- 1NIBIO, Norwegian Institute of Bioeconomy Research, Ås, Norway
- 2Department of Environmental Sciences, Norwegian University of Life Sciences, Ås, Norway
- 3Institute for Agricultural Sciences, ETH Zürich, Lindau, Switzerland
Minable rock phosphate is a finite resource. Replacing mineral phosphorus (P) fertilizer with P-rich secondary resources is one way to manage P more efficiently, but the importance of physicochemical and microbial soil processes induced by secondary resources for plant P uptake is still poorly understood. Using radioactive-labeling techniques, the fertilization effects of dairy manure, fish sludge, meat bone meal, and wood ash were studied as P uptake by barley after 44 days and compared with those of water-soluble mineral P (MinP) and an unfertilized control (NoP) in a pot experiment with an agricultural soil containing little available P at two soil pH levels, approximately pH 5.3 (unlimed soil) and pH 6.2 (limed soil). In a parallel incubation experiment, the effects of the secondary resources on physicochemical and microbial soil processes were studied. The results showed that the relative agronomic efficiency compared with MinP decreased in the order: manure ≥fish sludge ≥wood ash ≥meat bone meal. The solubility of inorganic P in secondary resources was the main driver for P uptake by barley (Hordeum vulgare). The effects of secondary resources on physicochemical and microbial soil processes were of little overall importance. Application of organic carbon with manure resulted in microbial P immobilization and decreased uptake by barley of P derived from the soil. On both soils, P uptake by barley was best explained by a positive linear relationship with the H2O + NaHCO3-soluble inorganic P fraction in fertilizers or by a linear negative relationship with the HCl-soluble inorganic P fraction in fertilizers.
Introduction
Minable rock phosphate is a finite resource. However, industrialized agroecosystems are today far from managing phosphorus (P) efficiently and Europe’s food production is largely dependent on imports of mined rock phosphate (1). The greatest reductions in P imports could be achieved by replacing mineral fertilizer with recycled P from secondary resources (2). In food systems, the accumulated P content in secondary resources is often of the same order of magnitude as that in mineral fertilizer, as shown for Europe (3). In Norway, the total amount of P in secondary resources (27,700 Mg P year−1) actually greatly exceeds the amount of P applied to soil with mineral fertilizer (8400 Mg P year−1) and the amount of P removed by crops (11,000 Mg P year−1) (4). The Norwegian secondary resources containing the largest amounts of P are manure (11,000 Mg P year−1), fish excrement, and feed losses from salmon and trout farming in open cages in fjords (fish sludge, 9000 Mg P year−1), meat bone meal (2100 Mg P year−1), and sewage (3100 Mg P year−1) (4). Wood ash, a residue from bioenergy plants and industrial timber production, also contains considerable amounts of secondary P (800 Mg P year−1) (5).
The P recycling potential of secondary resources is determined by, among other parameters, the solubility of the P species they contain (6, 7). Phosphorus in secondary resources is generally present as a complex mixture of inorganic P species, predominantly calcium (Ca) phosphates with differing solubility but also amorphous aluminum (Al)- or iron (Fe)-bound P, while organic P usually represents a small fraction (8). The P fertilization effects of secondary resources can be considerably affected by the pH in the target soil (7), as the solubility of Ca phosphates decreases with increasing soil pH, whereas the solubility of Al-/Fe-bound P decreases with decreasing soil pH (9). The best method for predicting the P fertilization effects of secondary resources also depends on the pH in the target soil (10). In a previous bioassay with ryegrass (Lolium multiflorum) grown in a sand–peat substrate containing little available P, the apparent P use efficiency of nine different secondary resources with predominantly Ca-bound P was best explained by a positive relationship with H2O-soluble inorganic P in acid soil and a negative relationship with HCl-soluble inorganic P in a near-neutral soil (7).
Plant P uptake following secondary resource application can also be influenced by their effects on microbial or physicochemical processes in the soil. Many secondary resources contain organic matter, meaning that organic carbon (C) is applied to the soil when they are used as alternatives to mineral fertilizers (e.g., manure, fish sludge, and meat bone meal). Organic C application can trigger microbial activity, which may result in immobilization of soil P and fertilizer P (11, 12) and in microbes competing with plants for available P. Microbial activity can also increase P availability by affecting physicochemical processes. Low molecular weight organic acids excreted by microorganisms during the breakdown of organic C have been shown to reduce phosphate retention on soil particles (13). Furthermore, these acids can solubilize P by complexing metal cations such as Al, Fe, and Ca that associate with P in insoluble forms, or by decreasing soil pH (14). Other secondary resources contain inorganic C, for example, in the form of calcium carbonate (CaCO3) (e.g., wood ash), which neutralizes pH in acid soils. Moreover, the solubility of applied fertilizer P can affect physicochemical soil processes depending on equilibrium processes in the target soil, since increased phosphate concentration in the soil solution can in turn result in reduced phosphate release from the soil (15).
To date, the P fertilization effects of fish sludge, meat bone meal, and wood ash have only been studied by the difference method (7, 16–18). This method compares the P uptake by a plant fertilized with the secondary resource with the P uptake by a plant receiving no P fertilizer (NoP). The difference in P uptake between the two treatments is defined as the P fertilization effect of the secondary resource. The underlying assumption in the difference method is that unfertilized and fertilized plants take up the same amount of P from the soil, i.e., that the secondary resource does not affect soil P availability. However, the effects of secondary resources such as fish sludge, meat bone meal, and wood ash on microbial and physicochemical soil P processes are still poorly understood, and it is not known whether the difference method actually reflects the net P fertilization effects of these secondary resources. Understanding the effects of complex secondary resources on soil processes is therefore important for a holistic evaluation of their fertilization effects.
Using radioisotopes of P in growth and incubation experiments provides the possibility to study P processes in soil/plant systems. In growth experiments, labeling soil with radioisotopes of P before application of secondary resources is a way to differentiate P taken up by the plants deriving from the fertilizer and from the soil (19–21). In incubation experiments, isotopic dilution can be used to study the incorporation of fertilizer into different soil P pools (22) or to quantify the amount of isotopically exchangeable phosphate (E-value) as affected by fertilizer application [e.g., Ref. (15, 20)].
The aim of this study was to determine the main drivers of plant P uptake following secondary resource application. To this end, the P fertilization effects of dairy manure (manure), fish sludge, meat bone meal, and wood ash were compared with those of water-soluble mineral P fertilizer (MinP) in a pot experiment with barley (Hordeum vulgare var. Heder) by 33P labeling of a loam soil at two soil pH levels (unlimed and limed). In parallel, soil incubation experiments were conducted to study the effects of the secondary resources on physicochemical and microbial soil processes and to explore their importance for plant P uptake.
Materials and Methods
Secondary Resources
The secondary resources are described in Table 1, while Table 2 gives an overview of their selected chemical properties. The secondary resources were also analyzed for heavy metal concentrations by ICP-OES after digestion in concentrated nitric acid in an ultraclave (23) (results not shown). Based on their heavy metal concentrations and Norwegian regulations (24), all the secondary resources studied here were eligible for application as fertilizer to agricultural land.
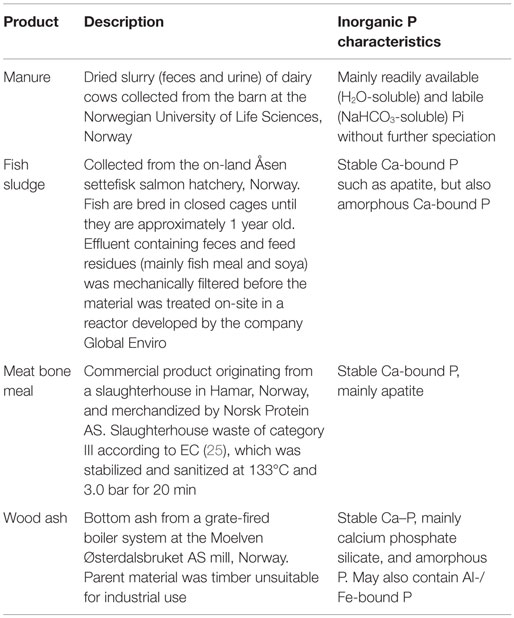
Table 1. Description of secondary resources and identified P characteristics (7).
