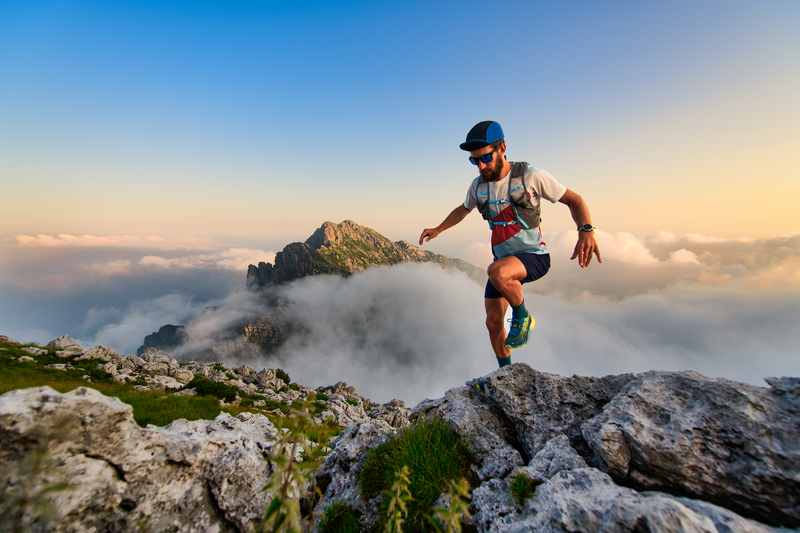
95% of researchers rate our articles as excellent or good
Learn more about the work of our research integrity team to safeguard the quality of each article we publish.
Find out more
OPINION article
Front. Neurol. , 05 February 2025
Sec. Stroke
Volume 16 - 2025 | https://doi.org/10.3389/fneur.2025.1543725
This article is part of the Research Topic Vascular Diseases of the Brain: Insights, Progress and Lessons Learned View all 4 articles
Nearly a decade ago, a paravascular pathway, enabling cerebrospinal fluid (CSF) to flow through the brain parenchyma, was discovered (1). Known as the “glymphatic” (glial-lymphatic) system, it has emerged as a critical process for clearing waste from the brain's interstitial tissue, which lacks histologically distinct lymphatic vessels (2).
In addition to the conventional CSF circulation from the choroid plexus through arachnoid granulations and venous sinuses; in the glymphatic system (Figure 1), arterial pulsations propagate CSF through the perivascular spaces (PVS) (2). These PVS are formed by the vascular endfeet of astrocytes, which facilitate CSF flow through the abundant expression of aquaporin-4 (AQP4) (1–3). The CSF then diffuses through the brain's interstitial fluid and exits via perivenous spaces, draining either through meningeal and cervical lymphatic vessels or arachnoid granulations (4, 5).
Figure 1. Glymphatic system. In the glymphatic system, arterial pulsations propagate CSF through the perivascular spaces as penetrating arteries arise from major arteries within the subarachnoid cisterns. These PVS are formed by the vascular endfeet of astrocytes, which facilitate CSF flow through the abundant expression of AQP4. The CSF then diffuses through the brain's interstitial fluid and exits via perivenous spaces, draining either through meningeal and cervical lymphatic vessels or arachnoid granulations. CSF: cerebrospinal fluid, PVS: perivascular space. ISF, Interstitial fluid.
The glymphatic system was initially discovered in rodent models (1), and its presence has also been demonstrated in humans (6–8) through intrathecal contrast MRI studies by demonstrating contrast flow through the subarachnoid spaces, perivascular spaces, and finally into the brain parenchyma (7, 8).
Interestingly, the glymphatic system is most active during non-rapid eye movement (NREM) sleep, particularly N3 stage (9, 10). Human studies support that glymphatic activity is enhanced during sleep, increasing with higher delta activity and decreasing with higher beta activity and heart rate (11). Moreover, sleep position influences glymphatic efficiency (lateral more efficient than supine or prone) (12).
The glymphatic system plays a vital role in clearing soluble proteins and brain metabolites, facilitating fluid and solute exchange across the brain parenchyma. This includes glucose for energy metabolism, lipid transport, signaling molecules, and the removal of waste products such as amyloid-beta and tau, as demonstrated in both animal models and humans (1, 5, 13–17). In humans, sleep deprivation impairs CSF-to-blood clearance of amyloid-beta and tau (17), and a sleep-active glymphatic system contributes to the clearance of these proteins from the brain (14). Demonstration of the glymphatic system in humans is a breakthrough that connects aging, sleep, cardiovascular health, and brain health.
Multiple approaches have been proposed to measure glymphatic activity or dysfunction in humans (18). The glymphatic system was initially demonstrated through intrathecal contrast-based studies, which revealed a centripetal enhancement pattern moving from the subarachnoid space into the parenchyma (gray and white matter) along the perivascular spaces (7, 8, 19).
Subsequently, intravenous contrast-based methods were also developed. These techniques employ region-of-interest analyses of the brain parenchyma and account for both vascular and CSF contributions to parenchymal enhancement (11, 20). However, IV or intrathecal contrast methods often require sequential MRI measurements to track contrast flow.
Another widely used approach is diffusion-derived MRI, specifically the assessment of diffusivity along the perivascular space (ALPS) (21, 22). This method is quick, non-invasive, does not require contrast; however, it does not capture the entire glymphatic system (i.e., gray matter). Instead, it relies on diffusion tensor imaging of white matter at the level of the lateral ventricles (23).
