- 1Department of Development and Research, Maruhachi Muramatsu Inc., Shizuoka, Japan
- 2Department of Bioregulatory Science (Physiology), Graduate School of Medicine, Nippon Medical School, Bunkyō, Japan
- 3Research Center for Basic Medicine, International University of Health and Welfare, Ohtawara, Tochigi, Japan
Objects: Recently, a non-neuronal cardiac cholinergic system, in which cardiomyocytes are equipped with components to synthesize acetylcholine, is considered to be important for maintaining physiological homeostasis in the heart, according to its anti-ischemia and hypoxia effects and angiogenesis-enhancing effects to salvage myocardium. Furthermore, it influences sustaining blood brain barrier functions. However, it remains to be fully elucidated whether any substance plays a role in activating the system.
Methods: Using Katsuo extract derived from dried bonito, called Katsuobushi in Japanese, we performed in vitro and in vivo studies whether Katsuo extract activates the non-neuronal cardiac cholinergic system and influences the associated physiological responses, specifically focusing on anti-inflammatory property and potentiation of blood brain barrier functions.
Results: Katsuo extract potently activates the non-neuronal cardiac cholinergic system and the parasympathetic nervous system. In vitro and in vivo murine models clearly showed that Katsuo extract also exerted anti-inflammatory action by suppressing cytokine production and microglial activation against pathogenic and non-pathogenic factors. Furthermore, it upregulated blood brain barrier components, such as claudin-5 and occludin, strengthened the function and prevented disruption in a brain injury model, and finally influenced murine higher brain functions by activating resiliency against depressive or anxiety-like behaviors.
Conclusion: Therefore, the novel findings of this study indicate that Katsuo extract possesses characteristic anti-inflammatory and blood brain barrier consolidation effects, and the non-neuronal cardiac cholinergic system activation. The intake might be effective in influencing pathophysiology of neuroinflammation-related diseases.
Introduction
Katsuo extract (KE) is derived from “Katsuobushi (Arabushi),” made of dried bonitos, releases umami ingredients, such as inosinic acid and amino acids, when dissolved in hot water. In Japan KE, which is similar to so called “katsuo dashi,” is an indispensable component of traditional Japanese food.
KE alters the taste of Japanese food and the mood of people who consume it (Funatsu et al., 2015). KE contains many ingredients, such as inosine which exerts an anti-depressive effect (Muto et al., 2014), and exerts an anti-sympathetic nervous system effect that enhances the parasympathetic nervous system (PNS) (Umeki et al., 2008). Therefore, KE intake often induces a relaxing effect in humans, modulates the autonomic nervous system (ANS) to decrease systolic blood pressure (Kouno et al., 2005), and prevents the progression of hyperlipidemia (Matsumoto et al., 2007). These studies provide us a consensus on the influence of KE on higher brain functions in terms of psychological aspects; however, constituents involved in them remain to be fully elucidated.
To date, we have investigated modalities, by which the non-neuronal cardiac cholinergic system (NNCCS), is activated (Kakinuma et al., 2009; Kakinuma et al., 2013; Oikawa et al., 2015; Oikawa et al., 2019a; Saw et al., 2021). The NNCCS is a system in which cardiomyocytes synthesize acetylcholine (ACh), independently of the PNS, using the ACh synthesis enzyme, choline acetyltransferase, and choline transporters (Kakinuma et al., 2009; Rana et al., 2010). The NNCCS has been extensively studied for its anti-ischemic role (Rocha-Resende et al., 2012; Kakinuma et al., 2013; Roy et al., 2013; Gavioli et al., 2014; Roy et al., 2016), anti-inflammatory role (Rocha-Resende et al., 2019) in the heart and the other organs including the brain, via the vagus nerve (Oikawa et al., 2016; Oikawa et al., 2019b). Our murine model with an enhanced NNCCS overexpressing the choline acetyltransferase (ChAT) gene specifically in the heart (ChAT tg), had a higher survival rate after myocardial infarction (MI) than wild-type mice (Kakinuma et al., 2013). Furthermore, this model presented enhancement of the blood-brain barrier (BBB), a striking phenotype in the brain (Oikawa et al., 2016; Oikawa et al., 2019b). Specifically, ChAT tg expressed more claudin-5, a component protein of the BBB, and showed a resilient phenotype against BBB dysfunction models (Oikawa et al., 2019b).
Based on these beneficial effects of the NNCCS on brain and heart functions, an inducer of the NNCCS may be expected, which can influence brain function probably through BBB components, as well as the heart or ANS. In the current study, we investigated whether KE can induce NNCCS and influence BBB function through the upregulation of its components.
Materials and methods
Animals
Male C57BL/6J mice, weighing 25–30 g, were used for the KE administration experiments. KE was dissolved in tap water at a concentration of 10 mg/ml. This concentration was determined based on the concentration (1 mg/ml) used in an in vitro experiment to investigate the anti-inflammatory effect of KE on 1 μg/ml lipopolysaccharides (LPS)-treated MG6 cells. KE was orally administered for 3 days to 2 weeks depending on the experimental protocol. Mice purchased from Tokyo Laboratory Animals Science Co., Ltd., Tokyo, Japan, were maintained and housed at 23°C under a strict 12-h light/12-h dark cycle. Murine chow and water were provided ad libitum. The experimental procedures and protocols included in this study were approved by the ethics committee of Nippon Medical School (permission number: 2020-011). All animal experiments were performed in strict accordance with the recommendations of the ARRIVE Guidelines and carried out in accordance with the National Research Council’s Guide for the Care and Use of Laboratory Animals.
Reagents
Dulbecco’s modified Eagle medium (DMEM) and 2-mercaptoethanol were purchased from ThermoFisher Scientific K. K. (Tokyo, Japan)., and LPS from Escherichia coli O 111:B4 and TWEEN20 were from Sigma-Aldrich Co. LLC (St. Louis, Missouri, United States). Fetal bovine serum (FBS) was from Biowest (Nuaillé, France). All the other chemical reagents were purchased from FUJIFILM Wako Pure Chemical Corporation (Osaka, Japan). LPS dissolved in PBS at 1 mg/ml was stored, and diluted 100 times before use.
Katsuo extract preparation
Dried bonito “arabushi” was purchased from Kaneninishi, Co., Ltd. “Arabushi” was sliced thinly, suspended in ultrapure water, and then boiled at 95°C for 30 min. The suspension containing boiled “Arabushi” slices was centrifuged and the supernatant was collected by decantation. The supernatant was concentrated in vacuo using a concentrator, and reconstituted in ultrapure water at a concentration of 100 mg/ml and used as KE after filtration using a 0.2 µm membrane (Pall Corporation, Port Washington, New York, United States). Nutritional ingredients of KE (100 mg/ml) were analyzed using general methods to show in Table 1.