Experimental Soil
The experimental soil originated from plots in a long-term field experiment in Norway (59°39′48.0″N 10°45′40.8″E) that has received 0 kg P year−1 and 0 or 5 kg potassium (K) year−1 since 1966. It is classified as an Albeluvisol in the World Reference Base for Soil Resources (31) and contains 27% clay, 40% silt, and 33% sand. Selected chemical characteristics of the soil are presented in Table 3. This soil was chosen because of its low content of plant-available P [measured as ammonium lactate-extractable P (P-AL)], in order to avoid P fertilization effects being masked by soil P. Before the soil was sampled at the end of the growing season in November 2013, barley, wheat and oats were grown in rotation for 16 years, with the last year of grass production being in 1997. After harvest of the cereals, including the straw, the soil was usually plowed in autumn. During sampling, random soil cores were taken from the 0–20 cm horizon in the middle of the plots. The soil was air-dried before sieving at mesh width 5 mm. To study the effect of soil pH on P uptake following secondary resource application, one part of the soil was limed with 2 g CaCO3 kg−1 soil dry matter (DM). Then, both the unlimed and the limed soils were incubated in portions of 15 kg at 60% of water-holding capacity (WHC, 100% WHC = 447 g H2O kg−1 soil) for 2.5 months in the dark before drying at 40°C. After transportation to Switzerland, the soil was again sieved at mesh width of 5 mm, carefully rewetted in portions of 1 kg soil DM, and incubated at 40% of WHC for at least 3 weeks. The pre-incubation aimed at reaching constant microbial activity, in order to minimize a microbial boost during setup of the experiment. When the experiments were set up, soil pH (measured in H2O) was 5.3 and 6.2 in the unlimed and limed soil, respectively.
Pot Experiment
The P fertilization effects of secondary resources were studied in a pot experiment using indirect labeling with 33P (21). Pre-incubated portions of 1 kg soil DM were mixed with carrier-free 33P-orthophosphate at a rate of 1.1 MBq kg−1 soil, which was added after dilution in H2O by 10 mL kg−1 soil. The soil was transferred into pots with sealed bottoms and again incubated at 16–18°C for 10 days to reach near-equilibrium conditions for the pools of plant-available 31P and 33P in the soil. Pots containing the same amount of unlabeled soil were also mixed and kept under the same conditions. The fertilization effects of manure, fish sludge, meat bone meal, and wood ash (all dried at 55°C and sieved at ≤2 mm) were compared with those of a treatment receiving NoP and a treatment receiving water-soluble mineral P [MinP, Ca(H2PO4)2⋅H2O in aqueous solution]. For the purposes of methodological control, the fertilization effect of MinP was also studied using direct labeling (MinPdir). MinPdir was produced by labeling Ca(H2PO4)2⋅H2O in aqueous solution with specific activity (SA) 40 kBq mg P−1 and applied corresponding to 1.2 MBq kg−1 soil. All fertilizers were applied based on a total P content equivalent to 30 mg P kg−1 and mixed into the whole soil volume. This P dose corresponded to 5.09 g manure kg−1 soil, 1.48 g fish sludge kg−1 soil, 0.57 g meat bone meal kg−1 soil, and 1.76 g wood ash kg−1 soil. To study the response of the soil to P fertilization, unlabeled MinP was also applied at rates of 15 and 45 mg P kg−1 soil. At the same time, all pots received a P-free nutrient solution containing 75 mg N [Ca(NO3)2⋅4H2O], 75 mg K (K2SO4), 15 mg magnesium (Mg; MgSO4⋅7H2O), 0.1 mg molybdenum (Mo; Na2MoO4⋅2H2O), 1 mg zinc (Zn; ZnSO4⋅7H2O), 1 mg Fe (Fe-chelate), 1 mg boron (B; H3BO3), 2 mg copper (Cu; CuSO4⋅5H2O), and 2 mg manganese (Mn; MnSO4⋅H2O) per kg soil. There were four replicates per treatment. Seven barley seeds (H. vulgare, var. Heder) were sown per pot and thinned out to five plants after germination. Seventeen days after setup of the experiment, when plants had developed three to four leaves, all pots were also given 75 mg N and 209 mg K as KNO3. All plants were watered with distilled water by weighing to 70% of WHC until germination, thereafter to 60% of WHC every 2 or 3 days, and daily toward the end of the experiment. Growing conditions in the greenhouse were set to 16 h photoperiod with artificial lights turning on when daylight <20 klx. Atmospheric humidity and mean temperature were set to 65% and 20°C during the day and 72% and 16°C at night. Pot positions were randomized three times a week. Forty-four days after setup of the experiment, when the first awns were visible [development stage varying between Zadoks 35 and 50 (34)], aboveground biomass was harvested by cutting the plants with scissors at 2 cm above the soil surface. Plant material was dried at 55°C for 48 h, DM production per pot was recorded and the plant material was milled in a Retsch ZM 200 mill (≤0.2 mm). For determination of P concentration in the plant tissue, 250 mg were incinerated at 550°C for 3 h and extracted with 3 mL concentrated, hot HNO3 [adapted according to (22)]. The P in the diluted filtrate (0.2 μm pore size) was determined colorimetrically according to Ohno and Zibilske (35). The P uptake per kg soil was computed by multiplying DM production by plant tissue P concentration. The 33P beta emissions in the labeling solutions and the extracts were measured in 1 mL sample after addition of 5 mL appropriate scintillation liquid (PerkinElmer Ultima Gold or PerkinElmer Ultima Gold AB) by liquid scintillation counting (TRI-CARB 2500 TR, liquid scintillation analyzer, Packard Instruments, Meriden, CT, USA) and corrected for radioactive decay back to the day when the soil was labeled. The N concentration in plant tissue was determined using a Thermo Electron FlashEA 1112 Automatic Elemental Analyser. Soil samples were taken in each pot and soil pH was measured in a solid-solution-ratio of 1:2.5 (v/v) in H2O after drying soil samples at 55°C and sieving at mesh width ≤2 mm.
Seed P Experiment
An additional experiment was conducted to determine the contribution of barley seed P to P uptake in aboveground biomass in response to increasing fertilization rate when the indirect method was used (22, 36). Sand (0.7–1.2 mm) was washed in 2% HCl before thorough rinsing with distilled H2O. Afterward, the pH of the sand was 4.97 [solid-solution-ratio of 1:2.5 (v/v) in H2O]. Portions of 1 kg sand DM were then fertilized with 0, 7.5, 15, 22.5 or 30 mg P kg−1 sand. The P fertilizer [Ca(H2PO4)2⋅H2O in aqueous solution] was labeled with 33P. The pots received 720 kBq kg−1 sand with the fertilizer, i.e., the SA of the P fertilizer was 96, 48, 32, and 24 kBq mg−1 P, respectively. The same P-free nutrient solution as given in the pot experiment was used. There were four replicates per treatment. Seven barley seeds were sown per pot and thinned out to five plants after germination. With five barley seeds, 0.71 ± 0.08 mg P were applied per kilogram of soil, as determined by the average weight of five barley seeds (0.20 ± 0.02 g DM kg−1, n = 20) and P concentration (3.49 ± 0.04 mg P g−1 DM, determined by colorimetric analysis after microwave digestion in concentrated H2O2 and HNO3, n = 4). During the first 19 days, the plants were watered up to 130 g H2O kg−1 sand, after which the water ratio was increased to 220 g H2O kg−1 sand. Plants were harvested by cutting with scissors at 2 cm above the sand surface 50 days after setup of the experiment when the first awns were visible (Zadoks 35–49). Plant material was analyzed in the same way as described for the pot experiment. During the seed P experiment, any isotopic dilution of the 33P in the shoot was caused by seed P, since this was the only non-labeled source. Therefore, this experiment allowed the P contribution from the seed and that from the fertilizer to be distinguished.
Calculations for Pot and Seed P Experiment
When labeled fertilizer was applied to the soil (direct method in pot study and seed P experiment), P derived from the fertilizer (Pdf fertilizer, mg P kg−1 soil) was calculated as
where SAplant (Bq mg−1 P) is the SA in the plant amended with the labeled fertilizer, SAfert (Bq mg−1 P) is the SA in the fertilizer, and (mg P kg−1 soil) is the amount of P taken up by the fertilized plant in aboveground biomass. In the seed P experiment, P derived from the seed (Pdf seed) was calculated as the difference between P uptake and Pdf fertilizer.
When the pool of plant-available P in the soil was labeled before application of an unlabeled fertilizer (indirect method), Pdf fertilizer was calculated as
where is the amount of P derived from the soil (mg P kg−1 soil) in the fertilized plant, which was calculated as
where (Bq mg−1 P) is the SA in the fertilized plants, SAplant NoP (Bq mg−1 P) is the average SA in the plants receiving NoP with P uptake corrected for Pdf seed, and is P derived from the seed (mg P kg−1 soil) in the fertilized plants, which was calculated from the seed P experiment as follows:
where a and b are the slope and intercept of the function presented in Figure 1.