Enlarged perivascular spaces (ePVS) have been proposed as an indirect marker of glymphatic dysfunction and can be visualized on conventional MRI, particularly T2-weighted sequences (18). Still, they may reflect diverse pathophysiological processes rather than glymphatic function specifically. For instance, basal ganglia ePVS are commonly associated with lacunar strokes, whereas centrum semiovale ePVS often correlate with cerebral amyloid angiopathy (24, 25).
Various other methods to evaluate glymphatic function are discussed elsewhere (18). A key challenge in the field is the development of techniques that are minimally invasive, widely accessible, and capable of real-time assessment.
More recently, an investigational non-invasive device for measuring brain parenchymal resistance (Rp) was introduced, offering a promising method for predicting sleep-active glymphatic function (11). This approach demonstrated that glymphatic activity in humans also increases with higher EEG delta power, lower EEG beta power, and reduced heart rate—mirroring findings in animal models (9). Because this device relies on measuring Rp, it provides high temporal resolution (nearly continuous measurement) but has limited anatomical information.
The glymphatic system plays a crucial role in clearing amyloid-beta and tau from CSF and the brain (14, 16, 17). Consequently, dysfunction of glymphatic system can contribute to the pathophysiology of dementia (2). Furthermore, glymphatic dysfunction and dementia share common risk factors, including aging, sleep abnormalities, and cardiovascular diseases. For example, in older adults or individuals with dementia, NREM sleep—the stage when the glymphatic system is most active—tends to decrease (26, 27). In addition, obstructive sleep apnea, a modifiable risk factor for Alzheimer's disease (AD) and Parkinson's disease (PD) (28), has been associated with reduced glymphatic function (demonstrated by a low ALPS index) (29, 30).
Glymphatic dysfunction has been observed in both AD and PD. Studies indicate reduced ALPS indices in individuals with AD, including those in prodromal and preclinical stages, and these lower ALPS values predict an accelerated accumulation of amyloid-beta on PET imaging (31–33). In PD, a decreased ALPS index is associated with more rapid clinical deterioration, as measured by MDS-UPDRS parts II and III, as well as the Symbol Digit Modalities Test (34, 35). Moreover, a recent meta-analysis encompassing 11 studies on AD and 12 studies on PD provided strong evidence for reduced ALPS indices in both diseases (36).
Additionally, Eide et al. (37) demonstrated impaired glymphatic function in normal pressure hydrocephalus (NPH) through an intrathecal contrast-based MRI study involving 30 patients with idiopathic NPH and 8 control patients. Their findings revealed delayed CSF contrast clearance in NPH, supporting the notion that diminished amyloid-beta clearance may contribute to cognitive dysfunction in this population.
Stroke risk factors, such as diabetes and hypertension, are associated with glymphatic dysfunction (38–41), as discussed in the next section. In turn, stroke itself can cause ipsilateral glymphatic impairment (42). Glymphatic dysfunction could also be a stroke risk factor; because ePVS is associated with increased stroke risk (5, 43); however, this relationship warrants further investigation, given that ePVS is only an indirect marker of glymphatic function.
Glymphatic impairment following stroke predominantly occurs on the side of the infarct (42, 44). Toh et al. (42) reported decreased ALPS indices ipsilateral to the stroke location in patients with ischemic stroke (n = 50) compared with controls (n = 44), and ALPS index inversely correlated with stroke size and improved over time. In a smaller cohort (n = 18) with large-vessel occlusion (LVO), Zhu et al. (44) corroborated these findings, demonstrating impaired ipsilateral glymphatic function early after stroke (days 1 and 3), which then recovered by day 7.
Zhu et al. (44) also proposed that glymphatic function could modulate the extent of brain edema following ischemic stroke. Using a rodent middle cerebral artery occlusion (MCAO) model, they found that glymphatic recovery coincided with improvements in post-stroke edema, despite a worsening of blood–brain barrier dysfunction by day 7 (44, 45). Moreover, pharmacological enhancement of glymphatic activity via adrenergic receptor antagonists alleviated edema by day 2, reduced amyloid-beta deposition, and improved cognitive function (44).
Furthermore, glymphatic dysfunction may play a role in post-stroke epileptogenesis and cognitive impairment (46), potentially through glutamate excitotoxicity and amyloid-beta/tau deposition. EPVS asymmetry has been linked to focal seizures (47), but longitudinal studies in stroke patients are needed to establish the relationship between asymmetric ePVS and the occurrence of focal seizures.
Glymphatic dysfunction is also evident in hemorrhagic stroke—indicated by an increased ePVS burden—and in subarachnoid hemorrhage, where preclinical models have shown altered AQP4 polarization (5). These conditions are discussed in more detail elsewhere (5).
Cerebral small vessel disease (CSVD) and glymphatic dysfunction share common risk factors and etiologies, including hypertension, diabetes, aging, sleep disruption, and neuroinflammation (48). EPVS acts as a marker of CSVD (49) and also serve as an indirect marker of glymphatic dysfunction (18). In older adults, ePVS is associated with the progression of white matter hyperintensities, subcortical infarcts, microbleeds, and vascular dementia (49, 50).