Measurement of cardiac acetylcholine contents
As previously reported, the cardiac ventricles alone were excised and homogenized in lysis buffer containing isopropyl homocholine as an internal control, specifically prepared for ACh measurement (Kakinuma et al., 2013). After centrifugation and adjustment of the pH, the supernatant was purified using an Amicon® Ultra column centrifuge filter. The flow-through was collected and injected (10 μl) into a high-performance liquid chromatography system to evaluate the ACh levels in the heart.
Western blot analysis
The heart (100 mg), with the atrium excluded to obtain only the ventricle, was excised, homogenized in 1 ml of TPER buffer, and centrifuged to obtain the supernatant. The protein concentration in each sample was measured to adjust the quantity in each well. The supernatant was mixed with sampling buffer and boiled for 10 min. Electrophoresed samples were blotted and followed by a blocking procedure with 4% skim milk, and subjected to a reaction with a primary antibody. Thereafter, the membrane was incubated with an HRP-conjugated second antibody. Following a chemical reaction with ImmunoStar (FUJIFILM Wako Pure Chemical Corporation), signal intensity was measured using a C-DiGit Blot Scanner (LI-COR Corporate, Lincoln, Nebraska, United States) (Oikawa et al., 2016; Oikawa et al., 2019a; Oikawa et al., 2019b). The primary antibodies used were as follows: a goat anti-ChAT polyclonal antibody (ab) (#AB144P, 1:2000, EMD Millipore Co., Temecula, CA, United States); rabbit anti-GAPDH monoclonal ab (#2118, 1:5000, Cell Signaling Technology, Danvers, Massachusetts, United States); rabbit anti-claudin-5 monoclonal ab (#ab131259, 1:1000, Abcam, Cambridge, United Kindom); mouse anti-occludin monoclonal ab (sc-271842, 1:500, Santa Cruz Biotechnology, Inc., Dallas, Texas, United States); and mouse β-actin monoclonal ab (sc-47778, 1:3000, Santa Cruz Biotechnology, Inc.).
Cold brain injury
As previously reported (Oikawa et al., 2019b), cold brain injury was induced using a bronzed cylinder with a diameter of 4 mm, which was chilled in liquid nitrogen. Mice were anesthetized with a mixture of medetomidine hydrochloride, midazolam, and butorphanol (0.3, 4.0, 5.0 mg/kg/dose). Then, the murine scalp was sagittally cut to see the right parietal bone. The chilled cylinder was attached to the right parietal region through the skull after skin incision for 5 s, followed by suturing the scalp. Twenty-4 hours after injury, 3% Evans blue (200 μl) was intraperitoneally administered. After confirming that the dye was completely distributed, mice were cervically dislocated and the brain was excised. A coronal slice of the brain (2-mm thick), which maximally included Evans blue-positive area, was halved into the right (injured region) and left (intact region). Each half-sliced brain sample was incubated overnight with formamide at 50°C. The concentration of the leaked Evans blue solution was determined by spectrophotometry at 590 nm absorption. In comparison with a standardized concentration of Evans blue, a leaked Evans blue level was determined. In addition, total RNA was isolated from the brain subjected to cold brain injury with or without KE treatment, followed by qPCR to evaluate mRNA levels of cytokines, IL-6, IL1β, TNF-α, and RANTES, using their specific primers as previously reported (Oikawa et al., 2019b).
Restraint stress
Mice were subjected to restraint stress using a wire restrainer for 2 h, which was tightly wrapped around the mice in the morning, as previously reported (Oikawa et al., 2016).
Tail suspension test and forced swimming test
As previously reported, TST was performed as follows: mice were suspended from a horizontal rod by their tails attached using an adhesive tape. They were suspended for 10 min and the time of immobility was measured (Oikawa et al., 2016). In the FST, mice were placed in a cylindrical water bath (40 cm in height × 20 cm in diameter) maintained at 23°C (a depth 25 cm) for 10 min (Oikawa et al., 2016). The floating duration was measured in which they quit swimming and only appeared to keep their head above water. The duration of immobile status was measured during the last 4 min of the test (Oikawa et al., 2016).
Lipopolysaccharides challenge test
To evaluate the effects of KE on inflammation, mice were treated with LPS (10 mg/kg/dose), and 4 h later, the liver was excised to isolate total RNA and measure the mRNA expression levels of cytokines, IL-1β and TNF-α, using qPCR (Oikawa et al., 2019b). In addition, blood was also collected 4 h after LPS treatment to measure the concentrations of TNF-α and IL-6 using ELISA kits (Oikawa et al., 2019b).
Immunohistochemical analysis
After deeply anesthetized with the mixture of medetomidine hydrochloride (0.3 mg/kg/dose), midazolam (4.0 mg/kg/dose), and butorphanol (5.0 mg/kg/dose), they were perfused with PBS through the cardiac ventricle, followed by perfusion with 4% paraformaldehyde. The excised brain was immersed in 4% paraformaldehyde overnight, followed by immersion in 20% sucrose for 1 day; after being embedded in OCT compound, the brain was sectioned. After inhibition of endogenous peroxidase and blocking, sectioned samples were reacted with a primary ab (rabbit anti-Iba1 polyclonal ab, #016-20001, 1:500, FUJIFILM Wako Pure Chemical Corporation), followed by a reaction with Alexa Fluor 568 goat anti-IgG ab. Immunofluorescence signals were observed using a confocal laser microscope (FV3000, OLYMPUS, Tokyo, Japan).
Cell culture
Primary rat brain endothelial cells (rBECs) were purchased from PharmaCo-Cell Company, Ltd. (Nagasaki, Japan). Cells were maintained in DMEM F-12 containing 10% FBS and heparin (100 μg/ml), bFGF (1.5 ng/ml), ITS (10 μg/ml insulin, 5.5 μg/ml transferrin, and 6.7 ng/ml selenite), hydrocortisone (500 nM), and gentamicin (50 μg/ml) according to the manufacturer’s instructions. Before treatment with KE (0.1, 1, 10 mg/ml), the cells were subjected to serum starvation for 20 min, then substituted to KE and cultured for 24 and 72 h. Samples were collected to isolate total RNA for qRT-PCR or proteins for western blot analysis. The following rat-specific primers for claudin-5 and occludin were used: claudin-5: (forward) 5′-TGTGTGGGCTTCTGGCACTG-3′ (reverse) 5′-GGCACCGTTGGATCATAGAACTC-3’; occludin: (forward) 5′-GGTGGCGAGTCCTGCG-3′ (reverse) 5′-CTGTTGATCTGAAGTGATAGGTGGA-3’.
MG6 cells, a cell line representing murine microglial cells immortalized with c-myc, were used for in vitro anti-inflammatory potency assay of KE (Takenouchi et al., 2005), and purchased from RIKEN Bio-Resource Center, Tsukuba, Japan. They were maintained in DMEM including 10% FBS, 10 μg/ml insulin, and 0.1 mM 2-mercaptoethanol. After reaching 70%–80% confluency, they were treated with 1 mg/ml KE and, 1 h later, with 1 μg/ml LPS for 6 h. Then, cell viability and cytokine concentrations in the supernatant were evaluated using kits.