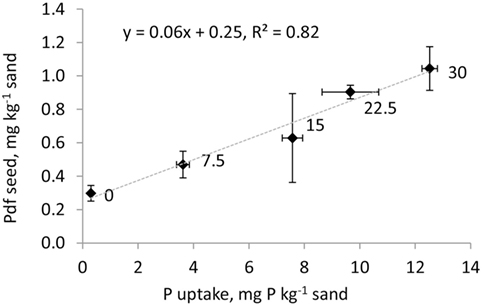
Figure 1. Relationship between P uptake in aboveground biomass (mg P kg−1 sand) and P derived from the seed (Pdf seed, mg P kg−1 sand) for barley grown on sand and fertilized with increasing rates of MinP labeled with 33P (0, 7.5, 15, 22.5, and 30 mg P kg−1 sand as indicated next to the experimental points). Error bars represent SD of the four replicates.
Moreover, fertilizer recovery (%) was calculated as the ratio between Pdf fertilizer and the amount of P applied with the fertilizer (P fert, mg P kg−1):
Relative agronomic efficiency (RAE, %) was calculated as the ratio between the fertilizer recovery of each secondary resource (P+) and the fertilizer recovery of MinP applied at the same rate (30 mg P kg−1 soil) as the secondary resource:
Incubation Experiment
Setup of the Incubation Experiment
In parallel, soil–fertilizer incubations were conducted to study physicochemical and microbial soil processes affected by the secondary resources in comparison with MinP and NoP on the unlimed and limed soil. Again, the soil was pre-incubated with distilled H2O at 40% of WHC to minimize a microbial boost during setup of the experiments. Three weeks after pre-incubation, aliquots of 1.2 kg soil were mixed with 33P corresponding to 5.2 MBq kg−1 soil that was added after dilution in H2O by 10 mL kg−1 soil for an incubation experiment with soil P labeling (indirect method). As for the pot experiment, the soil was kept in a controlled environment to reach near-equilibrium conditions for 31P and 33P for 10 days. A soil–fertilizer incubation experiment with no soil or fertilizer P labeling (termed unlabeled incubation) was also set up to determine isotopically exchangeable P as affected by P fertilizer (method described below). During the setup of both incubations, aliquots of 300 g soil were watered to 50% of WHC and mixed with fertilizer corresponding to 30 mg P kg−1 soil, but in contrast to the pot experiment, no P-free nutrient solution was added. Sub-aliquots of 100 g soil corresponding to three time points were placed in sealed plastic bags and kept in the dark under identical experimental conditions as in the pot experiment until analysis. There were four replicates per treatment. The effect of fertilizers on soil pH was studied after 7, 21, and 42 days in the incubation experiment with soil P labeling as described above.
P Concentration in Soil Solution and Isotopically Exchangeable P
The effects of fertilizers on the P concentration in the soil solution (CP, mg P L−1) and isotopically exchangeable P (E1min, in mg P kg−1) (37) were determined after 21 days in soil sampled in the unlabeled incubation experiment. The E1min comprises the Pi in the soil solution and Pi adsorbed to soil particles, which is exchangeable within the first minute of isotopic exchange kinetics (21). Incubated soil samples were dried at 40°C before extraction of 10 g soil in 99 mL H2O by end-over-end shaking. Isotopic exchange kinetics analyses were carried out after 16 h of shaking based on the assumption that steady-state equilibrium was reached, i.e., that CP was constant. The suspensions were then stirred at 300 rpm on a magnetic plate when 1 mL carrier-free 33P solution with a known amount of radioactivity was added to result in R = 600–900 Bq mL−1 in the sample. The soil:solution ratio was then 1:10. The suspensions were sampled with plastic syringes at 1, 4, 10, 30, 60, and 90 min after 33P addition (t). The remaining 33P in the filtrate [r(t), 0.2 μm pore size] was determined using scintillation counting as described above. After the last sampling, CP was determined colorimetrically (35). The E1min was calculated based on the assumption that R was evenly diluted with all inorganic P fractions having the same isotopic composition, according to
where Pi is the sum of Pi in the experimental soil (Total P minus Po; Table 3) and the fertilization rate 0 or 30 mg P kg−1. The isotopic dilution parameters m and n were calculated from a non-linear regression between r(t)/R and t before statistical refinement. The isotopic dilution parameter m is a measure of the remaining radioactivity in the solution after 1 min and n is a measure of how fast the radioactivity is disappearing from the solution.
Resin-Extractable P
The effects of fertilizers on resin-extractable P (Resin P) were studied after 7 and 21 days on soil sampled in the indirectly labeled incubation experiment. Moist samples equaling 2 g soil DM were extracted in 30 mL H2O upon horizontal shaking at 160 rec min−1 for 16 h with simultaneous adsorption to anion-exchange resin membranes (BDH 55164 2S, 6 cm × 2 cm) that had been shaken in advance twice in 0.5M NaHCO3 for 1 h. The P adsorbed to resin membranes was extracted by 0.1M NaCl/0.1M HCl. The P concentrations and radioactivity were determined as described above. The indirect labeling allowed estimation of the fraction of Resin P deriving from the fertilizer (Pdff Resin P, %) according to
where (Bq mg−1 P) is the SA in the soil amended with fertilizer and SANoP (Bq mg−1 P) is the SA in the soil receiving NoP.
Microbial P
The effects of fertilizer on P in microbial biomass (Pmic) were estimated after 7 and 21 days in the indirectly labeled incubation experiment as the difference between extracted P with simultaneous adsorption to anion-exchange resin membranes from fumigated and non-fumigated soil samples (Resin P). For the fumigation extraction, moist soil equaling 2 g soil DM was extracted in 30 mL H2O with 1 mL hexanol for 16 h. As a methodological control, 2 g soil DM were extracted in 30 mL H2O using anion-exchange resin membranes after addition of a P spike of 10 μg P g−1 soil for 16 h. The test showed that released P was effectively sorbed to the anion-exchange resin membranes, and it was not necessary to correct microbial biomass P for sorption to soil of P released during the fumigation-extraction.
Statistical Analysis
Equation 8 was adjusted using a non-linear procedure. Two-way ANOVA was applied to test the effect of the factors fertilizer treatment and pH level and their interaction on parameters studied in the pot and incubation experiment. Data sets were also analyzed using one-way ANOVA within the unlimed and the limed soil, respectively. Directly labeled treatments were excluded from the variance analyses and presented separately, including the SD of four replicates. Analyzed data were checked for normal distribution (normal quantile plots) and homogeneity of variance (residual versus fitted plots), and log transformed if indicated. For pair-wise comparisons, Tukey’s HSD test or t-tests were used at significance level α = 0.05. Moreover, simple linear regressions were run with selected parameters of the pot experiment as response variables and the parameters of the incubation experiment as explanatory variables, which were averaged over the four replicates. All statistical analyses were performed with JMP Pro 11.1.1 (38).
Results
P Concentration, Dry Matter Production, and Total P Uptake
There was a clear response of barley to P application on the experimental soil, as shown by linear increases in P uptake in aboveground biomass as a function MinP application rate (0, 15, 30, and 45 mg P kg−1 soil) on both the unlimed and limed soil. The slopes of the response curves for the two soils were not significantly different (p = 0.75), while the intercept was significantly higher on the limed than on the unlimed soil (p < 0.01) (see Supplementary Material). Phosphorus concentration in plant biomass ranged from 1.6 to 2.2 mg P g−1 DM. The P nutrition index for temperate grasses, calculated according to Liebisch et al. (39), clearly indicated P limitation in all fertilizer treatments (results not shown). Nitrogen concentrations (3.0–4.4 g 100 g−1 DM, results are not shown) were clearly above critical levels in temperate grasses as calculated according to Lemaire et al. (40), and observed differences between fertilizer treatments were therefore ascribed to P fertilization effects rather than N fertilization effects.
All secondary resources resulted in equally high P concentration as MinP on both soils except manure, which resulted in significantly lower P concentration than MinP on the limed soil. Aboveground DM production ranged from 3.5 to 5.2 g kg−1 soil and was equally high on the unlimed and limed soil (Table 4). None of the secondary resources increased DM compared with NoP on either soil, while MinP significantly increased DM compared with NoP on both soils. Phosphorus uptake in aboveground biomass ranged from 5.7 to 10.8 mg P kg−1 soil and was as result of slightly higher P concentration on the limed soil on average 0.5 mg P kg−1 soil greater on the limed than on the unlimed soil. All secondary resources resulted in significantly lower P uptake than MinP on both soils, except fish sludge, which resulted in equally large P uptake as MinP on the limed soil (Table 4).
