- Department of Pharmacology, Instituto de Ciências Biomédica, Universidade de Sao Paulo, Sao Paulo, Brazil
Parkinson’s disease (PD) is the second neurodegenerative disorder most prevalent in the world, characterized by the loss of dopaminergic neurons in the Substantia Nigra (SN). It is well known for its motor and non-motor symptoms including bradykinesia, resting tremor, psychiatric, cardiorespiratory, and other dysfunctions. Pathological apoptosis contributes to a wide variety of diseases including PD. Various insults and/or cellular phenotypes have been shown to trigger distinct signaling events leading to cell death in neurons affected by PD. The intrinsic or mitochondrial pathway, inflammatory or oxidative stress-induced extrinsic pathways are the main events associated with apoptosis in PD-related neuronal loss. Although SN is the main brain area studied so far, other brain nuclei are also affected by the disease leading to non-classical motor symptoms as well as non-motor symptoms. Among these, the respiratory symptoms are often overlooked, yet they can cause discomfort and may contribute to patients shortened lifespan after disease diagnosis. While animal and in vitro models are frequently used to investigate the mechanisms involved in the pathogenesis of PD in both the SN and other brain regions, these models provide only a limited understanding of the disease’s actual progression. This review offers a comprehensive overview of some of the most studied forms of cell death, including recent research on potential treatment targets for these pathways. It highlights key findings and milestones in the field, shedding light on the potential role of understanding cell death in the prevention and treatment of the PD. Therefore, unraveling the connection between these pathways and the notable pathological mechanisms observed during PD progression could enhance our comprehension of the disease’s origin and provide valuable insights into potential molecular targets for the developing therapeutic interventions.
1 Introduction
Parkinson’s Disease (PD) is a most recognized syndrome, clinically identifiable by a progression of motor and non-motor symptoms, such as bradykinesia, rigidity, akinesia, dystonia, dysphagia, cognitive impairments, and impairments in gut function and olfaction, among others (Poewe et al., 2017; Simon et al., 2020). Symptoms most commonly begin in elderly people (above 60 years), with a prevalence ranging from 1 to 2 per 1,000, rising to more than 4% in those over 85 years of age. This establishes PD as the second most common neurodegenerative disease worldwide (Tysnes and Storstein, 2017; de Rijk et al., 1995; Simon et al., 2020; Aarsland et al., 2021). Although PD is most prevalent in older populations, it is not absent in individuals younger than 61 years, the average onset age of the disease (Pagano et al., 2016). Recent studies indicate that while the incidence of PD is lower in younger individuals, there is a trend towards the development of the disease even in populations traditionally considered to be of lowered risk (Willis et al., 2022). The etiology of PD remains a topic of debate, as it has been shown that both genetic factors, such as mutations in SNCA (Polymeropoulos et al., 1997) and Parkin (Kitada et al., 1998), and environmental exposure, such as the pesticides paraquat and maneb (Cicchetti et al., 2005), may cause the disease (Kouli et al., 2018). The most prominent mutations associated with the increased risk of developing PD are in the GBA and LRRK2 genes. Certain populations, such as the Ashkenazi Jews and North African Imazighen, have shown an increased number of PD cases associated with mutation in these genes (Clark et al., 2007; Hulihan et al., 2008; Healy et al., 2008; Benamer and de Silva, 2010; Ross et al., 2011; Dagan et al., 2015). Other studies have demonstrated significant correlation between these genetic variants and more genetically diverse populations, such as in Brazil (dos Santos et al., 2010; Guimarães Bde et al., 2012) and well as PARK1 mutation in Filipinos (Rogaeva et al., 2004).
Although much remains to be discovered, the primary theoretical pathway through which Parkinson’s disease spreads was hypothesized by Braak and colleagues (Braak et al., 2003). This theory suggests that a pathogen may trigger the progression of the disease by initiating the production of α-synuclein aggregates. This process is thought to occur in two neuron-populated sites: the olfactory bulb and the gut, which may explain the early development of olfactory and gut dysfunctions that precede the motor symptoms defining the diagnosis (Rietdijk et al., 2017; Kim et al., 2019; Konings et al., 2023; Espinosa-Oliva et al., 2024). This hypothesis has led to the establishment of stagings of sporadic Parkinson’s disease based on the presence of α-synuclein aggregates throughout the nervous system (Braak et al., 2003). Stage 1 is characterized by lesions in the dorsal IX/X motor nucleus and/or the intermediate reticular zone; Stage 2 involves additional lesions in caudal raphe nuclei, gigantocellular reticular nucleus, and coeruleus–subcoeruleus complex; Stage 3 includes midbrain lesions, particularly in the pars compacta of the substantia nigra (SN); Stage 4 adds prosencephalic lesions, with cortical involvement limited to the temporal mesocortex (transentorhinal region) and allocortex (CA2-plexus), while the neocortex remains unaffected; Stage 5 sees the involvement of high-order sensory association areas of the neocortex and prefrontal neocortex; and Stage 6 involves lesions in first-order sensory association areas of the neocortex and premotor areas, with occasional mild changes in primary sensory areas and the primary motor field (Braak et al., 2003).
Regardless of the primary cause of the disease, there is broad consensus that the cellular mechanisms involved in cell death, along with inflammation and oxidative stress, play key roles in its development (Tansey et al., 2022; Subramaniam and Chesselet, 2013; Dionísio et al., 2021). In this review, we discuss the role of cell death mechanisms and explore current frontiers in research, both in humans and animal models, including potential treatment opportunities.
2 Cell death in neurodegenerative diseases
Although apoptosis is often regarded as a deleterious process, it is essential for developmental tissues to activate apoptosis under certain conditions to remodel tissue or form specific developmental structures (Phelan et al., 1997; Dong et al., 2015; Yamaguchi and Miura, 2015; Voss and Strasser, 2020). When genes related to the initiation of apoptosis are deleted, developing tissue cannot form properly, leading to neurodevelopmental issues such as spina bifida, improper neural tube closure, deficient removal of interdigital webs, and other tissue malformations (Kuida et al., 1996; Cecconi et al., 1998; Yoshida et al., 1998; Ke et al., 2018; Fogarty et al., 2019). In adults, however, apoptosis also contributes to the development of the neurodegenerative diseases through axonal degeneration and neuronal cell death (Pemberton et al., 2021). Numerous studies have shown that the apoptotic pathway is involved in the pathogenesis of these diseases, and it is activated only as a last resort when there is no possibility of neurons recovery (Chi et al., 2018; Dailah, 2022).
Neurodegenerative diseases are characterized by the slow, progressive loss of neuronal cells in the central nervous system (CNS) and the aggregation of misfolded proteins (Cenini et al., 2020). The most common neurodegenerative diseases, such as Alzheimer’s (AD), Huntington’s (HD) and PD, are all marked by the accumulation of misfolded proteins, which play a crucial role in the dysfunction or loss of neurons through their deposition within cells or the extracellular matrix (Singh et al., 2019). While the composition and location of these aggregates can vary between different neurodegenerative diseases, a higher concentration of these proteinaceous materials is generally associated with more severe disease progression (Singh et al., 2019). Although protein aggregation is a common feature, neurodegenerative diseases exhibit different patterns of neurons loss and affect distinct regions of the CNS (Dugger and Dickson, 2017).
Research efforts have led to the creation of animal and cellular models that are useful for unraveling many of the causes of neurodegenerative diseases. However, these models have significant limitations. Some models can display certain molecular or behavioral hallmarks of PD while failing to replicate others. For example, no rodent models that can replicate all the common behavioral symptoms of PD, which limits the choice of animal models depending on the specific behavioral trait being studied (Deumens et al., 2002). Additionally, gene regulation and expression may not bind to the same genes or even chromosomes, leading to different cellular responses to the model’ stimuli (Wilson et al., 2008). The main challenge, however, lies in modelling a disease as heterogeneous as PD, which can present cellular and molecular hallmarks differently between individuals, despite appearing similar among patients (Bloem et al., 2021). The underlying mechanisms of Parkinson’s disease seem to arise from a complex interplay of abnormal α-synuclein aggregation, mitochondrial and lysosomal dysfunction, disruptions in vesicle and synaptic transport, and neuroinflammatory processes (Bloem et al., 2021). To advance our understanding of PD, it is important to clearly define which aspects of the disease we aim to explore and how our research question aligns with the chosen model.
3 Mechanisms by which cells can die under various physiological and pathological conditions
3.1 Apoptosis
Apoptosis, notoriously known as a type of programmed cell death, is an energy-dependent cellular process that promotes cell death by activating endonucleases and proteases, which ultimately destroy cell molecules. This process leads to biochemical modifications within the cell, rendering it nonfunctional, which can deteriorate tissues and contribute to the development of various diseases (Elmore, 2007). More recent studies describe apoptosis as a regulator of cell fate, determining which cells should be eliminated due to DNA mutations or other proteins malfunctions and which cells should be preserved to maintain homeostasis. This process is largely dependent on the BCL-2 family of proteins (Singh et al., 2019). An imbalance between the pro-death and pro-survival proteins (also known as pro-apoptotic and anti-apoptotic proteins, respectively) can trigger downstream proteins in this pathway, leading to DNA fragmentation and cell death. Additionally, external signals, such as phosphatidylserine, when recognized by nearby phagocytic cells, stimulate the phagocytosis of the apoptotic debris, resulting in a non-inflammatory cell death (Nagata et al., 2016).
Apoptosis operates through two distinct pathways: the intrinsic pathway, which is dependent on internal signaling, primarily regulated by the BCL-2 family of proteins and involves mitochondria interactions, and the extrinsic pathway, which is dependent of external signaling and independent of BCL-2 and mitochondrial involvement (Jan and Chaudhry, 2019). Although these pathways involve different proteins, they converge on the same downstream effectors, known as the execution pathway (Belizário et al., 2015). In Parkinson’s Disease (PD), a sizable portion of neuronal cell death is attributed to apoptosis, as brains from human with PD, as well as those from animal models, exhibit abnormal protein profiles in regions such as the SN, hippocampus, hypothalamus, olfactory bulb and other areas (Erekat, 2018). Indeed, classical literature shows that apoptosis is a common mechanism by which cells respond to well-described apoptotic stimuli, including the drugs traditionally used to induce PD models in both animals (Mendez and Finn, 1975; Heikkila et al., 1984; Perese et al., 1989; Ferrante et al., 1997; Thiffault et al., 2000) and cells (Hartley et al., 1994; Mochizuki et al., 1994; Walkinshaw and Waters, 1994). During cellular respiration and ATP production in mitochondria, reactive oxygen species (ROS) are naturally produced in the electron transport chain (Subramaniam and Chesselet, 2013). However, when ROS are produced uncontrollably, they became toxic to the cell, generating oxidative stress that leads to cell death through apoptosis (Gorman et al., 1996). This type of mitochondrial dysfunction is one of the contributing factors to neurodegeneration in PD (Yan et al., 2013).
Despite evidence showing that apoptosis is a crucial mediator of neuronal death in PD, other cell death mechanisms should not be overlooked. Indeed, animal models exhibiting increased apoptotic signaling may also activate other signaling pathways that contribute to overall degeneration in the brain, such as autophagy (Garcia-Garcia et al., 2013; Zhu H. et al., 2023; Elesawy et al., 2024; Sophoronea et al., 2024), pyroptosis (Zhang M. et al., 2020; Zhu et al., 2022; Huang et al., 2024) and necroptosis (Roy et al., 2023; Kim et al., 2023; Leem et al., 2024). A wealth of research has explored these pathways in the context of Parkinson’s disease, as discussed below.
3.1.1 Intrinsic pathway
The intrinsic pathway is a well-characterized pathway, in which cell fate is regulated through the expression of proteins containing the BH3 domain, such as Bcl2, Bcl-XL, Bax, Bak, Bid, PUMA and NOXA (Alberts, 2022). These proteins are located on the mitochondrial membrane or in the cytosol of the mitochondrial. They play a critical role in maintaining cell survival. Upon activation of the intrinsic pathway, pro-apoptotic proteins are activated, allowing the passage of cytochrome C from the mitochondria, and initiating the apoptotic cascade (Morris et al., 2021; Figure 1). In PD animal models, Bcl-2 family proteins exhibit abnormal expression following neuronal lesions, which triggers apoptotic cascades and contributes to further degeneration of brain regions (Table 1). Reestablishment the balance between these proteins is crucial for returning the tissue to homeostasis (Rekha and Selvakumar, 2014; Liu et al., 2018). Additional studies have highlighted the involvement of both Bax and caspase 3 in PD neurodegeneration across various models, including SH-SY5Y cells (Itano and Nomura, 1995), PC12 cells (Blum et al., 1997), human post-mortem tissue (Tatton, 2000) and mice (Yamada et al., 2010).
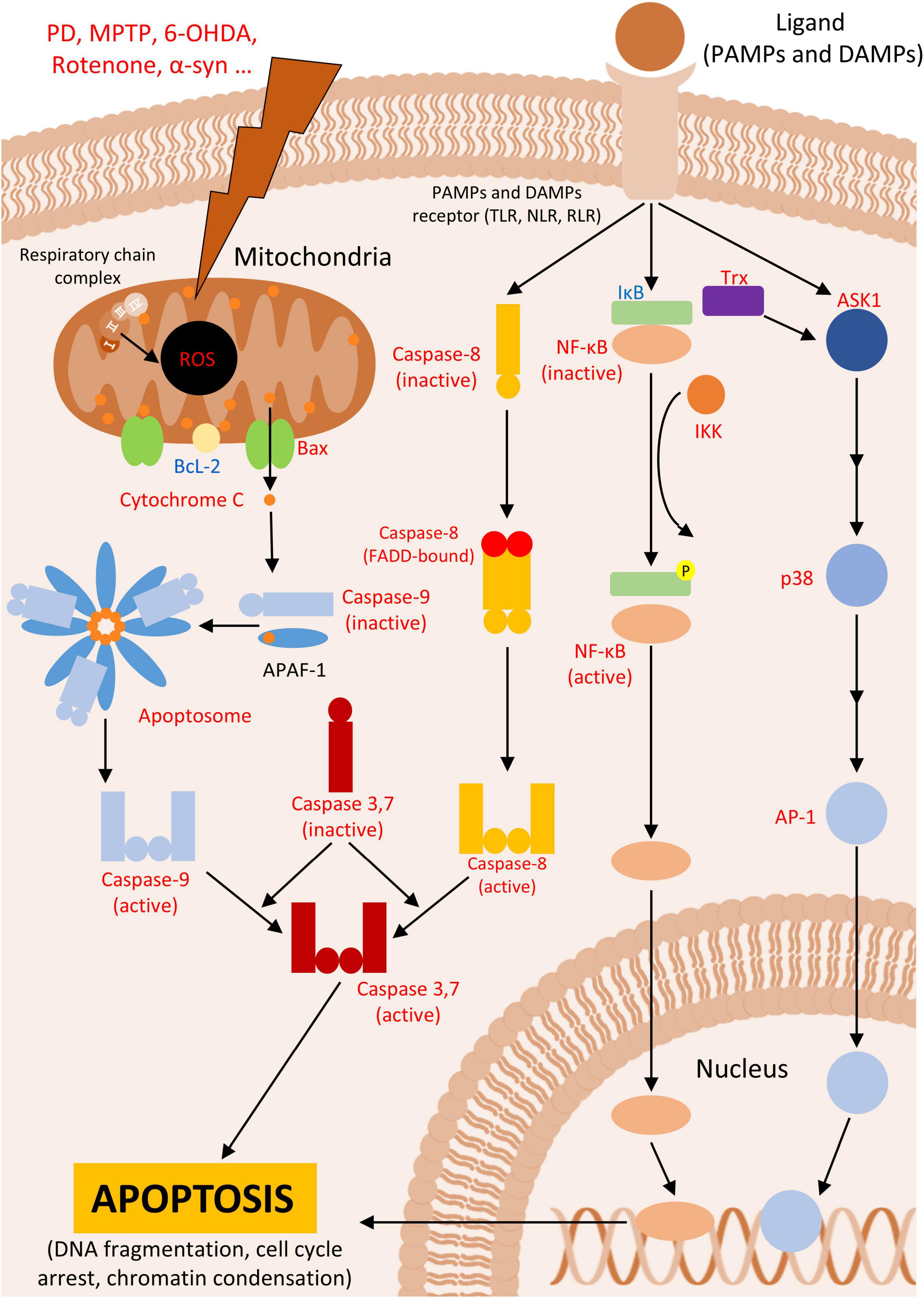
Figure 1. Apoptosis. Figure shows intrinsic and extrinsic pathways leading to apoptosis in Parkinson’s disease (PD) or PD animal models. In red are described upregulated- and in blue downregulated proteins in PD.
Following the release of cytochrome C, it interacts with Apaf1, exposing its CARD domain, which allows this protein to bind to other Apaf1 proteins, forming an oligomer known as the apoptosome (Dorstyn et al., 2018). The apoptosome the assembles initiator caspases, such as caspase 8 and 9 (Bao and Shi, 2007; McIlwain et al., 2013; Anson et al., 2021). These initiator caspases, once activated, lead to the activation of effector caspases (typically, caspases 3, 6, and 7) through the cleavage of the latter. These effector caspases are responsible for cleaving the cell’s DNA, thereby completing the apoptosis signaling cascade (Brentnall et al., 2013; Parrish et al., 2013; Figure 1). Research by Fall and Bennett indicates that apoptosis in SH-SY5Y cells induced with MPTP begins 9 to 12 hours after induction, during which ROS production continues and mitochondria membrane potential is lost, leading to apoptosis (Fall and Bennett, 1999).
Several factors can lead to the uncontrolled production of ROS, including damage to the mitochondrial complex I and III, which are the major sources of ROS, reduced ATP production, or malfunctioning of enzymes such as superoxide dismutase (SOD) that convert ROS into non-toxic molecules (Elfawy and Das, 2019). Postmortem analyses of brains from PD patients reveal colocalization between cytochrome c and other apoptosome-related proteins with Lewy bodies, highlighting the role of these proteins in the formation of such structures (Kawamoto et al., 2014). Dopaminergic neurons in the SN are particularly sensitive to oxidative stress (Sziráki et al., 1998) due to their elevated levels of pro-oxidant iron, which facilitates ROS production by reducing oxygen, and their low levels of glutathione, a crucial antioxidant in cellular metabolism (Sian-Hülsmann et al., 2011). Previous study has demonstrated a correlation between ROS production, as indicated by SOD activity, and PD models (Choi et al., 1999).
3.1.2 Extrinsic pathway
As its name suggests, the extrinsic pathway is triggered primarily by extracellular signals mediated by immune system cells, such as lymphocytes or macrophages. These cells produce soluble molecules, including members of the tumor necrosis factor superfamily (TNFSF), which diffuse through the tissue and bind to their receptors on target cells, leading to the progression of apoptosis (Yanumula and Cusick, 2023). Another extracellular mechanism for triggering cell death involves the interaction of receptors and ligands, such as the Fas/FasL system, which can also initiate apoptotic events under certain conditions (Yamada et al., 2017). Receptors involved in the extrinsic pathway form complexes with caspase 8, triggering apoptosis though the terminal pathway, which is also associated with the intrinsic apoptotic pathway (Medema et al., 1997; Bodmer et al., 2000; Figure 1). The activation of caspase 8 as an apoptotic inducer, has been well-described in PD in vitro models (Viswanath et al., 2001; Choi et al., 2004), along with the involvement of proteins from other proteins from the extrinsic pathway (Table 2).
The interaction between ligand and receptor proteins triggers intracellular responses involving proteins such as NF-κβ (Rickert et al., 2011), and JNK (Chang et al., 2006), or in some cases, no protein activation due to decoy receptors (MacFarlane et al., 1997). The role of NF-κβ in PD cellular models is well-documented, particularly concerning the neuroprotective effects of blocking this pathway (Cassarino et al., 2000; Huang et al., 2018; Meng et al., 2023; Figure 1).
To better understand the role of these proteins in apoptosis, it is crucial to comprehend how these pathways induce cell death. NF-κβ is a dimer, composed of proteins from the NF-κβ family, which is normally bound to IκBα, which sequesters the dimer in the cytoplasm (Jacobs and Harrison, 1998). NF-κβ proteins are produced through the proteolytic processing of two other precursor proteins, p100 and p105. This processing, which involves the cleavage of the C-terminal half of the protein, results in the formation of either NF-κβ2 or NF-κβ1, respectively (Lin and Ghosh, 1996; Yamada et al., 2000). Upon activation of TNFRSF and other inflammatory receptors, a protein complex known as IKK phosphorylates IκBα, targeting it for degradation by the proteasome. This action releases NF-κB, allowing it to translocate to the nucleus and function as a transcription factor, in what is known as the canonical pathway (Liu et al., 2017; Figure 1).