CSVD is generally categorized into two pathophysiological subtypes: amyloid [cerebral amyloid angiopathy (CAA)] and non-amyloid (hypertensive arteriopathy), both of which can present with microbleeds and microinfarcts (51). In the amyloid subtype, CAA is associated with a lower ALPS index, and reduced glymphatic function correlates with a higher CSVD burden, poorer cognitive performance, larger white matter hyperintensities, white matter lacunes, and disease recurrence (52). In non-amyloid CSVD, in addition to lesions (e.g., microinfarcts) that may contribute to glymphatic impairment (53), arteriosclerosis may theoretically further impair the glymphatic system by affecting arterial pulsatility (54).
Recent evidence also indicates that glymphatic dysfunction is linked to cognitive impairment in CSVD (55). Among 133 patients with CSVD, those with cognitive impairment (n = 83) showed a lower ALPS index compared to cognitively normal CSVD individuals (n = 50), after adjusting for multiple variables including other CSVD imaging markers. The ALPS index was positively associated with cognitive test scores (e.g., MoCA, AVLT-sum, SDMT) and negatively correlated with TMT B–A scores (55).
Glymphatic dysfunction has been observed in MS patients (21, 56). Cartenuto et al. (21) reported lower ALPS index in MS patients compared to HC, and progressive MS with even further lower ALPS compared to relapsing-remitting MS. Potential mechanisms for glymphatic disruption in MS include altered CSF dynamics, abnormal AQP4 expression near lesion sites, a predilection for lesions in perivenular areas, and impaired meningeal lymphatic vessels affecting CSF outflow (57).
A lower ALPS index has been reported in patients with traumatic brain injury, and potential mechanisms include sleep impairment and loss of polarized location of AQP4 (58). In patients with TBI, the ALPS index negatively correlating with serum neurofilament levels (59). This suggests that greater axonal injury is associated with more severe glymphatic dysfunction.
Preliminary studies have shown no significant decrease in the DTI-ALPS index among migraine patients (60, 61). However, two recent studies report varying glymphatic function in chronic migraine (62, 63). Further research is needed to clarify the role of the glymphatic system in migraine pathophysiology; it may act as an important modifier by serving as a sink for calcitonin gene-related peptide (64).
Lower glymphatic function has been demonstrated in patients with idiopathic intracranial hypertension (IIH) using intrathecal contrast MRI (15 IIH patients and 15 controls) (65). The study revealed delayed parenchymal clearance of the CSF tracer, particularly in regions such as the hippocampus and entorhinal cortex, which are susceptible to amyloid-beta and tau deposition and are associated with Alzheimer's disease (AD).
Poor sleep quality is a known trigger for migraines, provoked seizures, and delirium, and it is commonly observed in neurodegenerative disorders and depression. The glymphatic system may serve as a critical link between sleep and brain health.
During wakefulness, higher levels of norepinephrine increase brain parenchymal resistance, thereby suppressing glymphatic activity (10, 66). Interestingly, locally blocking norepinephrine receptors in awake mice significantly enhances glymphatic function, bringing it closer to the levels observed during sleep or anesthesia (10, 66).
Sleep architecture is an important determinant of glymphatic function, with the system being most active during NREM sleep, particularly in stage N3 when delta activity is highest. Disruption of N3 sleep has been associated with conditions such as dementia (26, 67), highlighting the potential benefit of interventions aimed at improving slow-wave sleep (67).
Addressing insomnia is thus essential for brain health, and future pharmacological and non-pharmacological interventions should be evaluated for their effects on sleep architecture and, in turn, glymphatic function. For example, in a randomized trial comparing melatonin, temazepam (a benzodiazepine), zolpidem (a benzodiazepine-like drug), and placebo, temazepam and zolpidem significantly decreased NREM slow-wave activity on EEG compared to placebo, whereas melatonin did not significantly alter overall slow-wave activity (68).
Other studies also indicate that benzodiazepines drugs disrupt sleep architecture by reducing delta activity and N3 sleep, which may explain why these agents, despite increasing total sleep duration, fail to improve daytime functioning (26, 69–71). Furthermore, benzodiazepines have been associated with brain atrophy in regions such as the hippocampus and amygdala (72).
Non-pharmacological interventions, such as acoustic stimulation, transcranial direct current stimulation, and transcranial magnetic stimulation, are also demonstrated to enhance slow-wave sleep (26, 73–75). Future research into these non-invasive methods and their impact on glymphatic function will be particularly valuable.
Finally, sleep-related breathing disorders can also play a relevant role in glymphatic dysfunction. For example, in patients with obstructive sleep apnea, the ALPS index has been reported to decrease, correlating with disease severity (29, 30).
Depression is frequently accompanied by insomnia and linked to increased REM sleep and decreased slow-wave sleep (76). The effects of antidepressant medications on the glymphatic system are not well studied. Nevertheless, antidepressants have varying impacts on sleep architecture. For example, SSRIs and SNRIs typically increase REM latency, may have mixed effects on slow-wave sleep, and can reduce sleep continuity in the short term (77). Clinicians should be mindful of these effects when managing sleep disturbances in patients with depression.
Cardiovascular health is critical for glymphatic function, as it relies on arterial pulsatility to drive CSF through PVS and into the interstitial fluid (ISF) of the brain.
Cardiovascular diseases that impair cardiac output, such as heart failure with reduced ejection fraction or arrhythmias, as well as conditions that reduce arterial wall elasticity—such as arteriosclerosis, hypertension, small vessel disease, and atherosclerosis—can theoretically disrupt CSF flow through the PVS in the glymphatic (2). In humans, hypertension is associated with glymphatic dysfunction, reflected by a lower ALPS index (41). Similarly, in mouse models, hypertension impairs glymphatic function, increases ePVS, and disrupts AQP4 polarity (54).