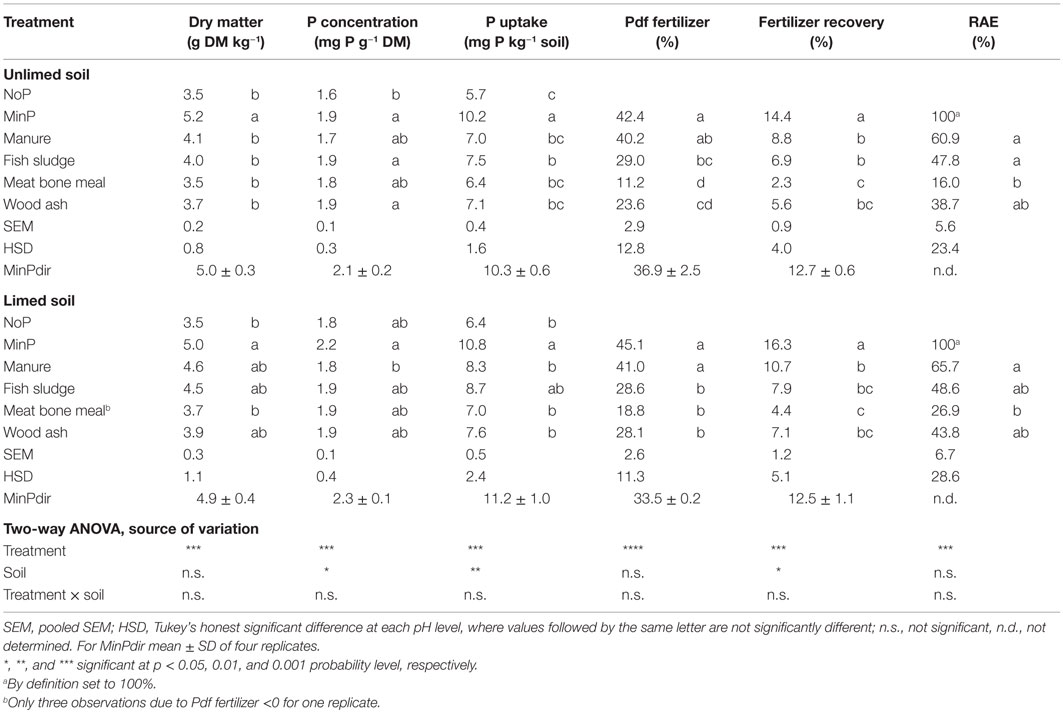
Table 4. Aboveground dry matter production (DM), P concentration and P uptake in aboveground biomass, P derived from fertilizer (Pdf fertilizer, %), fertilizer recovery (%), and relative agronomic efficiency (RAE, %) as an effect of different fertilizer treatments on unlimed and limed soil.
P Uptake from Different Sources
Phosphorus derived from soil was the most important P source for barley plants with all fertilizer treatments on both soils (Figure 2). All treatments resulted in equally large Pdf soil, except manure, which resulted in significantly smaller Pdf soil than all other treatments on the unlimed soil and in smaller Pdf soil than NoP on the limed soil.
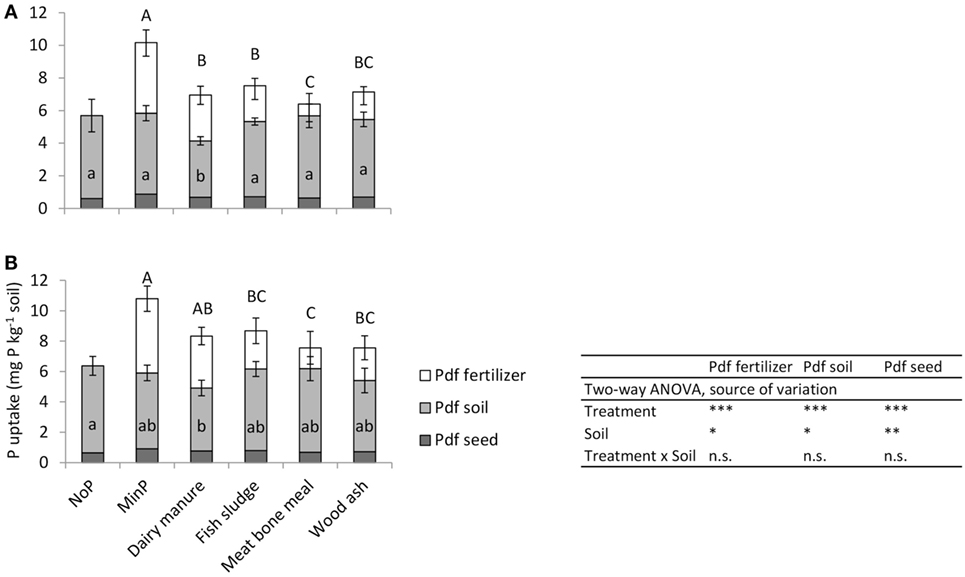
Figure 2. Phosphorus derived from fertilizer (Pdf fertilizer), soil (Pdf soil), and seed (Pdf seed) in barley (mg P kg−1 soil) as an effect of the different fertilizer treatments on (A) unlimed soil and (B) limed soil. Error bars represent the SD within each treatment. Letters indicate significant differences between treatments according to Tukey’s test (one-way ANOVA for each soil); uppercase letters refer to Pdf fertilizer and lowercase letters to Pdf soil. On the unlimed soil, data on Pdf soil were log transformed for the statistical analysis. On the limed soil, for Pdf fertilizer of meat bone meal, only three observations were considered. *, **, and *** significant at p < 0.05, 0.01, and 0.001 probability level, respectively.
Phosphorus derived from fertilizer was significantly smaller after application of secondary resources than after MinP on both soils (Figure 2). Only manure resulted in equally large Pdf fertilizer as MinP on the limed soil. Fish sludge and wood ash resulted in equally large Pdf fertilizer as manure, while meat bone meal resulted in significantly smaller Pdf fertilizer than manure on both soils. The Pdf fertilizer accounted for 40–44% of P uptake in plants after application of MinP or manure, and in significantly smaller fractions after application of the other secondary resources compared with MinP (Table 4). Fertilizer recovery was 14–16% of applied MinP and was significantly lower after application of all secondary resources on both soils. The RAE decreased in the order manure ≥fish sludge ≥wood ash ≥meat bone meal on both soils, but only meat bone meal resulted in significantly lower RAE than manure. Fish sludge and wood ash resulted in equally high RAE as manure.
The MinP treatment resulted in significantly smaller Pdf soil than MinPdir, the average difference being 1 mg P kg−1, and in significantly larger Pdf fertilizer than MinPdir, the difference being 0.7 mg P kg−1 (two-sided t-tests over both soils, n = 8). The variability between replicates was also generally lower for MinPdir than for MinP and all other treatments.
The Pdf soil and Pdf fertilizer were corrected for Pdf seed as estimated from the relationship between P uptake and Pdf seed studied in the seed P experiment (Figure 1). In the seed P experiment, Pdf seed significantly increased with increasing P fertilization rate. The P uptake by the highest P fertilization rate (1.04 ± 0.15 mg P kg−1) in aboveground biomass was higher than the average amount of P applied with the seeds (estimated to be 0.71 ± 0.08 mg P pot−1). With a and b from Figure 1, 0.65–0.95 mg P pot−1 in aboveground biomass was estimated to derive from seeds, representing 8.6–10.7% of P uptake in the pot experiment. The ranking of Pdf soil and fertilizer among treatments did not change when not corrected for Pdf seed (see Supplementary Material), emphasizing the low importance of Pdf seed in the present study.
Physicochemical and Microbial Soil Processes
On the unlimed soil, all fertilizers initially increased available P over NoP in the incubation experiment, as reflected by Resin P, measured 7 days after application (Table 5). Seven days after fertilizer application, Pdf fertilizer in the Resin P pool ranged from 25 to 38%, but there were no significant differences between treatments. Twenty-one days after application, Resin P was on average 4.6 mg P kg−1 soil lower than 7 days after fertilizer application (two-sided t-test over both time points, n = 48), and only MinP resulted in higher Resin P than NoP. The Pdf fertilizer in the Resin P pool ranged from 19 to 52%, again without significant differences between treatments. Twenty-one days after application, all secondary resources increased CP over NoP and resulted in equally high E1min as MinP.
