximum non-shivering thermogenesis is required (25, 26). In these circumstances, brown fat mitochondria utilise substantial amounts of O2 in order to sustain the oxidation of fatty acids and other substrates at high rates, with ATP synthesis being bypassed through a proton leakage pathway regulated by UCP1 (uncoupling protein-1) (27).
Deficiency of Oxygen
The partial pressure of O2 is highest at sea level, but falls with altitude leading to a decrease in the amount available. Altitude is one of the several environmental situations that result in a reduction in the availability of O2. Animals, including humans, that habitually live at high elevations have evolved distinct physiological adaptations which allow them to adapt to the relatively hypoxic conditions. Another environmental circumstance in which O2 deprivation occurs, albeit on a short-term basis, is that experienced by aquatic mammals such as whales during deep sea dives.
Even at sea level, a marked periodic lack of O2 is also evident in certain terrestrial species according to their precise ecological niche. Naked mole-rats (Heterocephalus glaber) are a potent example, these animals experiencing near anoxic conditions during prolonged periods in their subterranean burrows (28). The ability of naked mole-rats to withstand sustained anoxia is suggested as being due to the utilisation of fructose as a fuel for glycolysis, through high levels of the GLUT5 fructose transporter and of ketohexokinase, enabling the key glycolytic regulatory enzyme phosphofructokinase to be bypassed (28). Some ectothermic vertebrates, such as the American freshwater turtle and crucian carps, exhibit extreme capacities to withstand a low O2 tension, being able to survive for months under what is effectively complete anoxia (29).
These examples of O2 deprivation relate to specific environmental and ecological conditions to which particular species are exposed and effect provision of the macronutrient in whole-animal terms. A fall in the availability of O2 to the body as a whole can also occur in certain disease states, primarily disorders of the lungs (30). There are several major human lung diseases, some of which are often given the collective name of chronic obstructive pulmonary disease, that lead to impairments in O2 delivery; these include emphysema, pulmonary fibrosis and cystic fibrosis (31). Such conditions result in a chronic insufficiency of O2, but there are also situations in which there is an acute, periodic lack as in obstructive sleep apnoea (32).
The O2 tension of inspired air at sea level is 160 mmHg (21.3 kPa) while in alveolar blood it is around 104 mmHg (13.9 kPa), the level at which it leaves the lungs (7). The general level of tissue oxygenation is rather lower at some 40–50 mmHg (5.33–6.67 kPa); thus, even the “normal” tissue pO2 is in effect hypoxic relative to alveolar blood and ambient air at sea level (7, 33). However, a substantially lower O2 tension is evident in several tissues, including the spleen, thymus, retina and regions of the brain (which is a substantial consumer of O2) with a pO2 of 16 (2.13 kPa), 10 (1.33 kPa), 2–25 (0.27–3.33 kPa) and 0.4–8 mmHg (0.05–1.07 kPa), respectively (see Table 2) (34–36). Tumours are also markedly hypoxic, with pO2 values ranging from 1 to 10 mmHg (0.13–1.13 kPa) - and the centre of solid tumours may be essentially anoxic (7, 33, 34). These examples of low pO2 in certain tissues generally reflect limited vascularisation, as in the retina and in tumours, and the consequent distance that O2 has to travel to the cells where it is required. The diffusion distance of O2 is generally considered to be of the order of 100–200 μm – the size of large white adipocytes (38) - but there are reports of a pO2 close to zero at only 100 μm from the capillaries (7, 39).
There are other pathological conditions, in addition to tumours, in which overt tissue hypoxia is evident. These include psoriasis and ischaemic disorders, and at the site of wounds during healing (7, 40, 41). A further example is with white adipose tissue depots in obesity, at least in rodents (41). The pO2 of white fat in lean mice is around 45–50 mmHg (6.00–6.67 kPa), similar to the general level of tissue oxygenation, but in obese mice it is between 2- and 3.5-fold lower at ~15 mmHg (2.00 kPa), as assessed with needle-type fibre optic O2 sensors (37, 42–44). This reduction is evident in genetically obese (ob/ob) mice and in mice made obese through the provision of a high fat diet (37, 42, 43, 45). The functional implications of this marked reduction in pO2 in white fat depots in obesity is considered in a later section.
Cellular and Molecular Adaptations to Oxygen Deficiency
Cells have highly developed systems for detecting O2 levels and for adapting to deficiencies. O2 is sensed at the cell membrane by specific ion channels, particularly K+ channels (46–48). Within the cell, there are a series of transcription factors which are responsive to O2 and which drive the molecular adaptations to hypoxia. These include NFκB and CREB (cAMP response element-binding protein) (49), but the central transcription factors sensitive to hypoxia are the HIFs (hypoxia-inducible factors). There are three HIFs, of which HIF-1 is considered the most significant—and certainly the most widely studied (50–52).