Cytotoxicity assay
Relative cell viability was determined using Cell Count Reagent SF (Nacalai Tesque, Inc., Kyoto, Japan) according to the manufacturer’s protocol. WST-8 [2-(2-methoxy-4-nitrophenyl)-3-(4-nitrophenyl)-5-(2,4-disulfophenyl)-2H-tetrazolium, monosodium salt] produced a water-soluble formazan dye. Briefly, after sampling the cell culture supernatant (10 µl), 10 µl of Cell Count Reagent SF was added to it. The absorbance was measured after a 2 h reaction at 450 nm with 620 nm as the reference wavelength.
Enzyme-linked immunosorbent assay
MG6 cells were seeded in 96-well plates at a density of 5 × 103 cells/well in 90 µl of the specific culture medium mentioned above. After 30 min culture at 37°C, 1 µl of KE (0, 0.01, 0.03, 0.1, 0.3, and 1 mg/ml) was applied into each well and cultured for 1 h. The cells were then stimulated with LPS for 6 h. The supernatant (10 µl) was collected and diluted 25 times with PBS. Then, samples were assayed for TNF-α using Mouse TNF-α ELISA MAX Standard (BioLegend, San Diego, California, United States).
New object recognition test
According to a previous report, mice treated with KE were tested to determine whether KE influenced new object recognition ability (Domachevsky et al., 2013). Briefly, the mice were habituated to walk freely for 10 min in a cylinder, 30 cm in diameter and 30 cm in height, on day 1, followed by a test experiencing the same objects on day 2, and the last test experiencing different objects on day 3. The duration, distance, and entering frequency in the area of interest were measured to compare the differences between the control and KE-treated mice.
Statistics
All analyses were performed using ystat statistics software (Igakutosho-shuppan Ltd., Tokyo, Japan). Data are expressed as the mean ± standard deviation (SD). Comparisons between two groups were performed using a Mann−Whitney U test. For multiple comparison, differences were assessed by one-way ANOVA with post-hoc Dunnett’s tests.
Differences were considered statistically significant at p-value<0.05.
Results
Katsuo extract suppresses lipopolysaccharides-induced inflammatory responses in MG6 cells
We first examined the effects of KE on pro-inflammatory cytokine secretion in microglial MG6 cells. Various concentrations of KE were set and TNF-α secreted by MG6 after LPS stimulation was measured. The TNF-α levels were significantly decreased by KE in a concentration-dependent manner compared to that in control cells exposed to LPS (+). The inhibitory effects were 20.2, 33.9, 47.4, 60.6, and 83.0% at KE concentrations (0.01, 0.03, 0.1, 0.3, and 1.0 mg/ml), respectively (***p = 0.049 in 1 mg/ml KE, Figure 1). Similarly, the secretion of IL-6 was significantly inhibited in a KE concentration-dependent manner (data not shown). However, a significant decrease in cell viability was not observed; rather, cell viability significantly increased in LPS (+) as compared to LPS (−) (#p = 0.049, Figure 1), probably due to an increase in cell proliferation or mitochondrial metabolic activity. These results revealed that KE dose-dependently suppressed the release of LPS-induced pro-inflammatory cytokines in MG6 cells.
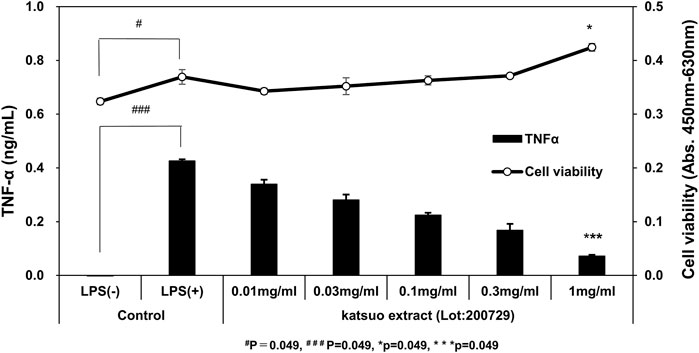
FIGURE 1. Katsuo Extract (KE) attenuates TNF-α secretion in the culture medium of MG6 cells treated with LPS. The TNF-α levels in the culture medium increased significantly by 1 μg/ml LPS stimulus, compared to LPS (−) (###p = 0.049). On the other hand, in the presence of LPS, the TNF-α levels were dose-dependently decreased by 0.01, 0.03, 0.1, 0.3, and 1 mg/ml KE (***p = 0.049) compared to LPS (+), without influencing cell viability [*p = 0.049 in 1 mg/mL KE vs. Control LPS(+)].
Katsuo extract attenuates inflammatory responses to lipopolysaccharides in vivo
The KE-induced anti-inflammatory responses of MG6 cells prompted us to further investigate anti-inflammatory influences of KE in vivo. Mice, orally administered KE (10 mg/ml) for 3 days, showed significantly decreased blood levels of TNF-α and IL-6 compared with control mice in response to 10 mg/ml LPS (TNF-α: 113.4 ± 56.4 pg/ml vs. 190.7 ± 36.0 pg/ml, n = 6, p = 0.023; IL-6: 33.9 ± 12.9 ng/ml vs. 67.0 ± 15.8 ng/ml, n = 4, p = 0.039, respectively; Figure 2A). The local effects of KE against LPS were also examined in the liver. KE significantly decreased cytokine mRNA expression levels in TNF-α, IL-1β, and RANTES (CCL5), which were corrected by β-actin mRNA levels (TNF-α: 4.5 ± 1.3 vs. 10.4 ± 2.9, n = 5, p = 0.014; IL-1β: 4.4 ± 1.1 vs. 8.0 ± 1.6, n = 5, p = 0.019; RANTES 18.1 ± 4.6 vs. 29.2 ± 4.8, p = 0.014; Figure 2B). These results clearly indicate that KE suppresses inflammatory responses to LPS throughout the body.