Additionally, these receptors can activate mitogen-activated protein kinases (MAPK), a superfamily of proteins known for their role in phosphorylating other proteins on serine and threonine residues. This phosphorylation leads to signaling cascades that can activate gene transcription through the complex formation between receptor and the mitogen (Gómez and Cohen, 1991). Some members of the MAPK family, such as JNK and p38, despite their key role in cell survival via the activation of growth factors, are also involved in apoptosis. These proteins can also be activated by receptors responsible to stress stimuli and inflammatory cytokines (Cano et al., 1994; Cano and Mahadevan, 1995), and their activation is sufficient to trigger apoptosis in PD models (Onyango et al., 2005; Ouyang and Shen, 2006). It has been demonstrated that deprivation of certain nutrients to cells in vitro (e.g., tropic stimuli, glucose, ions) can activate alternative pathways that lead to a detrimental activation of JNKs, promoting apoptosis (Xu et al., 2001; Wilms et al., 2003; Song and Lee, 2007; Ramiro-Cortés and Morán, 2009). Moreover, there are evidence that p38 pathways can trigger NF-κβ translocation, further exacerbating degeneration in dopaminergic neurons of the SN in PD animal models (Karunakaran and Ravindranath, 2009; Yan et al., 2017). Furthermore, ROS contribute to the apoptotic pathway mediated by Trx-ASK1 and p38 in microglia, as Trx is an oxidative stress-sensitive marker that can trigger this pathway to regulate cell death (Noguchi et al., 2008; Hirata et al., 2020).
The cascade leading to apoptosis involves the activation of the transcription factor AP-1. Depending on the combination of proteins such as c-jun, c-fos, and others, AP-1 can regulate target genes that determine cell fate through mechanisms such as cell cycle progression, arrest, or apoptosis (Lee et al., 1987; Ameyar et al., 2003). The apoptotic activation of AP-1, leading to cell death, can be delayed by the expression of Bcl-2, which underscores the interaction between JNK/p38 pathways and intrinsic apoptosis (Bossy-Wetzel et al., 1997). Research has shown that p38 interacts with components of the intrinsic pathway and p53 (Perfettini et al., 2005; Farley et al., 2006), and this interaction is also observed in PD models (Karunakaran et al., 2008; Chen et al., 2018; Chen et al., 2020; Figure 1).
Finally, an important apoptosis activation pathway involves various stress signals that triggers the JNK/p38 pathway and leads to apoptosis. One of the most common stress signals in PD animal models and other oxidative-dependent diseases is the oxidative stress. In this context, sensor proteins such as Ask1/Trx are activated, which in turn activate JNK/p38, leading to apoptosis (Hsieh and Papaconstantinou, 2006; Pan et al., 2010; Hu et al., 2011; Yamada et al., 2012).
3.2 Necroptosis
Previously thought of as an unregulated and uncoordinated form of cell death, necrosis (or necroptosis) has been identified as an alternative, regulated pathway of cell death, primarily dependent on the tumor necrosis factor receptor 1 (TNFR1) and its ligand, TNF? Research has also shown that this pathway can induce apoptosis (Laster et al., 1988; Gao et al., 2024; Kazmi et al., 2024). This association has been noted since the 1990’s, with studies showing increased levels of TNFα and its type 1 receptor in the SNC of PD patients (Boka et al., 1994; Mogi et al., 1994; Sriram et al., 2002). Other extrinsic apoptotic mechanisms, such as Toll-like receptors (TLR) and Fas/FasL, can also trigger necrosis. Despite the different pathways leading to cell death, there is a significant crosstalk between them, which depends on the cellular and tissue context, such as after PD lesion stimuli in animal models (Hartmann et al., 2001; Oberst et al., 2011; Kaiser et al., 2011; Zhang et al., 2011; Table 3).
In general, these receptors interact with their ligands, and most of these pathways respond to their stimuli inducing NF-κβ gene transcription. However, some stimuli (e.g., pharmacological agents) can inhibit either RIPK1 or caspase 8 activity, thereby favoring the activation of necrosis pathways in vivo (Lin et al., 1999; Thapa et al., 2013). There are three key phenomena essential for the mechanism of necrosis: (1) the recruitment of RIPK1 to the TNFR1 by the adaptor protein TRADD, followed by the recruitment of TRAF2, another adaptor protein, which then associates with cIAP1/2, leading to a reduced caspase activation; (2) the recruitment and phosphorylation of RIPK3 by this membrane complex; and (3) the recruitment of mixed-lineage kinase-like protein (MLKL), which, upon phosphorylation by the RIPK1-RIPK3 complex, assembles into a new complex, called the necrosome (Sun et al., 2012; Chen et al., 2013; Weber et al., 2018; Faergeman et al., 2020; Figure 2).
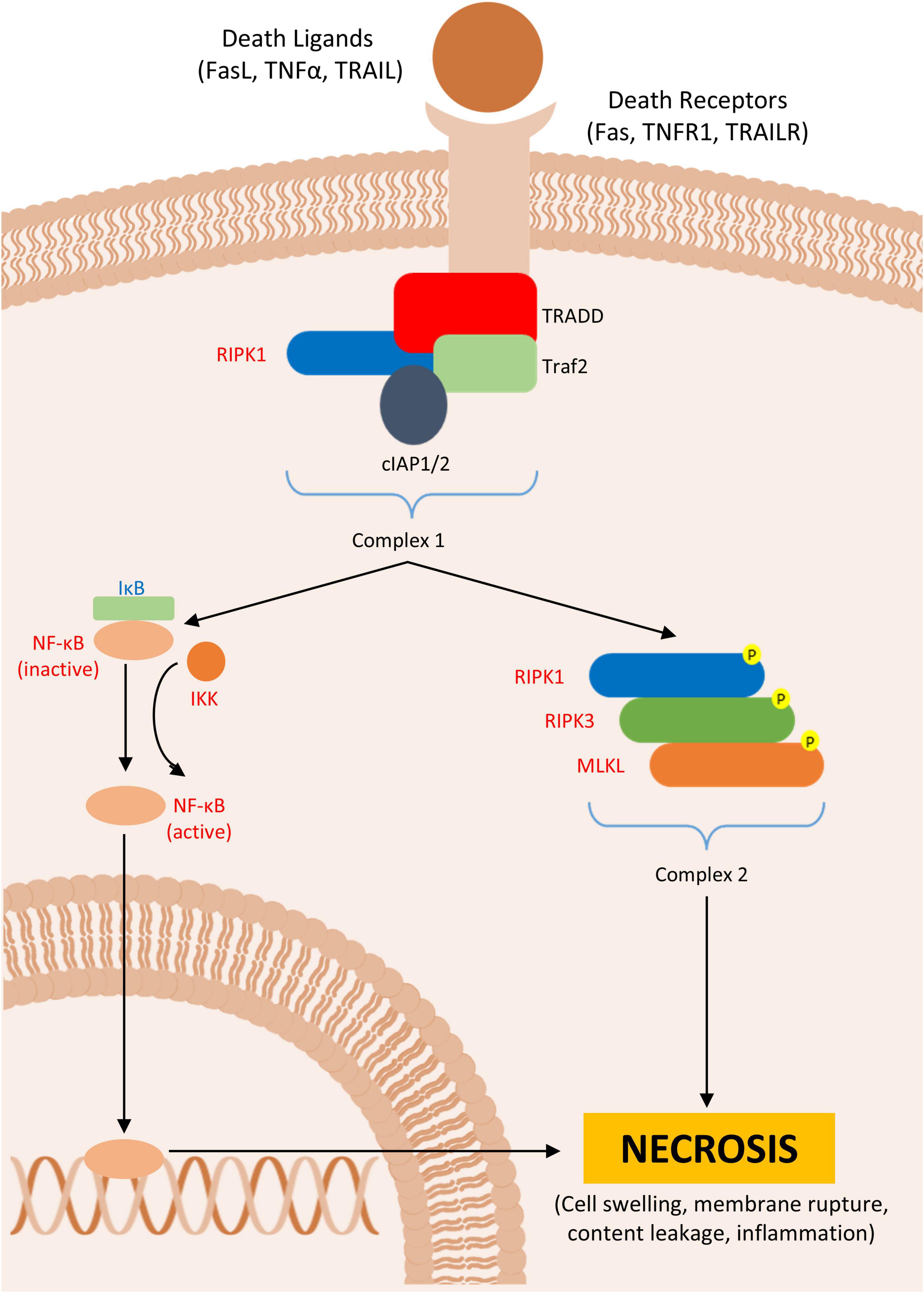
Figure 2. Necroptosis. Figure shows the formation of ripoptosome, which leads to necroptosis in Parkinson’s disease (PD) or PD animal models. In red are described upregulated- and in blue downregulated proteins in PD.
Necrosis is also observed in PD models. Inhibition of necrosis using necrostatin-1, a potent inhibitor of RIPK1, is associated with reduced dopaminergic cell death in the SN in both in vivo and in vitro models (Wu et al., 2015; Iannielli et al., 2018). Moreover, the formation of protein complexes associated with TNFR1 through RIPK1 has been observed in screenings of necrotic and apoptotic regulators. Genes associated with this protein aggregation have been correlated with the development of PD and other neurodegenerative diseases (Amin et al., 2018). It has been noted that the loss of cell integrity, associated with necrosis is an important hallmark of MPTP and rotenone PD cell models, with elevated expression of RIPK3 (Callizot et al., 2019).
Although studies have shown that inhibition of MLKL can reduce microglia activation and, consequently, inflammation (Lund et al., 2005; Geng et al., 2023), the presence of the necrosome cluster alone is not sufficient to initiate necrosis. MLKL is a self-inhibited protein; it requires binding of other proteins to expose its active domain and promote its migration to the plasma membrane, thereby completing the necroptotic pathway. This underscores the highly regulated nature of necrotic cell death (Dovey et al., 2018; McNamara et al., 2019). Upon activation, the MLKL complex migrates to the plasma membrane, where its accumulation forms hotspots that open ion channels, causing cell swelling, membrane rupture, and the formation of pores. This results in the extrusion of intracellular contents and subsequent necrosis (Yoon et al., 2014; Samson et al., 2020; Liu et al., 2024; Table 3).
Bioinformatic studies have shown that genes associated with necroptosis are altered in the brains of PD patients compared to control subjects, suggesting an increased susceptibility to necrotic cell death in these patients (Lei et al., 2023). Interestingly, investigations into the genetic variance risk related to intrinsic inhibition of TNFα or TNFR1-TNFα have found no correlation between the age of onset of PD and inhibition of this pathway, indicating that further research is needed to understand the role of necrosis in disease development (Kang et al., 2021). In PD models, evidence suggest that ablation of necroptosis effectors can attenuate inflammation and necrosis caused by neuroinflammation driven by agents like LPS or MPTP (Geng X. et al., 2023; Kim et al., 2023).
A particular form of necrosis, known as excitotoxicity, is caused by excessive stimulation of neurons by neurotransmitters (Choi, 1992). Increased activation of receptors, such as NMDAR and AMPAR, leads to an influx of ions like Ca2+, through the membrane. This increase ion concentration interacts with the endoplasmic reticulum, which in turn activates calpain, enhances ROS production and ultimately disrupts cell function. The disruptions can result in cell lysis, mitochondrial dysfunction, and organelle destruction (Brorson et al., 1995; D’Orsi et al., 2012; Gupta et al., 2013; Zhou et al., 2013; Polster et al., 2022). The relationship between excitotoxicity and development of PD has been extensively discussed (Ilijic et al., 2011; Pan et al., 2017; Soman et al., 2019)
3.3 Pyroptosis
Similar to necrosis, pyroptosis is a form of inflammatory, coordinated cell death. However, unlike necrosis, pyroptosis was initially described as a type of programmed cell death that, rather than being quiet, like apoptosis, triggers a robust inflammatory response, including the recruitment of immune cells to the site of death (Boise and Collins, 2001; Cookson and Brennan, 2001). A key characteristic of pyroptosis is the conversion of interleukin-1β (IL-1β) and interleukin-18 (IL-18) via caspase 1 (Thornberry et al., 1992). IL-1β is implicated in the neurodegeneration observed in PD as it can exacerbate neuroinflammation and promote the death of dopamine neurons, underscoring the significance of pyroptosis in PD (Koprich et al., 2008; Codolo et al., 2013; He et al., 2015; Table 4). Throughout the process of pyroptosis, caspase 1 is responsible for cell swelling (Fink and Cookson, 2006), DNA fragmentation (Bergsbaken and Cookson, 2007), the arrest of cell metabolism (Shao et al., 2007), and other related functions (Figure 3).
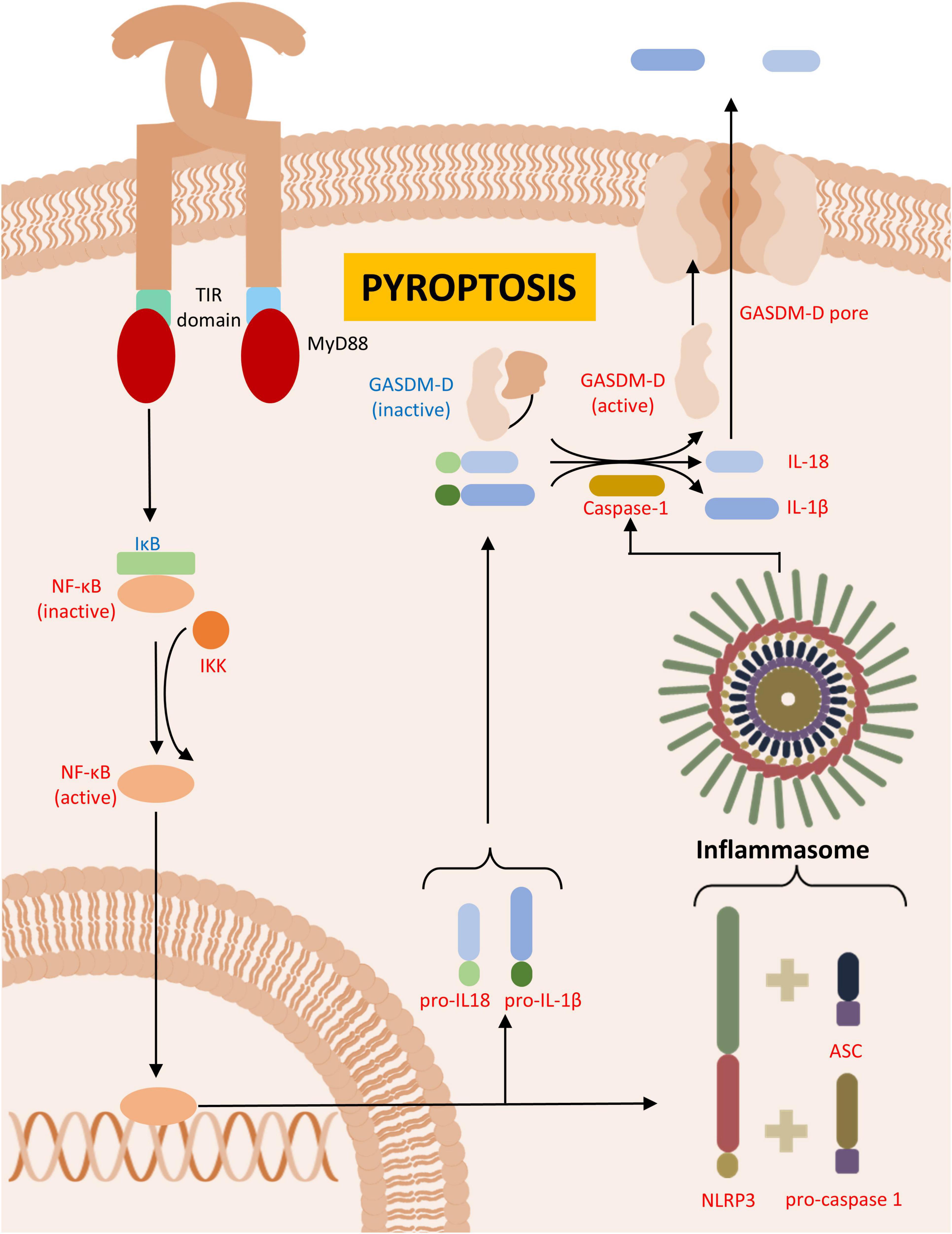
Figure 3. Pyroptosis. Figure illustrates the formation of inflammasome, leading to pyroptosis in Parkinson’s disease (PD) or PD animal models. In red are described upregulated- and in blue downregulated proteins in PD.
The initiation of pyroptosis depends on the sensing of the extracellular microenvironment, primarily through TLRs (Nyström et al., 2013) or the cytosolic space via NOD-like receptors (NLRs) (Qiu et al., 2017). TLRs are responsible for detecting pathogen-associated molecular patterns (PAMPs) and damage-associated molecular patterns (DAMPs) produced by cell death and pathogen elimination (Srikrishna and Freeze, 2009; Naqvi et al., 2022). Upon activation, the main pathway involved in the inflammatory responses is mediated by the production of cytokines such as TNFα, IL-6, IL-1β and IL-12 (Ozato et al., 2002; Cantó et al., 2006; Covacu et al., 2009; Rodriguez et al., 2019). This pathway relies on an intracellular domain, TIRAF, which recruits adapter proteins such as MyD88, triggering NF-κβ-regulated genes and modulating inflammatory responses (Bonnert et al., 1997; Zheng et al., 2019; Figure 3). Additional to this pathway, there are other pathways that involve different adaptor proteins, but ultimately lead to the activation of NF-κβ and other inflammatory signaling molecules, such as AP-1 (Yamamoto et al., 2002; Honda and Taniguchi, 2006).
NLRs can detect PAMPs and DAMPs and subsequently produce inflammatory cytokines regulated by NF-κβ (Abbott et al., 2004). However, certain NLRs belong to a specialized group that can form intracellular protein complexes known as inflammasomes, which contribute to ongoing pyroptosis and cytokines production – these are the NLRP receptors (Pétrilli et al., 2007; Hayrabedyan et al., 2016). For the inflammasome to assemble, the NLRP must interact with caspase 1-derived components, especially IL-1β (Martinon et al., 2002). It is known that the interaction of dopamine with NLRP3 can inhibit pyroptosis, further highlighting the relationship between this cell death mechanism and PD (Yan et al., 2015).
These receptors possess intracellular domains responsible for interacting with other proteins and protein complexes, such as CARDs bound to caspase 1, or using their pyrin domain to recruit CARDs – an essential stage for cytokines production and the binding of the adapter protein ASC (Boucher et al., 2018; Yang et al., 2019). In PD pathology, ASC specks are considered hallmarks of pyroptosis, as their expression has been observed in peripheral blood mononuclear cells, and this exacerbation is sufficient to enhance NLRP3 inflammasome formation (Fan et al., 2020; Zheng et al., 2023). The aggregation of multiple ASC specks forms the inflammasome, which role, through the action of bound caspase 1, is to promote cytokine processing. Moreover, by processing of gasdermin D (GDSM) into its active form, the inflammasome facilitates the inclusion of pores in the cell membrane, leading to cell lysis (Ding et al., 2016; Liu et al., 2016; Faria et al., 2021; Figure 3).
Conversely, drugs capable of inducing NLRP3 can establish PD-like models in animals (Wang Y. et al., 2022). Finally, other activation mechanisms contribute to the activation of the NLRP3 inflammasome, further aiding in the development of PD models in animals (Huang et al., 2024; Quan et al., 2024).
3.4 Autophagy
Autophagy, unlike the other pathways discussed here, is typically associated with cellular processes that promote cell survival. It plays a crucial role in energy conservation by recycling proteins and other cellular components to meet the energetic demand of cells (Yamamoto et al., 2023). Originally described by Christian de Duve in 1963 as a process involving lysosomes and their enzymes to degrade cellular components, autophagy is now understood to be as far more complex event that is intricately regulated by genetics, pathology, and other factors (Levine and Kroemer, 2008; Klionsky, 2008). There are three types of autophagy, each differing in morphology and mechanism, but leading to the degradation of cellular components within lysosome (Parzych and Klionsky, 2014).
The most studied type of autophagy is the macroautophagy, which involves the formation of bilipid membrane layer called an autophagosome. This structure engulfs organelles and proteins to be degraded, encloses the debris within the vesicular space, and degrades the proteins by fusing the autophagosome with a lysosome (Yi and Tang, 1999; Anding and Baehrecke, 2017; Figure 4). The formation of the autophagosome requires the expansion of its membrane, a process driven by autophagy-related genes known as Atgs (Takeshige et al., 1992). In both lower and higher eukaryotes, the initiation of autophagy requires the assembly of specific protein complexes: (1) the Atg1-Atg13-Atg17 (ULK1-Atg13-FIP200 in mammals) kinase complex, which initiates phagosome formation at the phagophore assembly site (PAS, an Atg8-rich cytoplasmatic site) (Kabeya et al., 2005; Chang and Neufeld, 2009); (2) the phosphatidylinositol 3-kinase complex 1, composed of vacuolar protein sorting proteins such as Beclin 1, PI3KR4, and PI3KC3 in mammals, responsible for the nucleation phase by directing proteins to the PAS through the generation of phosphatidylinositol 3-phosphate (PIP3) (Kihara et al., 2001; Reidick et al., 2017; Iershov et al., 2019); (3) ubiquitin-like assembly complexes, such as Atg12 and Atg8 (LC3 in mammals), which facilitated membrane elongation (Williams et al., 2009; Zhang et al., 2022); and (4) Atg9, a protein involved in the cycling of lipids between the autophagosome and other membranous structures like vesicles, aiding in protein enclose within the autophagosome and assisting in the elongation phase (Reggiori et al., 2005; Yen et al., 2007; Figure 4). After protein enclosure and autophagosome completion, lysosomes interact with this structure to degrade the autophagosome contents, a process mediated by SNARE complexes, which facilitate membrane fusion (Söllner et al., 1993; Hu et al., 2003; Hong, 2005; Jahn and Scheller, 2006).
