- 1Special Centre for Nano Sciences and AIRF, Jawaharlal Nehru University, New Delhi, India
- 2Gitanjali Patho Diagnostics, Vivekananda Marg, Patna, India
- 3CSIR-National Physical Laboratory, Dr. K. S. Krishnan Marg, New Delhi, India
Infectious microbial diseases are leading causes of morbidity and mortality worldwide. Further, emergence of Multidrug resistance (MDR) in microbes has posed a critical concern for public healthcare and microbial therapeutics. Nano-based drug compositions might be ideal candidates to address the challenges of microbial drug resistance. Herein, we synthesized monodispersed, spherical ZnO quantum dots (QDs) of average diameter 5–6 nm as biosafe, nanomicrobicidal agent against MDR pathogens. The broad spectrum microbicidal potential of ZnO QDs was evaluated against Extended Spectrum Beta Lactamase (ESBL) producing MDR isolates of E. coli (from UTI patients with resistance to antibiotics of different classes viz. third & fourth generation of cephalosporin, penicillin, monobactams, and quinolones) and MDR isolates of C. albicans (from three different AIDS patients who during therapy acquired drug resistance and sensitive isolates evolved into MDR strains). ZnO QDs exhibited dose dependent, broad spectrum microbicidal activity against all MDR isolates of E. coli and C. albicans. Highly reduced growth indicating highly reduced cell viability was observed in all MDR isolates of E. coli and C. albicans at minimum inhibitory concentration (MIC) of 200 μg/ ml ZnO QDs and the growth/ cell viability was further reduced in presence of 250 and 400 μg/ ml of ZnO QDs for E. coli and C. albicans, respectively. To the best of our knowledge, microbicidal potential of ZnO QDs against microbial strains exhibiting MDR for currently used drugs has not been studied. Results of present study indicate that ZnO QDs might be promising as next generation broad spectrum alternative for combating MDR in microbial pathogens.
Introduction
Infectious microbial diseases remain the leading cause of human mortality worldwide (Bloom and Cadarette, 2019). Among the diseases caused by various microbial species viz. bacteria, viruses, fungi, or parasites, most frequently encountered are bacterial infections such as diarrhea, cholecystitis, bacteraemia, cholangitis, urinary tract infections (UTI), meningitis, and pneumonia (Zhou et al., 2018). World Health Organization (WHO) reported millions of global deaths in 2016 due to diarrhoeal diseases, lower respiratory infections and tuberculosis (Global Health Estimates, 2016). One of the most common bacterial pathogens particularly responsible for UTI, sepsis/ meningitis and enteric/ diarrhoeal diseases is Escherichia coli (E. coli) (Allocati et al., 2013; Saeed et al., 2015). Of these, UTI is the second most common community-acquired infections and commonly treated by drugs such as penicillins, cepahlosporins (includes third and fourth generation agents), trimethoprim/ sulfamethoxazole, and quinolones (Picozzi et al., 2014). It has been observed that UTI is frequently caused by Extended Spectrum Beta Lactamase (ESBL) producing E. coli, which often acquires resistance to all penicillins, cephalosporins, and cross-resistance to trimethoprim/ sulfamethoxazole and quinolones (Picozzi et al., 2014). Other than bacterial infections, fungal infections also are life threatening and responsible for 1.5–2 million deaths annually (Denning and Bromley, 2015). As per Global Action Fund for Fungal Infections (GAFFI), more than 300 million people of all ages suffer from serious fungal diseases, but still fungal infections remain the most neglected among microbial infections (Rodrigues and Nosanchuk, 2020). Common fungal infections such as oral or vaginal candidiasis and life-threatening systemic infections (systemic candidiasis) are caused by Candida albicans (C. albicans) and around 400,000 cases of Candida bloodstream infections are annually recorded worldwide (Pal, 2017). C. albicans normally resides as commensal microbial flora of the human gastrointestinal (GI) tract but turns pathogenic in immunocompromised individuals (Mayer et al., 2013). The most prevalent and recurrent fungal infection in HIV-infected patients caused by C. albicans is oropharyngeal candidiasis (OPC) (Vazquez, 2010). Prolonged azole drug therapy for OPC often leads to the emergence of drug resistance in C. albicans and has emerged as a recognized problem in AIDS patients (Patil et al., 2018).
Various antimicrobial drugs are available for the treatment of microbial infections and each class of drug has a particular mode of action and definite cellular target such as cell wall, membrane lipids, translational machinery, and DNA replication (Liwa and Jaka, 2015; Silver, 2016). The extensive deployment and indiscriminate use of these drugs lead to the emergence of multi drug resistance (MDR) in microbial pathogens wherein microbes evolve with adaptive strategies either to subvert or evade host immune responses or to counter the antibiotics. The primary adaptive strategies of the microbes include drug target modifications, drug degradation, and overexpression of drug efflux pumps (Munita and Arias, 2016). Increasing incidence of microbial MDR is a critical issue in public healthcare and microbial therapeutics. The continuous evolution of drug resistant microbial strains therefore, constantly demands for new and safe antimicrobial agents with broad spectrum activity and minimal host toxicity.
Recent advances in nanotechnology have led to the inception of Nanomedicine with a promise for improved drug delivery, imaging, medical implants, antimicrobials, biosensing platforms for detection of microbial diseases, cancer therapeutics etc. (Nune et al., 2009; Holzinger et al., 2014; Singh et al., 2014; Zhu and Liao, 2015). In order to improve the treatment outcome of microbial infections, nano-based drug compositions might be ideal candidates to address the challenges of microbial drug resistance by favoring multi targeted action, broad spectrum activity and lower host toxicity (Singh et al., 2014). Several metal and metal oxide nanoparticles such as silver, gold, titanium oxide, and zinc oxide are known to possess antimicrobial properties (Singh et al., 2014; Gold et al., 2018). Among various nanoparticles, Zinc oxide (ZnO) nanoparticles maybe a novel antimicrobial agent of choice because of its non-toxic nature as compared to other competitive nanomaterials; further it can also act as micronutrient for regulation of innate and adaptive immune responses (Roy et al., 2014). Although, studies are available on antimicrobial activity of nano ZnO (Joshi et al., 2009; Xie et al., 2011; Ansari et al., 2012; Wahab et al., 2014; Hameed et al., 2016; Farzana et al., 2017; Duffy et al., 2018; Gupta et al., 2018; Hosseini et al., 2018; Jalal et al., 2018), no study so far has reported the antimicrobial activity of ZnO quantum dots (QDs) against MDR pathogens.
In order to develop a biosafe, nano-based therapeutic microbicidal agent, we investigated the broad spectrum microbicidal activity of ZnO QDs on MDR isolates of microbial pathogens (E. coli and C. albicans). The ZnO QDs were synthesized using wet chemical route and their microbicidal potential were demonstrated against ESBL producing MDR isolates of E. coli (with resistance to most of the currently used antibacterial drugs of different classes such as third & fourth generation of cephalosporin, penicillin, monobactams and quinolones) and MDR isolates of C. albicans (MDR strains evolved from sensitive strains by acquiring resistance). ZnO QDs appear as promising new-generation broad spectrum microbicidal agent to combat MDR pathogens.
Materials and Methods
Materials
For synthesis of ZnO QDs, zinc acetate dihydrate and lithium hydroxide monohydrate were procured from Sigma Aldrich (MO, USA), absolute ethanol from (Alfa Aesar, MA, USA) and hexane from Merck (NJ, USA). Media chemicals for culture of the microbial cells namely, Luria Broth from BD Difco (TX, USA), yeast extract, peptone and glucose were obtained from Fisher Scientific (Hampton, NH, USA) and agar from Hi-Media (Mumbai, India).