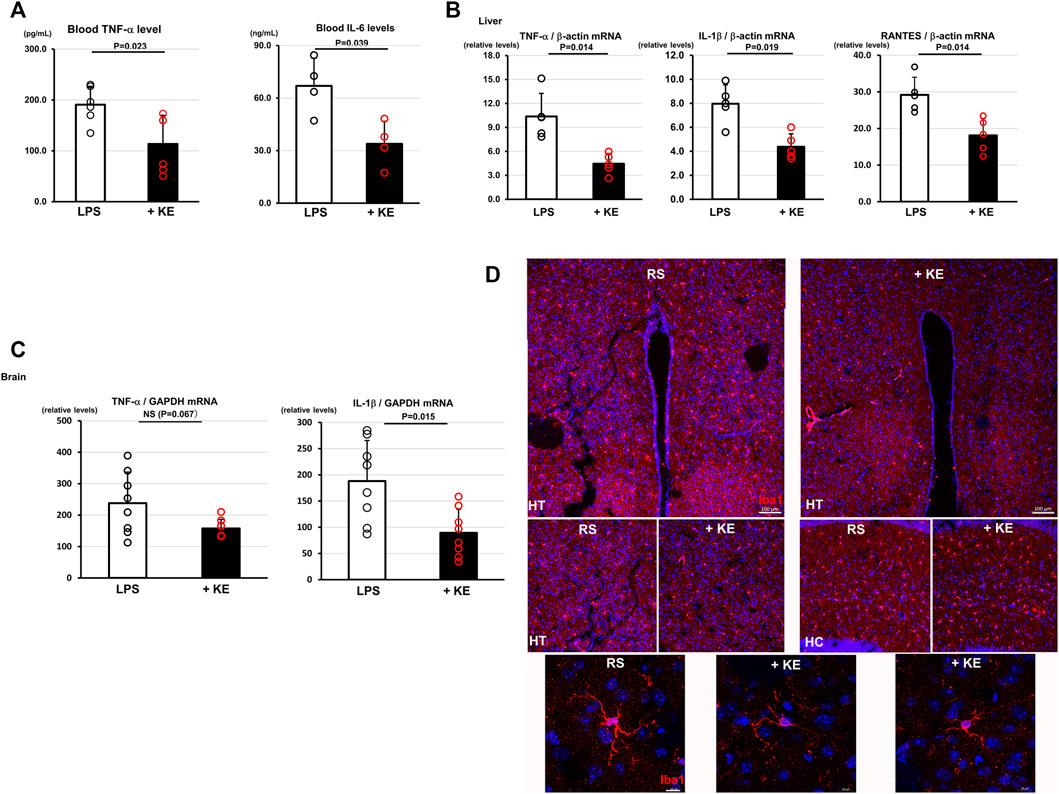
FIGURE 2. KE attenuates inflammatory responses against LPS and restraint stress in vivo. (A).Orally administered KE for 3 days significantly suppressed blood levels of TNF-α (p = 0.023) and IL-6 (p = 0.039) of mice which were once treated with 10 mg/ml LPS. (B).KE decreased the mRNA expression levels of TNF-α (p = 0.014), IL-1β (p = 0.019), and RANTES (p = 0.014) in the liver of KE administered mice which were challenged by 10 mg/ml LPS. (C).In restraint stress (RS) experiments, KE also decreased the mRNA level of IL-1β (p = 0.015); however, it was associated with a decreasing trend of TNF-α level (NS, p = 0.067), in the brain of treated mice compared with non-treated mice. (D).In KE-treated mice subjected to RS (+KE), Iba1 immunoreactive signals of microglia, which were evident in the non-treated brain, were decreased and the hypertrophied appearance of microglia was less evident in the hypothalamus (HT) and hippocampus (HC). Scale bar: 100 μm. The lower panels showed magnified views of microglia in the HT. Scale bar: 10 μm.
Katsuo extract attenuates neuroinflammatory responses against restraint stress
Mice were subjected to RS, which causes neuroinflammation through stress instead of a pathogen. A mRNA expression level of TNF-α showed a decreasing trend; however, that of IL-1β, corrected by the GAPDH mRNA level, in the brains of KE-treated mice was significantly decreased, compared to those of control mice, when subjected to 2 h of RS (TNF-α: 157.5 ± 28.8 vs. 238.2 ± 98.7, n = 8, p = 0.067; IL-1β: 89.4 ± 45.3 vs. 188.3 ± 77.3, n = 8, p = 0.015; Figure 2C), suggesting that KE also attenuates neuroinflammatory responses against RS. Consistent with this, immunohistochemical analysis revealed that KE suppressed microglial responses to RS with the decreased Iba1 positive cells and attenuated hypertrophic appearance (Figure 2D). The alteration of microglial appearance by KE also suggests that KE plays a crucial role in suppressing neuroinflammatory response, even in a pathogen-free inflammatory model.
Katsuo extract activates a non-neuronal cardiac cholinergic system, an intrinsic acetylcholine synthesis system equipped with cardiomyocytes
Since the cholinergic system, either neuronal or non-neuronal in origin including NNCCS, has been extensively revealed to play an anti-inflammatory role (Borovikova et al., 2000; Giunta et al., 2004; Oikawa et al., 2019b; Frinchi et al., 2019), these findings prompted us to investigate whether KE activates the NNCCS. As shown in Figure 3, KE-treated murine hearts (2 weeks) increased ChAT protein expression (158.7 ± 50.8% vs. 100.0 ± 14.1%, n = 5, p = 0.027), leading to significantly augmented ACh content in the heart, compared with control hearts [7.9 ± 1.4 vs. 3.4 ± 0.7 (× 10−10 mol/g tissue), n = 5, p = 0.014].
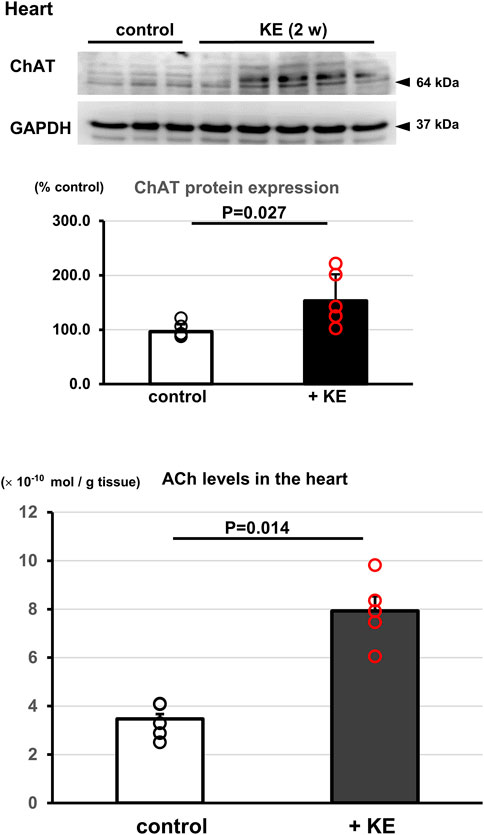
FIGURE 3. KE upregulates the NNCCS to increase ACh levels in the heart. Mice treated with KE for 2 weeks showed increased ChAT protein expression in the heart (p = 0.027), leading to a significant increase in ACh content in the heart (p = 0.014).
Katsuo extract reduces heart rate in mice, leading to activation of the parasympathetic nervous system
A hemodynamic study was further conducted in KE-treated mice. As shown in Table 2, 1 week KE-treated mice showed a significant decrease in HR alone (345 ± 22 bpm, n = 7, p = 0.005). Moreover, KE treatment (2 weeks) significantly decreased HR (361 ± 36 bpm vs. 461 ± 70 bpm, n = 7, p = 0.010) and decreased systolic and diastolic blood pressure (SBP: 122.5 ± 7.9 mmHg, n = 7, p = 0.017; DBP: 90.7 ± 9.1 mmHg, p = 0.010; Table 2). These results indicate that KE also influences the activation of the PNS.