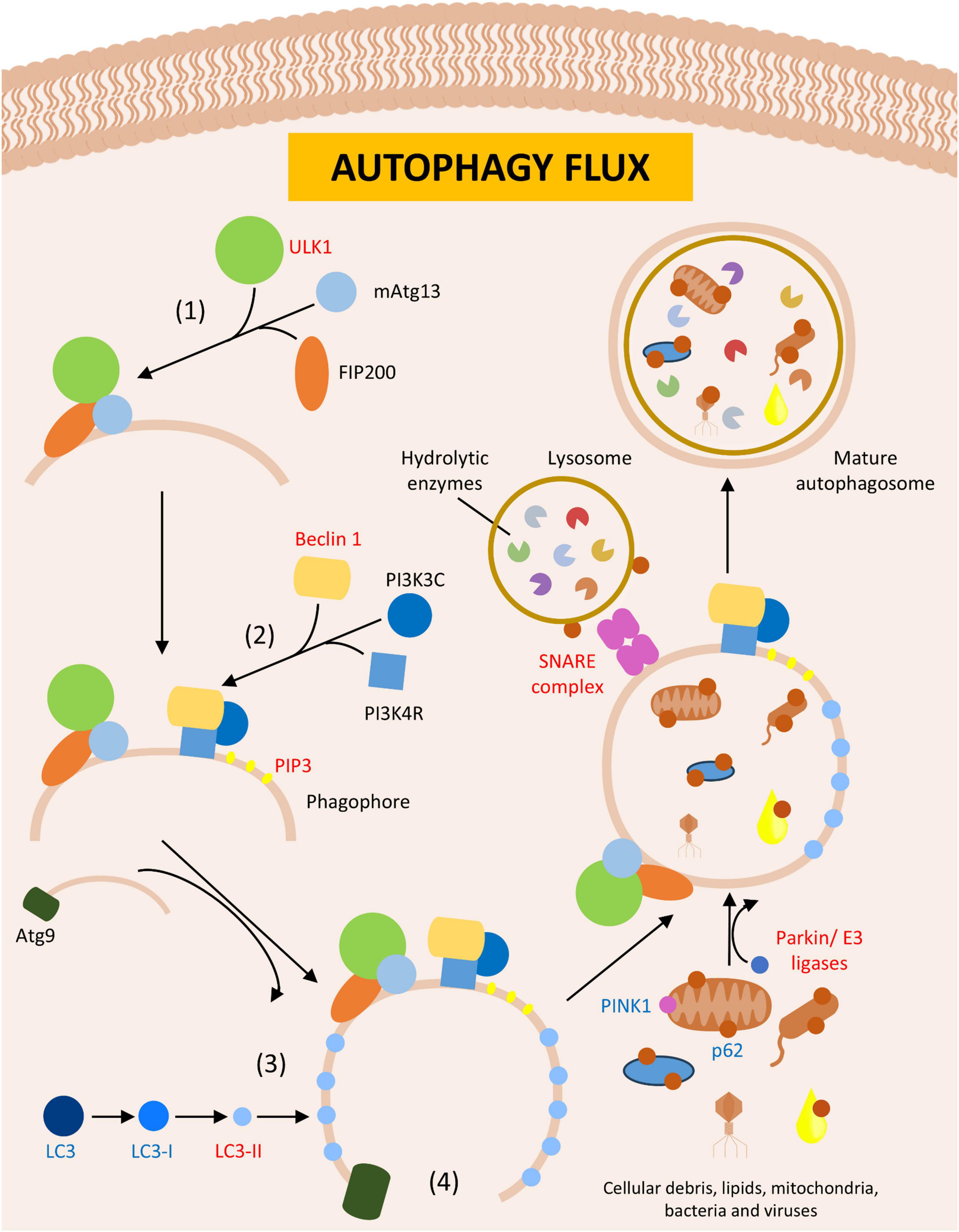
Figure 4. Autophagy. Figure shows the formation of autophagosome leading to dysregulation in autophagy flux in Parkinson’s disease (PD) or PD animal models. In red are described upregulated- and in blue downregulated proteins in PD.
Research on PD patients has shown abnormal expression of proteins involved in autophagy (Table 5) and Atg and ULK genes, which increased upstream regulators of autophagy and decreased downstream regulators, strengthening the link between the disease and this cellular mechanism (Miki et al., 2018). In mammals, LC3 is a widely observed gene related autophagy dysfunctions and serves as a marker of autophagy activity in cells (Kabeya et al., 2000). Additionally, Beclin1 is associated with autophagy and other vesicle transport pathways and plays an important role in the interplay between autophagy and other cell death mechanisms, as discussed further in this article (Liang et al., 1998; Kang et al., 2011; Lõrincz et al., 2014; Tran et al., 2021). Lastly, p62 is a protein that binds to ubiquitinated protein aggregates, cellular debris, bacteria, and viruses, targeting them for the autophagosome. It also interacts with proteins that lead to the autophagy of entire organelles, including the PINK1 protein, a genetic factor in PD, which is involved in mitochondria autophagy (Pankiv et al., 2007; Dagda et al., 2009; Lamark et al., 2009; Clausen et al., 2010; Geisler et al., 2010; Wurzer et al., 2015). Another important protein in PD development is PARK7/DJ1, which is involved in genetic variants of the disease. Its loss increases protein aggregation, overburdens autophagy and mitophagy, alters cellular machinery, and promotes oxidative stress (Krebiehl et al., 2010; Bai et al., 2020; Imberechts et al., 2022).
Another type of autophagy is the chaperone-mediated autophagy (CMA), a highly selective pathway that involves the transportation of specific proteins across the lysosome membrane via a receptor (Dice, 1990; Hubert et al., 2022; Fregno et al., 2018; Loi et al., 2019). In PD models, the role of this gene, along with LRRK2 - another key gene associated with autophagy in PD−has been shown to be crucial in stimulating autophagy and preventing neuronal degeneration (Issa et al., 2018; Ho et al., 2020).
4 Autophagy x apoptosis
The literature extensively debates the protective mechanisms involved in autophagy and whether, in neurodegenerative diseases, this mechanism acts as an inhibitor of cell death or, conversely, enhances death signaling and thus promotes cell death. Research indicates that, over the loss of critical transducer molecules within cell, such as MAPKs, allows adaptor proteins of receptors to interact with other proteins, which mobilize certain cell death mechanisms depending on the presence or absence of p62 (Goodall et al., 2016). Furthermore, there is an important connection between the permeabilization of mitochondria outer membrane and autophagy, as p62 serves as a tag regulating PUMA degradation, leading to a reduced apoptosis (Thorburn et al., 2014). More recent studies show that proteins such as mitochondria translation elongation factors are responsible for efficient mitochondrial autophagy (Zhu J. et al., 2023). Blocking these proteins results in caspase-8 activation and increased TNFα sensitivity, thereby enhancing and accelerating apoptosis (Choi et al., 2022). Conversely, autophagy appears to protect against apoptotic cell death by regulating proteins involved in both pathways, including Bcl-2 and Bcl-xL, and Beclin1 (Pattingre et al., 2005; Maiuri et al., 2007). According to Maycotte and colleagues, autophagy may precede apoptosis and is necessary for caspase activation, as seen in increased autophagosome formation and LC3 processing when the cells (rat cerebellar granule neurons) were treated with substances that increase ROS, thereby decreasing the cell viability (Maycotte et al., 2010).
On the other hand, in neurodegenerative diseases, the impaired function of the autophagy system itself appears to be direct consequence of blockage caused by the accumulation of misfolded proteins. This impairment is exarcebated by an increase in ROS, despite autophagy’s critical role in removing these proteins aggregates (Bandyopadhyay and Cuervo, 2007; Janda et al., 2012). Furthermore, the amount of Ca2+ released from the endoplasmic reticulum plays an important role in regulating apoptosis, as it signals the mitochondria, modulating autophagy suggesting a complex interplay between these pathways and necrosis, especially excitotoxicity-induced necrosis (Høyer-Hansen et al., 2007; Cárdenas et al., 2010).
However, studies also show that while autophagy may contribute to death signaling in neurons, it simultaneously acts as a mechanism to continuously monitoring the cell’s survival state. Research has described a reciprocal regulation between Atg7 and caspase 9, resulting in an autophagy-dependent apoptosis flux (Han et al., 2014; Ojha et al., 2016). Moreover, the preservation of cell integrity via autophagy is essential for preventing neurodegeneration in dopaminergic neurons through apoptosis, involving a regulatory mechanism linked to the AKT/mTOR pathway (Zhu et al., 2024).
It is known that autophagy is defective in dopaminergic neurons in the SN, as observed in post-mortem brains of patients and certain models of PD like 6-OHDA and rotenone-induced models, which show suppression of mTOR, a key enhancer of autophagy (Grassi et al., 2018). In α-synuclein-induced PD mouse model, Zhang and collaborators showed that the administration of caffeic acid prevents the neurodegeneration of dopaminergic neurons in SN and improves behavioral abnormalities by stimulating autophagy through the JNK/BcL2 pathway (Zhang et al., 2019). Moreover, miRNAs have an important role in regulating autophagy-related genes and signaling pathways. When downregulated, these miRNAs are responsible for neuroprotection by either activating protective autophagy or reducing autophagic neuronal cell death (Choi et al., 2016; Sarkar et al., 2022).
5 Novel revelations regarding cell death mechanisms in discrete nuclei affected in PD, notably within critical centers like the respiratory system
Current understanding about the role of cell death in Parkinson’s disease is due to neurodegeneration in midbrain, notably within the SN. Although other brain regions have been studied to explore the disease’s impact on the degeneration of unconventional areas, limited insight exists regarding the connection between cell death mechanisms, such as apoptosis, and the neurodegeneration observed in nuclei that govern neural control of breathing. This connection has been previously described alongside functional deficits in the 6-OHDA model of PD (Tuppy et al., 2015; Fernandes-Junior et al., 2018; Oliveira et al., 2019; Figure 5). It is important to note that, since death signaling is not confined to the SN and its related areas, similar signaling may also occur throughout the brain in PD patients and models. Through research is needed to understand the extent of such signaling in these regions. In post-mortem studies of the human brains affected by PD, Benarroch demonstrated that the ventrolateral medulla, which houses the nucleus responsible for respiratory rhythmicity, showed degeneration evidenced by a reduction in NK1 receptors (Benarroch et al., 2003). However, the mechanisms underlying the neurodegeneration of respiratory nuclei in PD remain poorly understood.
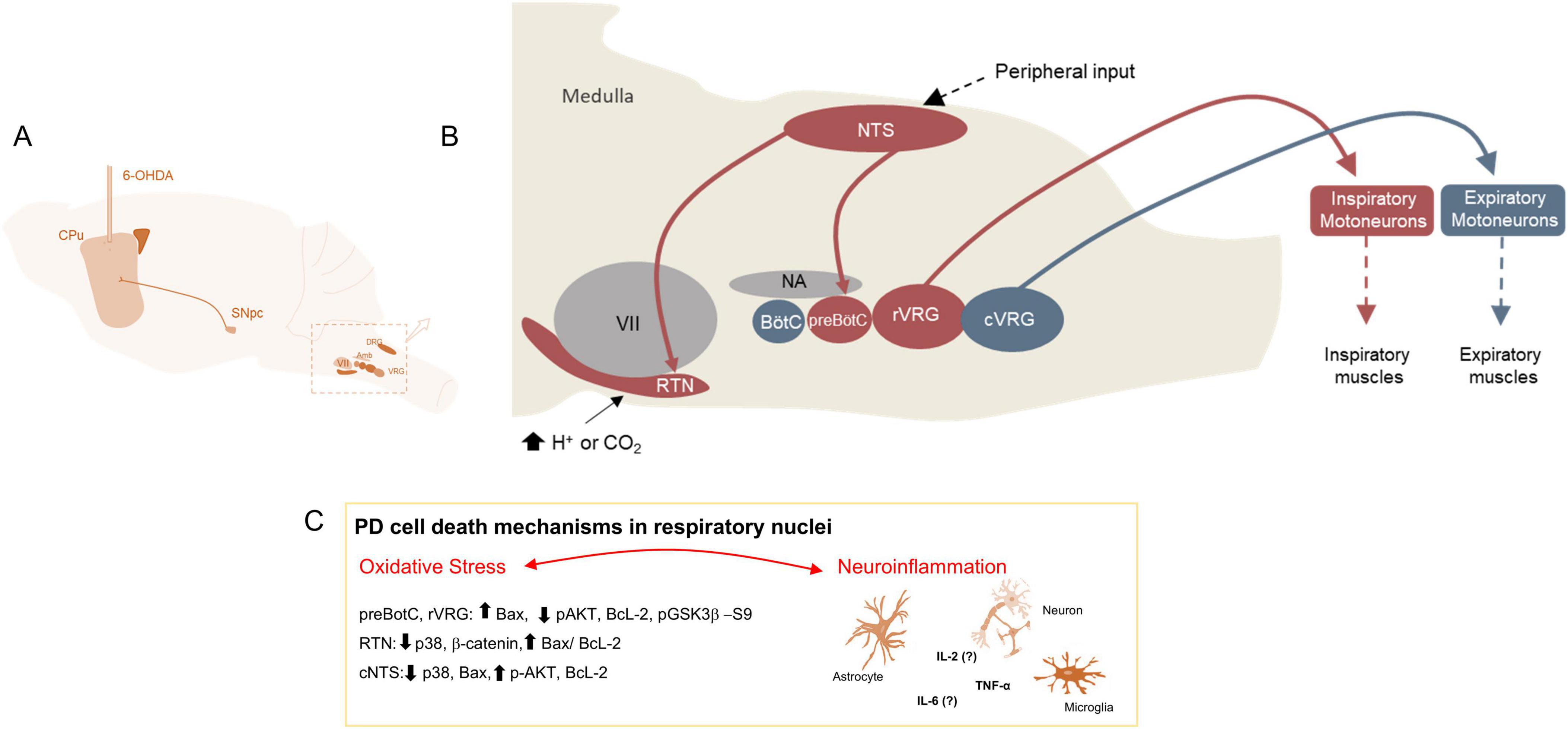
Figure 5. Changes in neural control of breathing in the PD model. (A) Figure shows the PD model induction by the injections of 6-OHDA in the CPu leading to degeneration of SN. (B) Figure shows the representation of medullary nuclei that are responsible for neural control of breathing. In red are represented nuclei that are degenerated in the PD model. (C) Figure shows the mechanisms involved in cell death in the respiratory nuclei. CPu, Caudate-Putamen; SN, Substantia Nigra; VRG, Ventral Respiratory Group; RTN, Retrotrapezoid Nucleus; BötC, Botzinger Complex; preBötC, PreBotzinger Complex; rVRG, Rostral portion of the Ventral Respiratory Group; cVRG, Caudal portion of the Ventral Respiratory Group; DRG, Dorsal Respiratory Group; NTS, Nucleus of the Solitary Tract; NA or Amb, Ambiguus Nucleus; VII: Facial Nucleus.
Briefly, the neuronal circuitry that controls ventilatory function is located within the medulla oblongata and pons. The pre-Bötzinger Complex (preBötC) is responsible for generating the inspiratory rhythm through pacemaker glutamatergic neurons, which project to the rostral ventral respiratory group (rVRG), a group of pre-motor neurons situated in the ventral region of the medulla (Yang C. F. et al., 2020). The rVRG innervates the diaphragm, initiating its contraction, which expands the thoracic cavity and allows air to enter the lungs (Smith et al., 1991; Vann et al., 2018; Dhingra et al., 2024). Additionally, other nuclei in the pons modulate upper airway muscle activity, creating optimal conditions for air to maximize contact with alveoli, thereby promoting gas exchange. This modulation is also essential for maintaining eupnea and generate various respiratory behaviors (Song et al., 2012; Levitt et al., 2015; Abdala et al., 2016; Farmer et al., 2014; Dutschmann et al., 2021).
The Bötzinger Complex (BötC) and lateral parafacial (pFL) control the expiration, the final phase of respiration, during which air is expelled from the lungs. This process occurs either through passive relaxation of the diaphragm or active contraction of muscles, enabling CO2-rich air to leave the lungs (Huckstepp et al., 2015; de Britto and Moraes, 2017; Zoccal et al., 2018; Silva et al., 2019). Finally, nuclei such as the retrotrapezoid nucleus (RTN) and the nucleus of solitary tract (NTS) play crucial role in sensing or receiving information related to the partial pressure of CO2 and O2, as well as variations in blood pH, thereby fine-tuning the neural control of breathing (Takakura et al., 2006; de Paula et al., 2007; Del Rio et al., 2012; Ott et al., 2012; Díaz et al., 2020).
Regarding the degeneration of respiratory nuclei, it is known that 30 days after the injection of 6-OHDA in the rat’s CPu, pro-apoptotic signaling occurs in the preBötC and the rVRG. This signaling is characterized by increased intrinsic and extrinsic signaling involving Bax/BcL-2 proteins, leading to the loss of NK1 receptors after 40 days of PD induction, which results in breathing dysfunction (Falquetto et al., 2020). Similarly, in the RTN and NTS, these nuclei experience loss of phox2b+ neurons 30 days post-6-OHDA injection. At the same time, they exhibit an anti-apoptotic signaling, as an attempt by the system to recover from the injury. This is demonstrated by a reduction in p38 and Bax and increase of pAKT levels (Falquetto et al., 2020; Aquino et al., 2022; Figure 5). Moreover, oxidative stress is the main candidate responsible for impaired breathing in the PD model, as observed in SN; treatment with apocynin, an antioxidant drug, prevented the neurodegeneration in respiratory nuclei and mitigated respiratory dysfunction in the PD rat model (Nascimento et al., 2022). Lastly, a study has shown the involvement of glial cells and TNF-α in the degeneration of respiratory nuclei and breathing dysfunction in mouse model of PD, underscoring the importance of neuroinflammation (Cabral et al., 2024; Figure 5).
Mechanism under which these neurons may die might connect with neuron’s death Braak’s hypothesis. According to this hypothesis, α-synuclein fibrils spread through the axons in a gut-brain orientation, with the dorsal motor nucleus of the vagus nerve (DMV) (Braak et al., 2003). This dorsal nucleus is known to be connected to other breathing control centers, such as the NTS (Rogers et al., 1980; Kalia and Sullivan, 1982; Davis et al., 2003; Davis et al., 2004), which in turn projects to and receives projections from other respiratory nuclei (Alheid et al., 2011; Yang x. et al., 2020; Biancardi et al., 2021). Conversely, neurodegeneration in brainstem breathing control nuclei might also be explained by the projection of olfactory bulb (OB) neurons to various brain areas. Notably, the OB connects to the NTS via the paraventricular nucleus (Guevara-Guzman et al., 1991), to the locus coeruleus (Shipley et al., 1985; McLean and Shipley, 1991), and to the SN (Höglinger et al., 2015). Moreover, evidence suggests connection between the SN, periaqueductal gray, and the RTN (Lima et al., 2018; Aquino et al., 2022). One might hypothesize the relative contribution of these pathways to the development of degeneration in the respiratory circuitry. However, there is limited understanding of neuronal death in these nuclei in both PD models and human patients, representing a potential area for further research. Overall, these studies underscore the importance of investigating the signaling pathways that lead to cell death in PD models, as these pathways can impair ventilation, potentially affecting the lifespan of animals and, consequently, human health.
6 Conclusion
Despite our current understanding of cell death in PD being insufficient to cure the disease, science has advanced considerably since James Parkinson first described it. As the global population ages and the incidence of neurodegenerative diseases increases, it is imperative for biomedical research to better understand the causes and progression of these diseases to improve health outcomes to the elderly. Basic research aimed at elucidating additional factors involved in these pathways is crucial for identifying more target molecules and developing novel therapies that may slow or halt the progression of neurodegenerative diseases. Our comprehension is that as these diseases progress, multiple cellular and tissue mechanisms are recruited to reestablish homeostasis. However, cellular damage often advances faster than the recovery mechanisms can address it, leading to multiple signaling pathways that promote different forms of cell death. This results in tissue damage, and especially on nervous tissue, creates a “dead space” where cells cannot recover due to the lack of neurogenesis. It is important to recognize that while the disease affects the SN neurons and cause the classical symptoms, other brain regions, such as respiratory nuclei, also undergo degeneration. This contributes to symptoms and suffering in patients, highlighting the need for further investigation into the relationship between SN neurons death and the degenerations of other brain areas.
