- 1Department of Ophthalmology, The Third Medical Center, Chinese PLA General Hospital, Beijing, China
- 2Medical School of Chinese PLA, Beijing, China
Dementia and cognitive impairment (CIM) carry high levels of mortality. Visual impairment (VI) is linked with CIM risk. High myopia (HM) is a chronic disease frequently leading to irreversible blindness. Current opinion has shifted from retinal injury as the cause of HM to the condition being considered an eye-brain disease. However, the pathogenesis of this disease and the manner in which neural structures are damaged are poorly understood. This review comprehensively discusses the relationship between HM, the central nervous system, and CIM, together with the novel concept of three visual pathways, and possible research perspectives.
Introduction
The prevalence of cognitive impairment (CIM) is estimated to triple by 2050 (1), as the proportion of the population over the age of 60 is predicted to reach 2 billion people by 2050 (2). CIM is the typical symptom of Alzheimer's dementia. Currently, approximately 6.2 million people in the USA are thought to suffer from Alzheimer's disease (AD) (3), and this could increase to 13.8 million by 2060 without the development of new treatments for the disease (3). AD and other types of dementia are ranked among the top 10 causes of death throughout the world and were ranked third over North and South America and Europe in 2019 by the WHO in 2020.
CIM currently ranks fifth in causes of disability in the elderly (4), leading to often severe challenges, including economic and social as well as psychological and physical burdens to the patients, families, and communities (5, 6). There are limited treatments for dementia (7). Thus, the identification of risk factors that could be addressed in the early stages of the disorder may be more effective than pharmaceutical strategies. This review discusses the role of visual impairment (VI) as a CIM risk factor (8).
Myopia is estimated to affect 28.3% (~2 billion individuals) of the world's population. Of these, 277 million (4.0% of the overall population) suffer from high myopia (HM). HM is the most common cause of blindness in some communities (9, 10). Worse still, HM is expected to increase in prevalence to affect almost 1 billion individuals or 9.8% of the global population in 2050 (11).
Both VI and CIM are relatively common in the elderly and result in significant economic and social burdens. These burdens could be reduced if the disorders could be timeously detected and suitably managed. VI is a documented CIM risk factor. Thus, HM-associated VI may be linked to cognitive decline. A review of the current literature shows that few studies have specifically addressed this issue, with most focusing on the relationships between neural morphology and function, using neuroimaging techniques. Moreover, many of these findings are equivocal. Here, we review the underlying concepts and current published evidence to investigate and understand the association between HM and CIM.
Association of ophthalmic manifestations with neurogeneration
The brain is specifically linked to the sense organs. In the case of vision, the eye forms an extension of the brain with both organs consisting of neurons derived from the embryonic neural tube. The eye is often described as a “window into the brain” as considerable amounts of vascular and neuroanatomical information on the brain can be collected in a non-invasive manner through the eye. This strong association between the eye and the central nervous system (CNS) is confirmed by the observation that ophthalmic changes occur during neurogenerative disorders of the CNS (12–14). For instance, in AD, β-amyloid plaques are seen not only in the brain but also in the inner retina (15, 16). As it is relatively simple to evaluate the retina using optical techniques such as optical coherence tomography (OCT), specific changes, such as thinning of the retinal ganglion cell layer (GCL) have been detected in early neurodegeneration, reflecting changes occurring in the brain (17). In addition, OCT can identify significant changes in the intraretinal vascular network in AD (18). A variety of ophthalmic problems have been described in patients suffering from neurodegeneration, most commonly AD, including damage to the extraocular muscles, changes in the pupil, and thinning of the RNFL and GCL, as well as visual complaints, such as reduced color and contrast sensitivities, and changes to the visual field (19–26). These issues have also been reported in other neurodegenerative diseases, specifically, Parkinson's disease (PD). PD is accompanied by a wide range of visual problems, involving most of the ocular and visual system components (27, 28), and appear to be identical to the problems described in AD. It thus appears that the eye is not merely a reflection of the neurodegenerative changes occurring within the CNS but may be directly linked with the pathogenesis. In this section, we discuss the eye in the context of injury caused by pathological changes originating within the brain. The question arises whether primary degenerative changes in ocular structures can induce changes in the CNS. Evidence supporting this has shown that neurodegenerative processes involve both the central nervous system and the retina, with anterograde (postsynaptic neurons) and retrograde (presynaptic neurons) transsynaptic neurodegeneration (29). Retrograde transsynaptic neurodegeneration frequently leads to the death of retinal ganglion cells. The following section discusses changes in the CNS resulting from primary disorders of the eye.
Neurodegenerative manifestations in ophthalmic diseases
The potential participation of the CNS in ophthalmic disease has attracted the attention of both the scientific and clinical communities (30). The optic nerve forms a direct connection between the eye and the brain, and recent evidence has suggested the existence of interrelationships between the two organs on both morphological and functional levels, including the sharing of neurodegenerative features (29, 31). The role of retrograde transsynaptic degeneration has recently been documented in the visual system, specifically in connection with glaucoma (32–34). This mechanism has also been linked to disorders of other posterior segments. Age-related macular degeneration (AMD) leads to degeneration of visual pathways associated with alterations of the connectivity networks in the CNS, resulting in visual impairment (35–37). Genetic retinal dystrophies may show similar features, including alterations in white matter networks and cortical remapping (38–40). Neuronal damage leads to disrupted intercellular communication to the point where entire networks collapse. In transsynaptic degeneration, the damage extends from the primary injury site to distant sites through neuronal communication. Changes in the peripheral visual system initially lead to attempted local compensation, followed at later stages by spreading to the brain accompanied by further impairment (41). Thus, although insufficiently investigated, the interconnections between the eye and the CNS are likely to have profound implications for both understanding visual processing and diseases affecting the two organs.
VI is a risk factor for CIM
In mammals, stimuli entering the eye are processed initially by the retinal mosaic and transmitted to the retinal ganglion cells. The axons of these cells constitute the optic nerve, leading to the lateral geniculate nucleus in the thalamus, after which the signal is transmitted to the primary visual cortex (42, 43) (Figure 1). Thus, retinal damage affects a variety of brain regions and systems, including the subcortical retinofugal system, and the primary, higher, and non-visual cortical areas (44–48). Retinal damage can result in VI, which is a risk factor for CIM (8). Indeed, it has been suggested that VI may be an early symptom of CIM (49).
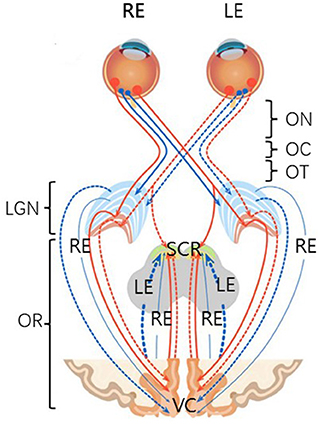
Figure 1. A schematic anatomical overview of the visual system. There are six major subdivisions, including optic nerve (ON), optic chiasm (OC), optic tract (OT), lateral geniculate nucleus of the thalamus (LGN), optic radiation (OR), and visual cortex (VC). Four non-decussating fiber pathways (nasal fibers, blue, and orange) connect the optic nerve and the optic tract in the same hemisphere, while four decussating fiber pathways (lateral fibers, blue, and orange) connect the optic nerve and the optic tract across the hemispheres. SCR, subcortical regions.