Microbial Strains
Bacterial Strains
Wild type Escherichia coli (DH5α) (Monk et al., 2016) and ESBL producing isolates of E. coli (16, 36, 105, and 153) were used in this study as representatives of bacterial pathogens. The E. coli isolates (16, 36, 105, and 153) were from Pathological and Diagnostics Laboratory (Gitanjali Patho Diagnostics Laboratory, Patna, India). Antibiotic susceptibility testing of the E. coli isolates was performed in accordance with the recommendations of Clinical Laboratory Standard Institute (CLSI) using Kirby-Bauer Method (Pouladfar et al., 2017). Kirby-Bauer Method is based on agar disc diffusion assay and is a widely used method for antimicrobial susceptibility testing. Herein, the filter paper discs impregnated with antimicrobial agents are applied onto the agar surface already inoculated with test microbe. On incubation, the antimicrobials diffuse out from the disc into the agar creating a concentration gradient and the area of no microbial growth around the disc is called zone of inhibition. The Antibiogram for the isolates of E. coli used in this study was performed using proper quality control protocols on Muller-Hinton agar disc diffusion plate and is shown in Table 1.
Fungal Strains
Wild type Candida albicans (SC5314) and three isogenic matched pairs of isolates (G1 & G5, F1 & F5, and GU4 & GU5) of C. albicans, used in this study are listed in Table 2 and the strain descriptions are already reported in many studies previously (Fonzi and Irwin, 1993; Franz et al., 1998, 1999). These three isogenic matched pairs of strains were provided by Joachim Morschhauser, University of Wurzburg, Germany and originally isolated from three different AIDS patient suffering from recurrent episodes of OPC. The azole therapy given over a period of time to these AIDS patients for OPC resulted in the acquisition of MDR by the sensitive strains and subsequent evolution of the azole sensitive G1, F1, and GU4 to highly resistant G5, F5, and GU5, respectively (Franz et al., 1998, 1999).
Growth Media and Culture Conditions
Wild type Escherichia coli (DH5α) and ESBL producing isolates of E. coli (16, 36, 105, and 153) were grown at 37°C in Luria Broth (LB) media for 12 hrs under continuous shaking at 140–150 rpm and these exponentially growing log phase cells were used for all experiments. Wild type SC5314 and drug sensitive-resistant isogenic strains (G1 & G5, F1 & F5, and GU4 & GU5) were grown on 1% (w/v) Yeast extract, 2% (w/v) Peptone and 2% (w/v) Dextrose (YEPD) media. The cells were grown at 30°C with continuous shaking at 140–150 rpm for 14–16 h and these exponentially growing cells were used for all experiments.
Synthesis of ZnO QDs
ZnO QDs were synthesized by wet chemical route (Spanhel and Anderson, 1991). A 0.1 M ethanolic solution of zinc acetate dihydrate was prepared in absolute ethanol at 90°C under continuous refluxing for complete dissolution, then cooled at room temperature and this solution was designated as Sol A. In a separate beaker 0.14 M ethanolic solution of lithium hydroxide monohydrate was prepared in absolute ethanol at room temperature using sonication. This solution was designated as Sol B. Sol A and Sol B were mixed at room temperature (25°C) under vigorous stirring for 1 h to form ZnO QDs. The QDs were precipitated using hexane and centrifuged to collect the particles. These QDs were dried at 60°C under vacuum. The obtained powder was characterized for their structural functional and optical properties using following techniques. A stock solution of ZnO QDs in deionized water (milli Q) was prepared for all the experiments.
Characterization of ZnO QDs
The structural aspects of QDs were studied using X-ray powder diffractometer (PANalyticalX'Pert PRO diffractometer, Almelo Netherlands) with Cu Kα radiation (λ = 1.5418 Å) in the scan range of 20°–80° diffraction angle and step size of 0.016°. The optical property, specifically excitonic absorbance of the ZnO QDs was analyzed using UV-Vis spectrophotometer (Perkin Elmer, UV-Vis Spectrophotometer Lambda 35, Singapore). The morphology and chemical composition of ZnO QDs were examined by Scanning Electron Microscopy (SEM) (Carl Zeiss E®AG-EVO®40 SEM, Jena, Germany) equipped with EDX Spectrometer (EDS; E-sprit 1.8 X-ray microanalysis, Quantax 200; Bruker Nano GmbH, Berlin, Germany). Further, a 200 kV Transmission Electron Microscope (TEM) (JEM 2100F; JEOL, Tokyo, Japan) having Image Tool Software (multipoint image database software for grain and particle analysis; Dietermann & Heuser Solution GmbH, Greifenstein, Germany) was used to determine mean particle size. High resolution TEM (HRTEM) analysis was used to visualize planar lattice spacing (d spacing) to ascertain the crystalline nature.
Assessment of Microbicidal Potential of ZnO QDs
Minimum Inhibitory Concentration (MIC) is the lowest concentration of an antimicrobial agent necessary to inhibit visible microbial growth, while minimum microbicidal concentration is the minimum concentration of an antimicrobial agent that results in microbial death. The closer the MIC is to the MBC, the more microbicidal the compound is. MIC is an endpoint measurement and represents microbial growth in the presence of antimicrobial agent and thus, the static/cidal effects of the antimicrobial agent over time and is employed both as the criteria for determination of susceptibility/ resistance/ static or cidal effect of compounds and also as the single, quantitative pharmacodynamic (PD) parameter formally used for the rational design of antibiotic treatment protocols.
In this study, the MIC and microbicidal activity of the ZnO QDs against the microbial pathogens E. coli and C. albicans were determined using Internationally accepted methods viz. Broth Microdilution Assays (Clinical and Laboratory Standards Institute, 2006, 2008; Holla et al., 2012; Preeti et al., 2020) and Spot Assays (Clinical and Laboratory Standards Institute, 2006; Tong, 2012; Thomas et al., 2015; Wang et al., 2017; Radhakrishnan et al., 2018b).
In broth microdilution assay, the antimicrobial agent is serially diluted in multiple tubes or wells of microtitre plate from very high to low concentrations to obtain a gradient. Here, 5 × 105 CFU/ ml and 0.5–1 × 104 CFU/ ml are standard inoculum for antimicrobial susceptibility testing of bacterial and fungal pathogens, respectively. This assay is used to determine the percentage of cell killing and growth inhibition at the respective concentration of the antimicrobial agent. On the other hand, the spot assay is a sensitive method which involves serial dilutions of fixed number of microbial cells as per standard protocol, followed by spotting of these dilutions of microbial cells on solid agar containing plates at concentrations of antimicrobial agents determined by broth microdilution assay. Spot assay is used to compare the viability of cultivable microbial cells under different conditions.
Broth Microdilution Assay
MIC of ZnO QDs for E. coli (DH5α) and C. albicans (SC5314) was determined by broth microdilution assay, which was performed in 96 well microplate, following the protocol described by CLSI (Clinical and Laboratory Standards Institute, 2006, 2008; Oh et al., 2012; Preeti et al., 2020). The exponentially growing log phase cells of E. coli and C. albicans were resuspended in 0.9% saline to give an optical density of 0.1 at 600 nm (OD600), which correspond to cell number of 5 × 107 CFU/ ml for E. coli and 0.5–1 × 106 CFU/ ml for C. albicans. The cells were subsequently diluted 100-fold in LB and YEPD media, respectively for E. coli and C. albicans to achieve the final concentration of cells 5 × 105 and 0.5–1 × 104 CFU/ ml. Thereafter, different concentrations of ZnO QDs (0–400 μg/ ml) were added to the respective media containing cells and incubated at 37°C for E. coli and at 30°C for C. albicans. Respective controls for growth of the microbial cells were maintained without ZnO QDs. Readings were then recorded at 600 nm and the differences in optical densities were compared with the control grown without ZnO QDs.