Katsuo extract-treated mice share critical brain phenotypes with the murine non-neuronal cardiac cholinergic system activated model
Our murine model of activated NNCCS, represented by ChAT tg (Kakinuma et al., 2013), included modification of higher brain functions, such as anti-stress, anti-depression, and anti-anxiety phenotypes, as well as consolidation of BBB functions, (Kakinuma et al., 2013; Oikawa et al., 2016; Oikawa et al., 2019b). We further studied whether KE-treated mice presented phenotypes in the CNS similar to those of ChAT tg. Figure 4 showed that KE attenuated depression-like phenotypes in mice because immobile durations of KE-treated mice both in the TST and FST were significantly shortened compared with non-treated mice (TST: 173 ± 26 s vs. 315 ± 38 s, n = 5–6, p = 0.010; FST: 78 ± 16 s vs. 120 ± 43 s, n = 10, p = 0.007). The anti-depression-like phenotypes of KE-treated mice were comparable to ChAT tg.
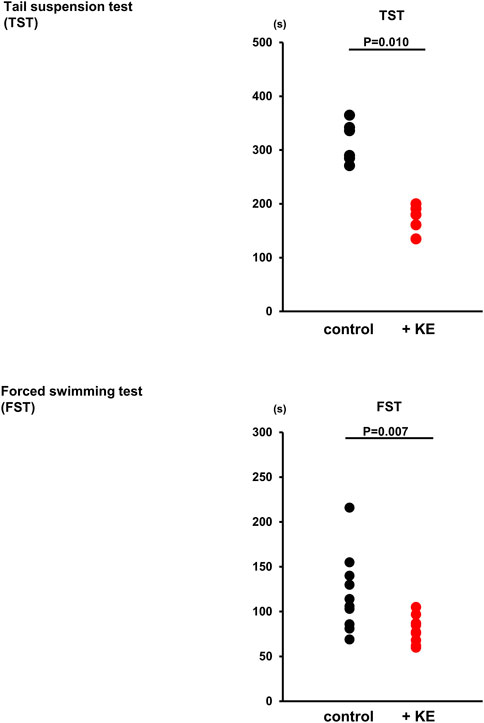
FIGURE 4. KE decreases the immobile duration of mice in a test evaluating a depression-like condition. In both tail suspension test (TST) (p = 0.010) and forced swimming test (FST) (p = 0.007), KE significantly decreased the duration of immobility in mice compared with that in non-treated mice.
Katsuo extract-treated mice upregulate blood-brain barrier component protein expression
KE was found to attenuate inflammatory responses in the brain with reduced Iba1 positive immunoreactivity (Figure 2D), suggesting that KE suppresses microglial activation induced in RS. Western blot analysis using brain lysates from mice subjected to RS revealed that the protein expression levels of BBB components, specifically claudin-5 and occludin, were influenced by KE. Specifically, as shown in Figure 5A, RS decreased occludin protein expression; however, KE suppressed this reduction (occludin: KE vs. RS alone, 105.9% ± 22.0% vs. 61.8% ± 10.6%, F (2,15) = 15.14, p < 0.01). In contrast, claudin-5 expression levels were upregulated by KE, even with RS (claudin-5: KE vs. RS alone, 179.2% ± 12.8% vs. 118.1% ± 5.7%, F (2,12) = 24.45, p < 0.01). Consequently, GFAP (a marker of astrocytes) protein expression was attenuated in KE compared with RS (KE vs. RS alone, 69.8% ± 8.1% vs. 100.3% ± 5.6%, F (2,15) = 6.67, p < 0.01) due to decreased extravasation triggered by increased BBB consolidation. (Figure 5A). These results suggest that KE upregulates the protein levels of the BBB components.
In addition, to strengthen a link between KE and BBB function, mice were subjected to cold brain injury (CBI), a representative BBB dysfunction model, in which BBB function was transiently disrupted by CBI leading to the leakage of Evans blue dye specifically in the injury region. Compared with control mice, KE-treated mice showed significantly less leakage of Evans blue in the brain (5.1 ± 1.1 μg/ml vs. 2.8 ± 0.8 μg/ml, n = 10, p = 0.002, Figure 5B).
These results prompted us to further investigate whether KE upregulates claudin-5 under intact conditions in vitro and in vivo. Rat primary brain endothelial cells (rBECs) treated with 1.0 mg/mL KE increased mRNA expression levels of both occludin and claudin-5 after 72 h (occludin: 432.1%; claudin-5: 442.6%, data not shown). This trend was confirmed using Western blot analysis of rBECs. Specifically, claudin-5 protein expression was upregulated after 72 h-treatment with KE (193.2% ± 9.2%, n = 3–4, p = 0.036, Figure 5C). Furthermore, compatible with this, control mice treated with KE also increased claudin-5 protein expression in the brain (197.2% ± 55.7%, p = 0.034, Figure 5D). Due to the upregulation of the BBB component in KE-treated murine brain, gene expression of cytokines was significantly attenuated even in the control mice compared with non-treated mice (IL-6: 4.9 ± 1.7 vs. 139.5 ± 85.0, p = 0.025; IL-1β: 3.7 ± 1.6 vs. 45.1 ± 29.2, p = 0.025; RANTES: 4.7 ± 1.9 vs. 73.5 ± 52.1, p = 0.025, Figure 5E). Together, these results clearly indicate that KE positively regulates BBB components and sustains its function in a pathological model, which are shared with ChAT tg, that is, consolidated functions of BBB in the ChAT tg brain.
Katsuo extract influences murine higher brain function
As demonstrated, KE plays a role in suppressing systemic and local inflammation and enhancing BBB function. These data prompted us to investigate whether KE can influence murine behavior using a new object recognition test. As shown in Figure 6, KE-treated mice stayed for a more prolonged time with more frequency and distance in the central area between two objects (duration: 22 ± 12 s vs. 7 ± 18 s, n = 11, p = 0.003; frequency: 11 ± 7 vs. 3 ± 5, n = 11, p = 0.004; distance: 751.9 ± 453.8 mm vs. 233.4 ± 376.0 mm, n = 11, p = 0.010). This finding suggests that KE attenuates the cautious mood of mice.