Diabetes, another significant cardiovascular risk factor, has also been associated with a decreased ALPS index (38–40). Moreover, insulin resistance has been shown to negatively correlate with glymphatic function as well (39).
Exercise improves overall cardiovascular health and can enhance glymphatic system during both the awake and sleeping states (78–81), through mechanisms such as improved cardiovascular dynamics and sleep quality.
Regular exercise lowers resting heart rate (82), which is associated with higher glymphatic function (11). Exercise also helps regulate hypertension, a condition linked to glymphatic dysfunction, though the causal relationship remains unclear (41). Preclinical mouse studies further support that voluntary running improves glymphatic function, except during active exercise when the brain deprioritizes waste clearance, likely due to the inhibitory effects of norepinephrine (78, 80).
Exercise also impacts sleep architecture. A recent study found that both low-intensity and moderate-to-vigorous physical activity increased NREM sleep in humans, decreased REM sleep, and extended REM latency (81).
The glymphatic system offers a novel framework for understanding the interplay between sleep, exercise, cardiovascular health, and brain function. Glymphatic dysfunction has been implicated in numerous neurological diseases (Figure 2), highlighting the need for further research to determine whether augmenting glymphatic activity can prevent or improve disease outcomes. From neuromodulation in post-stroke recovery to reducing toxic solute burden in neurodegenerative diseases and modulating the neuro-inflammatory milieu, the glymphatic system represents a promising area for clinical neurology.
Advancing this field requires two key steps. First, the development of faster, more accessible, and non-invasive tools to assess glymphatic function in humans is crucial, and progress is already underway (11). Second, more studies are needed to directly target glymphatic function—whether through revisiting established techniques like acoustic stimulation or exploring pharmacological and biological approaches, such as enhancing AQP4 functionality (83).
OC: Visualization, Writing – original draft, Writing – review & editing. AL: Supervision, Writing – review & editing.
The author(s) declare that no financial support was received for the research, authorship, and/or publication of this article.
The authors declare that the research was conducted in the absence of any commercial or financial relationships that could be construed as a potential conflict of interest.
The author(s) declare that Gen AI was used in the creation of this manuscript. Generative AI was used to assist with grammar and spell check in this article.
All claims expressed in this article are solely those of the authors and do not necessarily represent those of their affiliated organizations, or those of the publisher, the editors and the reviewers. Any product that may be evaluated in this article, or claim that may be made by its manufacturer, is not guaranteed or endorsed by the publisher.
1. Iliff JJ, Wang M, Liao Y, Plogg BA, Peng W, Gundersen GA, et al. A paravascular pathway facilitates CSF flow through the brain parenchyma and the clearance of interstitial solutes, including amyloid beta. Sci Transl Med. (2012) 4:147ra11. doi: 10.1126/scitranslmed.3003748
2. Nedergaard M, Goldman SA. Glymphatic failure as a final common pathway to dementia. Science. (2020) 370:50–6. doi: 10.1126/science.abb8739
3. Xu Z, Xiao N, Chen Y, Huang H, Marshall C, Gao J, et al. Deletion of aquaporin-4 in APP/PS1 mice exacerbates brain Abeta accumulation and memory deficits. Mol Neurodegener. (2015) 10:58. doi: 10.1186/s13024-015-0056-1
4. Louveau A, Da Mesquita S, Kipnis J. Lymphatics in neurological disorders: a neuro-lympho-vascular component of multiple sclerosis and Alzheimer's disease? Neuron. (2016) 91:957–73. doi: 10.1016/j.neuron.2016.08.027
5. Lv T, Zhao B, Hu Q, Zhang X. The glymphatic system: a novel therapeutic target for stroke treatment. Front Aging Neurosci. (2021) 13:689098. doi: 10.3389/fnagi.2021.689098
6. Naganawa S, Nakane T, Kawai H, Taoka T. Gd-based contrast enhancement of the perivascular spaces in the Basal Ganglia. Magn Reson Med Sci. (2017) 16:61–5. doi: 10.2463/mrms.mp.2016-0039
7. Ringstad G, Vatnehol SAS, Eide PK. Glymphatic MRI in idiopathic normal pressure hydrocephalus. Brain. (2017) 140:2691–705. doi: 10.1093/brain/awx191
8. Yamamoto EA, Bagley JH, Geltzeiler M, Sanusi OR, Dogan A, Liu JJ, et al. The perivascular space is a conduit for cerebrospinal fluid flow in humans: a proof-of-principle report. Proc Natl Acad Sci USA. (2024) 121:e2407246121. doi: 10.1073/pnas.2407246121