Neuroimaging investigations have demonstrated changes in the brain not only in neuropsychiatric disorders, such as CIM but also in disorders involving long-term aberrations in sensory input. Specifically, both hearing disorders and amblyopia lead to reductions in the gray matter of the brain. Furthermore, a number of systematic reviews and meta-analyses have suggested that sensory impairment, including VI and hearing and olfactory deficiencies, are risk factors for CIM (8, 50, 51). The brain is known to obtain over 80% of its information concerning the environment through the eyes (52). However, the mechanisms responsible for these risks are largely unknown. In VI, it is possible that the loss of visual information may result in cortical atrophy, leading to reorganizational changes in the brain, which have been reported by neuroimaging studies and pathological evaluations (12, 53, 54). In addition, damage to the visual input may lead to impaired perceptual processing, leading, in turn, to reductions in higher-order cognitive functioning (55). Thus, it is suggested that disruptions in the normal retina-brain connection by neurodegenerative changes in CIM may coincide with VI.
HM is one of the most common causes of retinopathy-induced irreversible VI, especially in older adults (≥50 years) (56). As HM is associated with both hypopsia and fundus lesions, it is essential to explore the structural and functional aberrations in the brain associated with HM.
Associations between CIM and HM
The link between CIM and myopia is a relatively novel concept and cognitive changes associated with HM are not well-documented (57), with a limited number of studies reporting disparate results. There is a certain amount of epidemiological evidence suggesting a link between myopia and cognitive dysfunction, for example, myopic patients have an almost 2-fold increased likelihood of suffering cognitive dysfunction compared with those with normal vision; this relationship remained significant after adjustment for age, sex, income, education, body mass index, and hours of reading and writing per day, as well as for uncorrected refractive errors or best-corrected visual acuity (58, 59). In contrast, another study found no significant association (60).
Despite correction for visual acuity, the functional performance of HM eyes, including contrast sensitivity (61), acuity of resolution (62), visual attention (63, 64), performance on backward visual masking tasks, and temporal retinal responses assessed by electrophysiological techniques (65), remains abnormal compared with individuals with normal vision.
Mammalian behavior is strongly regulated by the solar cycle, and light produces significant effects on both mood and cognition. In humans, light detection occurs exclusively in the retina. The photoreceptors include the classic rods and cones as well as a subpopulation of retinal ganglion cells that express the photopigment melanopsin (Opn4); these cells are described as intrinsically photosensitive retinal ganglion cells (ipRGCs) (66, 67). The ipRGCs mediate the influence of light through two pathways, namely, targeting the suprachiasmatic nucleus (SCN) in the hypothalamus and the perihabenular nucleus (PHb) in the dorsal thalamus (68). Ablation of the ipRGCs has been shown to completely block light perception in the SCN, indicating that the ipRGCs may be necessary for the modulation of circadian brain functions by light (69). The extended axial length in HM usually results in retinal thinning and retinal thickness has been found to decrease with HM severity. This may also affect the ganglion cells as mediators of mood and cognition.
CIM/Dementia is an overall description that includes a specific group of symptoms associated with deficiencies in memory, language, problem-solving, and cognitive skills that adversely affect the patient's performance in everyday activities. Specific neural regions are involved in cognitive activities, and HM is known to be linked with structural and functional alterations in both the visual and non-visual cortical areas. These overlaps are discussed below.
For example, a report on HM patients (70), observed reductions in the thickness of the cortical surface in the right primary visual area 1 (V1), right occipital pole, and primary motor cortex, amongst others, that were not apparent in healthy controls. Damage to V1 may lead to vision loss while the occipital pole functions during early visual and speech processing, spelling to sound conversion, and modulation of the visual cortex. Another article on HM patients (71) showed white matter abnormalities in the brain regions responsible for motor conduction and vision-related functions. Zhai et al. (72) observed, using fMRI, that HM patients had significantly reduced functional connectivity (FC) between the supramarginal gyrus and rostrolateral prefrontal cortex, together with their associated ventral attention and frontoparietal control networks. These findings indicate the presence of FC alterations in HM and may account for attention deficiency observed in patients with HM. HM is associated with dysfunctional changes in many regions of the brain, possibly indicative of neurobiological alterations associated with the understanding of language and regulation of attention (73). Alterations in the default mode network (DMN), formed by the posterior cingulate cortex, medial prefrontal cortex, and bilateral parietal cortices, have been observed on rsfMRI on patients with CIM and neurodegeneration (74, 75).
Significantly reduced low-frequency fluctuations (ALFFs) in the right inferior and middle temporal gyrus, as well as the left middle temporal gyrus, have been reported in HM patients (73). These regions are proximate to the superior temporal sulcus (STS) and compute a variety of sensory information relating to social interactions (76, 95). The STS is associated with a variety of brain regions linked with the processing of social information (77–82). It has been found that parts of the STS respond to specific visual and auditory stimuli Including facial expression and gaze (83–93), bodies (16, 17, 80, 88), point-light walkers (15, 68, 79, 89, 90), human voices (20, 91), language (21, 92), and the audiovisual integration of speech (22, 93). Furthermore, the temporoparietal junction (TPJ) which lies posterior and superior to the STS controls theory of mind tasks (94), in which participants interpret the actions of characters in stories.
Given the possible relationship between HM and CIM, the recognition of the association between VI from HM and cognitive dysfunction as a complication of HM will allow more successful management of these disorders, reducing economic and healthcare burdens. The use of neuroimaging further confirms these associations.
Neuroimaging in HM
In this section, the neuroimaging findings are summarized, and we argue that HM-associated changes potentially affect CIM. Abnormal visual experiences are known to influence both the structure and function of the brain. Visual functioning in patients with HM differs significantly from that in individuals with normal vision, despite correction of visual acuity. It is possible that long-term myopic vision may induce alterations in the neural activity of the brain. The most commonly used neuroimaging technique is MRI, which is able to evaluate structure, function, and metabolism throughout the nervous system. However, to the best of our knowledge, there are no reports on metabolic MRI in HM.
In terms of structure, MRI has shown reduced cortical thickness and disconnection in patients with HM (70) where thinning of the cortical surface was seen in the left middle occipital gyrus (MOG), left inferior parietal lobe (IPL), right inferior temporal gyrus (ITG), right precuneus, right primary visual area 1 (V1), right superior temporal gyrus (STG), right superior parietal lobule (SPL), right occipital pole, and right primary motor cortex (M1). Higher white matter concentrations have also been reported in HM patients, particularly in the calcarine area, as well as in smaller areas of the prefrontal and parietal lobes (95). Additional abnormalities in the white matter were observed in the bilateral corticospinal tract, right inferior longitudinal fasciculus, superior longitudinal fasciculus, inferior fronto-occipital fasciculus, and left thalamus (71). A further study using a specific MRI sequence, observed abnormal blood perfusion in the cerebellum of patients with HM (96), providing a different perspective on changes and plasticity in the brains of HM patients.