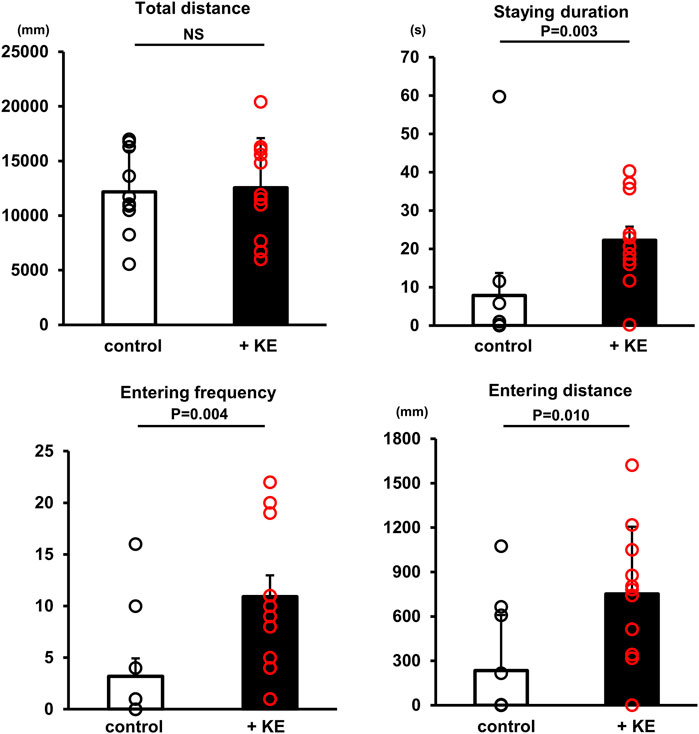
FIGURE 6. KE influences higher brain functions of mice. Although the total distance travelled by mice during the whole test was comparable between non-treated mice and KE-treated mice, mice with KE preferentially entered the central area and stayed there longer than non-treated mice.
Discussion
This study demonstrates for the first time that KE not only upregulates the NNCCS, but also shows the phenotypes of the murine activated NNCCS model, that is, heart-specific ChAT tg. Specifically, KE suppresses pro-inflammatory responses, provides mice with resilience to a depression-like phenotype and anxiety, and positively regulates BBB components to strengthen BBB functions. Although KE are known to exert a relaxing effect, the anti-inflammatory and BBB-reinforcing effects are considered to be novel. Particularly, no studies have reported on the aspects of upregulation of claudin-5 to further consolidate the BBB.
The NNCCS is a system equipped with cardiomyocytes to synthesize ACh independent of the PNS since cardiomyocytes possess all components of the machinery, including choline transporter (CHT1), ChAT, vesicular ACh transporter (VAChT), and acetylcholinesterase (AChE) (Kakinuma et al., 2009); although reports indicated that ACh was detected in cardiomyocytes, detailed investigation of these aspects had not been conducted. Recently, it has been proven that the NNCCS is a crucial machinery for cardiomyocytes in terms of physiological homeostasis (Kakinuma et al., 2009; Rana et al., 2010; Rocha-Resende et al., 2012; Kakinuma et al., 2013; Roy et al., 2013; Gavioli et al., 2014; Roy et al., 2016; Saw et al., 2021). Our recent study clearly showed that downregulation of NNCCS caused cardiac dysfunction due to enhanced ROS exposure to the heart, dysregulated cardiac energy metabolism, especially resulting in impaired glucose metabolism with downregulated protein expression of glucose transporter-4, and malfunction of NOS1 in the heart (Oikawa et al., 2021). However, when the NNCCS was activated, as shown by ChAT tg, they were remarkably resistant to ischemic insults, including myocardial infarction (MI); ChAT tg subjected to MI survived more than WT mice in the chronic MI phase (Kakinuma et al., 2013). Based on the murine model results, we suggest that an NNCCS inducer is mandatory as a therapeutic modality to positively influence the resilient properties of cardiomyocytes. We then searched for an inducer and finally obtained several candidates (Kakinuma et al., 2009; Oikawa et al., 2015; Oikawa et al., 2019a), among which KE was identified to enhance the NNCCS.
As a second novel result of this study, KE was found to play a role in inhibiting a proinflammatory reaction. Our in vitro study using MG6 cells showed that KE efficiently suppressed TNF-α production in response to LPS. The optimized concentration of KE for an in vitro anti-inflammatory response was approximately 0.1–1.0 mg/ml, which was preliminarily confirmed to have the comparable potency to that of several immunosuppressants (cyclosporine A and FK506; data not shown). KE can be a more suitable therapeutic compound, as it is derived from dried bonito and considered to be less harmful than immunosuppressants. Even in an in vivo model, KE exerted its anti-inflammatory action, for example, the effects of KE on LPS-induced systemic inflammation, as well as on RS and intact brain responses, where KE downregulated local cytokine expression levels in the liver, brain, and blood. Moreover, KE attenuated microglial responses to RS. Based on these results, KE was found to possess an anti-inflammatory activity.
From our nutritional analysis of KE, we consider that compounds containing nitrogen, such as polyamines, amino acids, peptides, and nucleic acids, which are well known to be included in “Katsuobushi,” may suppress inflammation and activate NNCCS. For example, it has been reported that spermidine and spermine possess anti-inflammatory effects (Zhang et al., 1997; Choi and Park, 2012) and that dipeptide Leucine-Histidine attenuates microglial activation (Ano et al., 2019). Therefore, we have now purified and analyzed KE to identify a specific and responsible active compound through monitoring effects of anti-inflammation and BBB consolidation, although some of compounds, mentioned above, are also found in KE as one of candidate ingredients for the specific effects.
Cholinergic agonists also play a role in suppressing inflammation. Nicotine, a representative nicotinic receptor agonist, an α7 nicotinic receptor agonist, and ACh have been reported to have anti-inflammatory effects in many in vitro and in vivo experimental models (Giunta et al., 2004; Frinchi et al., 2019). In addition, stimulation of the vagus nerve has been reported to negatively regulate inflammatory responses in vivo (Borovikova et al., 2000). All these studies have supported the concept that the cholinergic system acts as an anti-inflammatory machinery (Rosas-Ballina and Tracey, 2009). Thus, KE was confirmed to possess anti-inflammatory potency and activate the NNCCS. Furthermore, KE decreased the HR in the treated mice, suggesting that KE also activated the PNS. Whether KE includes ACh or its agonistic compounds needs further examination; however, it is thought that KE activates the local and systemic cholinergic systems, which may involve its anti-inflammatory effects. This is another novel finding of this study.
We have already reported that, when the NNCCS is activated by ChAT tg mice, extra-cardiac organs may be influenced probably through the vagus nerve ascending pathway. Among several organs innervated by the vagus nerve, the brain is initially influenced by the activated NNCCS to modulate higher brain functions and sustain BBB integrity (Oikawa et al., 2016; Oikawa et al., 2019b). Since KE stimulates the NNCCS, it is expected that KE also modulates BBB and higher brain functions. Exactly, KE-treated mice showed an anti-depression-like phenotype, which was shared by ChAT tg mice. In addition, they possessed an anti-BBB injury phenotype with less leakage of systemically administered Evans blue dye than untreated mice, when they were subjected to CBI. Furthermore, intact mice treated with KE alone showed upregulated claudin-5 protein expression, and primary cultured rBECs also upregulated gene expression of claudin-5 and occludin, and claudin-5 protein expression in response to KE. These results clearly demonstrate that KE upregulates BBB components and strengthens BBB integrity, which are common with ChAT tg. This is the third novel finding of this study, since it has never been reported that KE regulates BBB integrity. Recently, one report indicated that an α7 nicotinic receptor agonist upregulates the BBB component of endothelial cells in vitro (Kimura et al., 2019), supporting our speculation that KE directly regulates BBB function maybe through a cholinergic pathway.