HIF-1 is a heterodimer, the subunits being HIF-1α and HIF-1β. HIF-1β is constitutively expressed, and although HIF-1α is constantly synthesised it is rapidly degraded under normal cellular levels of O2 (7, 52–54). The mechanism by which HIF-1α is degraded has been extensively studied; in essence, degradation is through the 26S proteosomal system and involves the hydroxylation of HIF-1α. The HIF-1α subunit is stabilised when the O2 level is low, enabling the functional dimeric HIF-1 transcription factor to be produced.
The other HIFs - HIF-2 and HIF-3 - are formed by the dimerisation of HIF-1β with HIF-2α and HIF-3α, respectively (52, 55). These HIFs appear to be more limited in their action than HIF-1, particularly HIF-3, and are more tissue specific. HIF-1 regulates the transcription of in excess of 100 genes, these encoding proteins involved in several distinct cellular and metabolic systems (24, 40, 53). Importantly, enzymes and other proteins associated with glucose utilisation and glycolysis are regulated by HIF-1. Amongst the genes related to glycolysis that are up-regulated in response to hypoxia, though not necessarily by a HIF-1 dependent mechanism, are GPI (glucose-6-phosphate isomerase), HK2 (hexokinase 2) and PFKP (phosphofructokinse platelet). There is also up-regulation by hypoxia of the expression of the gene encoding GLUT1, the facilitative glucose transporter responsible for basal glucose uptake, and this is widely used as a molecular marker of the cellular response to low pO2(40, 41).
Increases in the expression of genes associated with glucose uptake and utilisation reflect the augmentation of anaerobic glycolysis that occurs under conditions of low pO2. One of the consequences of greater glycolysis in hypoxia is a rise in the production of lactate, associated with increased expression of lactate transporters, MCT1 in particular in the case of adipocytes for example (56). There are also parallel changes in the expression of genes encoding mitochondrial enzymes and other proteins involved in respiration and oxidative phosphorylation, consequent to the reduction of these processes (57, 58). Again using white adipocytes as an example, the expression of genes such as ATP5D (ATP synthase, H+ transporting, mitochondrial F1 complex, delta subunit) and COX4I1 (cytochrome c oxidase subunit 4, isoform 1) are down-regulated in hypoxia (41, 58).
Oxygen Deficiency: The Example of White Adipose Tissue
In addition to changes in glucose utilisation, oxidative phosphorylation, and lipid oxidation, exposure to a low pO2 leads to alterations in the expression of multiple genes involved in several other pathways in white adipocytes, including cell death and cell-to-cell signalling and interaction (58, 59); indeed, the expression of ~1,300 genes is altered in adipocytes by hypoxia (58). These reflect general responses to hypoxic conditions, many of which are near universal, especially those linked to anaerobic glycolysis and oxidative phosphorylation. However, some of cellular and molecular adaptations to a low pO2 are specific to individual tissues, reflecting their particular physiological function.
A clear example comes from white adipose tissue (Figure 1), a tissue that has been a continuing focus in nutritional science. This was originally in relation to the storage of triacylglycerols as fuel, but subsequently as a consequence of the surge in the incidence of obesity. More recently, white adipose tissue has been recognised as a major endocrine and signalling organ, being implicated in a range of physiological functions—from the regulation of appetite and blood pressure to insulin sensitivity and the inflammatory response (41, 60). Much of this recent regulatory perspective on white fat centres on the multiplicity of protein factors—the adipokines—that are released by white adipocytes, and which number several hundreds (61). This has followed from the discovery of the hormone leptin, adipocytes being the major site of production of this pleiotropic endocrine factor (62).