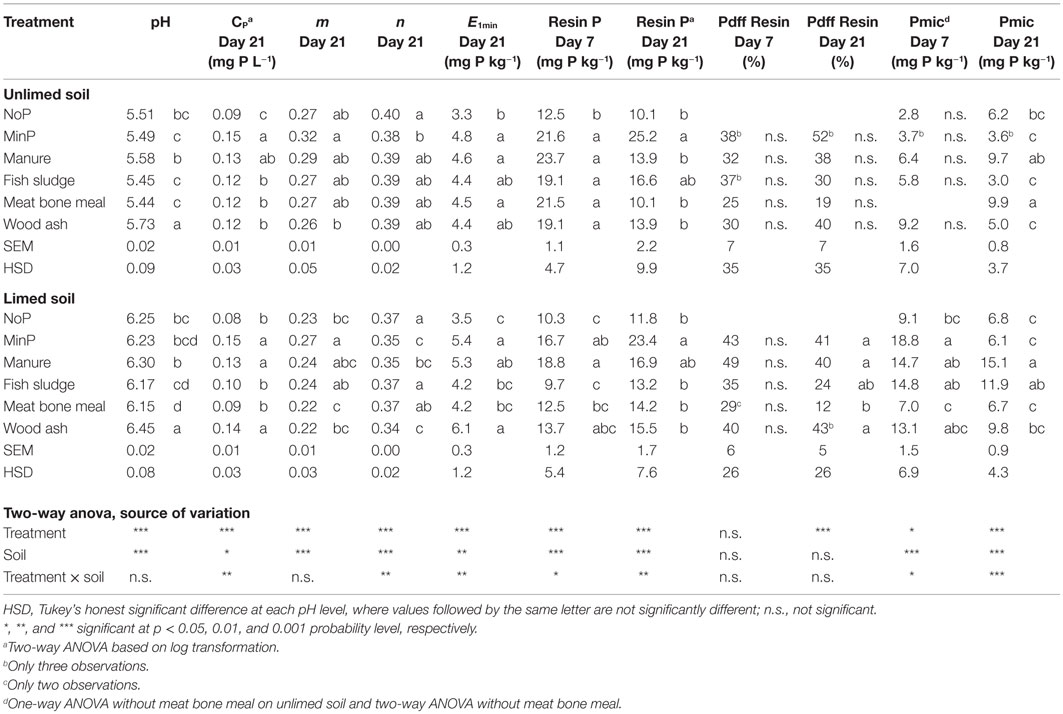
Table 5. pH measured in the pot experiment after harvest, CP = P concentration in the soil solution, m and n = isotopic dilution parameters, E1min = isotopically exchangeable P within 1 min, Resin P = resin-extractable P, Pdff Resin = P derived from the fertilizer in the resin-extractable P pool (%), and Pmic = microbial P 7 and 21 days after fertilizer application as an effect of different fertilizer treatments on unlimed and limed soil.
On the limed soil, meat bone meal and fish sludge did not increase available P over NoP as reflected by CP, E1min, and Resin P at any time point. Wood ash did not increase Resin P over NoP at any time point on the limed soil, but resulted in equally high CP and E1min as MinP. Seven days after fertilizer application, there were no differences in Pdf fertilizer in the Resin P pool between fertilizer treatments, with values ranging from 29 to 49%. Twenty-one days after fertilizer application, meat bone meal was the only secondary resource that resulted in significantly lower Pdf fertilizer in the resin-extractable P pool (12%) than MinP (41%).
In the pot experiment, wood ash increased soil pH compared with NoP on both soils and meat bone meal resulted in significantly lower soil pH than NoP on the limed soil, while the other fertilizers had no significant effect on soil pH compared with NoP in the pot experiment (Table 5). In the incubation experiment, there were no differences in soil pH between the three time points, but soil pH was generally 0.4 and 0.2 pH units lower than in the pot experiment on the unlimed and limed soil, respectively. In the incubation experiment, wood ash resulted in significantly higher soil pH than NoP on both soils, and the effects of fertilizer treatments generally followed a similar pattern as in the pot experiment. The results are therefore not shown.
Phosphorus uptake in microbial biomass (Table 5) was of the same order of magnitude as P uptake in plants (Table 4). It was generally higher on the limed than on the unlimed soil, the average difference being 8.4 mg P kg−1 soil at 7 days and 3.1 mg P kg−1 soil at 21 days after fertilizer application. On the unlimed soil, there were no differences in Pmic between treatments, except an increase over NoP following meat bone meal application 21 days after fertilizer application. On the limed soil at 7 days after fertilizer application, only MinP had significantly increased Pmic over NoP. However, this effect was transient, as 21 days after fertilizer application Pmic of MinP was significantly lower than at 7 days after fertilizer application, and MinP and meat bone meal resulted in equally low Pmic as NoP. In contrast, manure and fish sludge had significantly increased Pmic over NoP.
Drivers of P Uptake by Barley
Phosphorus uptake by barley was best explained by the solubility of inorganic P in fertilizers, whereas additional effects of fertilizers on physicochemical and microbial soil processes were of little overall importance. This is shown by linear positive relationships between P uptake in barley and the H2O + NaHCO3-soluble inorganic P (Pi) fraction in fertilizers and linear negative relationships between P uptake and the HCl-soluble Pi fraction in fertilizers on both soils (Figure 3; Table 6). According to the sequential fractionation based on Hedley et al. (41), the H2O + NaHCO3-soluble Pi fraction is operationally defined as readily available and labile Pi, while the HCl-soluble Pi fraction is defined as the slowly soluble Ca–P fraction. Phosphorus uptake by barley could further be explained by CP, m, and Resin P measured 21 days after fertilizer application on both soils, which all represent measures for the solubility of fertilizer P applied to the soil. The Pmic measured 7 days after fertilizer application resulted in significant relationships with P uptake by barley. However, while the relationship was negative on the unlimed soil, it was positive on the limed soil. Soil pH was unable to explain the variation in P uptake by barley between fertilizer treatments.
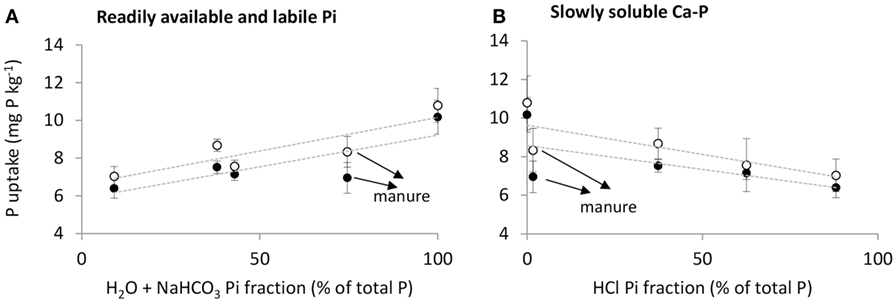
Figure 3. Phosphorus uptake by barley (mg P kg−1 soil) as an effect of (A) H2O + NaHCO3-soluble inorganic P fraction and (B) HCl-soluble inorganic P fraction in fertilizers for both soils (∙ = unlimed soil and ∘ = limed soil). All P in MinP was assumed to be present in the H2O + NaHCO3 Pi fraction. Error bars indicate SD of Pdf fertilizer between replicates.
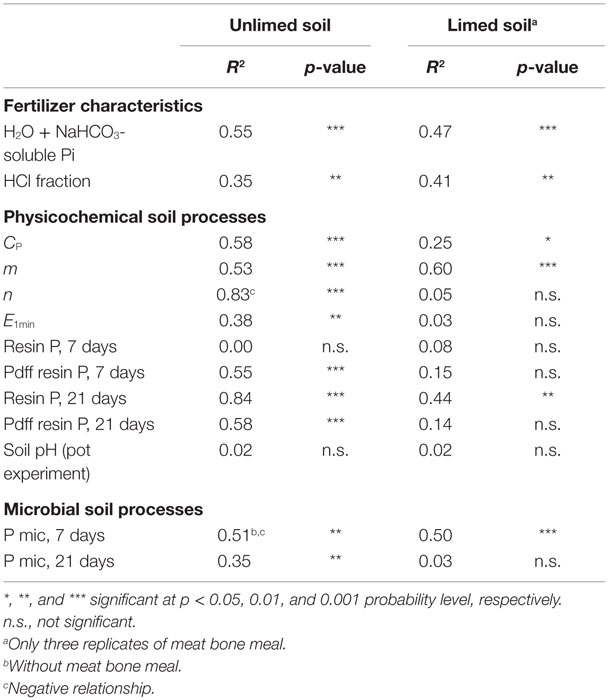
Table 6. Results of simple linear regression with Y = P uptake (mg P kg−1 soil) by barley plants on unlimed or limed soil without NoP, and X = explanatory variables, where fertilizer characteristics are sequentially extracted inorganic P (Pi) fractions in secondary P resources (Table 2), CP = water-soluble P (mg P L−1), m and n = isotopic dilution parameters, E1min = isotopically exchangeable P (mg P kg−1 soil), Resin P = resin-extractable P (mg P kg−1 soil), Pdff Resin P = P derived from the fertilizer in the resin-extractable P pool (%), and Pmic = microbial P (mg P kg−1 soil), measured, respectively, 7 and 21 days after fertilizer application.
