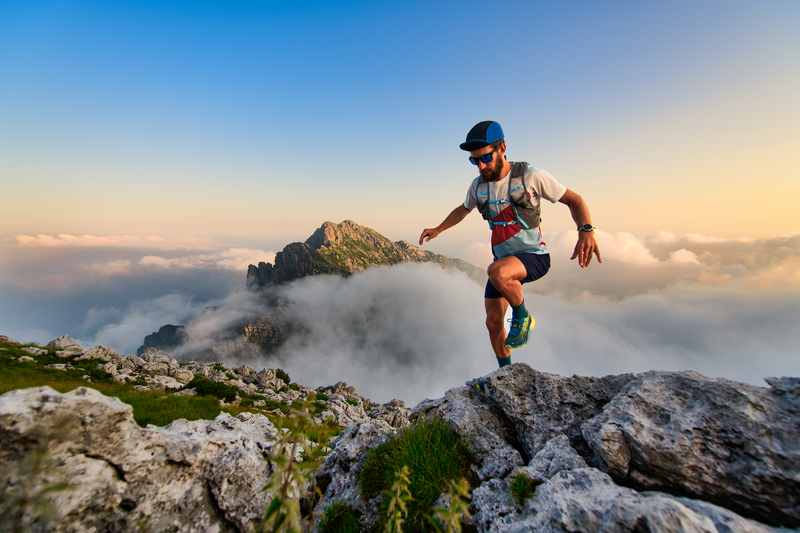
95% of researchers rate our articles as excellent or good
Learn more about the work of our research integrity team to safeguard the quality of each article we publish.
Find out more
MINI REVIEW article
Front. Mol. Neurosci. , 19 March 2025
Sec. Brain Disease Mechanisms
Volume 18 - 2025 | https://doi.org/10.3389/fnmol.2025.1499376
Propionate, a short-chain fatty acid (SCFA), has recently attracted attention for its various health benefits. However, elevated levels of propionate in certain pathological conditions can have adverse effects. Propionic acidemia (PA) is a rare metabolic disorder caused by mutations in the propionyl-CoA carboxylase (PCC) gene (PCCA or PCCB), leading to reduced PCC activity and impaired propionyl-CoA metabolism. This metabolic block at the PCC-mediated step results in the accumulation of propionyl-CoA and its metabolites, including propionate, contributing to various complications, such as neurological dysfunction, in patients with PA. This review examines propionate synthesis, its physiological role, its metabolism in healthy individuals and those with PA, and the pathological link between elevated propionate levels and neurological dysfunctions in PA patients. A deeper understanding of propionate metabolism under both normal and pathological conditions will help clarify the full spectrum of its metabolic effects.
Propionate, a short-chain fatty acid (SCFA) produced by the microbiome, is chemically similar to acetate but follows a distinct metabolic pathway. While acetate supports energy metabolism and fatty acid synthesis through the provision of acetyl-CoA, propionate acts as an anaplerotic substrate, replenishing tricarboxylic acid (TCA) cycle intermediates when cataplerosis depletes them (Wolever et al., 1991; Brunengraber and Roe, 2006). The metabolic flux of propionyl-CoA entering the TCA cycle is significantly slower than that of acetyl-CoA (Wang et al., 2024). However, anaplerosis is essential in organs with high cataplerotic activity, such as the liver, kidneys, intestines, pancreas, and brain (Weidemann et al., 1970; Mithieux and Gautier-Stein, 2014; Zhang et al., 2016; He et al., 2021; Marin-Valencia et al., 2024). Moreover, propionate supplementation has been shown to offer benefits beyond its role in anaplerosis, such as reducing lipogenesis, lowering serum cholesterol levels, mitigating depressive-like behavior, and reducing carcinogenesis risk (Hosseini et al., 2011). However, propionate’s effects on health can be double-edged, with its pathophysiological roles varying depending on the health context. For instance, in propionic acidemia (PA)—a recessive metabolic disorder caused by mutations in the PCCA or PCCB genes—impaired propionyl-CoA metabolism leads to a host of metabolic alterations. The accumulation of propionate and the blockade of its metabolism have synergistically detrimental effects on various organs, with neurological dysfunctions being one of the most common complications in PA patients (Marchuk et al., 2023).
In this minireview, we summarize the current understanding of propionate sources, metabolism, and its pathological roles in neurological dysfunctions when its metabolism is impaired in PA.
In addition to dietary sources, SCFAs are primarily produced through the fermentation of dietary carbohydrates and certain amino acids in the intestine (Louis and Flint, 2017). The intestinal origin of propionate has been confirmed by many studies, including our recent data showing that propionate levels in the portal vein are approximately 50 times higher than that in circulating plasma in a mouse study (Wang et al., 2024). Further evidence comes from germ-free mice, which display extremely low propionate levels (70 times lower) in the portal vein compared to control mice, strongly indicating that the majority of propionate is derived from the microbiome (Wang et al., 2024).