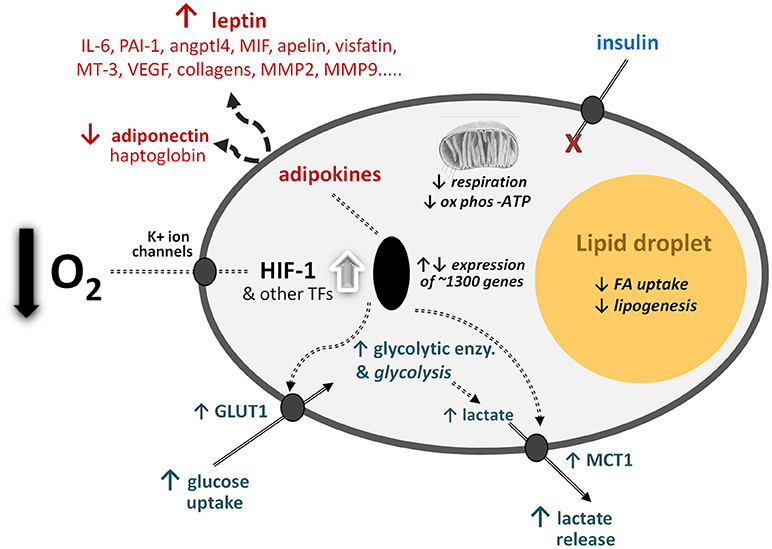
Figure 1. Schematic representation of some of the central cellular responses to hypoxia (oxygen deficiency) in white adipocytes. The figure illustrates adaptations that are universal to all cell types, particularly the increase in glucose utilisation through anaerobic glycolysis and the reduction in respiration and oxidative phosphorylation (ox phos). Adaptations that are specific to adipocytes are also shown, primarily those relating to lipid utilisation and the production of adipokines as key secretory proteins of these cell types; in some of the examples, such as MT-3 (metallothionein-3), only major changes at the gene expression level have been formally documented. angptl4, angiopoietin-like protein-4; enzy, enzyme; FA, fatty acid; GLUT1, facilitative glucose transporter 1; HIF-1, hypoxia-inducible factor-1; MCT1, monocarboxylate transporter-1; MIF, macrophage migration inhibitory factor; MMPs, matrix metalloproteinases; PAI-1, plasminogen activator inhibitor-1; TF, transcription factors (additional to HIF-1); VEGF, vascular endothelial growth factor. Adapted from (1).
Expression of the leptin (LEP) gene and secretion of the mature protein hormone are markedly stimulated by hypoxia (63), and indeed a low O2 tension induces preadipocytes to produce the hormone (64)—which they do not when incubated under so-called “normoxic” conditions (21% O2). Thus, exposure to O2 deficiency leads to a substantial increase in the production of a key adipocyte hormone. Another major adipocyte hormone, adiponectin, responds to hypoxia in the opposite direction, there being a reduction in the expression of the ADIPOQ gene and in the secretion of the encoded protein (63). This particular change would be expected to lead to a fall in insulin sensitivity and increased inflammation, given the insulin sensitising and anti-inflammatory actions of adiponectin (65–68).
Inflammation is one of the characteristics of adipose tissue as it enlarges in obesity, with recruitment of M1 macrophages and increased expression and release of a number of cytokines and chemokines, as well as of other inflammation-related factors (60, 69–71). Exposure of white adipocytes to hypoxic conditions, at least in cell culture, leads for example to increases in the expression of the IL6, VEGF, PAI1, MIF, MMP2, and MMP9 genes, in addition to the LEP gene, as part of the inflammatory response (45, 63, 72). Inflammation in adipose tissue, driven by hypoxia (41, 51), is considered to underpin the development of the major obesity-associated diseases, particularly insulin resistance, type 2 diabetes and the metabolic syndrome (73, 74).
Most in vitro and cell culture studies on adipocytes, and indeed of other types of cell, use a protocol in which the response to 1 or 2% O2 is compared to that of a reference level of 21% O2. As noted previously, this represents an extreme with 21% O2 being in effect hyperoxia while 1% O2 is at the bottom end of normal tissue oxygenation. Despite being an extreme, it can be justified by enabling “proof of principle” to be explored, even though it may exaggerate the scale of the response to O2 deprivation. Studies in which human adipocytes are incubated with graded levels of O2 between 21 and 1% indicate that indeed the response is exaggerated (75). They also demonstrate that there is not a critical threshold at which adaptive changes to low pO2 occur, but rather that there is a graded “dose response” (75). This is true for a range of processes, including glucose uptake and lactate release, and to the expression and secretion of leptin, VEGF and other adipokines (75). Thus, adipocytes titre small changes in O2 tension (provision) across a range of metabolic systems and processes, and this is also likely to occur in other cell types.
Oxygen Therapy and Toxicity
When there is a deficiency of O2, amelioration is possible through increasing its provision by O2 therapy, or supplementation. O2 therapy has its origins in the Pneumatic Institution founded in Bristol (UK) in 1799 by Thomas Beddoes, the aim of which was to explore the potential efficacy of the gas for the treatment of disease (76). Treatment now may be acute, as in medical emergencies such as for resuscitation, trauma and anaphylaxis, or chronic as in lung diseases, including chronic obstructive pulmonary disease and emphysema (30). Supplemental O2 is also utilised by mountaineers at high altitudes and in aircraft in the event of a fall in cabin pressure. These are, of course, relatively extreme conditions, and this is also the case with hyperbaric O2 therapy to treat decompression sickness in deep-sea divers.