Brain FC in HM patients has been investigated using fMRI. For instance, Zhai et al. (72) described reduced FC density (FCD) in the posterior cingulate cortex/precuneus of HM patients, together with reduced long-range FCD in the inferior temporal gyrus, supramarginal gyrus, and rostrolateral prefrontal cortex. The reduced FC was seen not only in these regions but also in the networks they belong to, namely, the ventral attention and frontoparietal control networks. These findings indicate changes in FC in HM and may assist in explaining attention deficits often seen in high myopes.
Moreover, at-rest functional MRI (RS-fMRI) using BOLD signals has been successfully used for the investigation of spontaneous neural activity in myopia. One study using this technique (73) observed that patients with HM showed significantly reduced ALFFs in the right inferior and middle temporal gyrus, left middle temporal gyrus, left inferior frontal gyrus/putamen, right inferior frontal gyrus/putamen/insula, right middle frontal gyrus, and right inferior parietal lobule, with raised ALFFs in the bilateral midcingulate cortex, left postcentral gyrus, and left precuneus/inferior parietal lobule. Patients with HM showed multiple abnormalities, which may account for deficiencies in understanding language and the regulation of attention seen in HM patients. A further study compared brain activity between patients with low or moderate myopia (LMM) and those with HM in terms of the ALFF (97), finding differences in activity in the limbic system, default mode network, and thalamo-occipital pathway. Specifically, although abnormalities in the visual and sensorimotor regions of the brain were seen in both LMM and HM patients, those with HM showed greater abnormalities in the border brain areas. In addition, patients with HM showed aberrant activity in both the limbic system and default mode network (DMN). Changes in the DMN which includes the posterior cingulate cortex (PCC), medial prefrontal cortex (mPFC), and bilateral parietal cortices in RS-fMRI have been reported in both CIM and neurodegeneration (74, 75). All the above brain regions, together with the adjacent areas, may be associated with the processing of information concerning cognition-related social interactions.
The question arises as to whether these cortical changes associated with HM are reversible. The answer lies in the plasticity of the adult cerebral cortex. The visual system is considered to be sufficiently plastic to allow modifications of the cerebral cortex only during the first decade of life. However, evidence suggests that the adult visual system may also have some degree of neural plasticity. A specific example concerns the use of refractive surgery for myopia. Abnormal activation of the visual cortex has been reported after corneal refractive surgery for myopia (98); this was observed in all patients with the highest increases in signals seen in the primary visual cortex. Additional signals were observed in the posterior cingulum, anterior cingulum, prefrontal cortex, and frontal cortex. A recent investigation into adult amblyopia observed aberrant FC between the visual cortex and subcortical regions such as the geniculostriate and corticotectal pathways, indicative of the effects of changed visual input on the brain neural activity (43). It has been hypothesized that anisometropic non-amblyopic patients may also suffer relative visual deprivation and that this could be reduced after refractive surgery by plasticity in the visual cortex. This was supported by a study on the effects of refractive surgery in anisometropic adult patients (99) that observed plastic changes in the primary visual cortex. Refractive surgery was found to improve the BSCVA in these patients, counteracting the idea that such visual defects are permanent (100). The control group comprised myopic non-anisometropic subjects. While improvement in visual acuity was observed in both groups, the improvement in the anisometropic patients was slower. As shown by fMRI studies on neuronal, specifically synaptic, activity in relation to increased blood flow, it appears that the visual cortex in adult anisometropic subjects undergoes plastic changes after refractive surgery (101).
Current limitations and future perspectives
MRI-HM analysis has demonstrated that HM results in changes in both the FC and morphology of the brain cortices. Despite limited epidemiological evidence linking HM and cognitive dysfunction (58–60), relatively little is known of cognitive changes in the presence of HM. To clarify the situation, two limitations of past studies should be discussed.
Lack of non-invasive neuroimaging with high spatial resolution in HM
The spatial resolution of 1.5 and 3T MRI imaging is relatively low, suggesting that equipment, such as 7T MRI, should be used for higher image resolution in HM investigations. At present, 7T MRI represents the most effective non-invasive technique for neuroimaging. The increased signal-to-noise ratios (SNRs) of 7T compared with conventional 1.5 and 3 T scanners have led to discussions on the benefits of the technology to neuro-ophthalmology (102, 103). Due to the technical limitations in identifying layer-specific activities in the subcortical nuclei in HM, very little is known of the effects of HM on the neuronal responses and circuitry of the subcortical visual pathways.
The initial stages of visual perception are controlled by two parallel pathways (retinogeniculocortical pathways), namely, the magnocellular and parvocellular pathways, which each transmit different types of visual information (43, 45, 104, 105). Physiological and behavioral studies have shown that the magnocellular pathway is most affected in HM, with patients showing impaired perception of motion and blue-yellow contrast (104). To date, there have been no reports on the neuroimaging of the retinogeniculocortical pathways in HM. However, these pathways, together with other subcortical regions, have been investigated in amblyopia using 7 T MRI (43).
No common framework for investigating the primate visual cortex
There appears to be no consensus on the cortical regions associated with HM from MRI studies. A possible explanation is the lack of a common framework for the investigation of the primate visual cortex.
A recent report by Pitcher, D. et al. described a third visual pathway specifically adapted for social perception [Figure 2, (106)]. These authors proposed the presence of a third visual pathway on the lateral brain surface that is anatomically distinct from the dorsal and ventral pathways. This third pathway is present in both human and non-human primates. In humans, the pathway projects from the early visual cortex into the superior temporal sulcus (STS) (77–82), which has been found to be associated with social cognition. This indicates that the two-visual pathway model of the primate visual cortex requires updating. The ventral pathway is responsible for identifying an object, while the dorsal pathway analyzes the object's location and actions associated with the object. Thus, the two-pathway model is essentially concerned with the “what,” “where,” and “how” of visual object recognition. However, while these attributes can describe many characteristics of visual objects, the terms are wholly inadequate for assessing the complexity and nuances of even basic social interactions. It is apparent that revision to this model is necessary. The presence of the third visual pathway has been documented by tractography investigations in humans and tracer and neuroanatomical studies in non-human primates (106). Various STS subregions have been shown to respond to specific types of social stimuli, such as visual or auditory stimuli, in a posterior-to-anterior direction. It has been reported that the STS is able to compute facial and body movements, such as expression, eye gaze, intention, audio-visual integration, and mood, suggesting specialization of the third pathway for the dynamic aspects of social perception (88, 91, 93, 107, 108). These functions of the STS and, by extension, of the third pathway, are well-documented (88, 107, 108). These findings create a framework for the further study of the cognitive functions of the primate visual cortex. Many experimental methods could be used for this, including neuroimaging (MRI) to evaluate neural responses to abnormal visual stimuli in HM.