Discussion
Effects of Inorganic P Species in Secondary Resources on P Uptake by Barley
The P uptake by barley following secondary resource application was mainly affected by the solubility of the inorganic P species the resource contained. The indirect effects of the secondary resources studied on P uptake through their influences on physicochemical and microbial soil processes were generally of less importance.
Poor P uptake following meat bone meal application can be explained by its large fraction of Ca-bound P such as hydroxyapatite and chlorfluorapatite (7) with low solubility, especially in soils with pH >6.5 (42). Similar results have been reported by Ylivainio et al. (18) and Brod et al. (7) after application of meat bone meal to ryegrass.
Fish sludge was the secondary resource that tended to result in the highest P uptake, probably because a considerable P fraction in fish sludge is readily available and labile, i.e., soluble in H2O and NaHCO3 (Table 2). However, fish sludge also contains apatite (7), which can explain why neither meat bone meal nor fish sludge increased CP, E1min, and Resin P over NoP in the limed soil of the incubation experiment.
Wood ash resulted in equally high P uptake as the other secondary resources, as expected from its large fraction of labile Pi, in addition to slowly soluble Ca–P [mainly (Ca2(SiO4))6Ca3(PO4)2] (7). The P uptake following wood ash application was equally high on both soils, but on the limed soil of the incubation experiment wood ash surprisingly increased CP and E1min to the same level as MinP. Similarly, Brod et al. (7) found increasing P fertilization effects of the same wood ash with increasing soil pH and attributed this effect to the likely presence of NaHCO3-soluble P adsorbed to Al-/Fe-(hydr)oxides. Wood ash was the secondary resource with the largest NaHCO3-soluble Pi fraction among all products studied. Phosphorus adsorbed to Al-/Fe-(hydr)oxides is characterized by increasing solubility with increasing soil pH (9). However, increased CP and E1min values after wood ash application on the limed soil could also be a methodological artifact if the magnetic stirrer mechanically destroyed (Ca2(SiO4))6Ca3(PO4)2, thereby solubilizing phosphate, while the same stable Ca–P was already solubilized in the unlimed soil. Similarly, Sinaj et al. (43) found that silicato-calcium phosphate present in Thomas slag quickly solubilized after application to an acidic soil with pH 6.2. A methodological artifact during determination of E1min would explain why increased wood ash P solubility on the limed compared with the unlimed soil was not reflected by increased Pdf fertilizer in the pot experiment or elevated Resin P on the limed soil.
Manure resulted in lower P uptake by barley than MinP, even though 75% of P in manure was present as readily available and labile Pi (Table 2). Phosphorus uptake following manure application tended to be lower than expected from the linear regression lines with the H2O + NaHCO3-soluble or HCl-soluble Pi fractions as explanatory variables (Figure 3). Oberson et al. (44) also reported lower P fertilization effects of cow feces than di-ammonium phosphate after a pot experiment using indirect labeling and soils with different fertilization histories.
Effects of Microbial Soil Processes on P Uptake by Barley
Our results indicate that organic C applied with manure may have resulted in microbial immobilization of soluble P, since Pdf soil (mg P kg−1) in barley was significantly lower after manure application than after NoP on both soils (Figure 2). This is also in agreement with manure increasing Pmic, compared with MinP, on both soils after 21 days (Table 5). Oberson et al. (44) reported microbial P immobilization following cow feces application to soils with different fertilization histories, and Bünemann et al. (45) describe increases in Pmic as a result of glucose addition during an incubation experiment with a P-deficient tropical soil. On the limed soil in the present study, total P uptake by barley was lower for the manure than the MinP treatment, but Pdf fertilizer (mg P kg−1) after manure application was equally high as after MinP. Therefore, the difference method would have underestimated the fertilization effect of manure because of microbial immobilization of soluble P in this soil and thereby a lower contribution from soil P.
Even though organic C also was applied with fish sludge and meat bone meal, microbial P immobilization seems not to have been a major competitor to barley plants in these cases, and Pdf soil (mg P kg−1) was equally high as after MinP. At the same fertilization rate of P, only 557 and 152 mg organic C kg−1 were applied with fish sludge and meat bone meal, compared with 2008 mg organic C kg−1 with manure (Table 2). In the incubation experiment, however, there were still signs of P immobilization, indicated as increased Pmic over NoP 21 days after application of fish sludge on the limed and meat bone meal on the unlimed soil (Table 5). This can be explained by a larger fraction of C in fish sludge and meat bone meal being soluble in H2O than the C in manure (Table 2). Accordingly, Bünemann et al. (46) point out a strong impact of C quality on microbial P immobilization. Still, due to too few products included in this study, we cannot specify the quantity and quality of organic C in secondary resources at which P uptake by plants might be negatively affected by activated microbial activity. Furthermore, it remains unknown whether the effect of secondary resources on microbial soil processes is also negligible for plant P uptake on soils with high microbial activity, because in the present study Pmic in both soils was overall rather low (47).
Effects of Physicochemical Soil Processes on P Uptake by Barley
Phosphorus uptake by barley was higher on the limed than on the unlimed soil (Figure 2), probably because growing conditions were better overall. Barley is known to be sensitive to low soil pH [e.g., Ref. (48)], which is often associated with high concentrations of soluble Al. However, higher P uptake on the limed than on the unlimed soil could also be due to higher P availability, as supported by higher P concentration in plants receiving NoP (two-sided t-test, n = 8) (Table 4). On the unlimed soil, more P was probably adsorbed to Al-/Fe-(hydr)oxides because of increased positive surface charges at lower soil pH. The increased importance of P adsorption on the unlimed soil was also indicated by a decrease in Resin P over time, which was not observed in the limed soil (Table 5).
Wood ash caused a significant increase in soil pH on both soils as result of the liming effect of CaCO3. Increasing effects of wood ash on soil pH are well known [e.g., Ref. (49)]. However, the effect of wood ash on pH was far too small to significantly influence P availability in the soil (Table 6). Equally high Pdf soil (mg P kg−1) after wood ash application and NoP showed that soil P availability was not affected by the pH-increasing effect of wood ash within the pH range in the present experiment. With wood ash, only 0.1 g CaCO3 kg−1 soil was applied [8 wt% CaCO3 in wood ash according to Brod et al. (7)], in comparison with 2 g CaCO3 kg−1 soil applied to the limed soil.
Methodological Considerations
Conducting the isotope dilution technique allowed us to conclude that the difference method would have led to similar results for P fertilization effects in this case, because P uptake by barley after secondary resource application was overall little affected by their influence on physicochemical and microbial soil processes. The underlying assumption in the difference method that fertilized and unfertilized treatments take up the same P amount from the soil would only have been violated after manure application due to microbial immobilization. Therefore, the results are also in agreement with those of a previous experiment in which a sand–peat mixture was used as a model soil (7), even though natural soil processes could not be studied. Thus, the results of the present study indicate that the difference method is reliable for secondary resources with low ratios of OM to P. However, these results should be confirmed with different soil types and extended with several secondary resources with a wide range of organic C content compared with P.
The indirect labeling method is based on the assumptions that plant-available soil P is homogeneously labeled and that dilution of the radioisotope is only due to the unlabeled fertilizer. The internal control treatment MinPdir resulted in significantly higher Pdf soil and lower Pdf fertilizer than MinP according to a comparison of the two treatments over both soils. The difference between the directly and indirectly labeled mineral control treatment suggests that unlabeled soil P contributed to the dilution of the SA in plants in the MinP treatment, e.g., via the mineralization of organic or microbial P (44). This means that fertilization effects might have been slightly overestimated when the indirect method was used. In fact, significantly increased Pmic over NoP after MinP application on the limed soil after 7 days indicates modified microbial activity also in the mineral control treatment, even though no organic C was applied with MinP. In that case, soil microbes were probably stimulated as a methodological artifact by introducing oxygen when mixing the experimental soil, as suggested by Oberson et al. (50) and Bünemann et al. (51). Twenty-one days after fertilizer application, the microbial boost could no longer be observed.