There is a Janus-faced dimension to O2 in that in addition to being essential, it is toxic under certain conditions. For example, giving O2 to newborn premature babies can lead to blindness (77, 78), and O2 toxicity is a well-recognised risk of deep diving. At a molecular level, O2 is toxic through the formation of reactive oxygen species (ROS), these including peroxides, singlet oxygen, hydroxy radical and superoxide (79, 80). Although ROS are produced during normal metabolic functions involving O2, and have important signalling roles, their accumulation leads to cell damage and oxidative stress (79). Antioxidant defence mechanisms detoxify ROS, and these encompass enzymatic systems, in particular the superoxide dismutases, catalase and glutathione peroxidases(79, 80).
Recommended ‘Dietary’ Allowance
The concept of the recommended dietary allowance (RDA) is based on the need to set standards for the intake of nutrients, and this has been a continuing concern for national agencies and for Governments. The terminology associated with the general concept has, however, become somewhat complex with different countries adopting differing terms—“dietary reference value” in the UK, “population reference intake” elsewhere in the European Union, and “dietary reference intake” in the USA and Canada, with many other countries still using RDA (81). Whichever term is employed, standards are available for almost all nutrients, including water.
The concept of a RDA (or equivalent) would appear problematic for O2 – not least because of the word “diet” in the term—and it is appropriate to ask whether in this case it is relevant. Under most circumstances O2 is freely available and essentially unlimited (with the exceptions described above), and is obtained without financial cost. Intake is tightly controlled by the respiratory system and is closely calibrated to requirements. It can be argued that in adults the “RDA” for O2 is determined essentially by the need to maintain energy balance so that intake and expenditure are equal, especially in those of normal body weight. This will depend, of course, on the overall dietary composition in terms of other macronutrients and whether lipids, proteins, or carbohydrates are being oxidised. It will also depend on the level of physical activity as well as the stage in the life cycle, the requirement being higher as with other nutrients during growth, pregnancy and lactation.
Conclusions
Gaseous oxygen is not framed as a nutrient in nutritional science (1), despite being essential for all aerobic organisms. This primarily reflects the route of entry—the nose and lungs (or gills), rather than the mouth and gastrointestinal tract which is the path by which all other nutrients enter the body in higher animals. In very simple organisms, there is no fundamental difference in the mechanism by which O2 and other nutrients are taken up, differentiation occurring only following the evolution of specialised systems for digestion and respiration. At the cellular level in higher animals, there is also no essential distinction between O2 and what are conventionally considered as nutrients.
Deficiency of O2 on a whole-body basis is apparent in a number of situations, relating either to the specific environmental niche or to particular disease states. Individual tissues may experience a reduced pO2–relative hypoxia—either as part of the physiological milieu to which they are customarily exposed, or as a response to specific diseases. In practise, most tissues are relatively hypoxic, and certainly in relation to the O2 tension in alveolar blood. There are pervasive adaptations at a cellular level to reduced pO2, with a major switch in energy metabolism from mitochondrial respiration and oxidative phosphorylation to anaerobic glycolysis. This involves substantial shifts in the pattern of gene expression, much of which is driven by specific transcription factors, particularly HIF-1.
The concept of the RDA can be applied to O2, as with other nutrients, and toxicity is evident in certain circumstances. It is concluded that O2 is an important nutrient, and indeed an essential one, that is required in large quantities and which should be considered in conjunction with what are conventionally regarded as nutrients. As such, O2 should be firmly viewed as being part of the landscape of nutritional science.
Coda
In the 4 August 2017 issue of Science, a series of Japanese haikus on each element (“Elemental Haiku”) was presented by Mary Soon Lee (82). The haiku for oxygen encapsulates the critical biological importance of this element:
“Most of me is you.
I strive for independence,
Fail with every breath.”
Author Contributions
The author confirms being the sole contributor of this work and has approved it for publication.
Conflict of Interest Statement
The author declares that the research was conducted in the absence of any commercial or financial relationships that could be construed as a potential conflict of interest.
Acknowledgments
I am grateful to Professor Mark Gardner for directing me to some key references on oxygen.