Spot Assay
Spot assay was used to confirm the susceptibility of wild type, drug sensitive and resistant isolates of E. coli and C. albicans toward ZnO QDs (Mukhopadhyay et al., 2004; Clinical and Laboratory Standards Institute, 2006; Thomas et al., 2015; Wang et al., 2017; Radhakrishnan et al., 2018b).
Overnight grown microbial strains of E. coli and C. albicans were resuspended in 0.9% saline and the OD600 of the cell suspension was first adjusted to 0.1 and CFU/ ml was calculated. Then, 5-fold serial dilutions of cell suspension containing 5 × 105 cells/ ml of E. coli and 1 × 106 cells/ ml of C. albicans were prepared. Subsequently, 5 μl of each serially diluted cell suspension were spotted onto LB plates (containing 0, 200, and 250 μg/ ml of ZnO QDs) for bacterial and YEPD plates (containing 0, 200 and 400 μg/ ml of ZnO QDs) for fungal strains, respectively. The LB plates were incubated for E. coli at 37°C for 24 h and for C. albicans at 30°C for 48 h. Growth controls without ZnO QDs were maintained for both E. coli and C. albicans separately. The plates were then imaged and reduction in cell viability in presence of ZnO QDs was evaluated by comparing with the control grown without ZnO QDs.
Statistical Analysis
All experiments were performed in triplicates and the results were represented as mean ± standard deviation. GraphPad Prism version 6.0 (GraphPad software, CA) was used to calculate p-value in Student t-test and for validating reproducibility and significance of experiments. Intergroup differences were considered significant for p < 0.05.
Results and Discussion
Physicochemical Properties of ZnO QDs
The crystalline structure of synthesized ZnO QDs was evaluated using X-Ray diffraction (XRD) technique by recording the diffraction pattern between 20 and 80° (Figure 1A). The XRD of ZnO QDs displayed Braggs reflections at 32.01, 34.48, 36.50, 47.71, 56.87, 62.99, and 68.34° corresponding to the planes (100), (002), (101), (102), (110), (103), and (112), respectively and confirmed the formation of single phase hexagonal wurtzite structure of QDs (Figure 1A). The diffraction planes were in accordance with JCPDS (Joint committee on powder diffraction standards) file no. 36–1451. No other characteristic XRD peaks were observed, indicating the phase purity of the synthesized ZnO QDs. The lattice spacing d(101) was found to be 0.245 nm, which was in agreement with the wurtzite ZnO nanoparticles (NPs) (Siddiqi et al., 2018). UV-Visible absorption spectrum (Figure 1B) exhibited the absorption maxima at 360 nm, which corresponded to enhanced band gap of 3.4 eV as a result of smaller size of the ZnO QDs. SEM micrographs revealed formation of spherical ZnO QDs with smooth surface (Figure 2A). Presence of strong peaks in the EDS spectrum were observed at around 1 keV from the Zn L lines, at 8.601 keV from Zn Ka, at 9.5 keV from Zn Kb line and at around 0.5 keV from O Ka (Figure 2B). EDS spectrum indicated that the elements present were only Zinc and Oxygen. TEM image confirmed synthesis of mono-dispersed and spherical ZnO QDs of average size ~5–6 nm which was in agreement with XRD results (Figure 2C). HRTEM clearly revealed the lattice structure and the average distance (d) between atomic planes of ZnO QDs as 0.25 nm (Figure 2D), which corroborated with XRD data.
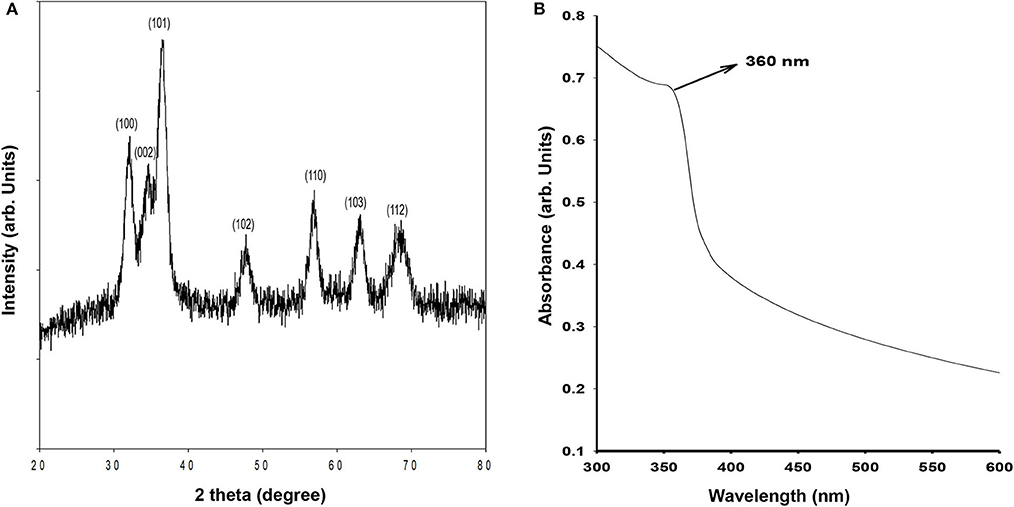
Figure 1. (A) X-ray diffraction of ZnO QDs; (B) Absorbance spectrum for ZnO QDs showing absorbance maxima at 360 nm.
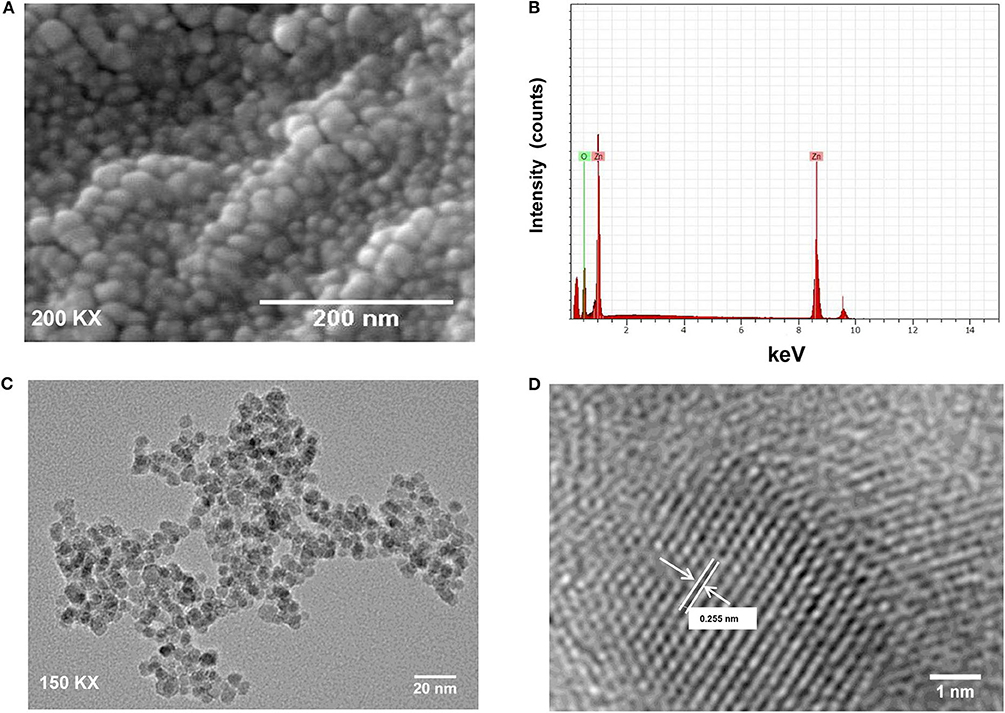
Figure 2. (A) SEM image of ZnO QDs; (B) SEM-EDS spectrum for ZnO QDs with strong peaks at 0.1, 8.6, and 0.5 keV; (C) TEM image showing spherical, mono-dispersed ZnO QDs of average size 5–6 nm; (D) High-resolution TEM image for ZnO QDs showing lattice spacing (d = 0.255 nm, where d represents the distance between two lattice fringes).