9. Hablitz LM, Vinitsky HS, Sun Q, Staeger FF, Sigurdsson B, Mortensen KN, et al. Increased glymphatic influx is correlated with high EEG delta power and low heart rate in mice under anesthesia. Sci Adv. (2019) 5:eaav5447. doi: 10.1126/sciadv.aav5447
10. Xie L, Kang H, Xu Q, Chen MJ, Liao Y, Thiyagarajan M, et al. Sleep drives metabolite clearance from the adult brain. Science. (2013) 342:373–7. doi: 10.1126/science.1241224
11. Dagum P, Giovangrandi L, Levendovszky SR, Winebaum JJ, Singh T, Cho Y, et al. The use of continuous brain parenchymal impedance dispersion to measure glymphatic function in humans. medRxiv. (2024) 2024:24300933. doi: 10.1101/2024.01.06.24300933
12. Lee H, Xie L, Yu M, Kang H, Feng T, Deane R, et al. The effect of body posture on brain glymphatic transport. J Neurosci. (2015) 35:11034–44. doi: 10.1523/JNEUROSCI.1625-15.2015
13. Iliff JJ, Chen MJ, Plog BA, Zeppenfeld DM, Soltero M, Yang L, et al. Impairment of glymphatic pathway function promotes tau pathology after traumatic brain injury. J Neurosci. (2014) 34:16180–93. doi: 10.1523/JNEUROSCI.3020-14.2014
14. Iliff JJ, Elbert DL, Giovangrandi L, Singh T, Venkatesh VV, Corbellini A, et al. The glymphatic system clears amyloid beta and tau from brain to plasma in humans. medRxiv. (2024) 2024:24311248. doi: 10.1101/2024.07.30.24311248
15. Mestre H, Kostrikov S, Mehta RI, Nedergaard M. Perivascular spaces, glymphatic dysfunction, and small vessel disease. Clin Sci (Lond). (2017) 131:2257–74. doi: 10.1042/CS20160381
16. Ju YS, Ooms SJ, Sutphen C, Macauley SL, Zangrilli MA, Jerome G, et al. Slow wave sleep disruption increases cerebrospinal fluid amyloid-beta levels. Brain. (2017) 140:2104–11. doi: 10.1093/brain/awx148
17. Liu H, Barthelemy NR, Ovod V, Bollinger JG, He Y, Chahin SL, et al. Acute sleep loss decreases CSF-to-blood clearance of Alzheimer's disease biomarkers. Alzheimers Dement. (2023) 19:3055–64. doi: 10.1002/alz.12930
18. Klostranec JM, Vucevic D, Bhatia KD, Kortman HGJ, Krings T, Murphy KP, et al. Current concepts in intracranial interstitial fluid transport and the glymphatic system: part ii-imaging techniques and clinical applications. Radiology. (2021) 301:516–32. doi: 10.1148/radiol.2021204088
19. Ringstad G, Valnes LM, Dale AM, Pripp AH, Vatnehol SS, Emblem KE, et al. Brain-wide glymphatic enhancement and clearance in humans assessed with MRI. JCI Insight. (2018) 3:121537. doi: 10.1172/jci.insight.121537
20. Richmond SB, Rane S, Hanson MR, Albayram M, Iliff JJ, Kernagis D, et al. Quantification approaches for magnetic resonance imaging following intravenous gadolinium injection: a window into brain-wide glymphatic function. Eur J Neurosci. (2023) 57:1689–704. doi: 10.1111/ejn.15974
21. Carotenuto A, Cacciaguerra L, Pagani E, Preziosa P, Filippi M, Rocca MA. Glymphatic system impairment in multiple sclerosis: relation with brain damage and disability. Brain. (2022) 145:2785–95. doi: 10.1093/brain/awab454
22. Taoka T, Masutani Y, Kawai H, Nakane T, Matsuoka K, Yasuno F, et al. Evaluation of glymphatic system activity with the diffusion MR technique: diffusion tensor image analysis along the perivascular space (DTI-ALPS) in Alzheimer's disease cases. Jpn J Radiol. (2017) 35:172–8. doi: 10.1007/s11604-017-0617-z
23. Ringstad G. Glymphatic imaging: a critical look at the DTI-ALPS index. Neuroradiology. (2024) 66:157–60. doi: 10.1007/s00234-023-03270-2
24. Charidimou A, Hong YT, Jager HR, Fox Z, Aigbirhio FI, Fryer TD, et al. White matter perivascular spaces on magnetic resonance imaging: marker of cerebrovascular amyloid burden? Stroke. (2015) 46:1707–9. doi: 10.1161/STROKEAHA.115.009090
25. Doubal FN, MacLullich AM, Ferguson KJ, Dennis MS, Wardlaw JM. Enlarged perivascular spaces on MRI are a feature of cerebral small vessel disease. Stroke. (2010) 41:450–4. doi: 10.1161/STROKEAHA.109.564914
26. Leger D, Debellemaniere E, Rabat A, Bayon V, Benchenane K, Chennaoui M. Slow-wave sleep: from the cell to the clinic. Sleep Med Rev. (2018) 41:113–32. doi: 10.1016/j.smrv.2018.01.008
27. Ohayon MM, Carskadon MA, Guilleminault C, Vitiello MV. Meta-analysis of quantitative sleep parameters from childhood to old age in healthy individuals: developing normative sleep values across the human lifespan. Sleep. (2004) 27:1255–73. doi: 10.1093/sleep/27.7.1255
28. Guay-Gagnon M, Vat S, Forget MF, Tremblay-Gravel M, Ducharme S, Nguyen QD, et al. Sleep apnea and the risk of dementia: a systematic review and meta-analysis. J Sleep Res. (2022) 31:e13589. doi: 10.1111/jsr.13589