References
1. Forster RE, Estabrook RW. Is oxygen an essential nutrient? Annu Rev Nutr. (1993) 13:383–403. doi: 10.1146/annurev.nu.13.070193.002123
2. Trayhurn P. Oxygen – the forgotten nutrient. J Nutr Sci. (2017) 6:e47 1–4. doi: 10.1017/jns.2017.53
4. Tian F, France K, Linsky JL, Mauas PJD, Vieytes MC. High stellar FUV/NUV ratio and oxygen contents in the atmospheres of potentially habitable planets. Earth Planet Sci Lett. (2014) 385:22–7. doi: 10.1016/j.epsl.2013.10.024
5. Luger R, Barnes R. Extreme water loss and abiotic O2 buildup on planets throughout the habitable zones of M Dwarfs. Astrobiol (2015) 15:119–43. doi: 10.1089/ast.2014.1231
6. Narita N, Enomoto T, Masaoka S, Kusakabe N. Titania may produce abiotic oxygen atmospheres on habitable exoplanets. Sci Rep. (2015) 5:13977. doi: 10.1038/srep13977
7. Brahimi-Horn MC, Pouysségur J. Oxygen, a source of life and stress. Febs Lett. (2007) 581:3582-91. doi: 10.1016/j.febslet.2007.06.018
9. Lyons TW, Reinhard CT, Planavsky NJ. The rise of oxygen in Earth's early ocean and atmosphere. Nature (2014) 506:307–15. doi: 10.1038/nature13068
10. Yamamoto M, Takai K. Sulfur metabolisms in epsilon- and gamma-proteobacteria in deep-sea hydrothermal fields. Front Microbiol. (2011) 2:192. doi: 10.3389/fmicb.2011.00192
11. Dick G, Anantharaman K, Baker B, Li M, Reed D, Sheik C. The microbiology of deep-sea hydrothermal vent plumes: ecological and biogeographic linkages to seafloor and water column habitats. Front Microbiol. (2013) 4:124. doi: 10.3389/fmicb.2013.00124
12. Blaustein R. The great oxidation event. evolving understandings of how oxygenic life on earth began. Bioscience (2016) 66:189–95. doi: 10.1093/biosci/biv193
13. Gumsley AP, Chamberlain KR, Bleeker W, Söderlund U, de Kock MO, Larsson ER, et al. Timing and tempo of the great oxidation event. Proc Natl Acad Sci USA. (2017) 114:1811–6. doi: 10.1073/pnas.1608824114
15. Martin WF, Garg S, Zimorski V. Endosymbiotic theories for eukaryote origin. Phil Trans Roy Soc B Biol Sci. (2015) 370:20140330. doi: 10.1098/rstb.2014.0330
16. Ordway GA, Garry DJ. Myoglobin: an essential hemoprotein in striated muscle. J Exp Biol. (2004) 207:3441–6. doi: 10.1242/jeb.01172
17. Quaye IK. Extracellular hemoglobin: the case of a friend turned foe. Front Physiol. (2015) 6:96. doi: 10.3389/fphys.2015.00096
18. Lehmann A, Hornby PJ. Intestinal SGLT1 in metabolic health and disease. Am J Physiol Gastrointest Liver Physiol. (2016) 310:G887–98. doi: 10.1152/ajpgi.00068.2016