Another methodological inconsistency was observed in the seed P experiment, where our results incorrectly indicated that the amount of P derived from the seed was larger than the amount applied with seed. It is possible that the acid-washed sand still contained P, some P added with the two removed seeds had leaked into the soil, or P applied with the seed was underestimated because the seeds sown per pot were not weighed. In addition, large variation between the replicates of Pdf seed (Figure 1) and relatively low transfer from the seed compared with total P uptake might have contributed to the inconsistency. However, the actual reasons could not be identified. An alternative to the approach used here is to estimate Pdf seed by comparing the P content in the seeds at seedling stage and the remaining P content in the seeds at harvest, as suggested by Achat et al. (52). Several studies have pointed out the importance of correcting for seed P contribution when the indirect method is applied [e.g., Ref. (22, 36, 52)] to avoid overestimation of P derived from the unlabeled fertilizer. Here, the fraction of Pdf seed in total P uptake was small in comparison with, e.g., results reported by Achat et al. (52), who used ryegrass and fescue as the experimental crop and suggested that 15–50% of P uptake at the first cut was Pdf seed. The difference between the different indirectly labeled treatments was also small and the relative fertilization effect of the secondary resources was not affected by Pdf seed.
Conclusion
This study explored the effects of secondary resources on physicochemical and microbial soil P processes and their importance for plant P uptake at two soil pH levels in the same arable soil. The main driver for P uptake was found to be the solubility of inorganic P species contained in the secondary resources, while indirect effects on P availability via influences on physicochemical and microbial soil processes were of little overall importance. This implies that P uptake following secondary resource application can be sufficiently predicted by intrinsic chemical P characteristics. The P uptake by barley was indeed best explained by a linear positive relationship with the H2O + NaHCO3-soluble inorganic P fraction and a linear negative relationship with the HCl-soluble inorganic P fraction in fertilizers on both unlimed and limed soil. Organic C resulted in microbial immobilization of labile P and decreased uptake in barley of P derived from the soil only after manure application. In this arable soil with rather low microbial biomass P, immobilization of P in microbial biomass could not challenge barley plants as the main P sink after application of fish sludge and meat bone meal. Further studies are needed to identify the critical organic C content in secondary resources at which microbial P processes influence plant P uptake. The significant increase in soil pH as result of wood ash application had no effect on P uptake by barley plants within the pH range in this study.
Author Contributions
EB and AO designed the experiment; EB conducted the experiment; and EB analyzed the data with the help of AO and wrote the paper. All authors contributed to the interpretation of the results, and read and approved the manuscript.
Conflict of Interest Statement
The authors declare that the research was conducted in the absence of any commercial or financial relationships that could be construed as a potential conflict of interest.
Funding
This work was part of the research projects CenBio (Bioenergy Innovation Centre, Grant No. 193817) and Innovative utilization of wood ash (Grant No. 215935). Both are co-funded by the Research Council of Norway and research and industry partners. The cooperation on which the present study was based was enabled by the research project IMPROVE-P, which is funded by transnational funding bodies that are partners of the FP7 ERA-net project, and CORE Organic Plus, with cofunding from the European Commission. We thank Dr. L. Schönholzer (ETH Zürich) for support with the analytical methods.
Supplementary Material
The Supplementary Material for this article can be found online at http://journal.frontiersin.org/article/10.3389/fnut.2016.00012
Abbreviations
P fert, P applied with the fertilizer; P uptake, P taken up by plant in aboveground biomass; Pdf fertilizer, P derived from the fertilizer; Pdf seed, P derived from the seed; Pdf soil, P derived from the soil; Pdff ResinP, Resin P deriving from the fertilizer; Pi, inorganic P; RAE, relative agronomic efficiency; SA, specific activity.
References
1. van Dijk KC, Lesschen JP, Oenema O. Phosphorus flows and balances of the European Union Member States. Sci Total Environ (2015) 542:1078–93. doi: 10.1016/j.scitotenv.2015.08.048
2. Schoumans OF, Bouraoui F, Kabbe C, Oenema O, van Dijk K. Phosphorus management in Europe in a changing world. Ambio (2015) 44:180–92. doi:10.1007/s13280-014-0613-9
3. Withers PJA, van Dijk KC, Neset T-SS, Nesme T, Oenema O, Rubæk GH, et al. Stewardship to tackle global phosphorus inefficiency: the case of Europe. Ambio (2015) 44:193–206. doi:10.1007/s13280-014-0614-8
4. Hamilton H, Brod E, Hanserud O, Gracey E, Vestrum M, Steinhoff F, et al. Investigating cross-sectoral synergies through integrated aquaculture, fisheries, and agriculture phosphorus assessments: a case study of Norway. J Ind Ecol (2015). doi:10.1111/jiec.12324
5. Norwegian Environment Agency. Bedre utnyttelse av fosfor i Norge. Rapport M-351. (2015). Available from: http://www.miljodirektoratet.no/no/Publikasjoner/2015/Juni/Bedre-utnyttelse-av-fosfor-i-Norge/
6. Nanzer S, Oberson A, Huthwelker T, Eggenberger U, Frossard E. The molecular environment of phosphorus in sewage sludge ash: implications for bioavailability. J Environ Qual (2014) 43:1050–60. doi:10.2134/jeq2013.05.0202
7. Brod E, Øgaard AF, Hansen E, Wragg D, Haraldsen TK, Krogstad T. Waste products as alternative phosphorus fertilizer. Part I: inorganic P species affect fertilization effects depending on soil pH. Nutr Cycl Agroecosyst (2015) 103:167–85. doi:10.1007/s10705-015-9731-4
8. Hedley M, McLaughlin M. Reactions of phosphate fertilizers and by-products in soils. In: Sims TJ, Sharpley AN, editors. Phosphorus: Agriculture and the Environment. Madison, WI: American Society of Agronomy, Inc., Crop Science Society of America, Inc., Soil Science Society of America, Inc (2005). p. 181–252. Agronomy Monograph No. 46.
10. Brod E, Øgaard AF, Haraldsen TK, Krogstad T. Waste products as alternative phosphorus fertilizer. Part II: predicting P fertilization effects by chemical extraction. Nutr Cycl Agroecosyst (2015) 103:187–99. doi:10.1007/s10705-015-9731-4
11. McLaughlin MJ, Alston AM. The relative contribution of plant residues and fertilizer to the phosphorus nutrition of wheat in a pasture/cereal system. Aust J Soil Res (1986) 24:517–26. doi:10.1071/SR9860517
12. Oberson A, Joner E. Microbial turnover of phosphorus in soil. In: Turner BL, Frossard E, Baldwin DS, editors. Organic Phosphorus in the Environment. Wallingford: CAB International (2005). p. 133–64.
13. Øgaard A. Effect of fresh and composted cattle manure on phosphate retention in soil. Acta Agric Scand Sect B Soil Plant Sci (1996) 46:98–105.
14. Jakobsen I, Leggett ME, Richardson AE. Rhizosphere microorganisms and plant phosphorus uptake. In: Sims TJ, Sharpley AN, editors. Phosphorus: Agriculture and the Environment. Madison, WI: American Society of Agronomy, Inc., Crop Science Society of America, Inc., Soil Science Society of America, Inc (2005). p. 437–94. Agronomy Monograph No. 46.
15. Achat DL, Daumer M-L, Sperandio M, Santellani A-C, Morel C. Solubility and mobility of phosphorus recycled from dairy effluents and pig manures in incubated soils with different characteristics. Nutr Cycl Agroecosyst (2014) 99:1–15. doi:10.1007/s10705-014-9614-0
16. Erich MS. Agronomic effectiveness of wood ash as source of phosphorus and potassium. J Environ Qual (1991) 20:576–81. doi:10.2134/jeq1991.203576x
17. Jeng AS, Haraldsen TK, Grønlund A, Pedersen PA. Meat and bone meal as nitrogen and phosphorus fertilizer to cereals and rye grass. Nutr Cycl Agroecosyst (2006) 76:183–91. doi:10.1007/s10705-005-5170-y
18. Ylivainio K, Uusitalo R, Turtola E. Meat bone meal and fox manure as P sources for ryegrass (Lolium multiflorum) grown on a limed soil. Nutr Cycl Agroecosyst (2008) 81:267–78. doi:10.1007/s10705-007-9162-y
19. Morel C, Fardeau JC. The uptake by crops of fresh and residual phosphatic fertilizers by simultaneous measurements with 32P and 33P. Appl Radiat Isot (1989) 40:273–8. doi:10.1016/0883-2889(89)90217-7
20. Frossard E, Sinaj S, Zhang LM, Morel JL. The fate of sludge phosphorus in soil-plant systems. Soil Sci Soc Am J (1996) 60:1248–53. doi:10.2136/sssaj1996.03615995006000040041x
21. Frossard E, Achat DL, Bernasconi SM, Bünemann E, Fardeau J-C, Jansa J, et al. The use of tracers to investigate phosphate cycling in soil-plant systems. In: Bünemann EK, editor. Phosphorus in Action. Berlin, Heidelberg: Springer Verlag (2011). p. 59–91.