Monodispersed, spherical ZnO QDs with average diameter 5–6 nm were thus synthesized, which exhibited hexagonal wurtzite structure and showed typical absorption peak at 360 nm corresponding to a band gap of 3.4 eV.
Microbicidal Potential of ZnO QDs
ZnO QDs showed dose dependent toxicity against wild type strains of E. coli and C. albicans, used as model microbial pathogens in this study. Here in this study, broth microdilution assay performed in presence of different concentrations of ZnO QDs (0–400 μg/ ml) revealed that the percentage of growth inhibition at 100 μg/ ml ZnO QDs was 44.76% for E. coli and 53.89% for C. albicans cells whereas 200 μg/ ml ZnO QDs was able to inhibit 70.87% (MIC70) growth of E. coli and 90.57% (MIC90) growth of C. albicans cells (Figures 3A,B). Thus, MIC70 and MIC90 of ZnO QDs were found to be 200 μg/ ml for E. coli and C. albicans, respectively, as determined by the reduction in respective optical densities measured at 600 nm (Optical density of 0.1 at 600 nm corresponds to 5 × 107 CFU/ ml for E. coli and 0.5–1 × 106 CFU/ ml for C. albicans). Figure 3 represents the optical density of microbial cells grown in presence of ZnO QDs at MIC concentration and at half the MIC concentration. The data represented was reproducible in least three independent set of experiments.
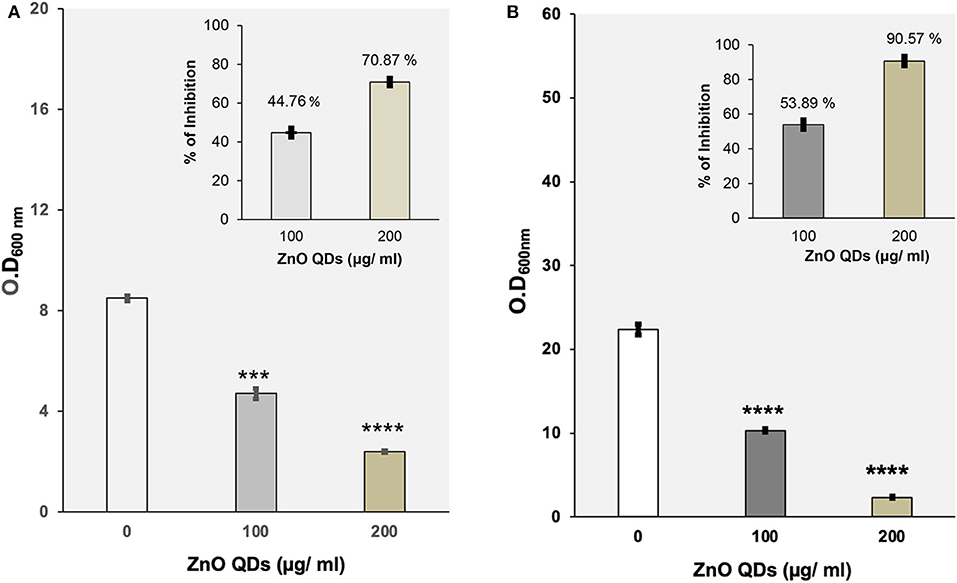
Figure 3. (A) Evaluation of antibacterial potential of ZnO QDs. Determination of growth by measurement of OD600nm using broth microdilution method for E. coli cells grown at 37°C in the presence of 0, 100, and 200 μg/ ml of ZnO QDs, respectively. The values are mean ± SD of three independent sets of experiments. ***represents p = 0.001 and ****represents p < 0.0001, calculated with respect to control. Inset shows the percentage of dose dependent growth inhibition of E. coli cells in presence of respective concentrations of ZnO QDs; (B) Evaluation of antifungal activity of ZnO QDs. Determination of growth by measurement of OD600nm using broth microdilution method for C. albicans cells grown at 30°C in the presence of 0, 100, and 200 μg/ ml of ZnO QDs, respectively. The values are mean ± SD of three independent sets of experiments. ****represents p < 0.0001, calculated with respect to control. Inset shows the percentage of dose dependent growth inhibition of C. albicans cells in presence of respective concentrations of ZnO QDs.
Interestingly, a recent study reported that smaller size ZnO QDs exhibited better bactericidal activity than larger ZnO NPs (da Silva et al., 2019). This was in agreement with our results wherein we observed 70 and 90% reduction in E. coli and C. albicans cells, respectively at MIC70 and MIC90 values of 200 μg/ ml ZnO QDs, demonstrating promising broad spectrum microbicidal potential of smaller sized 5–6 nm ZnO QDs against both bacterial and fungal pathogens.
Microbicidal Potential of ZnO QDs Against MDR Isolates of E. coli and C. albicans
Microbicidal activity of ZnO QDs was evaluated against MDR isolates of E. coli and C. albicans. Antibiotic susceptibility of ESBLs producing E. coli (16, 36, 105, and 153) revealed their resistance toward penicillins, cepahlosporins and cross-resistance to quinolones (Table 1). ESBL producing MDR isolates of E. coli acquire resistance to β-lactam antibiotics such as penicillin derivatives (penams), cephalosporins (cephems), monobactams, carbapenems, and carbacephems etc. through degradation of β-lactam rings of the antibiotics by production of extended spectrum beta lactamase (Shaikh et al., 2015). The three isogenic matched pairs (G1 & G5, F1 & F5, and GU4 & GU5) were originally collected from three different AIDS patient suffering from OPC (Franz et al., 1998, 1999). Due to continuous administration of high doses of azoles for recurrent episodes of OPC, the azole sensitive G1, F1, and GU4 acquired resistance during therapy and evolved to become highly azole resistant G5, F5, and GU5, respectively (Table 2). The isolates of Candida used in this study were reported to acquire resistance due to increase in amount of cellular ergosterol and overexpression of drug efflux pumps (Franz et al., 1998, 1999).