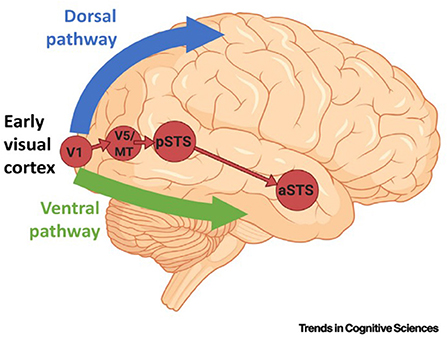
Figure 2. The pathway begins in the primary visual cortex (V1) and projects into the posterior banks of the superior temporal sulcus (STC) via the motion-selective area V5/middle temporal (MT). The corticocortical connections of the third pathway are independent of the ventral pathway (in green) and the dorsal pathway (in blue). a/pSTS, anterior/posterior STS.
Neural plasticity in adulthood
It is feasible that VI may lead to cognitive decline through the limitation of the individual's social activities, resulting in social isolation and reduced participation in mentally stimulating activities (109, 110). Numerous age-associated eye conditions, such as glaucoma and AMD, that are related to VI have been associated with CIM (111, 112). HM is also documented to be a leading cause of VI. CIM results from neuronal injury and death, together with aberrant synaptic communication. Healthy adult brains contain ~100 billion neurons, each of which connects via synapses with other neurons. Synapses allow the rapid transmission of signals within the brain, with the transmitted information forming the cellular basis of cognition.
Brain plasticity may be defined as the capacity of these synaptic connections to adapt in response to stimuli, such as injury, sensory deprivation, and environmental change. Thus, it is possible that changes in the FC or ALFF (shown by MRI) may initiate or promote the progression of neurodegenerative change. AD, which accounts for approximately 60–80% of CIM cases, is progressive and may even start 20 years or more before the appearance of symptoms (113–115). Clinically, the major risk factor for AD is older age (>65 years, although this is not fixed) (116). It has been suggested that degenerative changes in the brain may begin in the fourth decade of life. HM is a major cause of irreversible retinopathy-associated VI, especially in older patients (≥50 years) (56). In addition, HM is a good example of the existence of neural plasticity after development as it is usually of long duration. Plasticity is a distinctive, although not exclusive, feature of the developing nervous system; increasing numbers of experimental studies have indicated that plasticity occurs in the adult human cortex as well (117). It appears that there is a spatial and temporal overlap in the age of onset or progression between HM and CIM.
In terms of the bidirectional association between VI and CIM, Vu, T. A (8) observed using clinically validated cognitive screening tests that VI is a risk factor for CIM, although it is not known if CIM is a risk factor for VI. As both diseases may be present in elderly patients, it is possible that maladaptive alterations in the circuitry of the visual cortices could occur as in neurodegeneration (118). Such changes could result from qualitative or quantitative alterations in neural activity and could be of long duration. As discussed, cortical changes have been demonstrated after corneal refractive surgery, demonstrating the existence of plasticity in the adult cerebral cortex. From this perspective, we, as clinical ophthalmologists, suggest that if HM patients have their refractive surgeries (corneal refraction or posterior chamber phakic intraocular lens) in their third or fourth decades, the chances of their developing CIM later in life will be diminished.
Conclusion
This review discusses the main findings on the commonalities between neurocognitive structure and function in HM and CIM/dementia. Nevertheless, the interpretation of the association between HM and CIM is both complex and equivocal. We consider that neuroimaging may assist in the exploration of the underlying pathophysiology of CIM and HM. Characterization of the neural plasticity and reorganization resulting from VI is necessary for the development of effective disease management and rehabilitation.
The use of functional neuroimaging techniques with higher resolution, such as 7 T fMRI, would improve this type of analysis. In addition, further investigations into the framework of the three visual pathways, including both tractography studies in humans and tracer and neuroanatomical studies in non-human primates are required for a comprehensive evaluation of the bidirectional relationship between HM and CIM and to investigate the efficacy of vision-saving interventions in preventing the onset and progression of cognitive decline.
Author contributions
YH and LW designed the study. KL wrote the initial draft. QW, KL, and YH revised the manuscript. All authors listed have made a substantial, direct, and intellectual contribution to the work and approved it for publication.
Funding
This work was supported by Equipment Comprehensive Scientific Research Project of China (Grant No. LB20201A010024).
Conflict of interest
The authors declare that the research was conducted in the absence of any commercial or financial relationships that could be construed as a potential conflict of interest.
Publisher's note
All claims expressed in this article are solely those of the authors and do not necessarily represent those of their affiliated organizations, or those of the publisher, the editors and the reviewers. Any product that may be evaluated in this article, or claim that may be made by its manufacturer, is not guaranteed or endorsed by the publisher.
References
1. World Health Organization. Dementia. (2019). Available online at: https://www.who.int/news–room/fact–sheets/detail/dementia (accessed November 03, 2020).
2. World Health Organization. Aging and health. (2018). Available online at: https://www.who.int/news-room/fact-sheets/detail/ageing-and-health (accessed November 03, 2020).
3. Rajan KB, Weuve J, Barnes LL, McAninch EA, Wilson RS. Population estimate of people with clinical Alzheimer's disease and mild cognitive impairment in the United States (2020–2060). Alzheimer's Dem J Alzheimer's Assoc. (2021) 3:2362. doi: 10.1002/alz.12362
4. Prince M, Wimo A, Guerchet M. World Alzheimer Report. The global impact of dementia: an analysis of prevalence incidence cost & trends. Alzheimer's Disease International. (2015). Available online at: https://www.alz.co.uk/research/worldalzheimerreport2015summary.pdf (accessed November 03, 2020).
5. World Health Organization. Towards a dementia plan: a WHO guide. (2018). Available online at: https://apps.who.int/iris/bitstream/handle/10665/272642/9789241514132–eng.pdf?ua=1 (accessed November 03, 2020).