The characteristics of cell death presented here suggest that the molecular aspects most prevalent in PD models can be leveraged to prevent the degeneration of other regions affected during disease progression, potentially extending patient’s lifespan and improving their quality of life. Although a cure for PD has not yet been found, a better understanding of the molecular pathways and genetic variants involved could lead to improve early diagnosis protocols. The protocols could include assessments of patient’s genetic susceptibility and the identifications of better biomarkers to enhance diagnosis accuracy.
Given current hypothesis about the disease’s origins, a promising starting point is to investigate the role of α-synuclein more deeply. It is already established that Lewy’s bodies, which are composed of misfold α-synuclein, contribute to cellular stress that can trigger various forms of cell death (Jiang et al., 2017; Gordon et al., 2018; Ardah et al., 2021; Gao et al., 2022; Bae et al., 2022; Lin et al., 2023; Yildirim-Balatan et al., 2024; Jia et al., 2024). The critical question that remains is whether halting of α-synuclein misfolding and aggregation is enough to stop disease progression or, ideally, to prevent the disease from developing altogether. To address it, it is essential for scientific research to focus on the understanding the underlying causes of PD, the mechanisms of neuronal death, and the identification of new therapeutic targets. By improving early diagnosis and employing available tools to treat patients as soon as possible, we can work towards preventing the establishment of the disease and ensuring a healthier ageing process for the.
Author contributions
LP: Writing – original draft, Writing – review & editing. PM: Writing – original draft, Writing – review & editing. EL: Writing – review & editing. BF: Writing – original draft, Writing – review & editing.
Funding
The author(s) declare financial support was received for the research, authorship, and/or publication of the article. This study was supported by the São Paulo Research Foundation (FAPESP; grants: 2019/00065-1 and 2023/00980-7 to BF; fellowships: 2021/12538-1 and 2023/11274-6 to LP; 2021/08562-4 to PM; 2023/09695-3 to EL). This study was financed in part by the Coordenação de Aperfeiçoamento de Pessoal de Nível Superior-Brasil (CAPES)−Finance Code 001.
Conflict of interest
The authors declare that the research was conducted in the absence of any commercial or financial relationships that could be construed as a potential conflict of interest.
Publisher’s note
All claims expressed in this article are solely those of the authors and do not necessarily represent those of their affiliated organizations, or those of the publisher, the editors and the reviewers. Any product that may be evaluated in this article, or claim that may be made by its manufacturer, is not guaranteed or endorsed by the publisher.
References
Aarsland, D., Batzu, L., Halliday, G. M., Geurtsen, G. J., Ballard, C., Ray Chaudhuri, K., et al. (2021). Parkinson disease-associated cognitive impairment. Nat. Rev. Dis. Prim. 7, 1–21. doi: 10.1038/s41572-021-00280-3
Abbott, D. W., Wilkins, A., Asara, J. M., and Cantley, L. C. (2004). The Crohn’s disease protein, NOD2, requires RIP2 in order to induce ubiquitinylation of a novel site on NEMO. Curr. Biol. 14, 2217–2227. doi: 10.1016/j.cub.2004.12.032
Abdala, A. P., Toward, M. A., Dutschmann, M., Bissonnette, J. M., and Paton, J. F. R. (2016). Deficiency of GABAergic synaptic inhibition in the Kölliker-Fuse area underlies respiratory dysrhythmia in a mouse model of Rett syndrome. The J. Physiol. 594, 223–237. doi: 10.1113/JP270966
Ahmed, S., Kwatra, M., Ranjan Panda, S., Murty, U. S. N., and Naidu, V. G. M. (2021). Andrographolide suppresses NLRP3 inflammasome activation in microglia through induction of parkin-mediated mitophagy in in-vitro and in-vivo models of Parkinson disease. Brain Behav. Immun. 91, 142–158. doi: 10.1016/j.bbi.2020.09.017
Alheid, G. F., Jiao, W., and McCrimmon, D. R. (2011). Caudal nuclei of the rat nucleus of the solitary tract differentially innervate respiratory compartments within the ventrolateral medulla. Neuroscience 190, 207–227. doi: 10.1016/j.neuroscience.2011.06.005
Ameyar, M., Wisniewska, M., and Weitzman, J. B. (2003). A role for AP-1 in apoptosis: The case for and against. Biochimie 85, 747–752. doi: 10.1016/j.biochi.2003.09.006
Amin, P., Florez, M., Najafov, A., Pan, H., Geng, J., Ofengeim, D., et al. (2018). Regulation of a distinct activated RIPK1 intermediate bridging complex I and complex II in TNFα-mediated apoptosis. Proc. Natl. Acad. U.S.A. 115, E5944–E5953. doi: 10.1073/pnas.1806973115
Anding, A. L., and Baehrecke, E. H. (2017). Cleaning house: Selective autophagy of organelles. Dev. Cell 41, 10–22. doi: 10.1016/j.devcel.2017.02.016
Anson, F., Thayumanavan, S., and Hardy, J. A. (2021). Exogenous introduction of initiator and executioner caspases results in different apoptotic outcomes. JACS Au 1, 1240–1256. doi: 10.1021/jacsau.1c00261
Aquino, Y. C., Cabral, L. M., Miranda, N. C., Naccarato, M. C., Falquetto, B., Moreira, T. S., et al. (2022). Respiratory disorders of Parkinson’s disease. J. Neurophysiol. 127, 1–15. doi: 10.1152/jn.00363.2021
Ardah, M. T., Eid, N., Kitada, T., and Haque, M. E. (2021). Ellagic acid prevents α-synuclein aggregation and protects SH-SY5Y cells from aggregated α-synuclein-induced toxicity via suppression of apoptosis and activation of autophagy. Int. J. Mol. Sci. 22:13398. doi: 10.3390/ijms222413398
Bae, E.-J., Choi, M., Kim, J. T., Kim, D.-K., Jung, M. K., Kim, C., et al. (2022). TNF-α promotes α-synuclein propagation through stimulation of senescence-associated lysosomal exocytosis. Exp. Mol. Med. 54, 788–800. doi: 10.1038/s12276-022-00789-x
Bai, H., Ding, Y., Li, X., Kong, D., Xin, C., Yang, X., et al. (2020). Polydatin protects SH-SY5Y in models of Parkinson’s disease by promoting Atg5-mediated but parkin-independent autophagy. Neurochem. Int. 134:104671. doi: 10.1016/j.neuint.2020.104671
Bandyopadhyay, U., and Cuervo, A. M. (2007). Chaperone-mediated autophagy in aging and neurodegeneration: Lessons from alpha-synuclein. Exp. Gerontol. 42, 120–128. doi: 10.1016/j.exger.2006.05.019
Bao, Q., and Shi, Y. (2007). Apoptosome: A platform for the activation of initiator caspases. Cell Death Differ. 14:1. doi: 10.1038/sj.cdd.4402028
Belizário, J., Vieira-Cordeiro, L., and Enns, S. (2015). Necroptotic cell death signaling and execution pathway: Lessons from knockout mice. Mediat. Inflamm. 2015:128076. doi: 10.1155/2015/128076
Benamer, H. T. S., and de Silva, R. (2010). LRRK2 G2019S in the North African population: A review. Eur. Neurol. 63, 321–325. doi: 10.1159/000279653
Benarroch, E. E., Schmeichel, A. M., Low, P. A., and Parisi, J. E. (2003). Depletion of ventromedullary NK-1 receptor-immunoreactive neurons in multiple system atrophy. Brain J. Neurol. 126(Pt 10), 2183–2190. doi: 10.1093/brain/awg220
Bergsbaken, T., and Cookson, B. T. (2007). Macrophage activation redirects yersinia-infected host cell death from apoptosis to caspase-1-dependent pyroptosis. PLoS Pathog. 3:e161. doi: 10.1371/journal.ppat.0030161
Bhattacharyya, P., Biswas, A., and Biswas, S. C. (2022). Brain-enriched miR-128: Reduced in exosomes from Parkinson’s patient plasma, improves synaptic integrity, and prevents 6-OHDA mediated neuronal apoptosis. Front. Cell. Neurosci. 16:1037903. doi: 10.3389/fncel.2022.1037903
Biancardi, V., Saini, J., Pageni, A., Prashaad, M. H., Funk, G. D., and Pagliardini, S. (2021). Mapping of the excitatory, inhibitory, and modulatory afferent projections to the anatomically defined active expiratory oscillator in adult male rats. J. Comp. Neurol. 529, 853–884. doi: 10.1002/cne.24984
Bloem, B. R., Okun, M. S., and Klein, C. (2021). Parkinson’s disease. Lancet 397, 2284–2303. doi: 10.1016/S0140-6736(21)00218-X
Blum, D., Wu, Y., Nissou, M. F., Arnaud, S., and Alim-Louis-Benabid Verna, J. M. (1997). P53 and Bax activation in 6-hydroxydopamine-induced apoptosis in PC12 cells. Brain Res. 751, 139–142. doi: 10.1016/s0006-8993(96)01358-3
Bodmer, J. L., Holler, N., Reynard, S., Vinciguerra, P., Schneider, P., Juo, P., et al. (2000). TRAIL receptor-2 signals apoptosis through FADD and caspase-8. Nat. Cell Biol. 2, 241–243. doi: 10.1038/35008667
Boise, L. H., and Collins, C. M. (2001). Salmonella-induced cell death: Apoptosis, necrosis or programmed cell death? Trends Microbiol. 9, 64–67. doi: 10.1016/s0966-842x(00)01937-5
Boka, G., Anglade, P., Wallach, D., Javoy-Agid, F., Agid, Y., and Hirsch, E. C. (1994). Immunocytochemical analysis of tumor necrosis factor and its receptors in Parkinson’s disease. Neurosci. Lett. 172, 151–154. doi: 10.1016/0304-3940(94)90684-x
Bonnert, T. P., Garka, K. E., Parnet, P., Sonoda, G., Testa, J. R., and Sims, J. E. (1997). The cloning and characterization of human MyD88: A member of an IL-1 receptor related family 1. FEBS Lett. 402, 81–84. doi: 10.1016/S0014-5793(96)01506-2
Bossy-Wetzel, E., Bakiri, L., and Yaniv, M. (1997). Induction of apoptosis by the transcription factor c-Jun. EMBO J. 16, 1695–1709. doi: 10.1093/emboj/16.7.1695
Boucher, D., Monteleone, M., Coll, R. C., Chen, K. W., Ross, C. M., Teo, J. L., et al. (2018). Caspase-1 self-cleavage is an intrinsic mechanism to terminate inflammasome activity. J. Exp. Med. 215, 827–840. doi: 10.1084/jem.20172222
Braak, H., Rüb, U., Gai, W. P., and Del Tredici, K. (2003). Idiopathic Parkinson’s disease: Possible routes by which vulnerable neuronal types may be subject to neuroinvasion by an unknown pathogen. J. Neural Transm. 110, 517–536. doi: 10.1007/s00702-002-0808-2
Brentnall, M., Rodriguez-Menocal, L., De Guevara, R. L., Cepero, E., and Boise, L. H. (2013). Caspase-9, caspase-3 and caspase-7 have distinct roles during intrinsic apoptosis. BMC Cell Biol. 14:32. doi: 10.1186/1471-2121-14-32
Brorson, J. R., Marcuccilli, C. J., and Miller, R. J. (1995). Delayed antagonism of calpain reduces excitotoxicity in cultured neurons. Stroke 26, 1259–1267. doi: 10.1161/01.STR.26.7.1259
Cabral, L. M., Oliveira, L. M., Miranda, N. C., Kawamoto, E. M., Costa, K. P., Moreira, T. S., et al. (2024). TNFR1-mediated neuroinflammation is necessary for respiratory deficits observed in 6-hydroxydopamine mouse model of Parkinso’s disease. Brain Res. 1822:148586. doi: 10.1016/j.brainres.2023.148586
Cai, M., Zhuang, W., Lv, E., Liu, Z., Wang, Y., Zhang, W., et al. (2022). Kaemperfol alleviates pyroptosis and microglia-mediated neuroinflammation in Parkinson’s disease via inhibiting p38MAPK/NF-κB signaling pathway. Neurochem. Int. 152:105221. doi: 10.1016/j.neuint.2021.105221
Callizot, N., Combes, M., Henriques, A., and Poindron, P. (2019). Necrosis, apoptosis, necroptosis, three modes of action of dopaminergic neuron neurotoxins. PLoS One 14:e0215277. doi: 10.1371/journal.pone.0215277
Cano, E., and Mahadevan, L. C. (1995). Parallel signal processing among mammalian MAPKs. Trends Biochem. Sci. 20, 117–122. doi: 10.1016/s0968-0004(00)88978-1
Cano, E., Hazzalin, C. A., and Mahadevan, L. C. (1994). Anisomycin-activated protein kinases p45 and p55 but not mitogen-activated protein kinases ERK-1 and -2 are implicated in the induction of c-fos and c-jun. Mol. Cell. Biol. 14, 7352–7362. doi: 10.1128/mcb.14.11.7352-7362.1994
Cantó, E., Ricart, E., Monfort, D., González-Juan, D., Balanzó, J., Rodríguez-Sánchez, J. L., et al. (2006). TNF alpha production to TLR2 ligands in active IBD patients. Clin. Immunol. 119, 156–165. doi: 10.1016/j.clim.2005.12.005
Cárdenas, C., Miller, R. A., Smith, I., Bui, T., Molgó, J., Müller, M., et al. (2010). Essential regulation of cell bioenergetics by constitutive InsP3 receptor Ca2+ transfer to mitochondria. Cell 142, 270–283. doi: 10.1016/j.cell.2010.06.007
Cassarino, D. S., Halvorsen, E. M., Swerdlow, R. H., Abramova, N. N., Parker, W. D., Sturgill, T. W., et al. (2000). Interaction among mitochondria, mitogen-activated protein kinases, and nuclear factor-kappaB in cellular models of Parkinson’s disease. J. Neurochem. 74, 1384–1392. doi: 10.1046/j.1471-4159.2000.0741384.x
Cecconi, F., Alvarez-Bolado, G., Meyer, B. I., Roth, K. A., and Gruss, P. (1998). Apaf1 (CED-4 homolog) regulates programmed cell death in mammalian development. Cell 94, 727–737. doi: 10.1016/s0092-8674(00)81732-8
Cenini, G., Lloret, A., and Cascella, R. (2020). Oxidative stress and mitochondrial damage in neurodegenerative diseases: From molecular mechanisms to targeted therapies. Oxid. Med. Cell. Longev. 2020:1270256. doi: 10.1155/2020/1270256
Chang, L., Kamata, H., Solinas, G., Luo, J.-L., Maeda, S., Venuprasad, K., et al. (2006). The E3 ubiquitin ligase itch couples JNK activation to TNFα-induced cell death by inducing c-FLIPL turnover. Cell 124, 601–613. doi: 10.1016/j.cell.2006.01.021
Chang, Y.-Y., and Neufeld, T. P. (2009). An Atg1/Atg13 complex with multiple roles in TOR-mediated autophagy regulation. Mol. Biol. Cell 20, 2004–2014. doi: 10.1091/mbc.e08-12-1250
Chen, C., Ren, Y., Chen, J., Wu, X., Mao, K., Li, H., et al. (2020). P38 MAPK-DRP1 signaling is involved in mitochondrial dysfunction and cell death in mutant A53T α-synuclein model of Parkinson’s disease. Toxicol. Appl. Pharmacol. 388:4874. doi: 10.1016/j.taap.2019.114874
Chen, J., Ren, Y., Gui, C., Zhao, M., Wu, X., Mao, K., et al. (2018). Phosphorylation of Parkin at serine 131 by p38 MAPK promotes mitochondrial dysfunction and neuronal death in mutant A53T α-synuclein model of Parkinson’s disease. Cell Death Dis. 9:700. doi: 10.1038/s41419-018-0722-7
Chen, W., Zhou, Z., Li, L., Zhong, C.-Q., Zheng, X., Wu, X., et al. (2013). Diverse sequence determinants control human and mouse receptor interacting protein 3 (RIP3) and mixed lineage kinase domain-like (MLKL) interaction in necroptotic signaling. J. Biol. Chem. 288, 16247–16261. doi: 10.1074/jbc.M112.435545
Chi, H., Chang, H.-Y., and Sang, T.-K. (2018). Neuronal cell death mechanisms in major neurodegenerative diseases. Int. J. Mol. Sci. 19:3082. doi: 10.3390/ijms19103082
Choi, C.-Y., Vo, M. T., Nicholas, J., and Choi, Y. B. (2022). Autophagy-competent mitochondrial translation elongation factor TUFM inhibits caspase-8-mediated apoptosis. Cell Death Differ. 29, 451–464. doi: 10.1038/s41418-021-00868-y
Choi, I., Woo, J. H., Jou, I., and Joe, E. (2016). PINK1 deficiency decreases expression levels of mir-326, mir-330, and mir-3099 during brain development and neural stem cell differentiation. Exp. Neurobiol. 25, 14–23. doi: 10.5607/en.2016.25.1.14
Choi, W. S., Yoon, S. Y., Oh, T. H., Choi, E. J., O’Malley, K. L., and Oh, Y. J. (1999). Two distinct mechanisms are involved in 6-hydroxydopamine- and MPP+-induced dopaminergic neuronal cell death: Role of caspases, ROS, and JNK. J. Neurosci. Res. 57, 86–94. doi: 10.1002/(SICI)1097-4547(19990701)57:1<86::AID-JNR9>3.0.CO;2-E
Choi, W.-S., Eom, D.-S., Han, B. S., Kim, W. K., Han, B. H., Choi, E.-J., et al. (2004). Phosphorylation of p38 MAPK induced by oxidative stress is linked to activation of both caspase-8- and -9-mediated apoptotic pathways in dopaminergic neurons. J. Biol. Chem. 279, 20451–20460. doi: 10.1074/jbc.M311164200
Chou, T.-W., Chang, N. P., Krishnagiri, M., Patel, A. P., Lindman, M., Angel, J. P., et al. (2021). Fibrillar α-synuclein induces neurotoxic astrocyte activation via RIP kinase signaling and NF-κB. Cell Death Dis. 12:756. doi: 10.1038/s41419-021-04049-0
Cicchetti, F., Lapointe, N., Roberge-Tremblay, A., Saint-Pierre, M., Jimenez, L., Ficke, B. W., et al. (2005). Systemic exposure to paraquat and maneb models early Parkinson’s disease in young adult rats. Neurobiol. Dis. 20, 360–371. doi: 10.1016/j.nbd.2005.03.018