19. Frayn KN. Metabolic Regulation. A human Perspective. 3rd Edn. Chichester: Wiley-Blackwell (2010).
20. Bröer S. Amino acid transport across mammalian intestinal and renal epithelia. Physiol Rev. (2008) 88:249–86. doi: 10.1152/physrev.00018.2006
21. Gkouvatsos K, Papanikolaou G, Pantopoulos K. Regulation of iron transport and the role of transferrin. Biochim Biophys Acta (2012) 1820:188–202. doi: 10.1016/j.bbagen.2011.10.013
22. Blaner WS. Retinol-binding protein: the serum transport protein for vitamin A. Endocrine Rev. (1989) 10:308–16. doi: 10.1210/edrv-10-3-308
23. Olson RE. Discovery of the lipoproteins, their role in fat transport and their significance as risk factors. J Nutr. (1998) 128:439S−43S. doi: 10.1093/jn/128.2.439S
24. Nakazawa MS, Keith B, Simon MC. Oxygen availability and metabolic adaptations. Nat Rev Cancer (2016) 16:663–73. doi: 10.1038/nrc.2016.84
26. Cinti S. The adipose organ: morphological perspectives of adipose tissues. Proc Nutr Soc. (2001) 60:319–28. doi: 10.1079/PNS200192
27. Cannon B, Nedergaard J. Brown adipose tissue: function and physiological significance. Physiol Rev. (2004) 84:277–359. doi: 10.1152/physrev.00015.2003
28. Park TJ, Reznick J, Peterson BL, Blass G, Omerbasic D, Bennett NC, et al. Fructose-driven glycolysis supports anoxia resistance in the naked mole-rat. Science (2017) 356:307–11. doi: 10.1126/science.aab3896
29. Storz JF, McClelland GB. Rewiring metabolism under oxygen deprivation. Science (2017) 356:248–9. doi: 10.1126/science.aan1505
30. Qaseem A, Wilt TJ, Weinberger SE, Hanania NA, Criner G, van der Molen T, et al. Diagnosis and management of stable chronic obstructive pulmonary disease: a clinical practice guideline update from the American College of Physicians, American College of Chest Physicians, American Thoracic Society, and European Respiratory Society. Ann Intern Med. (2011) 155:179–91. doi: 10.7326/0003-4819-155-3-201108020-00008
31. Kulkarni T, O'Reilly P, Antony VB, Gaggar A, Thannickal VJ. Matrix remodeling in pulmonary fibrosis and emphysema. Am J Resp Cell Mol Biol. (2016) 54:751–60. doi: 10.1165/rcmb.2015-0166PS
32. Levy P, Bonsignore MR, Eckel J. Sleep, sleep-disordered breathing and metabolic consequences. Eur Respir J. (2009) 34:243–60. doi: 10.1183/09031936.00166808
33. Höpfl G, Ogunshola O, Gassmann M. HIFs and tumors–causes and consequences. Am J Physiol Reg Integr Comp Physiol. (2004) 286:R608–23. doi: 10.1152/ajpregu.00538.2003
34. Braun RD, Lanzen JL, Snyder SA, Dewhirst MW. Comparison of tumor and normal tissue oxygen tension measurements using oxylite or microelectrodes in rodents. Am J Physiol Heart Circ Physiol. (2001) 280:H2533–44. doi: 10.1152/ajpheart.2001.280.6.H2533
35. Yu DY, Cringle SJ. Retinal degeneration and local oxygen metabolism. Exp Eye Res. (2005) 80:745–51. doi: 10.1016/j.exer.2005.01.018
36. Erecinska M, Silver IA. Tissue oxygen tension and brain sensitivity to hypoxia. Respir Physiol (2001) 128:263–76. doi: 10.1016/S0034-5687(01)00306-1
37. Ye J, Gao Z, Yin J, He Q. Hypoxia is a potential risk factor for chronic inflammation and adiponectin reduction in adipose tissue of ob/ob and dietary obese mice. Am J Physiol Endocrinol Metab. (2007) 293:E1118–28. doi: 10.1152/ajpendo.00435.2007
38. Skurk T, Alberti-Huber C, Herder C, Hauner H. Relationship between adipocyte size and adipokine expression and secretion. J Clin Endocrinol Metab. (2007) 92:1023–33. doi: 10.1210/jc.2006-1055
39. Folkman J, Hahnfeldt P, Hlatky L. Cancer: looking outside the genome. Nat Rev Mol Cell Biol. (2000) 1:76–9. doi: 10.1038/35036100
40. Semenza GL. Targeting HIF-1 for cancer therapy. Nat Rev Cancer (2003) 3:721–32. doi: 10.1038/nrc1187
41. Trayhurn P. Hypoxia and adipose tissue function and dysfunction in obesity. Physiol Rev. (2013) 93:1–21. doi: 10.1152/physrev.00017.2012
42. Rausch ME, Weisberg SP, Vardhana P, Tortorielllo DV. Obesity in C57BL/6J mice is characterised by adipose tissue hypoxia and cytotoxic T-cell infiltration. Int J Obesity (2008) 32:451–63. doi: 10.1038/sj.ijo.0803744