6. Global regional and national incidence prevalence and years lived with disability for 354 diseases and injuries for 195 countries and territories 1990–2017: a systematic analysis for the global burden of disease study. Lancet. (2018) 392:1789–858. doi: 10.1016/S0140-6736(18)32279-7
7. Alzheimer's disease facts and figures. Alzheimer's Dem J Alzheimer's Assoc. (2021) 17:327–406. doi: 10.1002/alz.12328
8. Vu TA, Fenwick EK, Gan ATL, Man REK, Tan BKJ, Gupta P, et al. The Bidirectional relationship between vision and cognition: a systematic review and meta–analysis. Ophthalmology. (2021) 128:981–92. doi: 10.1016/j.ophtha.2020.12.010
9. Iwase A, Araie M, Tomidokoro A, Yamamoto T, Shimizu H. Prevalence and causes of low vision and blindness in a Japanese adult population: the Tajimi study. Ophthalmology. (2006) 113:1354–62. doi: 10.1016/j.ophtha.2006.04.022
10. Xu L, Wang Y, Li Y, Wang Y, Cui T, Li J, Jonas JB. Causes of blindness and visual impairment in urban and rural areas in Beijing: the Beijing eye study. Ophthalmology. (2006) 113:1134–11. doi: 10.1016/j.ophtha.2006.01.035
11. Holden BA, Fricke TR, Wilson DA, Jong M, Naidoo KS, Sankaridurg P, et al. Global prevalence of myopia and high myopia and temporal trends from. 2000 through 2050. Ophthalmology. (2016) 123:1036–42. doi: 10.1016/j.ophtha.2016.01.006
12. Voss P, Pike BG, Zatorre RJ. Evidence for both compensatory plastic and disuse atrophy–related neuroanatomical changes in the blind. Brain J Neurol. (2014) 137(Pt 4):1224–40. doi: 10.1093/brain/awu030
13. Liu S, Ong YT, Hilal S, Loke YM, Wong TY, Chen CL, et al. The association between retinal neuronal layer and brain structure is disrupted in patients with cognitive impairment and alzheimer's disease. J Alzheimer's Dis JAD. (2016) 54:585–95. doi: 10.3233/JAD-160067
14. Snyder PJ, Alber J, Alt C, Bain LJ, Bouma BE, Bouwman FH, et al. Retinal imaging in Alzheimer's and neurodegenerative diseases. Alzheimer's Dementia J Alzheimer's Assoc. (2021) 17:103–11. doi: 10.1002/alz.12179
15. Ning A, Cui J, To E, Ashe KH. Amyloid–beta deposits lead to retinal degeneration in a mouse model of Alzheimer disease. Invest Ophthalmol Visual Sci. (2008) 49:5136–43. doi: 10.1167/iovs.08-1849
16. Koronyo Y, Salumbides BC, Black KL, Koronyo-Hamaoui M. Alzheimer's disease in the retina: imaging retinal aβ plaques for early diagnosis and therapy assessment. Neuro–Degen Dis. (2012) 10:285–93. doi: 10.1159/000335154
17. Czakó C, Kovács T, Ungvari Z, Csiszar A, Yabluchanskiy A, Conley S, et al. Retinal biomarkers for Alzheimer's disease and vascular cognitive impairment and dementia (VCID): implication for early diagnosis and prognosis. GeroScience. (2020) 42:1499–525. doi: 10.1007/s11357-020-00252-7
18. Wang X, Zhao Q, Tao R, Lu H, Xiao Z, Zheng L, et al. Decreased retinal vascular density in Alzheimer's disease (AD) and mild cognitive impairment (MCI): an optical coherence tomography angiography (OCTA) study. Front Aging Neurosci. (2020) 12:572484. doi: 10.3389/fnagi.2020.572484
19. Hinton DR, Sadun AA, Blanks JC, Miller CA. Optic–nerve degeneration in Alzheimer's disease. New Eng J Med. (1986) 315:485–7. doi: 10.1056/NEJM198608213150804
20. Trick GL, Trick LR, Morris P. Visual field loss in senile dementia of the Alzheimer's type. Neurology. (1995) 45:68–74. doi: 10.1212/WNL.45.1.68
21. Rizzo M, Anderson SW, Dawson J, Nawrot M. Vision and cognition in Alzheimer's disease. Neuropsychologia. (2000) 38:1157–69. doi: 10.1016/S0028-3932(00)00023-3
22. Scinto LF. ApoE allelic variability influences pupil response to cholinergic challenge and cognitive impairment. Genes Brain Behav. (2007) 6:209–15. doi: 10.1111/j.1601-183X.2006.00247.x
23. Garbutt S, Matlin A, Hellmuth J, Schenk AK, Johnson JK, Rosen H, et al. Oculomotor function in frontotemporal lobar degeneration related disorders and Alzheimer's disease. Brain: a J Neurol. (2008) 131(Pt 5):1268–81. doi: 10.1093/brain/awn047
24. Lee YT, Pai MC. Recognition of personally familiar scenes in patients with very mild Alzheimer's disease: effects of spatial frequency and luminance. J Alzheimer's Dis JAD. (2012) 29:441–8. doi: 10.3233/JAD-2011-111601
25. Risacher SL, Wudunn D, Pepin SM, MaGee TR, McDonald BC, Flashman LA, et al. Visual contrast sensitivity in Alzheimer's disease mild cognitive impairment and older adults with cognitive complaints. Neurobiol Aging. (2013) 34:1133–44. doi: 10.1016/j.neurobiolaging.2012.08.007
26. Liu D, Zhang L, Li Z, Zhang X, Wu Y, Yang H, et al. Thinner changes of the retinal nerve fiber layer in patients with mild cognitive impairment and Alzheimer's disease. BMC Neurol. (2015) 15:14. doi: 10.1186/s12883-015-0268-6
27. Armstrong RA. Visual symptoms in Parkinson's disease. Parkinson's Dis. (2011) 2011:908306. doi: 10.4061/2011/908306
28. Sankhla CS. Visual symptoms in Parkinson's disease. Neurology India. (2019) 67:56–58. doi: 10.4103/0028-3886.253603
29. Mancino R, Cesareo M, Martucci A, Di Carlo E, Ciuffoletti E, Giannini C, et al. Neurodegenerative process linking the eye and the brain. Curr Med Chem. (2019). 26:3754–63. doi: 10.2174/0929867325666180307114332
30. Prins D, Hanekamp S, Cornelissen FW. Structural brain MRI studies in eye diseases: are they clinically relevant? A review of current findings. Acta Ophthalmol. (2016) 94:113–21. doi: 10.1111/aos.12825
31. Arrigo A, Aragona E, Saladino A, Arrigo D, Fantaguzzi F, Battaglia Parodi M, et al. Cognitive dysfunctions in glaucoma: an overview of morpho–functional mechanisms and the impact on higher–order visual function. Front Aging Neurosc. (2021) 13:747050. doi: 10.3389/fnagi.2021.747050
32. Lawlor M, Danesh-Meyer H, Levin LA, Davagnanam I, De Vita E, Plant GT. Glaucoma and the brain: trans–synaptic degeneration structural change and implications for neuroprotection. Survey Ophthalmol. (2018) 63:296–306. doi: 10.1016/j.survophthal.2017.09.010
33. Yücel Y, Gupta N. Glaucoma of the brain: a disease model for the study of transsynaptic neural degeneration. Prog Brain Res. (2008) 173:465–78. doi: 10.1016/S0079-6123(08)01132-1
34. You M, Rong R, Zeng Z, Xia X, Ji D. Transneuronal Degeneration in the Brain During Glaucoma. Front Aging Neurosc. (2021) 13:643685. doi: 10.3389/fnagi.2021.643685
35. Lešták J, Tintěra J, Karel I, Svatá Z, Rozsíval P. Functional magnetic resonance imaging in patients with the wet form of age–related macular degeneration. Neuro Ophthalmol. (2013) 37:192–7. doi: 10.3109/01658107.2013.819581