Clark, L. N., Ross, B. M., Wang, Y., Mejia-Santana, H., Harris, J., Louis, E. D., et al. (2007). Mutations in the glucocerebrosidase gene are associated with early-onset Parkinson disease. Neurology 69, 1270–1277. doi: 10.1212/01.wnl.0000276989.17578.02
Clausen, T. H., Lamark, T., Isakson, P., Finley, K., Larsen, K. B., Brech, A., et al. (2010). P62/SQSTM1 and ALFY interact to facilitate the formation of p62 bodies/ALIS and their degradation by autophagy. Autophagy 6, 330–344. doi: 10.4161/auto.6.3.11226
Codolo, G., Plotegher, N., Pozzobon, T., Brucale, M., Tessari, I., Bubacco, L., et al. (2013). Triggering of inflammasome by aggregated α–synuclein, an inflammatory response in synucleinopathies. PLoS One 8:e55375. doi: 10.1371/journal.pone.0055375
Cookson, B. T., and Brennan, M. A. (2001). Pro-inflammatory programmed cell death. Trends Microbiol. 9, 113–114. doi: 10.1016/S0966-842X(00)01936-3
Covacu, R., Arvidsson, L., Andersson, A., Khademi, M., Erlandsson-Harris, H., Harris, R. A., et al. (2009). TLR activation induces TNF-alpha production from adult neural stem/progenitor cells. J. Immunol. 182, 6889–6895. doi: 10.4049/jimmunol.0802907
D’Orsi, B., Bonner, H., Tuffy, L. P., Düssmann, H., Woods, I., Courtney, M. J., et al. (2012). Calpains are downstream effectors of bax-dependent excitotoxic apoptosis. J. Neurosci. 32, 1847–1858. doi: 10.1523/JNEUROSCI.2345-11.2012
Dagan, E., Schlesinger, I., Ayoub, M., Mory, A., Nassar, M., Kurolap, A., et al. (2015). The contribution of Niemann-Pick SMPD1 mutations to Parkinson disease in Ashkenazi Jews. Parkins. Relat. Disord. 21, 1067–1071. doi: 10.1016/j.parkreldis.2015.06.016
Dagda, R. K., Cherra, S. J., Kulich, S. M., Tandon, A., Park, D., and Chu, C. T. (2009). Loss of PINK1 function promotes mitophagy through effects on oxidative stress and mitochondrial fission. J. Biol. Chem. 284, 13843–13855. doi: 10.1074/jbc.M808515200
Dailah, H. G. (2022). Potential of therapeutic small molecules in apoptosis regulation in the treatment of neurodegenerative diseases: An updated review. Molecules 27:7207. doi: 10.3390/molecules27217207
Davis, S. F., Derbenev, A. V., Williams, K. W., Glatzer, N. R., and Smith, B. N. (2004). Excitatory and inhibitory local circuit input to the rat dorsal motor nucleus of the vagus originating from the nucleus tractus solitarius. Brain Res. 1017, 208–217. doi: 10.1016/j.brainres.2004.05.049
Davis, S. F., Williams, K. W., Xu, W., Glatzer, N. R., and Smith, B. N. (2003). Selective enhancement of synaptic inhibition by hypocretin (orexin) in rat vagal motor neurons: Implications for autonomic regulation. J. Neurosci. 23, 3844–3854. doi: 10.1523/JNEUROSCI.23-09-03844.2003
de Britto, A. A., and Moraes, D. J. A. (2017). Non-chemosensitive parafacial neurons simultaneously regulate active expiration and airway patency under hypercapnia in rats. J. Physiol. 595, 2043–2064. doi: 10.1113/JP273335
de Paula, P. M., Tolstykh, G., and Mifflin, S. (2007). Chronic intermittent hypoxia alters NMDA and AMPA-evoked currents in NTS neurons receiving carotid body chemoreceptor inputs. Am. J. Physiol. 292, R2259–R2265. doi: 10.1152/ajpregu.00760.2006
de Rijk, M. C., Breteler, M. M. B., Graveland, G. A., Ott, A., Grobbee, D. E., Van Der Meche, F. G. A., et al. (1995). Prevalence of Parkinson’s disease in the elderly: The Rotterdam study. Neurology 45, 2143–2146. doi: 10.1212/WNL.45.12.2143
Del Rio, R., Moya, E. A., Parga, M. J., Madrid, C., and Iturriaga, R. (2012). Carotid body inflammation and cardiorespiratory alterations in intermittent hypoxia. Eur. Respir. J. 39, 1492–1500. doi: 10.1183/09031936.00141511
Deumens, R., Blokland, A., and Prickaerts, J. (2002). Modeling Parkinson’s disease in rats: An evaluation of 6-OHDA lesions of the nigrostriatal pathway. Exp. Neurol. 175, 303–317. doi: 10.1006/exnr.2002.7891
Dhingra, R. R., Furuya, W. I., Yoong, Y. K., and Dutschmann, M. (2024). The pre-Bötzinger complex is necessary for the expression of inspiratory and post-inspiratory motor discharge of the vagus. Respir. Physiol. Neurobiol. 320:104202. doi: 10.1016/j.resp.2023.104202
Díaz, H. S., Andrade, D. C., Toledo, C., Pereyra, K. V., Schwarz, K. G., Díaz-Jara, E., et al. (2020). Episodic stimulation of central chemoreceptor neurons elicits disordered breathing and autonomic dysfunction in volume overload heart failure. Am. J. Physiol. 318, L27–L40. doi: 10.1152/ajplung.00007.2019
Dice, J. F. (1990). Peptide sequences that target cytosolic proteins for lysosomal proteolysis. Trends Biochem. Sci. 15, 305–309. doi: 10.1016/0968-0004(90)90019-8
Ding, J., Wang, K., Liu, W., She, Y., Sun, Q., Shi, J., et al. (2016). Pore-forming activity and structural autoinhibition of the gasdermin family. Nature 535:7610. doi: 10.1038/nature18590
Dionísio, P. A., Amaral, J. D., and Rodrigues, C. M. P. (2021). Oxidative stress and regulated cell death in Parkinson’s disease. Ageing Res. Rev. 67:101263. doi: 10.1016/j.arr.2021.101263
Dong, Y., Wang, X., Zhang, J., Guan, Z., Xu, L., Wang, J., et al. (2015). Raltitrexed’s effect on the development of neural tube defects in mice is associated with DNA damage, apoptosis, and proliferation. Mol. Cell. Biochem. 398, 223–231. doi: 10.1007/s11010-014-2222-0
Dorstyn, L., Akey, C. W., and Kumar, S. (2018). New insights into apoptosome structure and function. Cell Death Differ. 25:7. doi: 10.1038/s41418-017-0025-z
dos Santos Pereira, M., Abreu, G. H. D., Rocca, J., Hamadat, S., and Raisman-Vozari, R. (2021). Contributive role of TNF-α to L-DOPA-induced dyskinesia in a unilateral 6-OHDA lesion model of Parkinson’s disease. Front. Pharmacol. 11:617085. doi: 10.3389/fphar.2020.617085
dos Santos, A. V., Pestana, C. P., Diniz, K. R., da, S., Campos, M., Abdalla-Carvalho, C. B., et al. (2010). Mutational analysis of GIGYF2, ATP13A2 and GBA genes in Brazilian patients with early-onset Parkinson’s disease. Neurosci. Lett. 485, 121–124. doi: 10.1016/j.neulet.2010.08.083
Dovey, C. M., Diep, J., Clarke, B. P., Hale, A. T., McNamara, D. E., Guo, H., et al. (2018). MLKL Requires the Inositol Phosphate Code to Execute Necroptosis. Mol. Cell 70:936–948.e7. doi: 10.1016/j.molcel.2018.05.010
Dugger, B. N., and Dickson, D. W. (2017). Pathology of neurodegenerative diseases. Cold Spring Harb. Perspect. Biol. 9:a028035. doi: 10.1101/cshperspect.a028035
Dutta, D., Jana, M., Majumder, M., Mondal, S., Roy, A., and Pahan, K. (2021). Selective targeting of the TLR2/MyD88/NF-κB pathway reduces α-synuclein spreading in vitro and in vivo. Nat. Commun. 12:5382. doi: 10.1038/s41467-021-25767-1
Dutschmann, M., Bautista, T. G., Trevizan-Baú, P., Dhingra, R. R., and Furuya, W. I. (2021). The pontine Kölliker-Fuse nucleus gates facial, hypoglossal, and vagal upper airway related motor activity. Respir. Physiol. Neurobiol. 284:103563. doi: 10.1016/j.resp.2020.103563
Elesawy, W. H., El-Sahar, A. E., Sayed, R. H., Ashour, A. M., Alsufyani, S. E., Arab, H. H., et al. (2024). Repurposing ezetimibe as a neuroprotective agent in a rotenone-induced Parkinson’s disease model in rats: Role of AMPK/SIRT-1/PGC-1α signaling and autophagy. Int. Immunopharmacol. 138:112640. doi: 10.1016/j.intimp.2024.112640
Elfawy, H. A., and Das, B. (2019). Crosstalk between mitochondrial dysfunction, oxidative stress, and age related neurodegenerative disease: Etiologies and therapeutic strategies. Life Sci. 218, 165–184. doi: 10.1016/j.lfs.2018.12.029
Elmore, S. (2007). Apoptosis: A review of programmed cell death. Toxicol. Pathol. 35, 495–516. doi: 10.1080/01926230701320337
Erekat, N. S. (2018). “Apoptosis and its role in Parkinson’s disease,” in Parkinson’s disease: Pathogenesis and clinical aspects, eds T. B. Stoker and J. C. Greenland (Singapore: Codon Publications).
Espinosa-Oliva, A. M., Ruiz, R., Soto, M. S., Boza-Serrano, A., Rodriguez-Perez, A. I., Roca-Ceballos, M. A., et al. (2024). Inflammatory bowel disease induces pathological α-synuclein aggregation in the human gut and brain. Neuropathol. Appl. Neurobiol. 50:e12962. doi: 10.1111/nan.12962
Faergeman, S. L., Evans, H., Attfield, K. E., Desel, C., Kuttikkatte, S. B., Sommerlund, M., et al. (2020). A novel neurodegenerative spectrum disorder in patients with MLKL deficiency. Cell Death Dis. 11:5. doi: 10.1038/s41419-020-2494-0
Fall, C. P., and Bennett, J. P. (1999). Characterization and time course of MPP+-induced apoptosis in human SH-SY5Y neuroblastoma cells. J. Neurosci. Res. 55, 620–628. doi: 10.1002/(SICI)1097-4547(19990301)55:5<620::AID-JNR9<3.0.CO;2-S
Falquetto, B., Thieme, K., Malta, M. B., Rocha, E., Tuppy, M., Potje, S. R., et al. (2020). Oxidative stress in the medullary respiratory neurons contributes to respiratory dysfunction in the 6-OHDA model of Parkinson’s disease. J. Physiol. 598, 5271–5293. doi: 10.1113/JP279791
Fan, Z., Pan, Y.-T., Zhang, Z.-Y., Yang, H., Yu, S.-Y., Zheng, Y., et al. (2020). Systemic activation of NLRP3 inflammasome and plasma α-synuclein levels are correlated with motor severity and progression in Parkinson’s disease. J. Neuroinflamm. 17:11. doi: 10.1186/s12974-019-1670-6
Faria, S. S., Costantini, S., de Lima, V. C. C., de Andrade, V. P., Rialland, M., Cedric, R., et al. (2021). NLRP3 inflammasome-mediated cytokine production and pyroptosis cell death in breast cancer. J. Biomed. Sci. 28:26. doi: 10.1186/s12929-021-00724-8
Farley, N., Pedraza-Alva, G., Serrano-Gomez, D., Nagaleekar, V., Aronshtam, A., Krahl, T., et al. (2006). P38 mitogen-activated protein kinase mediates the fas-induced mitochondrial death pathway in CD8+ T cells. Mol. Cell. Biol. 26, 2118–2129. doi: 10.1128/MCB.26.6.2118-2129.2006
Farmer, D. G. S., Bautista, T. G., Jones, S. E., Stanic, D., and Dutschmann, M. (2014). The midbrain periaqueductal grey has no role in the generation of the respiratory motor pattern, but provides command function for the modulation of respiratory activity. Respir. Physiol. Neurobiol. 204, 14–20. doi: 10.1016/j.resp.2014.07.011
Fernandes-Junior, S. A., Carvalho, K. S., Moreira, T. S., and Takakura, A. C. (2018). Correlation between neuroanatomical and functional respiratory changes observed in an experimental model of Parkinson’s disease. Exp. Physiol. 103, 1377–1389. doi: 10.1113/EP086987
Ferrante, R. J., Schulz, J. B., Kowall, N. W., and Beal, M. F. (1997). Systemic administration of rotenone produces selective damage in the striatum and Globus pallidus, but not in the Substantia nigra. Brain Res. 753, 157–162. doi: 10.1016/s0006-8993(97)00008-5
Fink, S. L., and Cookson, B. T. (2006). Caspase-1-dependent pore formation during pyroptosis leads to osmotic lysis of infected host macrophages. Cell. Microbiol. 8, 1812–1825. doi: 10.1111/j.1462-5822.2006.00751.x
Fogarty, L. C., Flemmer, R. T., Geizer, B. A., Licursi, M., Karunanithy, A., Opferman, J. T., et al. (2019). Mcl-1 and Bcl-xL are essential for survival of the developing nervous system. Cell Death Differ. 26, 1501–1515. doi: 10.1038/s41418-018-0225-1
Fregno, I., Fasana, E., Bergmann, T. J., Raimondi, A., Loi, M., Soldà, T., et al. (2018). ER-to-lysosome-associated degradation of proteasome-resistant ATZ polymers occurs via receptor-mediated vesicular transport. EMBO J. 37:e99259. doi: 10.15252/embj.201899259
Fu, R.-H., Tsai, C.-W., Liu, S.-P., Chiu, S.-C., Chen, Y.-C., Chiang, Y.-T., et al. (2022). Neuroprotective capability of narcissoside in 6-OHDA-exposed Parkinson’s disease models through enhancing the MiR200a/Nrf-2/GSH axis and mediating MAPK/Akt associated signaling pathway. Antioxidants 11:2089. doi: 10.3390/antiox11112089
Gao, Q., Chen, R., Wu, L., Huang, Q., Wang, X.-X., Tian, Y.-Y., et al. (2022). Angiotensin-(1-7) reduces α-synuclein aggregation by enhancing autophagic activity in Parkinson’s disease. Neural Regener. Res. 17, 1138–1145. doi: 10.4103/1673-5374.324854
Gao, Y., Sheng, D., and Chen, W. (2024). Regulatory mechanism of miR-20a-5p in neuronal damage and inflammation in lipopolysaccharide-induced BV2 cells and MPTP-HCl-induced Parkinson’s disease mice. Psychogeriatrics 24, 752–764. doi: 10.1111/psyg.13109
Garcia-Garcia, A., Anandhan, A., Burns, M., Chen, H., Zhou, Y., and Franco, R. (2013). Impairment of Atg5-dependent autophagic flux promotes paraquat- and MPP+-induced apoptosis but not rotenone or 6-hydroxydopamine toxicity. Toxicol. Sci. 136, 166–182. doi: 10.1093/toxsci/kft188
Geisler, S., Holmström, K. M., Skujat, D., Fiesel, F. C., Rothfuss, O. C., Kahle, P. J., et al. (2010). PINK1/Parkin-mediated mitophagy is dependent on VDAC1 and p62/SQSTM1. Nat. Cell Biol. 12, 119–131. doi: 10.1038/ncb2012
Geng, L., Gao, W., Saiyin, H., Li, Y., Zeng, Y., Zhang, Z., et al. (2023). MLKL deficiency alleviates neuroinflammation and motor deficits in the α-synuclein transgenic mouse model of Parkinson’s disease. Mol. Neurodegener. 18:94. doi: 10.1186/s13024-023-00686-5
Geng, X., Zou, Y., Li, J., Li, S., Qi, R., Yu, H., et al. (2023). BDNF alleviates Parkinson’s disease by promoting STAT3 phosphorylation and regulating neuronal autophagy. Cell Tissue Res. 393, 455–470. doi: 10.1007/s00441-023-03806-1
Gómez, N., and Cohen, P. (1991). Dissection of the protein kinase cascade by which nerve growth factor activates MAP kinases. Nature 353, 170–173. doi: 10.1038/353170a0
Goodall, M. L., Fitzwalter, B., Zahedi, S., Wu, M., Rodriguez, D., Mulcahy-Levy, J. M., et al. (2016). The autophagy machinery controls cell death switching between apoptosis and necroptosis. Dev. Cell 37, 337–349. doi: 10.1016/j.devcel.2016.04.018
Gordon, R., Albornoz, E. A., Christie, D. C., Langley, M. R., Kumar, V., Mantovani, S., et al. (2018). Inflammasome inhibition prevents α-synuclein pathology and dopaminergic neurodegeneration in mice. Sci. Transl. Med. 10:eaah4066. doi: 10.1126/scitranslmed.aah4066
Gorman, A. M., McGowan, A., O’Neill, C., and Cotter, T. (1996). Oxidative stress and apoptosis in neurodegeneration. J. Neurol. Sci. 139, 45–52. doi: 10.1016/0022-510x(96)00097-4
Grassi, D., Howard, S., Zhou, M., Diaz-Perez, N., Urban, N. T., Guerrero-Given, D., et al. (2018). Identification of a highly neurotoxic α-synuclein species inducing mitochondrial damage and mitophagy in Parkinson’s disease. Proc Natl. Acad. Sci. U.S.A. 115, E2634–E2643. doi: 10.1073/pnas.1713849115
Guevara-Guzman, R., Garcia-Diaz, D. E., Solano-Flores, L. P., Wayner, M. J., and Armstrong, D. L. (1991). Role of the paraventricular nucleus in the projection from the nucleus of the solitary tract to the olfactory bulb. Brain Res. Bull. 27, 447–450. doi: 10.1016/0361-9230(91)90140-F
Guimarães, B. de C., Pereira, A. C. V., Rodrigues, F. da C., dos Santos, A. V., Campos, M., dos Santos, J. M., et al. (2012). Glucocerebrosidase N370S and L444P mutations as risk factors for Parkinson’s disease in Brazilian patients. Parkinsonism Relat. Disord. 18, 688–689. doi: 10.1016/j.parkreldis.2011.11.028
Gupta, K., Hardingham, G. E., and Chandran, S. (2013). NMDA receptor-dependent glutamate excitotoxicity in human embryonic stem cell-derived neurons. Neurosci. Lett. 543, 95–100. doi: 10.1016/j.neulet.2013.03.010
Han, J., Hou, W., Goldstein, L. A., Stolz, D. B., Watkins, S. C., and Rabinowich, H. (2014). A Complex between Atg7 and Caspase-9: A novel mechanism of cross-regulation between autophagy and apoptosis. J. Biol. Chem. 289, 6485–6497. doi: 10.1074/jbc.M113.536854
Hartley, A., Stone, J. M., Heron, C., Cooper, J. M., and Schapira, A. H. V. (1994). Complex I inhibitors induce dose-dependent apoptosis in PC12 cells: Relevance to Parkinson’s disease. J. Neurochem. 63, 1987–1990. doi: 10.1046/j.1471-4159.1994.63051987.x
Hartmann, A., Troadec, J. D., Hunot, S., Kikly, K., Faucheux, B. A., Mouatt-Prigent, A., et al. (2001). Caspase-8 is an effector in apoptotic death of dopaminergic neurons in Parkinson’s disease, but pathway inhibition results in neuronal necrosis. J. Neurosci. 21, 2247–2255. doi: 10.1523/JNEUROSCI.21-07-02247.2001