29. Roy B, Nunez A, Aysola RS, Kang DW, Vacas S, Kumar R. Impaired glymphatic system actions in obstructive sleep apnea adults. Front Neurosci. (2022) 16:884234. doi: 10.3389/fnins.2022.884234
30. Lee HJ, Lee DA, Shin KJ, Park KM. Glymphatic system dysfunction in obstructive sleep apnea evidenced by DTI-ALPS. Sleep Med. (2022) 89:176–81. doi: 10.1016/j.sleep.2021.12.013
31. Huang SY, Zhang YR, Guo Y, Du J, Ren P, Wu BS, et al. Glymphatic system dysfunction predicts amyloid deposition, neurodegeneration, and clinical progression in Alzheimer's disease. Alzheimers Dement. (2024) 20:3251–69. doi: 10.1002/alz.13789
32. Zhang X, Wang Y, Jiao B, Wang Z, Shi J, Zhang Y, et al. Glymphatic system impairment in Alzheimer's disease: associations with perivascular space volume and cognitive function. Eur Radiol. (2024) 34:1314–23. doi: 10.1007/s00330-023-10122-3
33. Kamagata K, Andica C, Takabayashi K, Saito Y, Taoka T, Nozaki H, et al. Association of MRI indices of glymphatic system with amyloid deposition and cognition in mild cognitive impairment and Alzheimer disease. Neurology. (2022) 99:e2648–e60. doi: 10.1212/WNL.0000000000201300
34. Cai X, Chen Z, He C, Zhang P, Nie K, Qiu Y, et al. Diffusion along perivascular spaces provides evidence interlinking compromised glymphatic function with aging in Parkinson's disease. CNS Neurosci Ther. (2023) 29:111–21. doi: 10.1111/cns.13984
35. He P, Shi L, Li Y, Duan Q, Qiu Y, Feng S, et al. The association of the glymphatic function with Parkinson's disease symptoms: neuroimaging evidence from longitudinal and cross-sectional studies. Ann Neurol. (2023) 94:672–83. doi: 10.1002/ana.26729
36. Costa T, Manuello J, Premi E, Mattioli I, Lasagna L, Lahoz CB, et al. Evaluating the robustness of DTI-ALPS in clinical context: a meta-analytic parallel on Alzheimer's and Parkinson's diseases. Sci Rep. (2024) 14:26381. doi: 10.1038/s41598-024-78132-9
37. Eide PK, Ringstad G. Delayed clearance of cerebrospinal fluid tracer from entorhinal cortex in idiopathic normal pressure hydrocephalus: a glymphatic magnetic resonance imaging study. J Cereb Blood Flow Metab. (2019) 39:1355–68. doi: 10.1177/0271678X18760974
38. Tian B, Zhao C, Liang JL, Zhang HT, Xu YF, Zheng HL, et al. Glymphatic function and its influencing factors in different glucose metabolism states. World J Diabetes. (2024) 15:1537–50. doi: 10.4239/wjd.v15.i7.1537
39. Tuerxun R, Kamagata K, Saito Y, Andica C, Takabayashi K, Uchida W, et al. Assessing interstitial fluid dynamics in type 2 diabetes mellitus and prediabetes cases through diffusion tensor imaging analysis along the perivascular space. Front Aging Neurosci. (2024) 16:1362457. doi: 10.3389/fnagi.2024.1362457
40. Yang G, Deng N, Liu Y, Gu Y, Yao X. Evaluation of glymphatic system using diffusion MR technique in T2DM cases. Front Hum Neurosci. (2020) 14:300. doi: 10.3389/fnhum.2020.00300
41. Kikuta J, Kamagata K, Takabayashi K, Taoka T, Yokota H, Andica C, et al. An investigation of water diffusivity changes along the perivascular space in elderly subjects with hypertension. AJNR Am J Neuroradiol. (2022) 43:48–55. doi: 10.3174/ajnr.A7334
42. Toh CH, Siow TY. Glymphatic dysfunction in patients with ischemic stroke. Front Aging Neurosci. (2021) 13:756249. doi: 10.3389/fnagi.2021.756249
43. Zhang J, Han F, Liang X, Li M, Zhang D, Zhai F, et al. Lacune and large perivascular space: two kinds of cavities are of different risk factors and stroke risk. Cerebrovasc Dis. (2020) 49:522–30. doi: 10.1159/000508732
44. Zhu J, Mo J, Liu K, Chen Q, Li Z, He Y, et al. Glymphatic system impairment contributes to the formation of brain edema after ischemic stroke. Stroke. (2024) 55:1393–404. doi: 10.1161/STROKEAHA.123.045941
45. Zhu J, Li Z, Ji Z, Wu Y, He Y, Liu K, et al. Glycocalyx is critical for blood-brain barrier integrity by suppressing caveolin1-dependent endothelial transcytosis following ischemic stroke. Brain Pathol. (2022) 32:e13006. doi: 10.1111/bpa.13006
46. Hlauschek G, Nicolo JP, Sinclair B, Law M, Yasuda CL, Cendes F, et al. Role of the glymphatic system and perivascular spaces as a potential biomarker for post-stroke epilepsy. Epilepsia Open. (2024) 9:60–76. doi: 10.1002/epi4.12877
47. Feldman RE, Rutland JW, Fields MC, Marcuse LV, Pawha PS, Delman BN, et al. Quantification of perivascular spaces at 7T: A potential MRI biomarker for epilepsy. Seizure. (2018) 54:11–8. doi: 10.1016/j.seizure.2017.11.004
48. Ang PS, Zhang DM, Azizi SA, Norton de. Matos SA, Brorson JR. The glymphatic system and cerebral small vessel disease. J Stroke Cerebrovasc Dis. (2024) 33:107557. doi: 10.1016/j.jstrokecerebrovasdis.2024.107557