36. Rosengarth K, Keck I, Brandl-Rühle S, Frolo J, Hufendiek K, Greenlee MW, et al. Functional and structural brain modifications induced by oculomotor training in patients with age–related macular degeneration. Frontiers Psychol. (2013) 4:428. doi: 10.3389/fpsyg.2013.00428
37. Hernowo AT, Prins D, Baseler HA, Plank T, Gouws AD, Hooymans JM, et al. Morphometric analyses of the visual pathways in macular degeneration. J Nerv Sys Behav. (2014) 56:99–110. doi: 10.1016/j.cortex.2013.01.003
38. OOlivo G, Melillo P, Cocozza S, D'Alterio FM, Prinster A, Testa F, et al. Cerebral involvement in stargardt's disease: a VBM and TBSS study. InvesteOphthalmol Visual Sci. (2015) 56:7388–97. doi: 10.1167/iovs.15-16899
39. Ferreira S, Pereira AC, Quendera B, Reis A, Silva ED, Castelo–Branco M. Primary visual cortical remapping in patients with inherited peripheral retinal degeneration. NeuroImage. Clin. (2017) 13:428–38. doi: 10.1016/j.nicl.2016.12.013
40. Rita Machado A, Carvalho Pereira A, Ferreira F, Ferreira S, Quendera B, Silva E, et al. Structure–function correlations in Retinitis Pigmentosa patients with partially preserved vision: a voxel–based morphometry study. Scient Reports. (2017) 7:11411. doi: 10.1038/s41598-017-11317-7
41. Nuzzi R, Dallorto L, Vitale A. Cerebral modifications and visual pathway reorganization in maculopathy: a systematic review. Frontiers Neurosci. (2020) 14:755. doi: 10.3389/fnins.2020.00755
42. Krug K. Principles of function in the visual system. in Sensory Perception: Mind and Matter Barth FG, Giampieri–Deutsch P, Klein HD. Eds. Springer Vienna: Vienna. (2012) 3:41–56. doi: 10.1007/978-3-211-99751-2_3
43. Wen W, Wang Y, Zhou J, He S, Sun X, Liu H, et al. Loss and enhancement of layer–selective signals in geniculostriate and corticotectal pathways of adult human amblyopia. Cell reports. (2021) 37:110117. doi: 10.1016/j.celrep.2021.110117
44. Kupers R, Ptito M. Structural metabolic and functional changes in the congenitally blind brain. Int J. (2014) 94:152. doi: 10.1016/j.ijpsycho.2014.08.681
45. Ptito M, Paré S, Dricot L, Cavaliere C, Tomaiuolo F, Kupers RA. Quantitative analysis of the retinofugal projections in congenital and late–onset blindness. NeuroImage Clin. (2021) 32:102809. doi: 10.1016/j.nicl.2021.102809
46. Reislev NL, Kupers R, Siebner HR, Ptito M. Blindness alters the microstructure of the ventral but not the dorsal visual stream. Brain Struct Funct. (2016) 221:2891–903. doi: 10.1007/s00429-015-1078-8
47. Hoffmann MB, Dumoulin SO. Congenital visual pathway abnormalities: a window onto cortical stability and plasticity. Trends Neurosci. (2015) 38:55–65. doi: 10.1016/j.tins.2014.09.005
48. Bedny M, Pascual-Leone A, Dodell-Feder D, Fedorenko E, Saxe R. Language processing in the occipital cortex of congenitally blind adults. Proc Nati Aca Sci USA. (2011) 108:4429–34. doi: 10.1073/pnas.1014818108
49. Kusne Y, Wolf AB, Townley K, Conway M, Peyman GA. Visual system manifestations of Alzheimer's disease. Acta Ophthalmol. (2017) 95:e668–76. doi: 10.1111/aos.13319
50. Loughrey DG, Kelly ME, Kelley GA, Brennan S, Lawlor BA. Association of age–related hearing loss with cognitive function cognitive impairment and dementia: a systematic review and meta–analysis. JAMA Otolaryngol—-Head Neck Surg. (2018) 144:115–26. doi: 10.1001/jamaoto.2017.2513
51. Silva MME, Mercer PBS, Witt MCZ, Pessoa RR. Olfactory dysfunction in Alzheimer's disease Systematic review and meta–analysis. Dementia Neuropsychol. (2018) 12:123–32. doi: 10.1590/1980-57642018dn12-020004
53. Lindenberger U, Baltes PB. Sensory functioning and intelligence in old age: a strong connection. Psychol Aging. (1994) 9:339–55. doi: 10.1037/0882-7974.9.3.339
54. Humes LE, Busey TA, Craig J, Kewley–Port D. Are age–related changes in cognitive function driven by age–related changes in sensory processing? Attent Percept Psychophysics. (2013) 75:508–24. doi: 10.3758/s13414-012-0406-9
55. Monge ZA, Madden D. Linking cognitive and visual perceptual decline in healthy aging: The information degradation hypothesis. Neurosci Biobehav Rev. (2016) 69:166–73. doi: 10.1016/j.neubiorev.2016.07.031
56. Ohno–Matsui K, Jonas JB. Posterior staphyloma in pathologic myopia. Prog Retinal Eye Res. (2019) 70:99–109. doi: 10.1016/j.preteyeres.2018.12.001
57. Zhang XW, Dai RP, Cheng GW, Zhang WH, Long Q. Altered amplitude of low–frequency fluctuations and default mode network connectivity in high myopia: a resting–state fMRI study. Int J ophthalmol. (2020) 13:1629–36. doi: 10.18240/ijo.2020.10.18
58. Ong SY, Ikram MK, Haaland BA, Cheng CY, Saw SM, Wong TY, et al. Myopia and cognitive dysfunction: the singapore malay eye study. Invest Ophthalmol Visual Sci. (2013) 54:799–803. doi: 10.1167/iovs.12-10460
59. Sun HP, Liu H, Xu Y, Pan CW. Myopia and cognitive dysfunction among elderly Chinese adults: a propensity score matching analysis. Ophthalmic Physiol Optics J Br Coll Ophthalmic Opticians. (2016) 36:191–6. doi: 10.1111/opo.12248
60. Mirshahi A, Ponto KA, Laubert-Reh D, Rahm B, Lackner KJ, Binder H, et al. Myopia and cognitive performance: results from the gutenberg health study. Invest Ophthalmol Visual Sci. (2016) 57:5230–6. doi: 10.1167/iovs.16-19507
61. Liou SW, Chiu C. J. Myopia and contrast sensitivity function. Curr Eye Res. (2001) 22:81–4. doi: 10.1076/ceyr.22.2.81.5530
62. Atchison DA, Schmid KL, Pritchard N. Neural and optical limits to visual performance in myopia. Vision research. (2006) 46:3707–22. doi: 10.1016/j.visres.2006.05.005
63. Mascetti GG, Turatto M, Facoetti A. Four paradigms to study visual—-spatial attention of myopic subjects. Brain Res Brain Res Protoc. (2001) 7:241–7. doi: 10.1016/S1385-299X(01)00070-8
64. Turatto M Facoetti A Serra G Benso F Angi M Umilta C Mascetti G. G. Visuospatial attention in myopia. Brain Res Cogn Brain Res. (1999) 8:369–72. doi: 10.1016/S0926-6410(99)00025-7
65. Kuo HY, Atchison DA, Schmid KL. Dot motion perception in young adult emmetropes and myopes. Optometry Vision Sci J Am Aca Optometry. (2018) 95:498–504. doi: 10.1097/OPX.0000000000001223
66. von Schantz M, Provencio I, Foster RG. Recent developments in circadian photoreception: more than meets the eye. Invest Ophthalmol Visual Sci. (2000) 41:1605–7.