Hayrabedyan, S., Todorova, K., Jabeen, A., Metodieva, G., Toshkov, S., Metodiev, M. V., et al. (2016). Sertoli cells have a functional NALP3 inflammasome that can modulate autophagy and cytokine production. Sci. Rep. 6:1. doi: 10.1038/srep18896
He, D., Liu, Y., Li, J., Wang, H., Ye, B., He, Y., et al. (2022). Isoalantolactone (IAL) regulates neuro-inflammation and neuronal apoptosis to curb pathology of Parkinson’s disease. Cells 11:2927. doi: 10.3390/cells11182927
He, W., Wan, H., Hu, L., Chen, P., Wang, X., Huang, Z., et al. (2015). Gasdermin D is an executor of pyroptosis and required for interleukin-1β secretion. Cell Res. 25, 1285–1298. doi: 10.1038/cr.2015.139
Healy, D. G., Falchi, M., O’Sullivan, S. S., Bonifati, V., Durr, A., Bressman, S., et al. (2008). Phenotype, genotype, and worldwide genetic penetrance of LRRK2-associated Parkinson’s disease: A case-control study. Lancet 7, 583–590. doi: 10.1016/S1474-4422(08)70117-0
Heikkila, R. E., Hess, A., and Duvoisin, R. C. (1984). Dopaminergic neurotoxicity of 1-methyl-4-phenyl-1,2,5,6-tetrahydropyridine in mice. Science 224, 1451–1453. doi: 10.1126/science.6610213
Hirata, Y., Nada, Y., Yamada, Y., Toyama, T., Fukunaga, K., Hwang, G.-W., et al. (2020). Elaidic acid potentiates extracellular ATP-induced apoptosis via the P2X7-ROS-ASK1-p38 axis in microglial cell lines. Biol. Pharm. Bull. 43, 1562–1569. doi: 10.1248/bpb.b20-00409
Ho, P. W.-L., Leung, C.-T., Liu, H., Pang, S. Y.-Y., Lam, C. S.-C., Xian, J., et al. (2020). Age-dependent accumulation of oligomeric SNCA/α-synuclein from impaired degradation in mutant LRRK2 knockin mouse model of Parkinson disease: Role for therapeutic activation of chaperone-mediated autophagy (CMA). Autophagy 16, 347–370. doi: 10.1080/15548627.2019.1603545
Höglinger, G. U., Alvarez-Fischer, D., Arias-Carrión, O., Djufri, M., Windolph, A., Keber, U., et al. (2015). A new dopaminergic nigro-olfactory projection. Acta Neuropathol. 130, 333–348. doi: 10.1007/s00401-015-1451-y
Honda, K., and Taniguchi, T. (2006). IRFs: Master regulators of signalling by Toll-like receptors and cytosolic pattern-recognition receptors. Nat. Rev. Immunol. 6:9. doi: 10.1038/nri1900
Hong, W. (2005). SNAREs and traffic. Biochim. Biophys. Acta 1744, 120–144. doi: 10.1016/j.bbamcr.2005.03.014
Høyer-Hansen, M., Bastholm, L., Szyniarowski, P., Campanella, M., Szabadkai, G., Farkas, T., et al. (2007). Control of macroautophagy by calcium, calmodulin-dependent kinase kinase-beta, and Bcl-2. Mol. Cell 25, 193–205. doi: 10.1016/j.molcel.2006.12.009
Hsieh, C.-C., and Papaconstantinou, J. (2006). Thioredoxin-ASK1 complex levels regulate ROS-mediated p38 MAPK pathway activity in livers of aged and long-lived Snell dwarf mice. FASEB J. 20, 259–268. doi: 10.1096/fj.05-4376com
Hu, C., Ahmed, M., Melia, T. J., Söllner, T. H., Mayer, T., and Rothman, J. E. (2003). Fusion of cells by flipped SNAREs. Science 300, 1745–1749. doi: 10.1126/science.1084909
Hu, X., Weng, Z., Chu, C. T., Zhang, L., Cao, G., Gao, Y., et al. (2011). Peroxiredoxin-2 protects against 6-hydroxydopamine-induced dopaminergic neurodegeneration via attenuation of the apoptosis signal-regulating kinase (ASK1) signaling cascade. J. Neurosci. 31, 247–261. doi: 10.1523/JNEUROSCI.4589-10.2011
Huang, B., Liu, J., Meng, T., Li, Y., He, D., Ran, X., et al. (2018). Polydatin prevents lipopolysaccharide (LPS)-induced Parkinson’s disease via regulation of the AKT/GSK3β-Nrf2/NF-κB signaling axis. Front. Immunol. 9:2527. doi: 10.3389/fimmu.2018.02527
Huang, P., Zhang, Z., Zhang, P., Feng, J., Xie, J., Zheng, Y., et al. (2024). TREM2 deficiency aggravates NLRP3 inflammasome activation and pyroptosis in MPTP-induced Parkinson’s disease mice and LPS-induced BV2 cells. Mol. Neurobiol. 61, 2590–2605. doi: 10.1007/s12035-023-03713-0
Hubert, V., Weiss, S., Rees, A. J., and Kain, R. (2022). Modulating chaperone-mediated autophagy and its clinical applications in cancer. Cells 11:2562. doi: 10.3390/cells11162562
Huckstepp, R. T. R., Cardoza, K. P., Henderson, L. E., and Feldman, J. L. (2015). Role of parafacial nuclei in control of breathing in adult rats. J. Neurosci. 35, 1052–1067. doi: 10.1523/JNEUROSCI.2953-14.2015
Hulihan, M. M., Ishihara-Paul, L., Kachergus, J., Warren, L., Amouri, R., Elango, R., et al. (2008). LRRK2 Gly2019Ser penetrance in Arab-Berber patients from Tunisia: A case-control genetic study. Lancet Neurol. 7, 591–594. doi: 10.1016/S1474-4422(08)70116-9
Iannielli, A., Bido, S., Folladori, L., Segnali, A., Cancellieri, C., Maresca, A., et al. (2018). Pharmacological inhibition of necroptosis protects from dopaminergic neuronal cell death in Parkinson’s disease models. Cell Rep. 22, 2066–2079. doi: 10.1016/j.celrep.2018.01.089
Idrissi, S. E., Fath, N., Ibork, H., Taghzouti, K., Alamy, M., and Abboussi, O. (2023). restraint stress exacerbates apoptosis in a 6-OHDA animal model of Parkinson disease. Neurotox. Res. 41, 166–176. doi: 10.1007/s12640-022-00630-3
Iershov, A., Nemazanyy, I., Alkhoury, C., Girard, M., Barth, E., Cagnard, N., et al. (2019). The class 3 PI3K coordinates autophagy and mitochondrial lipid catabolism by controlling nuclear receptor PPARα. Nat. Commun. 10:1. doi: 10.1038/s41467-019-09598-9
Ilijic, E., Guzman, J. N., and Surmeier, D. J. (2011). The L-type channel antagonist isradipine is neuroprotective in a mouse model of Parkinson’s disease. Neurobiol. Dis. 43, 364–371. doi: 10.1016/j.nbd.2011.04.007
Imberechts, D., Kinnart, I., Wauters, F., Terbeek, J., Manders, L., Wierda, K., et al. (2022). DJ-1 is an essential downstream mediator in PINK1/parkin-dependent mitophagy. Brain J. Neurol. 145, 4368–4384. doi: 10.1093/brain/awac313
Issa, A.-R., Sun, J., Petitgas, C., Mesquita, A., Dulac, A., Robin, M., et al. (2018). The lysosomal membrane protein LAMP2A promotes autophagic flux and prevents SNCA-induced Parkinson disease-like symptoms in the Drosophila brain. Autophagy 14, 1898–1910. doi: 10.1080/15548627.2018.1491489
Itano, Y., and Nomura, Y. (1995). 1-methyl-4-phenyl-pyridinium ion (MPP+) causes DNA fragmentation and increases the Bcl-2 expression in human neuroblastoma, SH-SY5Y cells, through different mechanisms. Brain Res. 704, 240–245. doi: 10.1016/0006-8993(95)01120-x
Jacobs, M. D., and Harrison, S. C. (1998). Structure of an IκBα/NF-κB complex. Cell 95, 749–758. doi: 10.1016/S0092-8674(00)81698-0
Jahn, R., and Scheller, R. H. (2006). SNAREs—Engines for membrane fusion. Nat. Rev. Mol. Cell Biol. 7, 631–643. doi: 10.1038/nrm2002
Jan, R., and Chaudhry, G.-S. (2019). Understanding apoptosis and apoptotic pathways targeted cancer therapeutics. Adv. Pharm. Bull. 9, 205–218. doi: 10.15171/apb.2019.024
Janda, E., Isidoro, C., Carresi, C., and Mollace, V. (2012). Defective autophagy in Parkinson’s disease: Role of oxidative stress. Mol. Neurobiol. 46, 639–661. doi: 10.1007/s12035-012-8318-1
Jia, X., Chen, Q., Yao, C., Asakawa, T., and Zhang, Y. (2024). α-synuclein regulates Cyclin D1 to promote abnormal initiation of the cell cycle and induce apoptosis in dopamine neurons. Biomed. Pharmacother. 173:116444. doi: 10.1016/j.biopha.2024.116444
Jiang, P., Gan, M., Yen, S.-H., McLean, P. J., and Dickson, D. W. (2017). Histones facilitate α-synuclein aggregation during neuronal apoptosis. Acta Neuropathol. 133, 547–558. doi: 10.1007/s00401-016-1660-z
Jiang, Z., Yin, X., Wang, M., Wang, Y., Li, F., Gao, Y., et al. (2022). β-Hydroxybutyrate alleviates pyroptosis in MPP+/MPTP-induced Parkinson’s disease models via inhibiting STAT3/NLRP3/GSDMD pathway. Int. Immunopharmacol. 113:109451. doi: 10.1016/j.intimp.2022.109451
Kabeya, Y., Kamada, Y., Baba, M., Takikawa, H., Sasaki, M., and Ohsumi, Y. (2005). Atg17 functions in cooperation with Atg1 and Atg13 in yeast autophagy. Mol. Biol. Cell 16, 2544–2553. doi: 10.1091/mbc.E04-08-0669
Kabeya, Y., Mizushima, N., Ueno, T., Yamamoto, A., Kirisako, T., Noda, T., et al. (2000). LC3, a mammalian homologue of yeast Apg8p, is localized in autophagosome membranes after processing. EMBO J. 19, 5720–5728. doi: 10.1093/emboj/19.21.5720
Kaiser, W. J., Upton, J. W., Long, A. B., Livingston-Rosanoff, D., Daley-Bauer, L. P., Hakem, R., et al. (2011). RIP3 mediates the embryonic lethality of caspase-8-deficient mice. Nature 471:7338. doi: 10.1038/nature09857
Kalia, M., and Sullivan, J. M. (1982). Brainstem projections of sensory and motor components of the vagus nerve in the rat. J. Comp. Neurol. 211, 248–265. doi: 10.1002/cne.902110304
Kang, R., Zeh, H. J., Lotze, M. T., and Tang, D. (2011). The Beclin 1 network regulates autophagy and apoptosis. Cell Death Differ. 18, 571–580. doi: 10.1038/cdd.2010.191
Kang, X., Ploner, A., Pedersen, N. L., Bandres-Ciga, S., Noyce, A. J., Wirdefeldt, K., et al. (2021). Tumor necrosis factor inhibition and Parkinson disease: A Mendelian randomization study. Neurology 96, e1672–e1679. doi: 10.1212/WNL.0000000000011630
Karunakaran, S., and Ravindranath, V. (2009). Activation of p38 MAPK in the Substantia nigra leads to nuclear translocation of NF-kappaB in MPTP-treated mice: Implication in Parkinson’s disease. J. Neurochem. 109, 1791–1799. doi: 10.1111/j.1471-4159.2009.06112.x
Karunakaran, S., Saeed, U., Mishra, M., Valli, R. K., Joshi, S. D., Meka, D. P., et al. (2008). Selective activation of p38 mitogen-activated protein kinase in dopaminergic neurons of Substantia nigra leads to nuclear translocation of p53 in 1-methyl-4-phenyl-1,2,3,6-tetrahydropyridine-treated mice. J. Neurosci. 28, 12500–12509. doi: 10.1523/JNEUROSCI.4511-08.2008
Kawamoto, Y., Ito, H., Ayaki, T., and Takahashi, R. (2014). Immunohistochemical localization of apoptosome-related proteins in Lewy bodies in Parkinson’s disease and dementia with Lewy bodies. Brain Res. 1571, 39–48. doi: 10.1016/j.brainres.2014.05.007
Kazmi, I., Al-Abbasi, F. A., Almalki, N., Sheikh, R. A., Al-Qahtani, S. D., Nadeem, M. S., et al. (2024). Malvidin attenuates behavioral and inhibits the TNF-α/Caspase-3/Nrf-2 expression in rotenone-induced Parkinson’s disease in rats: Insights from molecular docking. Eur. Rev. Med. Pharmacol. Sci. 28, 3330–3346. doi: 10.26355/eurrev_202405_36179
Ke, F. F. S., Vanyai, H. K., Cowan, A. D., Delbridge, A. R. D., Whitehead, L., Grabow, S., et al. (2018). Embryogenesis and adult life in the absence of intrinsic apoptosis effectors BAX, BAK, and BOK. Cell 173:1217–1230.e17. doi: 10.1016/j.cell.2018.04.036
Kihara, A., Kabeya, Y., Ohsumi, Y., and Yoshimori, T. (2001). Beclin–phosphatidylinositol 3-kinase complex functions at the trans-Golgi network. EMBO Rep. 2, 330–335. doi: 10.1093/embo-reports/kve061
Kim, D.-Y., Leem, Y.-H., Park, J.-S., Park, J.-E., Park, J.-M., Kang, J. L., et al. (2023). RIPK1 regulates microglial activation in lipopolysaccharide-induced neuroinflammation and MPTP-induced Parkinson’s disease mouse models. Cells 12:417. doi: 10.3390/cells12030417
Kim, S., Kwon, S.-H., Kam, T.-I., Panicker, N., Karuppagounder, S. S., Lee, S., et al. (2019). Transneuronal propagation of pathologic α-synuclein from the gut to the brain models Parkinson’s disease. Neuron 103:627-641.e7. doi: 10.1016/j.neuron.2019.05.035
Kitada, T., Asakawa, S., Hattori, N., Matsumine, H., Yamamura, Y., Minoshima, S., et al. (1998). Mutations in the parkin gene cause autosomal recessive juvenile Parkinsonism. Nature 392, 605–608. doi: 10.1038/33416
Klionsky, D. J. (2008). Autophagy revisited: A conversation with Christian de Duve. Autophagy 4, 740–743. doi: 10.4161/auto.6398
Konings, B., Villatoro, L., Van den Eynde, J., Barahona, G., Burns, R., McKnight, M., et al. (2023). Gastrointestinal syndromes preceding a diagnosis of Parkinson’s disease: Testing Braak’s hypothesis using a nationwide database for comparison with Alzheimer’s disease and cerebrovascular diseases. Gut 72, 2103–2111. doi: 10.1136/gutjnl-2023-329685
Koprich, J. B., Reske-Nielsen, C., Mithal, P., and Isacson, O. (2008). Neuroinflammation mediated by IL-1β increases susceptibility of dopamine neurons to degeneration in an animal model of Parkinson’s disease. J. Neuroinflamm. 5:8. doi: 10.1186/1742-2094-5-8
Kouli, A., Torsney, K. M., and Kuan, W.-L. (2018). “Parkinson’s disease: Etiology, neuropathology, and pathogenesis,” in Parkinson’s disease: Pathogenesis and clinical aspects, eds T. B. Stoker and J. C. Greenland (Singapore: Codon Publications).
Krebiehl, G., Ruckerbauer, S., Burbulla, L. F., Kieper, N., Maurer, B., Waak, J., et al. (2010). Reduced basal autophagy and impaired mitochondrial dynamics due to loss of Parkinson’s disease-associated protein DJ-1. PLoS One 5:e9367. doi: 10.1371/journal.pone.0009367
Kuida, K., Zheng, T. S., Na, S., Kuan, C., Yang, D., Karasuyama, H., et al. (1996). Decreased apoptosis in the brain and premature lethality in CPP32-deficient mice. Nature 384, 368–372. doi: 10.1038/384368a0
Lamark, T., Kirkin, V., Dikic, I., and Johansen, T. (2009). NBR1 and p62 as cargo receptors for selective autophagy of ubiquitinated targets. Cell Cycle 8, 1986–1990. doi: 10.4161/cc.8.13.8892
Laster, S. M., Wood, J. G., and Gooding, L. R. (1988). Tumor necrosis factor can induce both apoptic and necrotic forms of cell lysis. J. Immunol. 141, 2629–2634. doi: 10.4049/jimmunol.141.8.2629
Lee, W., Haslinger, A., Karin, M., and Tjian, R. (1987). Activation of transcription by two factors that bind promoter and enhancer sequences of the human metallothionein gene and SV40. Nature 325, 368–372. doi: 10.1038/325368a0
Leem, Y.-H., Kim, D.-Y., Park, J.-E., and Kim, H.-S. (2023). Necrosulfonamide exerts neuroprotective effect by inhibiting necroptosis, neuroinflammation, and α-synuclein oligomerization in a subacute MPTP mouse model of Parkinson’s disease. Sci. Rep. 13:8783. doi: 10.1038/s41598-023-35975-y
Leem, Y.-H., Park, J.-S., Park, J.-E., Kim, D.-Y., and Kim, H.-S. (2024). Creatine supplementation with exercise reduces α-synuclein oligomerization and necroptosis in Parkinson’s disease mouse model. J. Nutr. Biochem. 126:9586. doi: 10.1016/j.jnutbio.2024.109586
Lei, C., Zhongyan, Z., Wenting, S., Jing, Z., Liyun, Q., Hongyi, H., et al. (2023). Identification of necroptosis-related genes in Parkinson’s disease by integrated bioinformatics analysis and experimental validation. Front. Neurosci. 17:1097293. doi: 10.3389/fnins.2023.1097293
Levine, B., and Kroemer, G. (2008). Autophagy in the pathogenesis of disease. Cell 132:27. doi: 10.1016/j.cell.2007.12.018
Levitt, E. S., Abdala, A. P., Paton, J. F. R., Bissonnette, J. M., and Williams, J. T. (2015). μ opioid receptor activation hyperpolarizes respiratory-controlling Kölliker-Fuse neurons and suppresses post-inspiratory drive. J. Physiol. 593, 4453–4469. doi: 10.1113/JP270822
Li, R., Lu, Y., Zhang, Q., Liu, W., Yang, R., Jiao, J., et al. (2022). Piperine promotes autophagy flux by P2RX4 activation in SNCA/α-synuclein-induced Parkinson disease model. Autophagy 18, 559–575. doi: 10.1080/15548627.2021.1937897
Liang, X. H., Kleeman, L. K., Jiang, H. H., Gordon, G., Goldman, J. E., Berry, G., et al. (1998). Protection against fatal sindbis virus encephalitis by beclin, a novel Bcl-2-interacting protein. J. Virol. 72, 8586–8596. doi: 10.1128/JVI.72.11.8586-8596.1998
Lima, J. C., Oliveira, L. M., Botelho, M. T., Moreira, T. S., and Takakura, A. C. (2018). The involvement of the pathway connecting the substantia nigra, the periaqueductal gray matter and the retrotrapezoid nucleus in breathing control in a rat model of Parkinson’s disease. Exp. Neurol. 302, 46–56. doi: 10.1016/j.expneurol.2018.01.003
Lin, D., Zhang, H., Zhang, J., Huang, K., Chen, Y., Jing, X., et al. (2023). α-Synuclein induces neuroinflammation injury through the IL6ST-AS/STAT3/HIF-1α axis. Int. J. Mol. Sci. 24:1436. doi: 10.3390/ijms24021436
Lin, L., and Ghosh, S. (1996). A glycine-rich region in NF-kappaB p105 functions as a processing signal for the generation of the p50 subunit. Mol. Cell. Biol. 16, 2248–2254.