The microbicidal activity of ZnO QDs was further confirmed by spot assay. ZnO QDs exhibited dose dependent, broad spectrum microbicidal activity against all MDR isolates of E. coli and C. albicans tested (Figures 4, 5). Here it clearly showed significantly reduced cell viabilities for MDR strains of both microbial pathogens in comparison to the control plate (without ZnO QDs), which was indicated by the disappearance of cells on agar plates containing ZnO QDs. No such microbicidal effect or reduction in cell viability was observed on respective Luria Bertani (LB) and Yeast Extract, Peptone and Dextrose (YEPD) control plates, maintained without ZnO QDs. Spot assay involves spotting of dilutions of microbial cells at concentrations of antimicrobial agents determined by broth microdilution assay. In this study, the spot assay was performed at different concentrations between MIC values and four times MIC values. Of these, the best results for spot assay obtained at two concentrations (i.e., MIC concentration and the next higher concentration are represented in Figures 4, 5, to demonstrate significant loss of cell viability which is indicated by the significant disappearance of cells in presence of ZnO QDs). Studies evidence that microbicidal concentration is usually either equal to/ 2-fold/ 4-fold > MIC values (Microbicidal concentration ≥ 1x or 2x or 4x MIC) (Morrissey et al., 2014). This validated our results that highly reduced cell viability was observed for MDR isolates of both E. coli and C. albicans cells at MIC concentration of 200 μg/ ml ZnO QDs, which was further reduced in presence of 250 and 400 μg/ ml of ZnO QDs for E. coli and C. albicans, respectively (Figures 4, 5). Complete growth inhibition was observed at 4x MIC70 of ZnO QDs for E. coli and at 4x MIC90 of ZnO QDs for C. albicans cells, thus confirming the microbicidal potential of ZnO QDs (data not shown). The data represented was reproducible in at least three independent set of experiments. Therefore, our spot assay results demonstrated that ZnO QDs were able to counter MDR in microbial isolates tested and could render susceptibility to the drug resistant microbials cells.
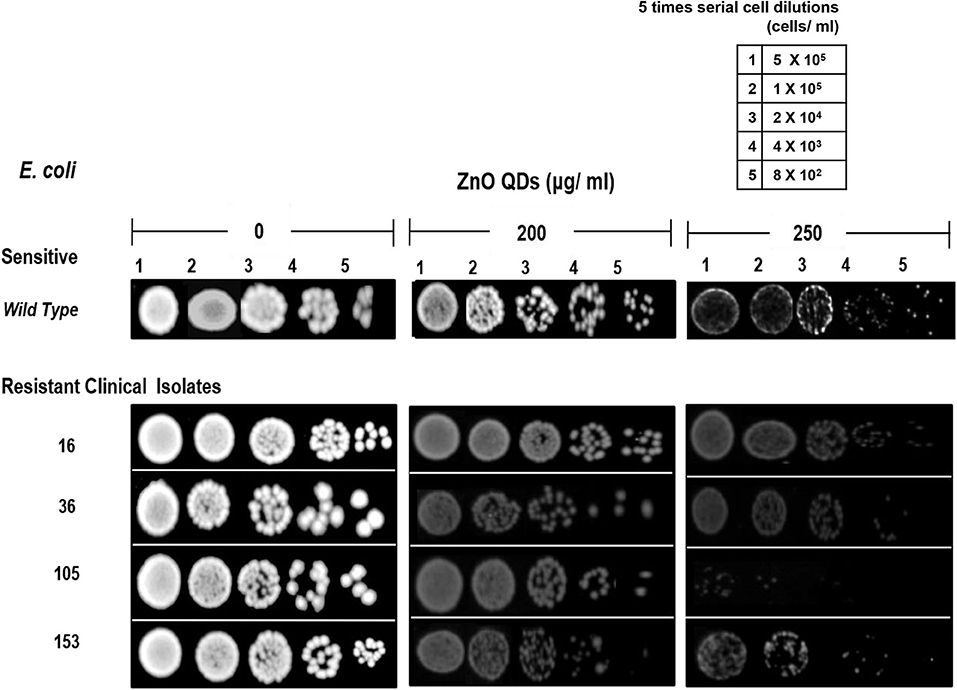
Figure 4. Antimicrobial activity of ZnO QDs against MDR strains of E. coli. Spot assay showing of ZnO QDs against MDR isolates (16, 36, 105, and 153) of E. coli in the presence of 0, 200, and 250 μg/ ml of ZnO QDs.
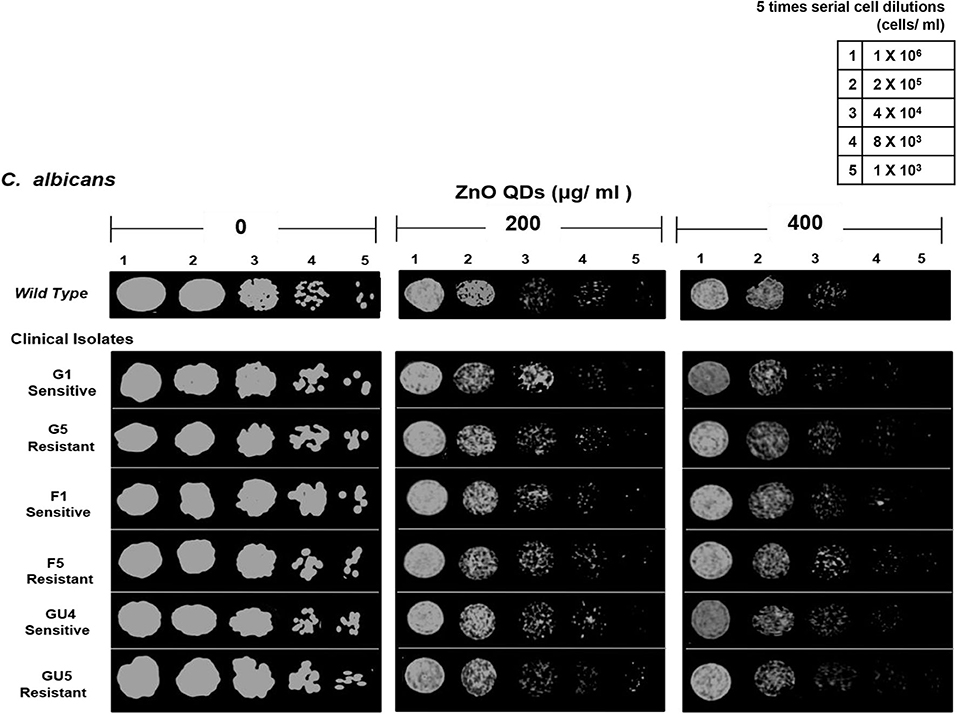
Figure 5. Antimicrobial activity of ZnO QDs against azoles resistant C. albicans. Spot assay showing anti-Candidal activity of ZnO QDs against azoles sensitive (G1, F1, and GU4) and azoles resistant (G5, F5, and GU5) isolates in the presence of 0, 200, and 400 μg/ ml of ZnO QDs.
Several studies have documented size dependent microbial toxicity of ZnO NPs (Sirelkhatim et al., 2015). In our study, the synthesized 5–6 nm ZnO QDs exhibited significant microbicidal activity against both drug sensitive and resistant isolates of E. coli and C. albicans. The small size of ZnO QDs might be responsible for this promising microbicidal activity. It is possible that antimicrobial or microbicidal activity of metal oxide NPs results from the interaction of metal ions (resulting from dissolution of metals ions from the surface of NPs) with cells, which lead to reactive oxygen species (ROS) generated oxidative stress. The dissolution of metal and metal oxide nanoparticles remains highly dependent on NPs type, concentration, size, coatings and pH of aqueous media. Higher dissolution of Zn2+ ions was reported from 4 nm ZnO QDs than larger NPs, resulting in presence of more Zn2+ ions in ZnO QDs solution (Mudunkotuwa et al., 2012), which in turn might be responsible for the observed higher antimicrobial activity of QDs against both drug susceptible and resistant pathogens (Siddiqi et al., 2018). Moreover, the quantum confinement of ZnO QDs creates surface defects on ZnO QDs and produces large number of electron donor or acceptor active sites, which react with oxygen and hydroxyl ions (in aqueous suspension) to produce highly reactive superoxide and hydroxyl radicals. These highly reactive free radicals contribute to enhanced ROS generation and result in peroxidation of lipids, nucleic acid and proteins, disruption of cell membrane and leakage of cytoplasmic content, thus leading to cell death (Asok et al., 2015; Gold et al., 2018; Radhakrishnan et al., 2018a; Tiwari et al., 2018).