67. Güler AD, Ecker JL, Lall GS, Haq S, Altimus CM, Liao HW, et al. Melanopsin cells are the principal conduits for rod–cone input to non–image–forming vision. Nature. (2008) 453:102–5. doi: 10.1038/nature06829
68. Fernandez DC, Fogerson PM, Lazzerini Ospri L, Thomsen MB, Layne RM, Severin D, et al. Light affects mood and learning through distinct retina–brain pathways. Cell. (2018) 175:71–84. doi: 10.1016/j.cell.2018.08.004
69. Altimus CM, Güler AD, Villa KL, McNeill DS, Legates TA, Hattar S. Rods–cones and melanopsin detect light and dark to modulate sleep independent of image formation. Proc Nat Aca Sci USA. (2008) 105:19998–20003. doi: 10.1073/pnas.0808312105
70. Wu YJ, Wu N, Huang X, Rao J, Yan L, Shi L, et al. Evidence of cortical thickness reduction and disconnection in high myopia. Scient Reports. (2020) 10:16239. doi: 10.1038/s41598-020-73415-3
71. Wang H, Wen H, Li J, Chen Q, Li S, Wang Y, et al. Characterization of brain microstructural abnormalities in high myopia patients: a preliminary diffusion kurtosis imaging study. Korean J Radiol. (2021) 22:1142–51. doi: 10.3348/kjr.2020.0178
72. Zhai L, Li Q, Wang T, Dong H, Peng Y, Guo M, et al. Altered functional connectivity density in high myopia. Behav Brain Res. (2016) 303:85–92. doi: 10.1016/j.bbr.2016.01.046
73. Huang X, Zhou FQ, Hu YX, Xu XX, Zhou X, Zhong YL, et al. Altered spontaneous brain activity pattern in patients with high myopia using amplitude of low–frequency fluctuation: a resting–state fMRI study. Neuropsychiatric Dis Treat. (2016) 12:2949–56. doi: 10.2147/NDT.S118326
74. Anticevic A, Cole MW, Murray JD, Corlett PR, Wang XJ, Krystal JH. The role of default network deactivation in cognition and disease. Trends Cog Sci. (2012) 16:584–92. doi: 10.1016/j.tics.2012.10.008
75. Putcha D, Ross RS, Cronin-Golomb A, Janes AC, Stern CE. Salience and default mode network coupling predicts cognition in aging and Parkinson's disease. J Int Neuropsychol Soc JINS. (2016) 22:205–15. doi: 10.1017/S1355617715000892
76. Deen B, Koldewyn K, Kanwisher N, Saxe R. Functional organization of social perception and cognition in the superior temporal sulcus. Cereb Cortex. (2015) 25:4596–609. doi: 10.1093/cercor/bhv111
77. Fox CJ, Iaria G, Barton JJ. Defining the face processing network: optimization of the functional localizer in fMRI. Human Brain Mapp. (2009) 30:1637–51. doi: 10.1002/hbm.20630
78. Pitcher D, Dilks DD, Saxe RR. Triantafyllou C Kanwisher N. Differential selectivity for dynamic versus static information in face–selective cortical regions. NeuroImage. (2011) 56:2356–63. doi: 10.1016/j.neuroimage.2011.03.067
79. Grossman ED, Blake R. Brain areas active during visual perception of biological motion. Neuron. (2002) 35 1167–75. doi: 10.1016/S0896-6273(02)00897-8
80. Beauchamp MS, Lee KE, Haxby JV, Martin A. Parallel visual motion processing streams for manipulable objects and human movements. Neuron. (2002) 34:149–59. doi: 10.1016/S0896-6273(02)00642-6
81. Fisher C, Freiwald WA. Contrasting specializations for facial motion within the macaque face–processing system. Curr Biol CB. (2015) 25:261–66. doi: 10.1016/j.cub.2014.11.038
82. Russ BE, Leopold DA. Functional MRI mapping of dynamic visual features during natural viewing in the macaque. NeuroImage. (2015) 109:84–94. doi: 10.1016/j.neuroimage.2015.01.012
83. Phillips ML, Young AW, Senior C, Brammer M, Andrew C, Calder AJ, et al. A specific neural substrate for perceiving facial expressions of disgust. Nature. (1997) 389:495–8. doi: 10.1038/39051
84. Calder AJ, Beaver JD, Winston JS, Dolan RJ, Jenkins R, Eger E, et al. Separate coding of different gaze directions in the superior temporal sulcus and inferior parietal lobule. Curr Bio CB. (2007) 17:20–5. doi: 10.1016/j.cub.2006.10.052
85. Pitcher D. Facial expression recognition takes longer in the posterior superior temporal sulcus than in the occipital face area. J Neurosci J Soc Neurosci. (2014) 34:9173–7. doi: 10.1523/JNEUROSCI.5038-13.2014
86. Sliwinska MW, Elson R, Pitcher D. Dual–site TMS demonstrates causal functional connectivity between the left and right posterior temporal sulci during facial expression recognition. Brain Stimulat. (2020) 13:1008–13. doi: 10.1016/j.brs.2020.04.011
87. Hoffman EA, Haxby JV. Distinct representations of eye gaze and identity in the distributed human neural system for face perception. Nature Neurosci. (2000) 3:80–4. doi: 10.1038/71152
88. Allison T, Puce A, McCarthy G. Social perception from visual cues: role of the STS region. Trends Cog Sci. (2000) 4:267–78. doi: 10.1016/S1364-6613(00)01501-1
89. Hur JW, Blake R, Cho KI, Kim J, Kim SY, Choi SH, et al. Biological motion perception brain responses and schizotypal personality disorder. JAMA Psychiatry. (2016) 73:260–7. doi: 10.1001/jamapsychiatry.2015.2985
90. Grossman E, Donnelly M, Price R, Pickens D, Morgan V, Neighbor G, et al. Brain areas involved in perception of biological motion. J Cogn Neurosci. (2000) 12:711–20. doi: 10.1162/089892900562417
91. Belin P, Fecteau S, Bédard C. Thinking the voice: neural correlates of voice perception. Trends Cog Sci. (2004) 8:129–35. doi: 10.1016/j.tics.2004.01.008
92. Binder JR, Frost JA, Hammeke TA, Cox RW, Rao SM, Prieto T. Human brain language areas identified by functional magnetic resonance imaging. J Neurosci So Neurosc. (1997) 17:353–62. doi: 10.1523/JNEUROSCI.17-01-00353.1997
93. Young AW, Frühholz S, Schweinberger SR. Face and voice perception: understanding commonalities and differences. Trends in cognitive sciences. (2020) 24:398–410. doi: 10.1016/j.tics.2020.02.001
94. Saxe R, Kanwisher N. People thinking about thinking people. The role of the temporo–parietal junction in “theory of mind”. NeuroImage. (2003) 19:1835–42. doi: 10.1016/S1053-8119(03)00230-1
95. Li Q, Guo M, Dong H, Zhang Y, Fu Y, Yin X. Voxel–based analysis of regional gray and white matter concentration in high myopia. VisionRres. (2012) 58:45–50. doi: 10.1016/j.visres.2012.02.005