Lin, Y., Devin, A., Rodriguez, Y., and Liu, Z. G. (1999). Cleavage of the death domain kinase RIP by caspase-8 prompts TNF-induced apoptosis. Genes Dev. 13, 2514–2526. doi: 10.1101/gad.13.19.2514
Liu, J., Hu, H., and Wu, B. (2021). RIPK1 inhibitor ameliorates the MPP+/MPTP-induced Parkinson’s disease through the ASK1/JNK signalling pathway. Brain Res. 1757:147310. doi: 10.1016/j.brainres.2021.147310
Liu, J., Liu, W., Lu, Y., Tian, H., Duan, C., Lu, L., et al. (2018). Piperlongumine restores the balance of autophagy and apoptosis by increasing BCL2 phosphorylation in rotenone-induced Parkinson disease models. Autophagy 14, 845–861. doi: 10.1080/15548627.2017.1390636
Liu, S., Perez, P., Sun, X., Chen, K., Fatirkhorani, R., Mammadova, J., et al. (2024). MLKL polymerization-induced lysosomal membrane permeabilization promotes necroptosis. Cell Death Differ. 31:1. doi: 10.1038/s41418-023-01237-7
Liu, T., Zhang, L., Joo, D., and Sun, S.-C. (2017). NF-κB signaling in inflammation. Signal Transd. Target. Ther. 2:1. doi: 10.1038/sigtrans.2017.23
Liu, X., Zhang, Z., Ruan, J., Pan, Y., Magupalli, V. G., Wu, H., et al. (2016). Inflammasome-activated gasdermin D causes pyroptosis by forming membrane pores. Nature 535:7610. doi: 10.1038/nature18629
Loi, M., Raimondi, A., Morone, D., and Molinari, M. (2019). ESCRT-III-driven piecemeal micro-ER-phagy remodels the ER during recovery from ER stress. Nat. Commun. 10:5058. doi: 10.1038/s41467-019-12991-z
Lõrincz, P., Lakatos, Z., Maruzs, T., Szatmári, Z., Kis, V., and Sass, M. (2014). Atg6/UVRAG/Vps34-containing lipid kinase complex is required for receptor downregulation through endolysosomal degradation and epithelial polarity during Drosophila wing development. BioMed Res. Int. 2014:851349. doi: 10.1155/2014/851349
Lund, S., Porzgen, P., Mortensen, A. L., Hasseldam, H., Bozyczko-Coyne, D., Morath, S., et al. (2005). Inhibition of microglial inflammation by the MLK inhibitor CEP-1347. J. Neurochem. 92, 1439–1451. doi: 10.1111/j.1471-4159.2005.03014.x
MacFarlane, M., Ahmad, M., Srinivasula, S. M., Fernandes-Alnemri, T., Cohen, G. M., and Alnemri, E. S. (1997). Identification and molecular cloning of two novel receptors for the cytotoxic ligand TRAIL*. J. Biol. Chem. 272, 25417–25420. doi: 10.1074/jbc.272.41.25417
Maiuri, M. C., Le Toumelin, G., Criollo, A., Rain, J.-C., Gautier, F., Juin, P., et al. (2007). Functional and physical interaction between Bcl-XL and a BH3-like domain in Beclin-1. EMBO J. 26, 2527–2539. doi: 10.1038/sj.emboj.7601689
Martinon, F., Burns, K., and Tschopp, J. (2002). The inflammasome: A molecular platform triggering activation of inflammatory caspases and processing of proIL-β. Mol. Cell 10, 417–426. doi: 10.1016/S1097-2765(02)00599-3
Maycotte, P., Guemez-Gamboa, A., and Moran, J. (2010). Apoptosis and autophagy in rat cerebellar granule neuron death: Role of reactive oxygen species. J. Neurosci. Res. 88, 73–85. doi: 10.1002/jnr.22168
McIlwain, D. R., Berger, T., and Mak, T. W. (2013). Caspase functions in cell death and disease. Cold Spring Harb. Perspect. Biol. 5:a008656. doi: 10.1101/cshperspect.a008656
McLean, J. H., and Shipley, M. T. (1991). Postnatal development of the noradrenergic projection from locus coeruleus to the olfactory bulb in the rat. J. Comp. Neurol. 304, 467–477. doi: 10.1002/cne.903040310
McNamara, D. E., Dovey, C. M., Hale, A. T., Quarato, G., Grace, C. R., Guibao, C. D., et al. (2019). Direct activation of human MLKL by a select repertoire of inositol phosphate metabolites. Cell Chem. Biol. 26:863–877.e7. doi: 10.1016/j.chembiol.2019.03.010
Medema, J. P., Scaffidi, C., Kischkel, F. C., Shevchenko, A., Mann, M., Krammer, P. H., et al. (1997). FLICE is activated by association with the CD95 death-inducing signaling complex (DISC). EMBO J. 16, 2794–2804. doi: 10.1093/emboj/16.10.2794
Mendez, J. S., and Finn, B. W. (1975). Use of 6-hydroxydopamine to create lesions in catecholamine neurons in rats. J. Neurosurg. 42, 166–173. doi: 10.3171/jns.1975.42.2.0166
Meng, H.-W., Shen, Z.-B., Meng, X.-S., Leng-Wei, Yin, Z.-Q., Wang, X.-R., et al. (2023). Novel flavonoid 1,3,4-oxadiazole derivatives ameliorate MPTP-induced Parkinson’s disease via Nrf2/NF-κB signaling pathway. Bioorg. Chem. 138:106654. doi: 10.1016/j.bioorg.2023.106654
Meshkini, F., Moradi, A., and Hosseinkhani, S. (2023). Upregulation of RIPK1 implicates in HEK 293T cell death upon transient transfection of A53T-α-synuclein. Int. J. Biol. Macromol. 230:123216. doi: 10.1016/j.ijbiomac.2023.123216
Miki, Y., Shimoyama, S., Kon, T., Ueno, T., Hayakari, R., Tanji, K., et al. (2018). Alteration of autophagy-related proteins in peripheral blood mononuclear cells of patients with Parkinson’s disease. Neurobiol. Aging 63, 33–43. doi: 10.1016/j.neurobiolaging.2017.11.006
Mochizuki, H., Nakamura, N., Nishi, K., and Mizuno, Y. (1994). Apoptosis is induced by 1-methyl-4-phenylpyridinium ion (MPP+) in ventral mesencephalic-striatal co-culture in rat. Neurosci. Lett. 170, 191–194. doi: 10.1016/0304-3940(94)90271-2
Mogi, M., Harada, M., Riederer, P., Narabayashi, H., Fujita, K., and Nagatsu, T. (1994). Tumor necrosis factor-alpha (TNF-alpha) increases both in the brain and in the cerebrospinal fluid from Parkinsonian patients. Neurosci. Lett. 165, 208–210. doi: 10.1016/0304-3940(94)90746-3
Morris, J. L., Gillet, G., Prudent, J., and Popgeorgiev, N. (2021). Bcl-2 family of proteins in the control of mitochondrial calcium signalling: An old chap with new roles. Int. J. Mol. Sci. 22:3730. doi: 10.3390/ijms22073730
Nagata, S., Suzuki, J., Segawa, K., and Fujii, T. (2016). Exposure of phosphatidylserine on the cell surface. Cell Death Differ. 23:6. doi: 10.1038/cdd.2016.7
Naqvi, I., Giroux, N., Olson, L., Morrison, S. A., Llanga, T., Akinade, T. O., et al. (2022). DAMPs/PAMPs induce monocytic TLR activation and tolerance in COVID-19 patients; nucleic acid binding scavengers can counteract such TLR agonists. Biomaterials 283:121393. doi: 10.1016/j.biomaterials.2022.121393
Nascimento, A. L. F., Medeiros, P. O. S., Pedrão, L. F. A. T., Queiroz, V. C., Oliveira, L. M., Novaes, L. S., et al. (2022). Oxidative stress inhibition via apocynin prevents medullary respiratory neurodegeneration and respiratory pattern dysfunction in a 6-hydroxydopamine animal model of Parkinson’s disease. Neuroscience 502, 91–106. doi: 10.1016/j.neuroscience.2022.07.034
Noguchi, T., Ishii, K., Fukutomi, H., Naguro, I., Matsuzawa, A., Takeda, K., et al. (2008). Requirement of reactive oxygen species-dependent activation of ASK1-p38 MAPK pathway for extracellular ATP-induced apoptosis in macrophage. J. Biol. Chem. 283, 7657–7665. doi: 10.1074/jbc.M708402200
Nyström, S., Antoine, D. J., Lundbäck, P., Lock, J. G., Nita, A. F., Högstrand, K., et al. (2013). TLR activation regulates damage-associated molecular pattern isoforms released during pyroptosis. EMBO J. 32, 86–99. doi: 10.1038/emboj.2012.328
Oberst, A., Dillon, C. P., Weinlich, R., McCormick, L. L., Fitzgerald, P., Pop, C., et al. (2011). Catalytic activity of the caspase-8–FLIPL complex inhibits RIPK3-dependent necrosis. Nature 471:7338. doi: 10.1038/nature09852
Ojha, R., Jha, V., and Singh, S. K. (2016). Gemcitabine and mitomycin induced autophagy regulates cancer stem cell pool in urothelial carcinoma cells. Biochim. Biophys. Acta 1863, 347–359. doi: 10.1016/j.bbamcr.2015.12.002
Oliveira, L. M., Oliveira, M. A., Moriya, H. T., Moreira, T. S., and Takakura, A. C. (2019). Respiratory disturbances in a mouse model of Parkinson’s disease. Exp. Physiol. 104, 729–739. doi: 10.1113/EP087507
Oñate, M., Catenaccio, A., Salvadores, N., Saquel, C., Martinez, A., Moreno-Gonzalez, I., et al. (2020). The necroptosis machinery mediates axonal degeneration in a model of Parkinson disease. Cell Death Differ. 27:4. doi: 10.1038/s41418-019-0408-4
Onyango, I. G., Tuttle, J. B., and Bennett, J. P. (2005). Activation of p38 and N-acetylcysteine-sensitive c-Jun NH2-terminal kinase signaling cascades is required for induction of apoptosis in Parkinson’s disease cybrids. Mol. Cell. Neurosci. 28, 452–461. doi: 10.1016/j.mcn.2004.10.006
Ott, M. M., Nuding, S. C., Segers, L. S., O’Connor, R., Morris, K. F., and Lindsey, B. G. (2012). Central chemoreceptor modulation of breathing via multipath tuning in medullary ventrolateral respiratory column circuits. J. Neurophysiol. 107, 603–617. doi: 10.1152/jn.00808.2011
Ouyang, M., and Shen, X. (2006). Critical role of ASK1 in the 6-hydroxydopamine-induced apoptosis in human neuroblastoma SH-SY5Y cells. J. Neurochem. 97, 234–244. doi: 10.1111/j.1471-4159.2006.03730.x
Ozato, K., Tsujimura, H., and Tamura, T. (2002). Toll-like receptor signaling and regulation of cytokine gene expression in the immune system. BioTechniques 70, 66–68.
Pagano, G., Ferrara, N., Brooks, D. J., and Pavese, N. (2016). Age at onset and Parkinson disease phenotype. Neurology 86, 1400–1407. doi: 10.1212/WNL.0000000000002461
Pan, J., Chang, Q., Wang, X., Son, Y., Zhang, Z., Chen, G., et al. (2010). Reactive oxygen species-activated Akt/ASK1/p38 signaling pathway in nickel compound-induced apoptosis in BEAS 2B cells. Chem. Res. Toxicol. 23, 568–577. doi: 10.1021/tx9003193
Pan, P.-Y., Li, X., Wang, J., Powell, J., Wang, Q., Zhang, Y., et al. (2017). Parkinson’s disease-associated LRRK2 hyperactive kinase mutant disrupts synaptic vesicle trafficking in ventral midbrain neurons. J. Neurosci. 37, 11366–11376. doi: 10.1523/JNEUROSCI.0964-17.2017
Panicker, N., Kam, T.-I., Wang, H., Neifert, S., Chou, S.-C., Kumar, M., et al. (2022). Neuronal NLRP3 is a parkin substrate that drives neurodegeneration in Parkinson’s disease. Neuron 110:2422–2437.e9. doi: 10.1016/j.neuron.2022.05.009
Pankiv, S., Clausen, T. H., Lamark, T., Brech, A., Bruun, J.-A., Outzen, H., et al. (2007). P62/SQSTM1 binds directly to Atg8/LC3 to facilitate degradation of ubiquitinated protein aggregates by autophagy. J. Biol. Chem. 282, 24131–24145. doi: 10.1074/jbc.M702824200
Parrish, A. B., Freel, C. D., and Kornbluth, S. (2013). Cellular mechanisms controlling caspase activation and function. Cold Spring Harb. Perspect. Biol. 5:a008672. doi: 10.1101/cshperspect.a008672
Parzych, K. R., and Klionsky, D. J. (2014). An overview of autophagy: Morphology, mechanism, and regulation. Antioxid. Redox Signal. 20, 460–473. doi: 10.1089/ars.2013.5371
Pattingre, S., Tassa, A., Qu, X., Garuti, R., Liang, X. H., Mizushima, N., et al. (2005). Bcl-2 antiapoptotic proteins inhibit Beclin 1-dependent autophagy. Cell 122, 927–939. doi: 10.1016/j.cell.2005.07.002
Pemberton, J. M., Pogmore, J. P., and Andrews, D. W. (2021). Neuronal cell life, death, and axonal degeneration as regulated by the BCL-2 family proteins. Cell Death Differ. 28, 108–122. doi: 10.1038/s41418-020-00654-2
Perese, D. A., Ulman, J., Viola, J., Ewing, S. E., and Bankiewicz, K. S. (1989). A 6-hydroxydopamine-induced selective Parkinsonian rat model. Brain Res. 494, 285–293. doi: 10.1016/0006-8993(89)90597-0
Perfettini, J.-L., Castedo, M., Nardacci, R., Ciccosanti, F., Boya, P., Roumier, T., et al. (2005). Essential role of p53 phosphorylation by p38 MAPK in apoptosis induction by the HIV-1 envelope. J. Exp. Med. 201, 279–289. doi: 10.1084/jem.20041502
Pétrilli, V., Dostert, C., Muruve, D. A., and Tschopp, J. (2007). The inflammasome: A danger sensing complex triggering innate immunity. Curr. Opin. Immunol. 19, 615–622. doi: 10.1016/j.coi.2007.09.002
Phelan, S. A., Ito, M., and Loeken, M. R. (1997). Neural tube defects in embryos of diabetic mice: Role of the Pax-3 gene and apoptosis. Diabetes 46, 1189–1197. doi: 10.2337/diab.46.7.1189
Piri, H., Sharifi, S., Nigjeh, S., and Haghdoost-Yazdi, H. (2022). Dopaminergic neuronal death in the substantia nigra associates with change in serum levels of TNF-α and IL-1β; evidence from early experimental model of Parkinson’s disease. Neurol. Res. 44, 544–553. doi: 10.1080/01616412.2021.2024726
Poewe, W., Seppi, K., Tanner, C. M., Halliday, G. M., Brundin, P., Volkmann, J., et al. (2017). Parkinson disease. Nat. Rev. Dis. Prim. 3, 1–21. doi: 10.1038/nrdp.2017.13
Polster, B. M., Mark, K. A., Arze, R., and Hudson, D. (2022). Calpain-independent intracellular protease activity is elevated in excitotoxic cortical neurons prior to delayed calcium deregulation and mitochondrial dysfunction. Biomolecules 12:1004. doi: 10.3390/biom12071004
Polymeropoulos, M. H., Lavedan, C., Leroy, E., Ide, S. E., Dehejia, A., Dutra, A., et al. (1997). Mutation in the alpha-synuclein gene identified in families with Parkinson’s disease. Science 276, 2045–2047. doi: 10.1126/science.276.5321.2045
Qin, Y., Qiu, J., Wang, P., Liu, J., Zhao, Y., Jiang, F., et al. (2021). Impaired autophagy in microglia aggravates dopaminergic neurodegeneration by regulating NLRP3 inflammasome activation in experimental models of Parkinson’s disease. Brain Behav. Immun. 91, 324–338. doi: 10.1016/j.bbi.2020.10.010
Qiu, Z., Lei, S., Zhao, B., Wu, Y., Su, W., Liu, M., et al. (2017). NLRP3 inflammasome activation-mediated pyroptosis aggravates myocardial ischemia/reperfusion injury in diabetic rats. Oxid. Med. Cell. Longev. 2017:9743280. doi: 10.1155/2017/9743280
Qu, L., Lin, B., Zeng, W., Fan, C., Wu, H., Ge, Y., et al. (2022). Lysosomal K+ channel TMEM175 promotes apoptosis and aggravates symptoms of Parkinson’s disease. EMBO Rep. 23:e53234. doi: 10.15252/embr.202153234
Quan, W., Liu, Y., Li, J., Chen, D., Xu, J., Song, J., et al. (2024). Investigating the TLR4/TAK1/IRF7 axis in NLRP3-mediated pyroptosis in Parkinson’s disease. Inflammation 47, 404–420. doi: 10.1007/s10753-023-01918-y
Que, R., Zheng, J., Chang, Z., Zhang, W., Li, H., Xie, Z., et al. (2021). Dl-3-n-butylphthalide rescues dopaminergic neurons in Parkinson’s disease models by inhibiting the NLRP3 inflammasome and ameliorating mitochondrial impairment. Front. Immunol. 12:794770. doi: 10.3389/fimmu.2021.794770
Ramiro-Cortés, Y., and Morán, J. (2009). Role of oxidative stress and JNK pathway in apoptotic death induced by potassium deprivation and staurosporine in cerebellar granule neurons. Neurochem. Int. 55, 581–592. doi: 10.1016/j.neuint.2009.05.015
Reggiori, F., Shintani, T., Nair, U., and Klionsky, D. J. (2005). Atg9 cycles between mitochondria and the pre-autophagosomal structure in yeasts. Autophagy 1, 101–109.
Reidick, C., Boutouja, F., and Platta, H. W. (2017). The class III phosphatidylinositol 3-kinase Vps34 in Saccharomyces cerevisiae. Biol. Chem. 398, 677–685. doi: 10.1515/hsz-2016-0288
Rekha, K. R., and Selvakumar, G. P. (2014). Gene expression regulation of Bcl2, Bax and cytochrome-C by geraniol on chronic MPTP/probenecid induced C57BL/6 mice model of Parkinson’s disease. Chem. Biol. Interact. 217, 57–66. doi: 10.1016/j.cbi.2014.04.010
Rickert, R. C., Jellusova, J., and Miletic, A. V. (2011). Signaling by the TNFR superfamily in B-cell biology and disease. Immunol. Rev. 244, 115–133. doi: 10.1111/j.1600-065X.2011.01067.x
Rietdijk, C. D., Perez-Pardo, P., Garssen, J., van Wezel, R. J. A., and Kraneveld, A. D. (2017). Exploring Braak’s hypothesis of Parkinson’s disease. Front. Neurol. 8:37. doi: 10.3389/fneur.2017.00037
Rodriguez, A. E., Bogart, C., Gilbert, C. M., McCullers, J. A., Smith, A. M., Kanneganti, T.-D., et al. (2019). Enhanced IL-1β production is mediated by a TLR2-MYD88-NLRP3 signaling axis during coinfection with influenza A virus and Streptococcus pneumoniae. PLoS One 14:e0212236. doi: 10.1371/journal.pone.0212236
Rogaeva, E., Johnson, J., Lang, A. E., Gulick, C., Gwinn-Hardy, K., Kawarai, T., et al. (2004). Analysis of the PINK1 gene in a large cohort of cases with Parkinson disease. Arch. Neurol. 61, 1898–1904. doi: 10.1001/archneur.61.12.1898
Rogers, R. C., Kita, H., Butcher, L. L., and Novin, D. (1980). Afferent projections to the dorsal motor nucleus of the vagus. Brain Res. Bull. 5, 365–373. doi: 10.1016/s0361-9230(80)80006-2
Ross, O. A., Soto-Ortolaza, A. I., Heckman, M. G., Aasly, J. O., Abahuni, N., Annesi, G., et al. (2011). Association of LRRK2 exonic variants with susceptibility to Parkinson’s disease: A case-control study. Lancet Neurol. 10, 898–908. doi: 10.1016/S1474-4422(11)70175-2
Roy, T., Chatterjee, A., and Swarnakar, S. (2023). Rotenone induced neurodegeneration is mediated via cytoskeleton degradation and necroptosis. Biochim. Biophys. Acta 1870:119417. doi: 10.1016/j.bbamcr.2022.119417
Samson, A. L., Zhang, Y., Geoghegan, N. D., Gavin, X. J., Davies, K. A., Mlodzianoski, M. J., et al. (2020). MLKL trafficking and accumulation at the plasma membrane control the kinetics and threshold for necroptosis. Nat. Commun. 11:1. doi: 10.1038/s41467-020-16887-1
Sarkar, A., Shamsuzzama, Kumar, L., Hameed, R., and Nazir, A. (2022). Multiple checkpoints of protein clearance machinery are modulated by a common microRNA, miR-4813-3p, through its putative target genes: Studies employing transgenic C. elegans model. Bioch. Biophys. Acta Mol. Cell Res. 1869:119342. doi: 10.1016/j.bbamcr.2022.119342
Shao, W., Yeretssian, G., Doiron, K., Hussain, S. N., and Saleh, M. (2007). The caspase-1 digestome identifies the glycolysis pathway as a target during infection and septic shock. J. Biol. Chem. 282, 36321–36329. doi: 10.1074/jbc.M708182200
Shipley, M. T., Halloran, F. J., and de la Torre, J. (1985). Surprisingly rich projection from locus coeruleus to the olfactory bulb in the rat. Brain Res. 329, 294–299. doi: 10.1016/0006-8993(85)90537-2
Sian-Hülsmann, J., Mandel, S., Youdim, M. B. H., and Riederer, P. (2011). The relevance of iron in the pathogenesis of Parkinson’s disease. J. Neurochem. 118, 939–957. doi: 10.1111/j.1471-4159.2010.07132.x
Silva, J. N., Oliveira, L. M., Souza, F. C., Moreira, T. S., and Takakura, A. C. (2019). Distinct pathways to the parafacial respiratory group to trigger active expiration in adult rats. Am. J. Physiol. 317, L402–L413. doi: 10.1152/ajplung.00467.2018
Simon, D. K., Tanner, C. M., and Brundin, P. (2020). Parkinson disease epidemiology, pathology, genetics and pathophysiology. Clin. Geriatr. Med. 36, 1–12. doi: 10.1016/j.cger.2019.08.002
Singh, A., Kukreti, R., Saso, L., and Kukreti, S. (2019). Oxidative stress: A key modulator in neurodegenerative diseases. Molecules 24:1583. doi: 10.3390/molecules24081583
Smith, J. C., Ellenberger, H. H., Ballanyi, K., Richter, D. W., and Feldman, J. L. (1991). Pre-Bötzinger complex: A brainstem region that may generate respiratory rhythm in mammals. Science 254, 726–729. doi: 10.1126/science.1683005
Söllner, T., Whiteheart, S. W., Brunner, M., Erdjument-Bromage, H., Geromanos, S., Tempst, P., et al. (1993). SNAP receptors implicated in vesicle targeting and fusion. Nature 362, 318–324. doi: 10.1038/362318a0
Soman, S. K., Bazała, M., Keatinge, M., Bandmann, O., and Kuznicki, J. (2019). Restriction of mitochondrial calcium overload by mcu inactivation renders a neuroprotective effect in zebrafish models of Parkinson’s disease. Biol. Open 8:bio044347. doi: 10.1242/bio.044347
Song, J. J., and Lee, Y. J. (2007). Differential activation of the JNK signal pathway by UV irradiation and glucose deprivation. Cell. Signal. 19, 563–572. doi: 10.1016/j.cellsig.2006.08.016
Song, G., Wang, H., Xu, H., and Poon, C.-S. (2012). Kölliker–Fuse neurons send collateral projections to multiple hypoxia-activated and nonactivated structures in rat brainstem and spinal cord. Brain Struct. Funct. 217, 835–858. doi: 10.1007/s00429-012-0384-7
Sophoronea, T., Agrawal, S., Kumari, N., Mishra, J., Walecha, V., and Luthra, P. M. (2024). A2AR antagonists triggered the AMPK/m-TOR autophagic pathway to reverse the calcium-dependent cell damage in 6-OHDA induced model of PD. Neurochem. Int. 178:105793. doi: 10.1016/j.neuint.2024.105793
Srikrishna, G., and Freeze, H. H. (2009). Endogenous damage-associated molecular pattern molecules at the crossroads of inflammation and cancer. Neoplasia (New York, N.Y.) 11, 615–628.