In addition, the small size of ZnO QDs also provide larger surface area for their higher interaction with microbial cells. Furthermore, the surface defects of ZnO QDs might also increase the density of positive surface charge as particle size decreases, which might increase interaction with microbial cells (possessing negative charge). This can lead to higher agglomeration of ZnO QDs on the surface of microbes leading to altered metabolic activity and increased antimicrobial activity (Asok et al., 2015). Thus, small size of ZnO QDs might exert excellent antimicrobial activity through multiple mechanisms.
However, the major limitation of microbial therapeutics is that each class of antimicrobial drug has a particular mode of action and definite cellular target such as cell wall, membrane lipids, translational machinery, or DNA replication (Liwa and Jaka, 2015; Silver, 2016). For example, the mode of action of β-lactam antibiotics is through inhibition of bacterial cell wall biosynthesis and that of antifungal azoles is via inhibition of fungal membrane ergosterol biosynthetic pathway (Prasad et al., 2011; Shaikh et al., 2015). But on emergence of MDR in microbial pathogens, drug therapy becomes ineffective and poses a bottleneck in microbial therapeutics. MDR being multifactorial, microbial pathogens acquire resistance by various adaptive strategies such as drug target modifications, drug degradation, target amplification, alterations in cell wall, modifications in cell membrane, and overexpression of drug efflux pumps (Shaikh et al., 2015; Munita and Arias, 2016). The challenges faced due to acquisition of microbial drug resistance can likely be overcome by favoring multi-targeted action of antimicrobial agents. In view of the previous reports that interactions of nanoparticles with microbes lead to membrane lipid peroxidation, cell membrane disruption, leakage of cytoplasmic contents, and cell death (Jalal et al., 2018; Radhakrishnan et al., 2018b), it appears that small sized ZnO QDs can address the multifactorial challenges of microbial drug resistance (Graphical illustration in Scheme 1) by favoring multi targeted action, broad spectrum activity and lower host toxicity. In the light of such multi targeted action, it is unlikely for the microbes to simultaneously develop a host of cellular changes or mutations to exhibit resistance.
Conclusion
In this study, monodispersed ZnO QDs of average particle size ~5–6 nm were synthesized using wet chemical route and the broad spectrum, microbicidal activity of ZnO QDs was demonstrated against both drug susceptible and drug resistant isolates of E. coli and Candida albicans. No study so far has reported the antimicrobial activity of ZnO QDs against MDR pathogens. Table 3 gives a comparison of our study with previous studies reported on antimicrobial potential of ZnO NPs/ ZnO QDs against drug susceptible and MDR microbial isolates. In our study, ZnO QDs were found to counter MDR in both bacterial and fungal pathogens tested (ESBL producing MDR isolates of E. coli which showed resistance to most of the currently used antibacterial drugs of different classes such as third and fourth generation of cephalosporins, penicillins, monobactams, and quinolones and MDR isolates of C. albicans collected from three different AIDS patients who during therapy for OPC had acquired drug resistance). The study clearly indicates that ZnO QDs possess broad spectrum microbicidal potential and could serve as a next generation alternative of antimicrobial therapy by combating MDR in microbes and help in limiting the extensive use of antibiotics.
Data Availability Statement
The raw data supporting the conclusions of this article will be made available by the authors, without undue reservation.
Author Contributions
Preeti, SS, and TP contributed to the concept and design of the study. Preeti conducted the experiments. Preeti and VR participated in the acquisition of data and statistical analysis. Preeti, SS, and TP participated in interpretation of data and drafting of the manuscript. SS and TP contributed to editing the manuscript and revised it critically for significant intellectual content. SusM and SujM isolated and performed antibiotic susceptibility profiling of the E. coli strains and completed the antibiogram. All authors read and approved the final manuscript.
Funding
This work has been supported by grants to TP from Department of Biotechnology (BT/PR5110/MED/29/497/2012 and BT/BI/12/045/2008), JNU-UPOE II scheme (ID-161), and JNU-DST-PURSE Phase-II, India.
Conflict of Interest
The authors declare that the research was conducted in the absence of any commercial or financial relationships that could be construed as a potential conflict of interest.
Acknowledgments
JNU for providing infrastructural support and Council of Scientific and Industrial Research (CSIR), India for the award of Junior Research Fellowship to Preeti are gratefully acknowledged. We acknowledge Joachim Morschhauser, University of Wurzburg, Germany for generously providing clinical isogenic isolates of Candida albicans. SS acknowledges CSIR-NPL for providing the nanoparticle synthesis and characterization infrastructure.
References
Allocati, N., Masulli, M., Alexeyev, M. F., and Di-Ilio, C. (2013). Escherichia coli in Europe: an overview. Int. J. Environ. Res. Public Health 10, 6235–6254. doi: 10.3390/ijerph10126235
Ansari, M. A., Khan, H. M., Khan, A. A., Sultan, A., and Azam, A. (2012). Synthesis and characterization of the antibacterial potential of ZnO nanoparticles against extended-spectrum β-lactamases-producing Escherichia coli and Klebsiella pneumoniae isolated from a tertiary care hospital of North India. Appl. Microbiol. Biotechnol. 94, 467–477. doi: 10.1007/s00253-011-3733-1
Asok, A., Ghosh, S., More, P. A., Chopade, B. A., Gandhi, M. N., and Kulkarni, A. R. (2015). Surface defect rich ZnO quantum dots as antioxidants inhibiting α-amylase and α-glucosidase: a potential anti-diabetic nanomedicine. J. Mater. Chem. B 3, 4597–4606. doi: 10.1039/C5TB00407A
Bloom, D. E., and Cadarette, D. (2019). Infectious disease threats in the 21st century: strengthening the global response. Front. Immunol. 10:549. doi: 10.3389/fimmu.2019.00549
Clinical Laboratory Standards Institute (2006). Performance Standards for Antimicrobial Susceptibility Testing; Sixteenth Informational Supplement. CLSI Document M100-S16CLSI. Wayne, PA. Available online at: https://clsi.org/media/1928/m07ed11_sample.pdf
Clinical Laboratory Standards Institute (2008). Reference Method for Broth Dilution Antifungal Susceptibility Testing of Filamentous Fungi; Approved Standard-Second Edition. CLSI document M38-A2. Wayne: Clinical and Laboratory Standards Institute. Available online at: https://clsi.org/media/1455/m38a2_sample.pdf
da Silva, B. L., Caetano, B. L., Chiari-Andréo, B. G., Pietro, R. C. L. R., and Chiavacci, L. A. (2019). Increased antibacterial activity of ZnO nanoparticles: influence of size and surface modification. Colloids Surf. B Biointerf. 177, 440–447. doi: 10.1016/j.colsurfb.2019.02.013
Denning, D. W., and Bromley, M. J. (2015). How to bolster the antifungal pipeline. Science 347, 1414–1416. doi: 10.1126/science.aaa6097
Duffy, L. L., Osmond-McLeod, M. J., Judy, J., and King, T. (2018). Investigation into the antibacterial activity of silver, zinc oxide and copper oxide nanoparticles against poultry-relevant isolates of Salmonella and Campylobacter. Food Control 92, 293–300. doi: 10.1016/j.foodcont.2018.05.008
Farzana, R., Iqra, P., Shafaq, F., Sumaira, S., Zakia, K., Hunaiza, T., et al. (2017). Antimicrobial behaviour of Zinc oxide nanoparticles and β-lactam antibiotics against pathogenic bacteria. Arch. Clin. Microbiol. 8:57. doi: 10.4172/1989-8436.100057
Fonzi, W. A., and Irwin, M. Y. (1993). Isogenic strain construction and gene mapping in Candida albicans. Genetics 134, 717–728.