43. Yin J, Gao Z, He Q, Zhou D, Guo Z, Ye J. Role of hypoxia in obesity-induced disorders of glucose and lipid metabolism in adipose tissue. Am J Physiol Endocrinol Metab. (2009) 296:E333–42. doi: 10.1152/ajpendo.90760.2008
44. Trayhurn P, Alomar SY. Oxygen deprivation and the cellular response to hypoxia in adipocytes - perspectives on white and brown adipose tissues in obesity. Front Endocrinol. (2015) 6:19. doi: 10.3389/fendo.2015.00019
45. Hosogai N, Fukuhara A, Oshima K, Miyata Y, Tanaka S, Segawa K, et al. Adipose tissue hypoxia in obesity and its impact on adipocytokine dysregulation. Diabetes (2007) 56:901–11. doi: 10.2337/db06-0911
46. López-Barneo J, Pardal R, Ortega-Saenz P. Cellular mechanism of oxygen sensing. Annu Rev Physiol. (2001) 63:259–87. doi: 10.1146/annurev.physiol.63.1.259
47. López-Barneo J, del Toro R, Levitsky KL, Chiara MD, Ortega-Saenz P. Regulation of oxygen sensing by ion channels. J Appl Physiol. (2004) 96:1187–95. doi: 10.1152/japplphysiol.00929.2003
48. Kemp PJ, Peers C. Oxygen sensing by ion channels. Essays Biochem. (2007) 43:77–90. doi: 10.1042/BSE0430077
49. Cummins EP, Taylor CT. Hypoxia-responsive transcription factors. Pflügers Arch Eur J Physiol. (2005) 450:363–71. doi: 10.1007/s00424-005-1413-7
50. Rocha S. Gene regulation under low oxygen: holding your breath for transcription. Trends Biochem Sci. (2007) 32:389–97. doi: 10.1016/j.tibs.2007.06.005
51. Trayhurn P, Wang B, Wood IS. Hypoxia in adipose tissue: a basis for the dysregulation of tissue function in obesity? Br J Nutr. (2008) 100:227–35. doi: 10.1017/S0007114508971282
52. Pugh CW. Modulation of the hypoxic response. Adv Exp Med Biol. (2016) 903:259–71. doi: 10.1007/978-1-4899-7678-9_18
54. Coleman ML, Ratcliffe PJ. Oxygen sensing and hypoxia-induced responses. Essays Biochem. (2007) 43:1–15. doi: 10.1042/BSE0430001
55. Duan C. Hypoxia-inducible factor 3 biology: complexities and emerging themes. Am J Physiol Cell Physiol. (2016) 310:C260–9. doi: 10.1152/ajpcell.00315.2015
56. Pérez de Heredia F, Wood IS, Trayhurn P. Hypoxia stimulates lactate release and modulates monocarboxylate transporter (MCT1, MCT2, and MCT4) expression in human adipocytes. Pflügers Arch Eur J Physiol. (2010) 459:509–18. doi: 10.1007/s00424-009-0750-3
57. Semenza GL. Oxygen-dependent regulation of mitochondrial respiration by hypoxia-inducible factor 1. Biochem J. (2007) 405:1–9. doi: 10.1042/BJ20070389
58. Mazzatti D, Lim F-L, O'Hara A, Wood IS, Trayhurn P. A microarray analysis of the hypoxia-induced modulation of gene expression in human adipocytes. Arch Physiol Biochem. (2012) 118:112–20. doi: 10.3109/13813455.2012.654611
59. Geiger G, Leiherer A, Muendlein A, Stark N, Geller-Rhomberg S, Saely CH, et al. Identification of hypoxia-induced genes in human SGBS adipocytes by microarray analysis. PLoS ONE (2011) 6:e26465. doi: 10.1371/journal.pone.0026465
60. Trayhurn P, Wood IS. Adipokines: inflammation and the pleiotropic role of white adipose tissue. Br J Nutr. (2004) 92:347–55. doi: 10.1079/BJN20041213
61. Dahlman I, Elsen M, Tennagels N, Korn M, Brockmann B, Sell H, et al. Functional annotation of the human fat cell secretome. Arch Physiol Biochem. (2012) 118:84–91. doi: 10.3109/13813455.2012.685745
62. Zhang Y, Proenca R, Maffei M, Barone M, Leopold L, Friedman JM. Positional cloning of the mouse obese gene and its human homologue. Nature (1994) 372:425–32. doi: 10.1038/372425a0
63. Wang B, Wood IS, Trayhurn P. Dysregulation of the expression and secretion of inflammation-related adipokines by hypoxia in human adipocytes. Pflügers Archiv Eur J Physiol. (2007) 455:479–92. doi: 10.1007/s00424-007-0301-8
64. Wang B, Wood IS, Trayhurn P. Hypoxia induces leptin gene expression and secretion in human preadipocytes: differential effects of hypoxia on adipokine expression by preadipocytes. J Endocrinol. (2008) 198:127–34. doi: 10.1677/joe-08-0156
65. Ouchi N, Kihara S, Arita Y, Maeda K, Kuriyama H, Okamoto Y, et al. Novel modulator for endothelial adhesion molecules - Adipocyte-derived plasma protein adiponectin. Circulation (1999) 100:2473-6.