Consequently, anti-inflammatory and BBB consolidation effects of KE influenced higher brain function, as shown by a new object recognition test. KE accelerated mice to enter an open central region more frequently and stayed there for a long time. These results suggest that KE attenuates anxiety in mice. Therefore, it is possible that KE may positively modulate mood partly through the loss of anxiety and depression-like phenotypes.
Conclusion
Systemic administration of KE plays a role in suppressing inflammatory responses, activating the NNCCS and the PNS, and strengthening BBB functions, which are evidently shared by phenotypes of the NNCCS-activating murine model. Therefore, this study provides novel characteristics of KE which may be used for influencing those targets.
Data availability statement
The raw data supporting the conclusion of this article is made available in doi: 10.6084/m9.figshare.21001702.
Ethics statement
The animal study was reviewed and approved by the ethics committee of Nippon Medical School (permission number: 2020-011).
Author contributions
YH: Conceptualization, Methodology, Validation, Formal analysis, Investigation, Writing–original draft, Supervision, Project administration, Visualization. AS: Validation, Formal analysis, Investigation. YK: Investigation. SO: Investigation. AM: Investigation, Validation. HO: Validation, Formal analysis, Investigation, Visualization. SS: Validation, Investigation, Visualization. YK: Conceptualization, Methodology, Validation, Formal analysis, Investigation, Writing–original draft, Supervision, Project administration, Funding acquisition.
Funding
This work was principally supported by the Adaptable and Seamless Technology Transfer Program through target-driven R and D, Japan Science and Technology Agency, Tokyo, Japan, Grant Number JPMJTM20D4, and partly supported by the Japan Society for the Promotion of Science Grants-in-Aid for Scientific Research (JSPS KAKENHI), Grant Number 21K11658.
Acknowledgments
The authors would like to thank Dr. Takato Takenouchi, Institute of Agrobiological Sciences, National Agriculture and Food Research Organization, Tsukuba, Japan, for providing MG6 cells and supporting us in the culture procedure.
Conflict of interest
Authors YH and AS were employed by the company Maruhachi Muramatsu Inc.
The remaining author declares that the research was conducted in the absence of any commercial or financial relationships that could be construed as a potential conflict of interest.
Publisher’s note
All claims expressed in this article are solely those of the authors and do not necessarily represent those of their affiliated organizations, or those of the publisher, the editors and the reviewers. Any product that may be evaluated in this article, or claim that may be made by its manufacturer, is not guaranteed or endorsed by the publisher.
References
Ano, Y., Kita, M., Kitaoka, S., and Furuyashiki, T. (2019). Leucine–Histidine dipeptide attenuates microglial activation and emotional disturbances induced by brain inflammation and repeated social defeat stress. Nutrients 11 (2), 2161. doi:10.3390/nu11092161
Borovikova, L. V., Ivanova, S., Zhang, M., Yang, H., Botchkina, G. I., Watkins, L. R., et al. (2000). Vagus nerve stimulation attenuates the systemic inflammatory response to endotoxin. Nature 405 (6785), 458–462. doi:10.1038/35013070
Choi, Y. H., and Park, H. Y. (2012). Anti-inflammatory effects of spermidine in lipopolysaccharide-stimulated BV2 microglial cells. J. Biomed. Sci. 19 (1), 31. doi:10.1186/1423-0127-19-31
Domachevsky, L., Rachmany, L., Barak, Y., Rubovitch, V., Abramovich, A., and Pick, C. G. (2013). Hyperbaric oxygen-induced seizures cause a transient decrement in cognitive function. Neuroscience 247, 328–334. doi:10.1016/j.neuroscience.2013.05.052
Frinchi, M., Nuzzo, D., Scaduto, P., Carlo, D. M., Massenti, F. M., Belluardo, N., et al. (2019). Anti-inflammatory and antioxidant effects of muscarinic acetylcholine receptor (mAChR) activation in the rat hippocampus. Sci. Rep. 9 (1), 14233. doi:10.1038/s41598-019-50708-w
Funatsu, S., Kondoh, T., Kawase, T., Ikeda, H., Nagasawa, M., Denbow, D. M., et al. (2015). Long-term consumption of dried bonito dashi (a traditional Japanese fish stock) reduces anxiety and modifies central amino acid levels in rats. Nutr. Neurosci. 18 (6), 256–264. doi:10.1179/1476830514Y.0000000124
Gavioli, M., Lara, A., Almeida, P. W., Lima, A. M., Damasceno, D. D., Rocha-Resende, C., et al. (2014). Cholinergic signaling exerts protective effects in models of sympathetic hyperactivity-induced cardiac dysfunction. PLoS ONE 9 (7), e100179. doi:10.1371/journal.pone.0100179
Giunta, B., Ehrhart, J., Townsend, K., Sun, N., Vendrame, M., Shytle, D., et al. (2004). Galantamine and nicotine have a synergistic effect on inhibition of microglial activation induced by HIV-1 gp120. Brain Res. Bull. 64 (2), 165–170. doi:10.1016/j.brainresbull.2004.06.008
Kakinuma, Y., Akiyama, T., and Sato, T. (2009). Cholinoceptive and cholinergic properties of cardiomyocytes involving an amplification mechanism for vagal efferent effects in sparsely innervated ventricular myocardium. Febs. J. 276 (18), 5111–5125. doi:10.1111/j.1742-4658.2009.07208.x
Kakinuma, Y., Tsuda, M., Okazaki, K., Akiyama, T., Arikawa, M., Noguchi, T., et al. (2013). Heart-specific overexpression of choline acetyltransferase gene protects murine heart against ischemia through hypoxia-inducible factor-1α-related defense mechanisms. J. Am. Heart Assoc. 2 (1), e004887. doi:10.1161/JAHA.112.004887
Kimura, I., Dohgu, S., Takata, F., Matsumoto, J., Kawahara, Y., Nishihira, M., et al. (2019). Activation of the α7 nicotinic acetylcholine receptor upregulates blood-brain barrier function through increased claudin-5 and occludin expression in rat brain endothelial cells. Neurosci. Lett. 694, 9–13. doi:10.1016/j.neulet.2018.11.022