Franz, R., Kelly, S. L., Lamb, D. C., Kelly, D. E., Ruhnke, M., and Morschhauser, J. (1998). Multiple molecular mechanisms contribute to a stepwise development of fluconazole resistance in clinical Candida albicans strains. Antimicrob. Agents. Chemother. 42, 3065–3072. doi: 10.1128/AAC.42.12.3065
Franz, R., Ruhnke, M., and Morschhauser, J. (1999). Molecular aspects of fluconazole resistance development in Candida albicans. Mycoses 42, 453–458. doi: 10.1046/j.1439-0507.1999.00498.x
Global Health Estimates (2016). Deaths by Cause Age, Sex, Country and by Region, 2000-2016. Geneva: World Health Organization 2018. Available online at: http://www.who.int/healthinfo/global_burden_disease/estimates/en/index1.html
Gold, K., Slay, B., Knackstedt, M., and Gaharwar, A. K. (2018). Antimicrobial activity of metal and metal oxide based nanoparticles. Adv. Ther. 1:1700033. doi: 10.1002/adtp.201700033
Gupta, M., Tomar, R. S., Kaushik, S., Mishra, R. K., and Sharma, D. (2018). Effective antimicrobial activity of green ZnO nanoparticles of Catharanthus roseus. Front. Microbiol. 9:2030. doi: 10.3389/fmicb.2018.02030
Hameed, A. S. H., Karthikeyan, C., Ahamed, A. P., Thajuddin, N., Alharbi, N. S., Alharbi, S. A., et al. (2016). In vitro antibacterial activity of ZnO and Nd doped ZnO nanoparticles against ESBL producing Escherichia coli and Klebsiella pneumoniae. Sci. Rep. 6:24312. doi: 10.1038/srep24312
Holla, G., Yeluri, R., and Munshi, A. K. (2012). Evaluation of minimum inhibitory and minimum bactericidal concentration of nano-silver base inorganic anti-microbial agent (Novaron®) against Streptococcus mutans. Contemp. Clin. Dent. 3, 288–93. doi: 10.4103/0976-237X.103620
Holzinger, M., Le Goff, A., and Cosnier, S. (2014). Nanomaterials for biosensing applications: a review. Front. Chem. 2:63. doi: 10.3389/fchem.2014.00063
Hosseini, S. S., Ghaemi, E., and Koohsar, F. (2018). Influence of ZnO nanoparticles on Candida albicans isolates biofilm formed on the urinary catheter. Iran. J. Microbiol. 10, 424–432.
Jalal, M., Ansari, M. A., Ali, S. G., Khan, H. M., and Rehman, S. (2018). Anticandidal activity of bioinspired ZnO QDs: effect on growth, cell morphology and key virulence attributes of Candida species. Artif. Cells. Nanomed. Biotechnol. 46, 912–925. doi: 10.1080/21691401.2018.1439837
Joshi, P., Chakraborti, S., Chakrabarti, P., Haranath, D., Shanker, V., Ansari, Z. A., et al. (2009). Role of surface adsorbed anionic species in antibacterial activity of ZnO quantum dots against Escherichia coli. J. Nanosci. Nanotechno. 9, 6427–6433. doi: 10.1166/jnn.2009.1584
Liwa, A. C., and Jaka, H. (2015). “Antimicrobial resistance: mechanisms of action of antimicrobial agents,” in The Battle Against Microbial Pathogens: Basic Science, Technological Advances and Educational Programs, ed A. Méndez-Vilas (Badajoz: Formatex Research Center), 876–885.
Mayer, F. L., Wilson, D., and Hube, B. (2013). Candida albicans pathogenicity mechanisms. Virulence 4, 119–128. doi: 10.4161/viru.22913
Monk, J. M., Koza, A., Campodonico, M. A., Machado, D., Seoane, J. M., Palsson, B. O., et al. (2016). Multi-omics quantification of species variation of Escherichia coli links molecular features with strain phenotypes. Cell Syst. 3, 238–251. doi: 10.1016/j.cels.2016.08.013
Morrissey, I., Oggioni, M. R., Knight, D., Curiao, T., Coque, T., Kalkanci, A., et al. (2014). Evaluation of epidemiological cut-off values indicates that biocide resistant subpopulations are uncommon in natural isolates of clinically-relevant microorganisms. PLoS ONE 9:e86669. doi: 10.1371/journal.pone.0086669
Mudunkotuwa, I. A., Rupasinghe, T., Wu, C. M., and Grassian, V. H. (2012). Dissolution of ZnO nanoparticles at circumneutral pH: a study of size effects in the presence and absence of citric acid. Langmuir 28, 396–403. doi: 10.1021/la203542x
Mukhopadhyay, K., Prasad, T., Saini, P., Pucadyil, T. J., Chattopadhyay, A., and Prasad, R. (2004). Membrane sphingolipid–ergosterol interactions are important determinants of multidrug resistance in Candida albicans. Antimicrob. Agents. Chemother. 48, 1778–1787. doi: 10.1128/AAC.48.5.1778-1787.2004
Munita, J. M., and Arias, C. A. (2016). Mechanisms of antibiotic resistance. Microbiol Spectr. 4, 1–37. doi: 10.1128/microbiolspec.VMBF-0016-2015
Nune, S. K., Gunda, P., Thallapally, P. K., Lin, Y. Y., Laird Forrest, M., and Berkland, C. J. (2009). Nanoparticles for biomedical imaging. Expert Opin. Drug Deliv. 6, 1175–1194. doi: 10.1517/17425240903229031
Oh, H. Y., Lee, J. O., and Kim, O. B. (2012). Increase of organic solvent tolerance of Escherichia coli by the deletion of two regulator genes, fadR and marR. Appl. Microbiol. Biotechnol. 96, 1619–1627. doi: 10.1007/s00253-012-4463-8
Pal, M. (2017). Morbidity and mortality due to fungal infections. J. Appl. Microbiol. Biochem. 1:2. doi: 10.21767/2576-1412.100002
Patil, S., Majumdar, B., Sarode, S. C., Sarode, G. S., and Awan, K. H. (2018). Oropharyngeal candidosis in HIV-infected patients-an update. Front. Microbiol. 9:980. doi: 10.3389/fmicb.2018.00980
Picozzi, S. C., Casellato, S., Rossini, M., Paola, G., Tejada, M., Costa, E., et al. (2014). Extended-spectrum beta-lactamase-positive Escherichia coli causing complicated upper urinary tract infection: urologist should act in time. Urol. Ann. 6, 107–12. doi: 10.4103/0974-7796.130536
Pouladfar, G., Basiratnia, M., Anvarinejad, M., Abbasi, P., Amirmoezi, F., and Zare, S. (2017). The antibiotic susceptibility patterns of uropathogens among children with urinary tract infection in Shiraz. Medicine 96:e7834. doi: 10.1097/MD.0000000000007834
Prasad, T., Sethumadhavan, S., and Fatima, Z. (2011). “Altered ergosterol biosynthetic pathway-an alternate multidrug resistance mechanism independent of drug efflux pump in human pathogenic fungi C. albicans,” in Science Against Microbial Pathogens: Communicating Current Research and Technological Advances, Microbiology Series, ed A. Méndez-Vilas (Badajoz: Formatex Research Center), 757–768.