Sriram, K., Matheson, J. M., Benkovic, S. A., Miller, D. B., Luster, M. I., and O’Callaghan, J. P. (2002). Mice deficient in TNF receptors are protected against dopaminergic neurotoxicity: Implications for Parkinson’s disease. FASEB J. 16, 1474–1476. doi: 10.1096/fj.02-0216fje
Subramaniam, S. R., and Chesselet, M.-F. (2013). Mitochondrial dysfunction and oxidative stress in Parkinson’s disease. Prog. Neurobiol. 10, 17–32. doi: 10.1016/j.pneurobio.2013.04.004
Sun, L., Wang, H., Wang, Z., He, S., Chen, S., Liao, D., et al. (2012). Mixed lineage kinase domain-like protein mediates necrosis signaling downstream of RIP3 kinase. Cell 148, 213–227. doi: 10.1016/j.cell.2011.11.031
Sziráki, I., Mohanakumar, K. P., Rauhala, P., Kim, H. G., Yeh, K. J., and Chiueh, C. C. (1998). Manganese: A transition metal protects nigrostriatal neurons from oxidative stress in the iron-induced animal model of Parkinsonism. Neuroscience 85, 1101–1111. doi: 10.1016/S0306-4522(97)00660-X
Takakura, A. C. T., Moreira, T. S., Colombari, E., West, G. H., Stornetta, R. L., and Guyenet, P. G. (2006). Peripheral chemoreceptor inputs to retrotrapezoid nucleus (RTN) CO2-sensitive neurons in rats. J. Physiol. 572, 503–523. doi: 10.1113/jphysiol.2005.103788
Takeshige, K., Baba, M., Tsuboi, S., Noda, T., and Ohsumi, Y. (1992). Autophagy in yeast demonstrated with proteinase-deficient mutants and conditions for its induction. J. Cell Biol. 119, 301–311. doi: 10.1083/jcb.119.2.301
Tamura, H., Sasaki, M., Nakajima, S., Nishio, R., Saeki, N., Katahira, M., et al. (2023). Reactive oxygen species produced by Zn2+ influx after exposure to AMPA, but not NMDA and their capturing effect on nigral dopaminergic protection. NeuroToxicology 95, 173–180. doi: 10.1016/j.neuro.2023.02.003
Tansey, M. G., Wallings, R. L., Houser, M. C., Herrick, M. K., Keating, C. E., and Joers, V. (2022). Inflammation and immune dysfunction in Parkinson disease. Nat. Rev. Immunol. 22, 657–673. doi: 10.1038/s41577-022-00684-6
Tatton, N. A. (2000). Increased Caspase 3 and Bax immunoreactivity accompany nuclear GAPDH translocation and neuronal apoptosis in Parkinson’s disease. Exp. Neurol. 166, 29–43. doi: 10.1006/exnr.2000.7489
Thapa, R. J., Nogusa, S., Chen, P., Maki, J. L., Lerro, A., Andrake, M., et al. (2013). Interferon-induced RIP1/RIP3-mediated necrosis requires PKR and is licensed by FADD and caspases. Proc. Natl. Acad. Sci. U.S.A. 110, E3109–E3118. doi: 10.1073/pnas.1301218110
Thiffault, C., Langston, J. W., and Di Monte, D. A. (2000). Increased striatal dopamine turnover following acute administration of rotenone to mice. Brain Res. 885, 283–288. doi: 10.1016/s0006-8993(00)02960-7
Thorburn, J., Andrysik, Z., Staskiewicz, L., Gump, J., Maycotte, P., Oberst, A., et al. (2014). Autophagy controls the kinetics and extent of mitochondrial apoptosis by regulating PUMA levels. Cell Rep. 7, 45–52. doi: 10.1016/j.celrep.2014.02.036
Thornberry, N. A., Bull, H. G., Calaycay, J. R., Chapman, K. T., Howard, A. D., Kostura, M. J., et al. (1992). A novel heterodimeric cysteine protease is required for interleukin-1 beta processing in monocytes. Nature 356, 768–774. doi: 10.1038/356768a0
Tran, S., Fairlie, W. D., and Lee, E. F. (2021). BECLIN1: Protein structure, function and regulation. Cells 10:1522. doi: 10.3390/cells10061522
Tuppy, M., Barna, B. F., Alves-dos-Santos, L., Britto, L. R. G., Chiavegatto, S., Moreira, T. S., et al. (2015). Respiratory deficits in a rat model of Parkinson’s disease. Neuroscience 297, 194–204. doi: 10.1016/j.neuroscience.2015.03.048
Tysnes, O.-B., and Storstein, A. (2017). Epidemiology of Parkinson’s disease. J. Neural Transm. 124, 901–905. doi: 10.1007/s00702-017-1686-y
Vann, N. C., Pham, F. D., Dorst, K. E., and Del Negro, C. A. (2018). Dbx1 Pre-Bötzinger complex interneurons comprise the core inspiratory oscillator for breathing in unanesthetized adult mice. eNeuro 5, doi: 10.1523/ENEURO.0130-18.2018
Viswanath, V., Wu, Y., Boonplueang, R., Chen, S., Stevenson, F. F., Yantiri, F., et al. (2001). Caspase-9 activation results in downstream caspase-8 activation and bid cleavage in 1-methyl-4-phenyl-1,2,3,6-tetrahydropyridine-induced Parkinson’s disease. J. Neurosci. 21, 9519–9528. doi: 10.1523/JNEUROSCI.21-24-09519.2001
Voss, A. K., and Strasser, A. (2020). The essentials of developmental apoptosis. F1000Research 9:148. doi: 10.12688/f1000research.21571.1
Walkinshaw, G., and Waters, C. M. (1994). Neurotoxin-induced cell death in neuronal PC12 cells is mediated by induction of apoptosis. Neuroscience 63, 975–987. doi: 10.1016/0306-4522(94)90566-5
Wang, L., Wu, X., Yang, G., Hu, N., Zhao, Z., Zhao, L., et al. (2022). Cannabidiol alleviates the damage to dopaminergic neurons in 1-methyl-4-Phenyl-1,2,3,6-tetrahydropyridine-induced parkinson’s disease mice via regulating neuronal apoptosis and neuroinflammation. Neuroscience 498, 64–72. doi: 10.1016/j.neuroscience.2022.06.036
Wang, Y., Wu, S., Li, Q., Lang, W., Li, W., Jiang, X., et al. (2022). Salsolinol induces Parkinson’s disease through activating NLRP3-dependent pyroptosis and the neuroprotective effect of acteoside. Neurotox. Res. 40, 1948–1962. doi: 10.1007/s12640-022-00608-1
Wang, Y., Li, C., Zhang, X., Kang, X., Li, Y., Zhang, W., et al. (2021). Exposure to PM2.5 aggravates Parkinson’s disease via inhibition of autophagy and mitophagy pathway. Toxicology 456:152770. doi: 10.1016/j.tox.2021.152770
Weber, K., Roelandt, R., Bruggeman, I., Estornes, Y., and Vandenabeele, P. (2018). Nuclear RIPK3 and MLKL contribute to cytosolic necrosome formation and necroptosis. Commun. Biol. 1:1. doi: 10.1038/s42003-017-0007-1
Williams, R. A. M., Woods, K. L., Juliano, L., Mottram, J. C., and Coombs, G. H. (2009). Characterisation of unusual families of ATG8-like proteins and ATG12 in the protozoan parasite Leishmania major. Autophagy 5, 159–172. doi: 10.4161/auto.5.2.7328
Willis, A. W., Roberts, E., Beck, J. C., Fiske, B., Ross, W., Savica, R., et al. (2022). Incidence of Parkinson disease in North America. NPJ Parkinsons Dis. 8:1. doi: 10.1038/s41531-022-00410-y
Wilms, H., Rosenstiel, P., Sievers, J., Deuschl, G., Zecca, L., and Lucius, R. (2003). Activation of microglia by human neuromelanin is NF-kappaB dependent and involves p38 mitogen-activated protein kinase: Implications for Parkinson’s disease. FASEB J. 17, 500–502. doi: 10.1096/fj.02-0314fje
Wilson, M. D., Barbosa-Morais, N. L., Schmidt, D., Conboy, C. M., Vanes, L., Tybulewicz, V. L. J., et al. (2008). Species-specific transcription in mice carrying human chromosome 21. Science 322, 434–438. doi: 10.1126/science.1160930
Wu, J., Wang, J., Zhou, S., Yang, L., Yin, J., Cao, J., et al. (2015). Necrostatin-1 protection of dopaminergic neurons. Neural Regener. Res. 10, 1120–1124. doi: 10.4103/1673-5374.160108
Wurzer, B., Zaffagnini, G., Fracchiolla, D., Turco, E., Abert, C., Romanov, J., et al. (2015). Oligomerization of p62 allows for selection of ubiquitinated cargo and isolation membrane during selective autophagy. eLife 4:e08941. doi: 10.7554/eLife.08941
Xing, R., Liu, X., Tian, B., Cheng, Y., and Li, L. (2021). Neuroprotective effect of Na + /H + exchangers isoform-1 inactivation against 6-hydroxydopamine-induced mitochondrial dysfunction and neuronal apoptosis in Parkinson’s disease models. Drug Dev. Res. 82, 969–979. doi: 10.1002/ddr.21799
Xu, Z., Maroney, A. C., Dobrzanski, P., Kukekov, N. V., and Greene, L. A. (2001). The MLK family mediates c-Jun N-terminal kinase activation in neuronal apoptosis. Mol. Cell. Biol. 21, 4713–4724. doi: 10.1128/MCB.21.14.4713-4724.2001
Yamada, A., Arakaki, R., Saito, M., Kudo, Y., and Ishimaru, N. (2017). Dual role of Fas/FasL-mediated signal in peripheral immune tolerance. Front. Immunol. 8:403. doi: 10.3389/fimmu.2017.00403
Yamada, M., Kida, K., Amutuhaire, W., Ichinose, F., and Kaneki, M. (2010). Gene disruption of caspase-3 prevents MPTP-induced Parkinson’s disease in mice. Biochem. Biophys. Res. Commun. 402, 312–318. doi: 10.1016/j.bbrc.2010.10.023
Yamada, T., Egashira, N., Bando, A., Nishime, Y., Tonogai, Y., Imuta, M., et al. (2012). Activation of p38 MAPK by oxidative stress underlying epirubicin-induced vascular endothelial cell injury. Free Radic. Biol. Med. 52, 1285–1293. doi: 10.1016/j.freeradbiomed.2012.02.003
Yamada, T., Mitani, T., Yorita, K., Uchida, D., Matsushima, A., Iwamasa, K., et al. (2000). Abnormal immune function of hemopoietic cells from alymphoplasia (aly) mice, a natural strain with mutant NF-kappa B-inducing kinase. J. Immunol. 165, 804–812. doi: 10.4049/jimmunol.165.2.804
Yamaguchi, Y., and Miura, M. (2015). Programmed cell death and caspase functions during neural development. Curr. Top. Dev. Biol. 114, 159–184. doi: 10.1016/bs.ctdb.2015.07.016
Yamamoto, H., Zhang, S., and Mizushima, N. (2023). Autophagy genes in biology and disease. Nat. Rev. Genet. 24, 382–400. doi: 10.1038/s41576-022-00562-w
Yamamoto, M., Sato, S., Mori, K., Hoshino, K., Takeuchi, O., Takeda, K., et al. (2002). Cutting edge: A novel Toll/IL-1 receptor domain-containing adapter that preferentially activates the IFN-β promoter in the toll-like receptor signaling1. J. Immunol. 169, 6668–6672. doi: 10.4049/jimmunol.169.12.6668
Yan, M. H., Wang, X., and Zhu, X. (2013). Mitochondrial defects and oxidative stress in Alzheimer disease and Parkinson disease. Free Radic. Biol. Med. 62, 90–101. doi: 10.1016/j.freeradbiomed.2012.11.014
Yan, X., Liu, D.-F., Zhang, X.-Y., Liu, D., Xu, S.-Y., Chen, G.-X., et al. (2017). Vanillin protects dopaminergic neurons against inflammation-mediated cell death by inhibiting ERK1/2, P38 and the NF-κB signaling pathway. Int. J. Mol. Sci. 18:389. doi: 10.3390/ijms18020389
Yan, Y., Jiang, W., Liu, L., Wang, X., Ding, C., Tian, Z., et al. (2015). Dopamine controls systemic inflammation through inhibition of NLRP3 inflammasome. Cell 160, 62–73. doi: 10.1016/j.cell.2014.11.047
Yang, C. F., Kim, E. J., Callaway, E. M., and Feldman, J. L. (2020). Monosynaptic projections to excitatory and inhibitory preBötzinger complex neurons. Front. Neuroanat. 14:58. doi: 10.3389/fnana.2020.00058
Yang, X., Zhang, M., Wei, M., Wang, A., Deng, Y., and Cao, H. (2020). MicroRNA-216a inhibits neuronal apoptosis in a cellular Parkinson’s disease model by targeting Bax. Metab. Brain Dis. 35, 627–635. doi: 10.1007/s11011-020-00546-x
Yang, H., Li, L., Jiao, Y., Zhang, Y., Wang, Y., Zhu, K., et al. (2021). Thioredoxin-1 mediates neuroprotection of Schisanhenol against MPP+-induced apoptosis via suppression of ASK1-P38-NF-κB pathway in SH-SY5Y cells. Sci. Rep. 11:21604. doi: 10.1038/s41598-021-01000-3
Yang, Y., Wang, H., Kouadir, M., Song, H., and Shi, F. (2019). Recent advances in the mechanisms of NLRP3 inflammasome activation and its inhibitors. Cell Death Dis. 10, 1–11. doi: 10.1038/s41419-019-1413-8
Yanumula, A., and Cusick, J. K. (2023). Biochemistry, Extrinsic pathway of apoptosis: StatPearls. Treasure Island, FL: StatPearls Publishing.
Yen, W.-L., Legakis, J. E., Nair, U., and Klionsky, D. J. (2007). Atg27 is required for autophagy-dependent cycling of Atg9. Mol. Biol. Cell 18, 581–593. doi: 10.1091/mbc.e06-07-0612
Yi, J., and Tang, X. M. (1999). The convergent point of the endocytic and autophagic pathways in leydig cells. Cell Res. 9:4. doi: 10.1038/sj.cr.7290023
Yildirim-Balatan, C., Fenyi, A., Besnault, P., Gomez, L., Sepulveda-Diaz, J. E., Michel, P. P., et al. (2024). Parkinson’s disease-derived α-synuclein assemblies combined with chronic-type inflammatory cues promote a neurotoxic microglial phenotype. J. Neuroinflamm. 21:54. doi: 10.1186/s12974-024-03043-5
Yoon, S., Park, S. J., Han, J. H., Kang, J. H., Kim, J., Lee, J., et al. (2014). Caspase-dependent cell death-associated release of nucleosome and damage-associated molecular patterns. Cell Death Dis. 5:e1494. doi: 10.1038/cddis.2014.450
Yoshida, H., Kong, Y. Y., Yoshida, R., Elia, A. J., Hakem, A., Hakem, R., et al. (1998). Apaf1 is required for mitochondrial pathways of apoptosis and brain development. Cell 94, 739–750. doi: 10.1016/s0092-8674(00)81733-x
Zhang, C., Zhao, M., Wang, B., Su, Z., Guo, B., Qin, L., et al. (2021). The Nrf2-NLRP3-caspase-1 axis mediates the neuroprotective effects of Celastrol in Parkinson’s disease. Redox Biol. 47:102134. doi: 10.1016/j.redox.2021.102134
Zhang, H., Zhou, X., McQuade, T., Li, J., Chan, F. K.-M., and Zhang, J. (2011). Functional complementation between FADD and RIP1 in embryos and lymphocytes. Nature 471:7338. doi: 10.1038/nature09878
Zhang, M., He, Q., Chen, G., and Li, P. A. (2020). Suppression of nlrp3 inflammasome, pyroptosis, and cell death by NIM811 in rotenone-exposed cells as an in vitro model of Parkinson’s disease. Neuro Degener. Dis. 20, 73–83. doi: 10.1159/000511207
Zhang, X., Zhang, Y., Li, R., Zhu, L., Fu, B., and Yan, T. (2020). Salidroside ameliorates Parkinson’s disease by inhibiting NLRP3-dependent pyroptosis. Aging 12, 9405–9426. doi: 10.18632/aging.103215
Zhang, S., Yazaki, E., Sakamoto, H., Yamamoto, H., and Mizushima, N. (2022). Evolutionary diversification of the autophagy-related ubiquitin-like conjugation systems. Autophagy 18, 2969–2984. doi: 10.1080/15548627.2022.2059168
Zhang, Y., Wu, Q., Zhang, L., Wang, Q., Yang, Z., Liu, J., et al. (2019). Caffeic acid reduces A53T α-synuclein by activating JNK/Bcl-2-mediated autophagy in vitro and improves behaviour and protects dopaminergic neurons in a mouse model of Parkinson’s disease. Pharmacol. Res. 150:104538. doi: 10.1016/j.phrs.2019.104538
Zhao, J., Yang, M., Li, Q., Pei, X., and Zhu, X. (2020). miR-132-5p regulates apoptosis and autophagy in MPTP model of Parkinson’s disease by targeting ULK1. Neuroreport 31, 959–965. doi: 10.1097/WNR.0000000000001494
Zheng, C., Chen, J., Chu, F., Zhu, J., and Jin, T. (2019). Inflammatory Role of TLR-MyD88 Signaling in Multiple Sclerosis. Front. Mol. Neurosci. 12:314. doi: 10.3389/fnmol.2019.00314
Zheng, R., Yan, Y., Dai, S., Ruan, Y., Chen, Y., Hu, C., et al. (2023). ASC specks exacerbate α synuclein pathology via amplifying NLRP3 inflammasome activities. J. Neuroinflamm. 20:26. doi: 10.1186/s12974-023-02709-w
Zhong, Y., Cai, X., Ding, L., Liao, J., Liu, X., Huang, Y., et al. (2022). Nrf2 inhibits the progression of Parkinson’s disease by upregulating AABR07032261.5 to repress pyroptosis. J. Inflamm. Res. 15, 669–685. doi: 10.2147/JIR.S345895
Zhou, X., Hollern, D., Liao, J., Andrechek, E., and Wang, H. (2013). NMDA receptor-mediated excitotoxicity depends on the coactivation of synaptic and extrasynaptic receptors. Cell Death Dis. 4:3. doi: 10.1038/cddis.2013.82
Zhu, D., Zhang, S., Wang, X., Xiao, C., Cui, G., and Yang, X. (2024). Secretory clusterin inhibits dopamine neuron apoptosis in MPTP mice by preserving autophagy activity. Neuroscience 540, 38–47. doi: 10.1016/j.neuroscience.2024.01.010
Zhu, H., Xiao, F., Xiao, Y., Guo, Y., Shan, X., Zhang, Z., et al. (2023). Targeting CB2R in astrocytes for Parkinson’s disease therapy: Unraveling the Foxg1-mediated neuroprotective mechanism through autophagy-mediated NLRP3 degradation. J. Neuroinflamm. 20:304. doi: 10.1186/s12974-023-02989-2
Zhu, J., Xu, F., Lai, H., Yuan, H., Li, X.-Y., Hu, J., et al. (2023). ACO2 deficiency increases vulnerability to Parkinson’s disease via dysregulating mitochondrial function and histone acetylation-mediated transcription of autophagy genes. Commun. Biol. 6:1201. doi: 10.1038/s42003-023-05570-y
Zhu, W., Zhang, H., Gao, J., and Xu, Y. (2021). Silencing of miR-497-5p inhibits cell apoptosis and promotes autophagy in Parkinson’s disease by upregulation of FGF2. Environ. Toxicol. 36, 2302–2312. doi: 10.1002/tox.23344
Zhu, Z., Huang, P., Sun, R., Li, X., Li, W., and Gong, W. (2022). A novel long-noncoding RNA LncZFAS1 prevents MPP+-induced neuroinflammation through MIB1 activation. Mol. Neurobiol. 59, 778–799. doi: 10.1007/s12035-021-02619-z
Zoccal, D. B., Silva, J. N., Barnett, W. H., Lemes, E. V., Falquetto, B., Colombari, E., et al. (2018). Interaction between the retrotrapezoid nucleus and the parafacial respiratory group to regulate active expiration and sympathetic activity in rats. Am. J. Physiol. 315, L891–L909. doi: 10.1152/ajplung.00011.2018
Keywords: Parkinson’s disease (PD), cell death mechanisms, apoptosis, neural control of breathing, autophagy, PD animal models
Citation: Pedrão LFAT, Medeiros POS, Leandro EC and Falquetto B (2024) Parkinson’s disease models and death signaling: what do we know until now? Front. Neuroanat. 18:1419108. doi: 10.3389/fnana.2024.1419108
Received: 17 April 2024; Accepted: 04 September 2024;
Published: 29 October 2024.
Edited by:
Huijiao Liu, China Agricultural University, ChinaReviewed by:
Claudio Da Cunha, Federal University of Paraná, BrazilJordi Bové, Vall d’Hebron Research Institute (VHIR), Spain
Copyright © 2024 Pedrão, Medeiros, Leandro and Falquetto. This is an open-access article distributed under the terms of the Creative Commons Attribution License (CC BY). The use, distribution or reproduction in other forums is permitted, provided the original author(s) and the copyright owner(s) are credited and that the original publication in this journal is cited, in accordance with accepted academic practice. No use, distribution or reproduction is permitted which does not comply with these terms.
*Correspondence: Barbara Falquetto, YmFyYmFyYWZAaWNiLnVzcC5icg==