49. Ding J, Sigurð*sson S, Jónsson PV, Eiriksdottir G, Charidimou A, Lopez OL, et al. Large perivascular spaces visible on magnetic resonance imaging, cerebral small vessel disease progression, and risk of dementia: the age, gene/environment susceptibility–reykjavik study. JAMA Neurol. (2017) 74:1105–12. doi: 10.1001/jamaneurol.2017.1397
50. Li Y, Li M, Yang L, Qin W, Yang S, Yuan J, et al. The relationship between blood-brain barrier permeability and enlarged perivascular spaces: a cross-sectional study. Clin Interv Aging. (2019) 14:871–8. doi: 10.2147/CIA.S204269
51. Cuadrado-Godia E, Dwivedi P, Sharma S, Ois Santiago A, Roquer Gonzalez J, Balcells M, et al. Cerebral small vessel disease: a review focusing on pathophysiology, biomarkers, and machine learning strategies. J Stroke. (2018) 20:302–20. doi: 10.5853/jos.2017.02922
52. Xu J, Su Y, Fu J, Wang X, Nguchu BA, Qiu B, et al. Glymphatic dysfunction correlates with severity of small vessel disease and cognitive impairment in cerebral amyloid angiopathy. Eur J Neurol. (2022) 29:2895–904. doi: 10.1111/ene.15450
53. Wang M, Ding F, Deng S, Guo X, Wang W, Iliff JJ, et al. Focal solute trapping and global glymphatic pathway impairment in a murine model of multiple microinfarcts. J Neurosci. (2017) 37:2870–7. doi: 10.1523/JNEUROSCI.2112-16.2017
54. Xue Y, Liu N, Zhang M, Ren X, Tang J, Fu J. Concomitant enlargement of perivascular spaces and decrease in glymphatic transport in an animal model of cerebral small vessel disease. Brain Res Bull. (2020) 161:78–83. doi: 10.1016/j.brainresbull.2020.04.008
55. Tang J, Zhang M, Liu N, Xue Y, Ren X, Huang Q, et al. The association between glymphatic system dysfunction and cognitive impairment in cerebral small vessel disease. Front Aging Neurosci. (2022) 14:916633. doi: 10.3389/fnagi.2022.916633
56. Ding Z, Fan X, Zhang Y, Yao M, Wang G, Dong Y, et al. The glymphatic system: a new perspective on brain diseases. Front Aging Neurosci. (2023) 15:1179988. doi: 10.3389/fnagi.2023.1179988
57. Alghanimy A, Work LM, Holmes WM. The glymphatic system and multiple sclerosis: an evolving connection. Mult Scler Relat Disord. (2024) 83:105456. doi: 10.1016/j.msard.2024.105456
58. Gao Y, Liu K, Zhu J. Glymphatic system: an emerging therapeutic approach for neurological disorders. Front Mol Neurosci. (2023) 16:1138769. doi: 10.3389/fnmol.2023.1138769
59. Butler T, Zhou L, Ozsahin I, Wang XH, Garetti J, Zetterberg H, et al. Glymphatic clearance estimated using diffusion tensor imaging along perivascular spaces is reduced after traumatic brain injury and correlates with plasma neurofilament light, a biomarker of injury severity. Brain Commun. (2023) 5:fcad134. doi: 10.1093/braincomms/fcad134
60. Cao Y, Huang M, Fu F, Chen K, Liu K, Cheng J, et al. Abnormally glymphatic system functional in patients with migraine: a diffusion kurtosis imaging study. J Headache Pain. (2024) 25:118. doi: 10.1186/s10194-024-01825-z
61. Lee DA, Lee HJ, Park KM. Normal glymphatic system function in patients with migraine: a pilot study. Headache. (2022) 62:718–25. doi: 10.1111/head.14320
62. Wu CH, Chang FC, Wang YF, Lirng JF, Wu HM, Pan LH, et al. Impaired glymphatic and meningeal lymphatic functions in patients with chronic migraine. Ann Neurol. (2024) 95:583–95. doi: 10.1002/ana.26842
63. Zhang X, Wang W, Bai X, Zhang X, Yuan Z, Jiao B, et al. Increased glymphatic system activity in migraine chronification by diffusion tensor image analysis along the perivascular space. J Headache Pain. (2023) 24:147. doi: 10.1186/s10194-023-01673-3
64. Messlinger K. The big CGRP flood - sources, sinks and signalling sites in the trigeminovascular system. J Headache Pain. (2018) 19:22. doi: 10.1186/s10194-018-0848-0
65. Eide PK, Pripp AH, Ringstad G, Valnes LM. Impaired glymphatic function in idiopathic intracranial hypertension. Brain Commun. (2021) 3:fcab043. doi: 10.1093/braincomms/fcab043
66. Jessen NA, Munk AS, Lundgaard I, Nedergaard M. The glymphatic system: a beginner's guide. Neurochem Res. (2015) 40:2583–99. doi: 10.1007/s11064-015-1581-6
67. Himali JJ, Baril AA, Cavuoto MG, Yiallourou S, Wiedner CD, Himali D, et al. Association between slow-wave sleep loss and incident dementia. JAMA Neurol. (2023) 80:1326–33. doi: 10.1001/jamaneurol.2023.3889
68. Arbon EL, Knurowska M, Dijk DJ. Randomised clinical trial of the effects of prolonged-release melatonin, temazepam and zolpidem on slow-wave activity during sleep in healthy people. J Psychopharmacol. (2015) 29:764–76. doi: 10.1177/0269881115581963