Preeti Prasad, T., Magoo, D., Meena, K., Ghorai, S. M., and Kaur, H. (2020). Elucidation of the antimicrobial capabilities of spirooxindole fused heterocycles synthesized via catalyst free method. Indian J. Nat. Sci. 10, 27323–27330.
Radhakrishnan, V. S., Dwivedi, S. P., Siddiqui, M. H., and Prasad, T. (2018a). In vitro studies on oxidative stress independent, Ag-nanoparticles induced cell toxicity of Candida albicans, an opportunistic pathogen. Int. J. Nanomed. 13, 91–96. doi: 10.2147/IJN.S125010
Radhakrishnan, V. S., Mudiam, M. K. R., Kumar, M., Dwivedi, S. P., Singh, S. P., and Prasad, T. (2018b). Silver nanoparticles induced alterations in multiple cellular targets, which are critical for drug susceptibilities and pathogenicity in fungal pathogen (Candida albicans). Int. J. Nanomed. 13, 2647–2663. doi: 10.2147/IJN.S150648
Rajivgandhi, G., Maruthupandy, M., Muneeswaran, T., Anand, M., and Manoharan, N. (2018). Antibiofilm activity of zinc oxide nanosheets (ZnO NSs) using Nocardiopsis sp. GRG1 (KT235640) against MDR strains of gram negative Proteus mirabilis and Escherichia coli. Process Biochem. 67, 8–18. doi: 10.1016/j.procbio.2018.01.015
Rodrigues, M. L., and Nosanchuk, J. D. (2020). Fungal diseases as neglected pathogens: a wake-up call to public health officials. PLoS Negl. Trop. Dis. 14:e0007964. doi: 10.1371/journal.pntd.0007964
Roy, R., Kumar, S., Verma, A. K., Sharma, A., Chaudhari, B. P., Tripathi, A., et al. (2014). Zinc oxide nanoparticles provide an adjuvant effect to ovalbumin via a Th2 response in Balb/c mice. Int. Immunol. 26, 159–172. doi: 10.1093/intimm/dxt053
Saeed, A., Abd, H., and Sandstrom, G. (2015). Microbial aetiology of acute diarrhoea in children under five years of age in Khartoum, Sudan. J. Med. Microbiol. 62, 432–437. doi: 10.1099/jmm.0.000043
Shaikh, S., Fatima, J., Shakil, S., Rizvi, S. M. D., and Kamal, M. A. (2015). Antibiotic resistance and extended spectrum beta-lactamases: types, epidemiology and treatment. Saudi J. Biol. Sci. 22, 90–101. doi: 10.1016/j.sjbs.2014.08.002
Siddiqi, K. S., Rahman, A. U., and Tajuddin Husan, A. (2018). Properties of zinc oxide nanoparticles and their activity against microbes. Nanoscale Res. Lett. 13:141. doi: 10.1186/s11671-018-2532-3
Silver, L. L. (2016). Appropriate targets for antibacterial drugs. Cold Spring Harb. Perspect. Med. 6:a030239. doi: 10.1101/cshperspect.a030239
Singh, R., Smitha, M. S., and Singh, S. P. (2014). The role of nanotechnology in combating multi-drug resistant bacteria. J. Nanosci. Nanotechnol. 14, 4745–4756. doi: 10.1166/jnn.2014.9527
Sirelkhatim, A., Mahmud, S., Seeni, A., Kaus, N. H. M., Ann, L. C., Bakhori, S. K. M., et al. (2015). Review on zinc oxide nanoparticles: antibacterial activity and toxicity mechanism. Nano Micro Lett. 7, 219–242. doi: 10.1007/s40820-015-0040-x
Spanhel, L., and Anderson, M. A. (1991). Semiconductor clusters in the sol-gel process: quantized aggregation, gelation, and crystal growth in concentrated ZnO colloids. J. Am. Chem. Soc. 113, 2826–2833. doi: 10.1021/ja00008a004
Thomas, P., Sekhar, A. C., Upreti, R., Mujawar, M. M., and Pasha, S. S. (2015). Optimization of single plate-serial dilution spotting (SP-SDS) with sample anchoring as an assured method for bacterial and yeast cfu enumeration and single colony isolation from diverse samples. Biotechnol. Rep. 8, 45–55. doi: 10.1016/j.btre.2015.08.003
Tiwari, V., Mishra, N., Gadani, K., Solanki, P. S., Shah, N. A., and Tiwari, M. (2018). Mechanism of anti-bacterial activity of zinc oxide nanoparticle against carbapenem-resistant Acinetobacter baumannii. Front. Microbiol. 9:1218. doi: 10.3389/fmicb.2018.01218
Vazquez, J. A. (2010). Optimal management of oropharyngeal and esophageal candidiasis in patients living with HIV infection. HIV/AIDS 2, 89–101. doi: 10.2147/HIV.S6660
Wahab, R., Khan, F., Singh, R. B., and Khan, A. (2014). Enhance antimicrobial activity of ZnO nanomaterials (QDs and NPs) and their analytical applications. Physica E Low Dimens. Syst. Nanostruct. 62, 111–117. doi: 10.1016/j.physe.2014.04.017
Wang, J., Woo, M., and Yan, C. (2017). Spot plating assay for the determination of survival and plating efficiency of Escherichia coli in sub-MIC levels of antibiotics. JEMI Methods. 1, 26–29.
Xie, Y., He, Y., Irwin, P. L., Jin, T., and Shi, X. (2011). Antibacterial activity and mechanism of action of zinc oxide nanoparticles against Campylobacter jejuni. Appl. Environ. Microbiol. 77, 2325–2331. doi: 10.1128/AEM.02149-10
Zhou, Y., Zhu, X., Hou, H., Lu, Y., Yu, J., Mao, L., et al. (2018). Characteristics of diarrheagenic Escherichia coli among children under 5 years of age with acute diarrhoea: a hospital-based study. BMC Infect. Dis. 18:63. doi: 10.1186/s12879-017-2936-1
Keywords: ZnO QDs, multi drug resistance (MDR), antimicrobial therapy, broad spectrum, microbicidal
Citation: Preeti, Radhakrishnan VS, Mukherjee S, Mukherjee S, Singh SP and Prasad T (2020) ZnO Quantum Dots: Broad Spectrum Microbicidal Agent Against Multidrug Resistant Pathogens E. coli and C. albicans. Front. Nanotechnol. 2:576342. doi: 10.3389/fnano.2020.576342
Received: 25 June 2020; Accepted: 14 October 2020;
Published: 13 November 2020.
Edited by:
Yogendra Kumar Mishra, University of Southern Denmark, DenmarkReviewed by:
Won Min Park, Kansas State University, United StatesMubarak A. Mujawar, Florida International University, United States
Copyright © 2020 Preeti, Radhakrishnan, Mukherjee, Mukherjee, Singh and Prasad. This is an open-access article distributed under the terms of the Creative Commons Attribution License (CC BY). The use, distribution or reproduction in other forums is permitted, provided the original author(s) and the copyright owner(s) are credited and that the original publication in this journal is cited, in accordance with accepted academic practice. No use, distribution or reproduction is permitted which does not comply with these terms.
*Correspondence: Tulika Prasad, prasadtulika@hotmail.com; prasadtulika@mail.jnu.ac.in; Surinder P. Singh, singh.uprm@gmail.com