96. Wang H, Li S, Chen X, Wang Y, Li J, Wang Z. Cerebral blood flow alterations in high myopia: an arterial spin labeling study. Neural Plasticity. (2020) 2020:6090262. doi: 10.1155/2020/6090262
97. Cheng Y, Huang X, Hu YX, Huang MH, Yang B, Zhou FQ, Wu X. R. Comparison of intrinsic brain activity in individuals with low/moderate myopia versus high myopia revealed by the amplitude of low–frequency fluctuations. Acta Radiol. (2020) 61:496–507. doi: 10.1177/0284185119867633
98. Malecaze FJ, Boulanouar KA, Demonet JF, Guell JL. Abnormal activation in the visual cortex after corneal refractive surgery for myopia: demonstration by functional magnetic resonance imaging. Ophthalmology. (2001) 108:2213–8. doi: 10.1016/S0161-6420(01)00843-0
99. Vuori E, Vanni S, Henriksson L, Tervo TM, M Holopainen JM. Refractive surgery in anisometropic adult patients induce plastic changes in primary visual cortex. Acta Ophthalmol. (2012) 90:669–76. doi: 10.1111/j.1755-3768.2011.02152.x
100. Vuori E, Tervo TM, Holopainen MV. Improvement of visual acuity following refractive surgery for myopia and myopic anisometropia. J Refract Surg. (2007) 23:447–55. doi: 10.3928/1081-597X-20070501-05
101. Logothetis NK, Pauls J, Augath M, Trinath T, Oeltermann AN. Neurophysiological investigation of the basis of the fMRI signal. Nature. (2001) 412:150–7. doi: 10.1038/35084005
102. Glarin RK, Nguyen BN, Cleary JO, Kolbe SC, Ordidge RJ, Bui BV, et al. MR–EYE: High–resolution MRI of the human eye and orbit at ultrahigh field (7T). Mag Res Imag Clin North Am. (2021) 29:103–16. doi: 10.1016/j.mric.2020.09.004
103. Hoffmann MB, Stadler J, Kanowski M. Retinotopic mapping of the human visual cortex at a magnetic field strength of 7T. J Int Fed Clin Neurophysiol. (2009) 120:108–16. doi: 10.1016/j.clinph.2008.10.153
104. GGarcía-Domene MC, Luque MJ, Díez-Ajenjo MA, Desco-Esteban MC, Artigas JM. Chromatic and achromatic visual fields in relation to choroidal thickness in patients with high myopia: a pilot study. J francais d'ophtalmologie. (2018) 41:109–15. doi: 10.1016/j.jfo.2017.07.006
105. Morand S, Thut G, de Peralta RG, Clarke S, Khateb A, Landis T. Electrophysiological evidence for fast visual processing through the human koniocellular pathway when stimuli move. Cereb Cortex. (2000) 10:817–25. doi: 10.1093/cercor/10.8.817
106. Pitcher D, Ungerleider LG. Evidence for a third visual pathway specialized for social perception. Trends Cog Sci. (2021) 25:100–10. doi: 10.1016/j.tics.2020.11.006
107. Perrett DI, Hietanen JK, Oram MW. Organization and functions of cells responsive to faces in the temporal cortex. Philosophical transactions of the royal society of London. Series B Biol Sci. (1992) 335:23–30. doi: 10.1098/rstb.1992.0003
108. Hein G, Knight RT. Superior temporal sulcus—-It's my area: or is it? J Cog Neurosc. (2008) 20:2125–36. doi: 10.1162/jocn.2008.20148
109. Livingston G, Huntley J, Sommerlad A, Ames D, Ballard C, Banerjee S, et al. Dementia prevention intervention and care:. (2020 report of the lancet commission. Lancet. (2020) 396:413–46. doi: 10.1016/S0140-6736(20)30367-6
110. Varadaraj V, Munoz B, Simonsick EM. Vision impairment and participation in cognitively stimulating activities: the health ABC study. J Gerontol Series A Biol Sci Med Sci. (2021) 76:835–841. doi: 10.1093/gerona/glaa184
111. Rong SS, Lee BY, Kuk AK, Yu XT, Li SS, Li J, et al. Comorbidity of dementia and age–related macular degeneration calls for clinical awareness: a meta–analysis. Br J Ophthalmol. (2019) 103:1777–83. doi: 10.1136/bjophthalmol-2018-313277
112. Zhang HJ, Mi XS. Normal tension glaucoma: from the brain to the eye or the inverse? Neural Regen Res. (2019) 14:1845–50. doi: 10.4103/1673-5374.259600
113. Quiroz YT, Zetterberg H, Reiman EM, Chen Y, Su Y, Fox-Fuller JT, et al. Plasma neurofilament light chain in the presenilin 1 E280A autosomal dominant Alzheimer's disease kindred: a cross–sectional and longitudinal cohort study. Lancet Neurol. (2020) 19:513–21. doi: 10.1016/S1474-4422(20)30137-X
114. Gordon BA, Blazey TM, Su Y, Hari-Raj A, Dincer A, Flores S, et al. A soluble phosphorylated tau signature links tau amyloid and the evolution of stages of dominantly inherited Alzheimer's disease. Nat Med. (2020) 26:398–407. doi: 10.1038/s41591-020-0781-z
115. Scheltens P, De Strooper B, Kivipelto M, Holstege H, Chételat G, Teunissen CE, et al. Spatial patterns of neuroimaging biomarker change in individuals from families with autosomal dominant Alzheimer's disease: a longitudinal study. Lancet Neurol. (2018) 17:241–50. doi: 10.1016/S1474-4422(18)30028-0
116. Scheltens P, De Strooper B, Kivipelto M, Holstege H, Chételat G, Teunissen CE, et al. Alzheimer's disease. Lancet. (2021) 397:1577–90. doi: 10.1016/S0140-6736(20)32205-4
117. Baroncelli L, Lunghi C. Neuroplasticity of the visual cortex: in sickness and in health. Exp Neurol. (2021) 335:113515. doi: 10.1016/j.expneurol.2020.113515
Keywords: Parkinson's disease, cognitive impairment, dementia, high myopia, MRI, neuroimage, the visual system, neuroplasticity
Citation: Li K, Wang Q, Wang L and Huang Y (2022) Cognitive dysfunctions in high myopia: An overview of potential neural morpho-functional mechanisms. Front. Neurol. 13:1022944. doi: 10.3389/fneur.2022.1022944
Received: 22 August 2022; Accepted: 10 October 2022;
Published: 03 November 2022.
Edited by:
Enrico Borrelli, Vita-Salute San Raffaele University, ItalyReviewed by:
Andrea Saladino, San Raffaele Hospital (IRCCS), ItalyZhentao Zuo, Institute of Biophysics (CAS), China
Copyright © 2022 Li, Wang, Wang and Huang. This is an open-access article distributed under the terms of the Creative Commons Attribution License (CC BY). The use, distribution or reproduction in other forums is permitted, provided the original author(s) and the copyright owner(s) are credited and that the original publication in this journal is cited, in accordance with accepted academic practice. No use, distribution or reproduction is permitted which does not comply with these terms.
*Correspondence: Yifei Huang, MzAxeWsmI3gwMDA0MDtzaW5hLmNvbQ==