Kouno, K., Hirano, S., Kuboki, H., Kasai, M., and Hatae, K. (2005). Effects of dried bonito (katsuobushi) and captopril, an angiotensin I-converting enzyme inhibitor, on rat isolated aorta: A possible mechanism of antihypertensive action. Biosci. Biotechnol. Biochem. 69 (5), 911–915. doi:10.1271/bbb.69.911
Matsumoto, J., Enami, K., Doi, M., Kishida, T., and Ebihara, K. (2007). Hypocholesterolemic effect of katsuobushi, smoke-dried bonito, prevents ovarian hormone deficiency-induced hypercholesterolemia. J. Nutr. Sci. Vitaminol. (Tokyo). 53 (3), 225–231. doi:10.3177/jnsv.53.225
Muto, J., Lee, H., Lee, H., Uwaya, A., Park, J., Nakajima, S., et al. (2014). Oral administration of inosine produces antidepressant-like effects in mice. Sci. Rep. 4, 4199. doi:10.1038/srep04199
Oikawa, S., Kai, Y., Mano, A., Nakamura, S., and Kakinuma, Y. (2019a). A novel nitric oxide donor, S-nitroso-N-pivaloyl-D-penicillamine, activates a non-neuronal cardiac cholinergic system to synthesize acetylcholine and augments cardiac function. Cell. Physiol. biochem. 52 (4), 922–934. doi:10.33594/000000064
Oikawa, S., Kai, Y., Mano, A., Sugama, S., Mizoguchi, N., Tsuda, M., et al. (2019b). Potentiating a non-neuronal cardiac cholinergic system reinforces the functional integrity of the blood brain barrier associated with systemic anti-inflammatory responses. Brain Behav. Immun. 81, 122–137. doi:10.1016/j.bbi.2019.06.005
Oikawa, S., Kai, Y., Mano, A., Ohata, H., Kurabayashi, A., Tsuda, M., et al. (2021). Non-neuronal cardiac acetylcholine system playing indispensable roles in cardiac homeostasis confers resiliency to the heart. J. Physiol. Sci. 71 (1), 2. doi:10.1186/s12576-020-00787-6
Oikawa, S., Kai, Y., Tsuda, M., Ohata, H., Mano, A., Mizoguchi, N., et al. (2016). Non-neuronal cardiac cholinergic system influences CNS via the vagus nerve to acquire a stress-refractory propensity. Clin. Sci. (Lond). 130 (21), 1913–1928. doi:10.1042/CS20160277
Oikawa, S., Mano, A., Takahashi, R., and Kakinuma, Y. (2015). Remote ischemic preconditioning with a specialized protocol activates the non-neuronal cardiac cholinergic system and increases ATP content in the heart. Int. Immunopharmacol. 29 (1), 181–184. doi:10.1016/j.intimp.2015.06.004
Rana, O. R., Schauerte, P., Kluttig, R., Schröder, J. W., Koenen, R. R., Weber, C., et al. (2010). Acetylcholine as an age-dependent non-neuronal source in the heart. Aut. Neurosci. 156 (1-2), 82–89. doi:10.1016/j.autneu.2010.04.011
Rocha-Resende, C., Roy, A., Resende, R., Ladeira, M. S., Lara, A., de Morais Gomes, E. R., et al. (2012). Non-neuronal cholinergic machinery present in cardiomyocytes offsets hypertrophic signals. J. Mol. Cell. Cardiol. 53 (2), 206–216. doi:10.1016/j.yjmcc.2012.05.003
Rocha-Resende, C., Weinheimer, C., Bajpai, G., Adamo, L., Matkovich, S. J., Schilling, J., et al. (2019). Immunomodulatory role of non-neuronal cholinergic signaling in myocardial injury. JCI Insight 5 (14), e128961. doi:10.1172/jci.insight.128961
Rosas-Ballina, M., and Tracey, K. J. (2009). Cholinergic control of inflammation. J. Intern Med. 265 (6), 663–679. doi:10.1111/j.1365-2796.2009.02098.x
Roy, A., Dakroub, M., Tezini, G. C., Liu, Y., Guatimosim, S., Feng, Q., et al. (2016). Cardiac acetylcholine inhibits ventricular remodeling and dysfunction under pathologic conditions. FASEB J. 30 (2), 688–701. doi:10.1096/fj.15-277046
Roy, A., Fields, W. C., Rocha-Resende, C., Resende, R. R., Guatimosim, S., Prado, V. F., et al. (2013). Cardiomyocyte-secreted acetylcholine is required for maintenance of homeostasis in the heart. FASEB J. 27 (12), 5072–5082. doi:10.1096/fj.13-238279
Saw, E. L., Pearson, J. T., Schwenke, D. O., Munasinghe, P. E., Tsuchimochi, H., Rawal, S., et al. (2021). Activation of the cardiac non-neuronal cholinergic system prevents the development of diabetes-associated cardiovascular complications. Cardiovasc. Diabetol. 20 (1), 50. doi:10.1186/s12933-021-01231-8
Takenouchi, T., Ogihara, K., Sato, M., and Kitani, H. (2005). Inhibitory effects of U73122 and U73343 on Ca2+ influx and pore formation induced by the activation of P2X7 nucleotide receptors in mouse microglial cell line. Biochimica Biophysica Acta - General Subj. 1726 (2), 177–186. doi:10.1016/j.bbagen.2005.08.001
Umeki, Y., Hayabuchi, H., Hisano, M., Kuroda, M., Honda, M., Ando, B., et al. (2008). The effect of the dried-bonito broth on blood pressure, 8-Hydroxydeoxyguanosine (8-OHdG), an oxidative stress marker, and emotional states in elderly subjects. J. Clin. Biochem. Nutr. 43 (3), 175–184. doi:10.3164/jcbn.2008061
Keywords: katsuo extract, acetylcholine, non-neuronal cardiac cholinergic system, anti-inflammation, blood brain barrier, higher brain function
Citation: Hokari Y, Sekine A, Kai Y, Oikawa S, Mano A, Ohata H, Sugama S and Kakinuma Y (2022) Katsuo extract derived from dried bonito plays a role in systemic anti-inflammation and consolidation of the blood-brain barrier to regulate higher brain functions. Front. Nat. Prod. 1:969433. doi: 10.3389/fntpr.2022.969433
Received: 15 June 2022; Accepted: 17 August 2022;
Published: 29 September 2022.
Edited by:
Eng Shi Ong, Singapore University of Technology and Design, SingaporeReviewed by:
Benita Wiatrak, Wroclaw Medical University, PolandMing Yuan Heng, Technology and Research, Singapore
Copyright © 2022 Hokari, Sekine, Kai, Oikawa, Mano, Ohata, Sugama and Kakinuma. This is an open-access article distributed under the terms of the Creative Commons Attribution License (CC BY). The use, distribution or reproduction in other forums is permitted, provided the original author(s) and the copyright owner(s) are credited and that the original publication in this journal is cited, in accordance with accepted academic practice. No use, distribution or reproduction is permitted which does not comply with these terms.
*Correspondence: Yoshihiko Kakinuma, k12417853@nms.ac.jp