66. Yokota T, Oritani K, Takahashi I, Ishikawa J, Matsuyama A, Ouchi N, et al. Adiponectin, a new member of the family of soluble defense collagens, negatively regulates the growth of myelomonocytic progenitors and the functions of macrophages. Blood (2000) 96:1723-32.
67. Berg AH, Combs TP, Du X, Brownlee M, Scherer PE. The adipocyte-secreted protein Acrp30 enhances hepatic insulin action. Nat Med. (2001) 7:947–53. doi: 10.1038/90992
68. Yamauchi T, Kamon J, Waki H, Terauchi Y, Kubota N, Hara K, et al. The fat-derived hormone adiponectin reverses insulin resistance associated with both lipoatrophy and obesity. Nat Med. (2001) 7:941–6. doi: 10.1038/90984
69. Weisberg SP, McCann D, Desai M, Rosenbaum M, Leibel RL, Ferrante AW, Jr. Obesity is associated with macrophage accumulation in adipose tissue. J Clin Invest. (2003) 112:1796–808. doi: 10.1172/JCI19246
70. Xu H, Barnes GT, Yang Q, Tan G, Yang D, Chou CJ, et al. Chronic inflammation in fat plays a crucial role in the development of obesity-related insulin resistance. J Clin Invest (2003) 112:1821–30. doi: 10.1172/JCI19451
71. Rajala MW, Scherer PE. The adipocyte - at the crossroads of energy homeostasis, inflammation, and atherosclerosis. Endocrinology (2003) 144:3765–73. doi: 10.1210/en.2003-0580
72. Lolmède K, Durand de Saint Front V, Galitzky J, Lafontan M, Bouloumié A. Effects of hypoxia on the expression of proangiogenic factors in differentiated 3T3-F442A adipocytes. Int J Obesity (2003) 27:1187–95. doi: 10.1038/sj.ijo.0802407
74. Blüher M. Adipose tissue dysfunction in obesity. Exp Clin Endocrinol Diabet. (2009) 117:241–50. doi: 10.1055/s-0029-1192044
75. Wood I, Stezhka T, Trayhurn P. Modulation of adipokine production, glucose uptake and lactate release in human adipocytes by small changes in oxygen tension. Pflügers Archiv Eur J Physiol. (2011) 462:469–77. doi: 10.1007/s00424-011-0985-7
76. Grainge C. Breath of life: the evolution of oxygen therapy. J Roy Soc Med. (2004) 97:489-93. doi: 10.1258/jrsm.97.10.489
77. Stenson BJ. Oxygen targets for preterm infants. Neonatology (2013) 103:341–5. doi: 10.1159/000349936
78. Hartnett ME, Lane RH. Effects of oxygen on the development and severity of retinopathy of prematurity. J Am Assoc Pediat Ophthalmol Strabismus (2013) 17:229–34. doi: 10.1016/j.jaapos.2012.12.155
79. Martindale JL, Holbrook NJ. Cellular response to oxidative stress: signaling for suicide and survival. J Cellular Physiol. (2002) 192:1–15. doi: 10.1002/jcp.10119
80. de Roos B, Duthie Garry G. Role of dietary pro-oxidants in the maintenance of health and resilience to oxidative stress. Mol Nutr Food Res. (2014) 59:1229–48. doi: 10.1002/mnfr.201400568
81. Younger K. Dietary reference standards. In: Introduction to Human Nutrition, 2nd Edn Chichester: Wiley-Blackwell (2009), pp 122–31.
Keywords: adipocyte, adipose tissue, hypoxia, hypoxia-inducible factor-1 (HIF-1), mitochondria, oxygen deficiency, respiration
Citation: Trayhurn P (2019) Oxygen—A Critical, but Overlooked, Nutrient. Front. Nutr. 6:10. doi: 10.3389/fnut.2019.00010
Received: 20 June 2018; Accepted: 21 January 2019;
Published: 12 February 2019.
Edited by:
Rakesh Bhardwaj, National Bureau of Plant Genetic Resources (ICAR), IndiaReviewed by:
Arivalagan Manivannan, Indian Institute of Horticultural Research (ICAR), IndiaKathleen L. Hefferon, Cornell University, United States
Copyright © 2019 Trayhurn. This is an open-access article distributed under the terms of the Creative Commons Attribution License (CC BY). The use, distribution or reproduction in other forums is permitted, provided the original author(s) and the copyright owner(s) are credited and that the original publication in this journal is cited, in accordance with accepted academic practice. No use, distribution or reproduction is permitted which does not comply with these terms.
*Correspondence: Paul Trayhurn, cC50cmF5aHVybkBsaXZlcnBvb2wuYWMudWs=