69. Gillin H-PLaIC. GABA(A1a) Receptors - Involvement in Sleep Regulation and Potential of Selective Agonists in the Treatment of Insomnia. CNS Drugs. (2000). Available at: https://www.researchgate.net/publication/224953804_GABAA1a_receptors_-_Involvement_in_sleep_regulation_and_potential_of_selective_agonists_in_the_treatment_of_insomnia
70. Roehrs T, Roth T. Drug-related sleep stage changes: functional significance and clinical relevance. Sleep Med Clin. (2010) 5:559–70. doi: 10.1016/j.jsmc.2010.08.002
71. Dawson J, Boyle J, Stanley N, Johnsen S, Hindmarch I, Skene DJ. Benzodiazepine-induced reduction in activity mirrors decrements in cognitive and psychomotor performance. Hum Psychopharmacol. (2008) 23:605–13. doi: 10.1002/hup.961
72. Hofe IV, Stricker BH, Vernooij MW, Ikram MK, Ikram MA, Wolters FJ. Benzodiazepine use in relation to long-term dementia risk and imaging markers of neurodegeneration: a population-based study. BMC Med. (2024) 22:266. doi: 10.1186/s12916-024-03437-5
73. Van den Bulcke L, Peeters AM, Heremans E, Davidoff H, Borzee P, De Vos M, et al. Acoustic stimulation as a promising technique to enhance slow-wave sleep in Alzheimer's disease: results of a pilot study. J Clin Sleep Med. (2023) 19:2107–12. doi: 10.5664/jcsm.10778
74. Wunderlin M, Zeller CJ, Senti SR, Feher KD, Suppiger D, Wyss P, et al. Acoustic stimulation during sleep predicts long-lasting increases in memory performance and beneficial amyloid response in older adults. Age Ageing. (2023) 52:228. doi: 10.1093/ageing/afad228
75. Huber R, Esser SK, Ferrarelli F, Massimini M, Peterson MJ, Tononi G. TMS-induced cortical potentiation during wakefulness locally increases slow wave activity during sleep. PLoS ONE. (2007) 2:e276. doi: 10.1371/journal.pone.0000276
76. Medina AB, Lechuga DA, Escandon OS, Moctezuma JV. Update of sleep alterations in depression. Sleep Sci. (2014) 7:165–9. doi: 10.1016/j.slsci.2014.09.015
77. Wichniak A, Wierzbicka A, Jernajczyk W. Sleep and antidepressant treatment. Curr Pharm Des. (2012) 18:5802–17. doi: 10.2174/138161212803523608
78. He XF, Liu DX, Zhang Q, Liang FY Dai GY, Zeng JS, et al. Voluntary exercise promotes glymphatic clearance of amyloid beta and reduces the activation of astrocytes and microglia in aged mice. Front Mol Neurosci. (2017) 10:144. doi: 10.3389/fnmol.2017.00144
79. Olegario RL, Nobrega OT, Camargos EF. The newly discovered glymphatic system: the missing link between physical exercise and brain health? Front Integr Neurosci. (2024) 18:1349563. doi: 10.3389/fnint.2024.1349563
80. von Holstein-Rathlou S, Petersen NC, Nedergaard M. Voluntary running enhances glymphatic influx in awake behaving, young mice. Neurosci Lett. (2018) 662:253–8. doi: 10.1016/j.neulet.2017.10.035
81. Zapalac K, Miller M, Champagne FA, Schnyer DM, Baird B. The effects of physical activity on sleep architecture and mood in naturalistic environments. Sci Rep. (2024) 14:5637. doi: 10.1038/s41598-024-56332-7
82. Reimers AK, Knapp G, Reimers CD. Effects of exercise on the resting heart rate: a systematic review and meta-analysis of interventional studies. J Clin Med. (2018) 7:7120503. doi: 10.3390/jcm7120503
Keywords: glymphatic system, perivascular space, slow wave sleep, brain health, dementia, CSVD, stroke
Citation: Corbali O and Levey AI (2025) Glymphatic system in neurological disorders and implications for brain health. Front. Neurol. 16:1543725. doi: 10.3389/fneur.2025.1543725
Received: 11 December 2024; Accepted: 15 January 2025;
Published: 05 February 2025.
Edited by:
Rafael Rehwald, University College London, United KingdomReviewed by:
Ambra Stefani, Innsbruck Medical University, AustriaCopyright © 2025 Corbali and Levey. This is an open-access article distributed under the terms of the Creative Commons Attribution License (CC BY). The use, distribution or reproduction in other forums is permitted, provided the original author(s) and the copyright owner(s) are credited and that the original publication in this journal is cited, in accordance with accepted academic practice. No use, distribution or reproduction is permitted which does not comply with these terms.
*Correspondence: Osman Corbali, bWNvcmJhbEBlbW9yeS5lZHU=
Disclaimer: All claims expressed in this article are solely those of the authors and do not necessarily represent those of their affiliated organizations, or those of the publisher, the editors and the reviewers. Any product that may be evaluated in this article or claim that may be made by its manufacturer is not guaranteed or endorsed by the publisher.
Research integrity at Frontiers
Learn more about the work of our research integrity team to safeguard the quality of each article we publish.