22. Nanzer S, Oberson A, Berger L, Berset E, Hermann L, Frossard E. The plant availability of phosphorus from thermo-chemically treated sewage sludge ashes as studied by 33P labelling techniques. Plant Soil (2014) 377:439–56. doi:10.1007/s11104-013-1968-6
23. EN ISO 11885. Water Quality: Determination of Selected Elements by Inductively Coupled Plasma Optical Emission Spectrometry (ICP-OES). Brussels: CEN (2009).
24. Norwegian Ministry of Agriculture and Food. Forskrift om gjødselvarer mv. av organisk opphav. (2003). Available from: http://www.lovdata.no/for/sf/ld/xd-20030704-0951.html#27
25. EC. Regulation (EC) No 1774/2002 of the European Parliament and of the Council of 3 October 2002 Laying Down Health Rules Concerning Animal by-Products Not Intended for Human Consumption. (2002). Available from: http://eur-lex.europa.eu/legal-content/EN/TXT/PDF/?uri=CELEX:32002R1774&from=EN
26. Møberg JP, Petersen L. Øvelsesvejledning til geologi og jordbundslære II. København: Den Kongelige Veterinær- og Landbohøyskole (1982).
27. Murphy J, Riley JP. A modified single solution method for the determination of phosphate in natural waters. Anal Chim Acta (1962) 27:31–6. doi:10.1016/S0003-2670(00)88444-5
28. EN 13654-1. Soil Improvers and Growing Media: Determination of Nitrogen. Part 1: Modified Kjeldahl Method. Brussels: CEN (2001).
29. Henriksen A, Selmer-Olsen AR. Automatic methods for determining nitrate and nitrite in water and soil extracts. Analyst (1970) 95:514–8. doi:10.1039/an9709500514
30. Selmer-Olsen AR. Determination of ammonium in soil extracts by an automated indophenol method. Analyst (1971) 96:565–8. doi:10.1039/an9719600565
31. NIBIO. WRB Grupper. (2015). Available from: http://kilden.skogoglandskap.no/?topic=jordsmonn&X=7334000.00&Y=400000.00&zoom=0&lang=nb&bgLayer=graatone_cache&layers=jm_wrb_grupper&layers_opacity=0.75
32. Egnér H, Riehm H, Domingo WR. Untersuchungen über die chemische Bodenanalyse als Grundlage für die Beurteilung des Nährstoffzustandes der Böden. Kungl Lantbrukshögskolans Annaler (1960) 26:199–215.
33. van Reeuwijk LP. Procedures for Soil Analysis. 12-2. Acid Oxalate Extractable Fe. Wageningen: FAO (1995).
34. Zadoks JC, Chang TT, Konzak CF. A decimal code for the growth stages of cereals. Weed Res (1974) 14:415–21. doi:10.1111/j.1365-3180.1974.tb01084.x
35. Ohno T, Zibilske LM. Determination of low concentrations of phosphorus in soil extracts using malachite green. Soil Sci Soc Am J (1991) 55:892–5. doi:10.2136/sssaj1991.03615995005500030046x
36. Pypers P, Van Loon L, Diels J, Abaidoo R, Smolders E, Merckx R. Plant-available P for maize and cowpea in P-deficient soils from the Nigerian Northern Guinea savannah – comparison of E- and L-values. Plant Soil (2006) 283:251–64. doi:10.1007/s11104-006-0016-1
37. Fardeau JC, Guiraud G, Marol C. The role of isotopic techniques on the evaluation of the agronomic effectiveness of P fertilizers. Fert Res (1996) 45:101–9. doi:10.1007/BF00790659
39. Liebisch F, Bünemann EK, Huguenin-Elie O, Jeangros B, Frossard E, Oberson A. Plant phosphorus nutrition indicators evaluated in agricultural grasslands managed at different intensities. Eur J Agron (2013) 44:67–77. doi:10.1016/j.eja.2012.08.004
40. Lemaire G, Jeuffroy M-H, Gastal F. Diagnosis tool for plant and crop N status in vegetative stage: theory and practices for crop N management. Eur J Agron (2008) 28:614–24. doi:10.1016/j.eja.2008.01.005
41. Hedley MJ, Stewart JWB, Chauhan BS. Changes in inorganic and organic soil phosphorus fractions induced by cultivation practices and by laboratory incubations. Soil Sci Soc Am J (1982) 46:970–6. doi:10.2136/sssaj1982.03615995004600050017x
42. Morel C, Fardeau JC. Agronomical evaluation of phosphate fertilizer as a nutrient source of phosphorus in crops: isotopic procedure. Fert Res (1990) 24:115–22. doi:10.1007/BF01073230
43. Sinaj S, Frossard E, Fardeau JC, Lhote F, Morel JL. Observation directe de l’altération de scories de déphosphoration après incorporation dans un sol acide cultivé. C R Acad Sci Sér II (1994) 319:1207–14.
44. Oberson A, Tagmann HU, Langmeier M, Dubois D, Mäder P, Frossard E. Fresh and residual phosphorus uptake by ryegrass from soils with different fertilization histories. Plant Soil (2010) 334:391–407. doi:10.1007/s11104-010-0390-6
45. Bünemann EK, Smithson PC, Jama B, Frossard E, Oberson A. Maize productivity and nutrient dynamics in maize-fallow rotations in western Kenya. Plant Soil (2004) 264:195–208. doi:10.1023/B:PLSO.0000047749.43017.fd
46. Bünemann E, Bossio DA, Smithson PC, Frossard E, Oberson A. Microbial community composition and substrate use in a highly weathered soil as affected by crop rotation and P fertilization. Soil Biol Biochem (2004) 36:889–901. doi:10.1016/j.soilbio.2004.02.002
47. Frossard E, Buchmann N, Bünemann EK, Kiba DI, Lompo F, Oberson A, et al. Soil properties and not inputs control carbon, nitrogen, phosphorus ratios in cropped soils in the long-term. Soil Discuss (2015) 2:995–1038. doi:10.5194/soild-2-995-2015
48. Bona L, Wright RJ, Baligar VC, Matuz J. Screening wheat and other small grains for acid soil tolerance. Landscape Urban Plan (1993) 27:175–8. doi:10.1016/0169-2046(93)90046-G
49. Demeyer A, Voundi Nkana JC, Verloo MG. Characteristics of wood ash and influence on soil properties and nutrient uptake: an overview. Bioresource Technol (2001) 77:287–95. doi:10.1016/S0960-8524(00)00043-2
50. Oberson A, Friesen DK, Rao IM, Bühler S, Frossard E. Phosphorus transformations in an Oxisol under contrasting land-use systems: the role of the soil microbial biomass. Plant Soil (2001) 237:197–210. doi:10.1023/A:1013301716913
51. Bünemann E, Marschner P, McNeill AM, McLaughlin MJ. Measuring rates of gross and net mineralisation of organic phosphorus in soils. Soil Biol Biochem (2007) 39:900–13. doi:10.1016/j.soilbio.2006.10.009
Keywords: waste products, fish sludge, meat bone meal, wood ash, animal manure, isotope techniques
Citation: Brod E, Øgaard AF, Krogstad T, Haraldsen TK, Frossard E and Oberson A (2016) Drivers of Phosphorus Uptake by Barley Following Secondary Resource Application. Front. Nutr. 3:12. doi: 10.3389/fnut.2016.00012
Received: 01 February 2016; Accepted: 26 April 2016;
Published: 12 May 2016
Edited by:
Philippe Hinsinger, INRA, FranceCopyright: © 2016 Brod, Øgaard, Krogstad, Haraldsen, Frossard and Oberson. This is an open-access article distributed under the terms of the Creative Commons Attribution License (CC BY). The use, distribution or reproduction in other forums is permitted, provided the original author(s) or licensor are credited and that the original publication in this journal is cited, in accordance with accepted academic practice. No use, distribution or reproduction is permitted which does not comply with these terms.
*Correspondence: Eva Brod, ZXZhLmJyb2RAbmliaW8ubm8=