The production of acetic, propionic, and butyric acid by the gut microbiome occurs in a molar ratio of approximately 3:1:1 (Hosseini et al., 2011). For a 70 kg human, the gut microbiome produces roughly 2 g of propionate per day (Morrison and Preston, 2016; Killingsworth et al., 2020), with concentrations reaching up to 10–30 mM in the proximal colon (Cummings et al., 1987). Propionate is produced through several metabolic pathways, including the succinate, acrylate, propanediol, and 2-ketobutyrate pathways (Figure 1; Hosseini et al., 2011; Louis and Flint, 2017). Among these, the succinate pathway is the most prevalent in the human gut microbiota (Kundra et al., 2024). Thus, propionate production by the microbiome can be influenced by diet, probiotics, and antibiotics. Interestingly, our recent findings show that a 23 h fasting significantly reduces microbiome-derived propionate (He et al., 2024).
Figure 1. Propionate synthesis in microbiome. Four pathways (acrylate pathway in gray, succinate pathway in blue, 2-oxobutyrate pathway in orange, and 1,2-propanediol pathway in purple) lead to the synthesis of propionate in microbiome. PEP, phosphoenolpyruvate; Dihydroxyacetone-P, dihydroxyacetone phosphate.
Propionate can also be generated through the hydrolysis of propionyl-CoA. Acyl-CoA thioesterases are enzymes that catalyze the hydrolysis of CoA esters, converting them into free acids and CoA (Reaction formula 1) (Hunt and Alexson, 2002; Bai et al., 2024). These enzymes, also referred to as acyl-CoA hydrolases or palmitoyl-CoA hydrolases, help regulate intracellular levels of CoA esters together with carnitine acyl-CoA transferase, preventing the accumulation of acyl-CoAs, which cannot cross cellular membranes.
The hydrolysis of propionyl-CoA to propionate is not a reversible reaction of short-chain acyl-CoA synthetases (ACSSs). The activation of propionate to propionyl-CoA by a major isozyme (ACSS3) requires ATP (Yoshimura et al., 2017). These two opposing reactions complicate the assessment of propionate production from various metabolic sources. Consequently, using 13C-labeled propionate to measure the contributions of the microbiome, amino acids, and other sources to propionate production may be misleading due to the hydrolysis of propionyl-CoA to propionate (Thompson et al., 1990). Furthermore, overnight fasting may underestimate microbiome-derived propionate production, as indicated in our recent experiment with Pcca–/–(A138T) mice, an animal model for PA (He et al., 2024).
The intestinal microbiome produces significant amounts of SCFAs, including propionate (∼200 μM in the portal vein). However, circulating blood levels of propionate are much lower, approximately 0.4–5 μM, compared to acetate, which ranges from 50 to 200 μM (Bose et al., 2019; Kiasat et al., 2023). This difference in circulating levels of propionate and acetate is consistent with their corresponding acyl-CoA and acylcarnitine levels in tissues, where the C3/C2 (either acyl-CoA or acylcarnitine form) ratio is roughly 0.1 (Zhao et al., 2022).
The healthy liver efficiently metabolizes propionate, resulting in low systemic exposure in other organs (Wang et al., 2024). Given the liver’s high capacity to metabolize propionate, dietary propionate supplementation is not expected to substantially increase the circulating levels of propionate in healthy subjects. However, in metabolic conditions like PA, where liver metabolism of propionate is compromised, circulating propionate can rise markedly, reaching millimolar levels and leading to increased exposure in peripheral tissues (Wang et al., 2024).
Propionate is considered beneficial to health and plays multiple physiological roles in the human body. For example, it promotes enteric smooth muscle contractions and modulates colonic motility (Mitsui et al., 2005). Additionally, propionate stimulates the synthesis of host defense peptides, which are critical in the body’s first line of defense against bacteria, fungi, parasites, and enveloped viruses (Sunkara et al., 2012). The metabolism of propionate is associated with glucose production and energy metabolism (Jones et al., 1997). Through a series of reactions, propionate is first converted to propionyl-CoA before ultimately being converted to succinyl-CoA (Deng et al., 2009; Jin et al., 2015; Wilson et al., 2017; Wang et al., 2018). Succinyl-CoA is a substrate in the TCA cycle and is further metabolized to oxaloacetate, which is a substrate for glucose synthesis. Thus, dietary propionate could impact the TCA cycle and gluconeogenesis (Brunengraber and Roe, 2006; Killingsworth et al., 2020).
Interestingly, propionate produced by the microbiome has been shown to improve various aspects of energy metabolism, such as reducing adiposity and body weight. This is achieved through the following mechanisms: (1) Propionate stimulates intestinal gluconeogenesis via a gut-brain neural circuit involving the fatty acid receptor FFAR3 (De Vadder et al., 2014). (2) Increased intestinal propionate has also been linked to reduced stress behaviors in mice (Burokas et al., 2017) and attenuated reward-based eating behaviors via striatal pathways in humans (Byrne et al., 2016). (3) Propionate exhibits antilipogenic and cholesterol-lowering effects by competitively inhibiting acetate uptake by liver cells (Delzenne and Williams, 2002) and reducing cholesterol biosynthesis (Arora et al., 2011). (4) Propionate may also help reduce obesity by promoting the secretion of PYY and GLP-1 hormones through binding and activating G-protein coupled receptors (GPR41 and GPR43) from enteroendocrine cells, which induce satiety, reduce energy intake, and promote weight loss (Arora et al., 2011; Killingsworth et al., 2020; Zhang et al., 2022).
Propionate has also demonstrated immune-modulatory and anti-inflammatory effects (Langfeld et al., 2021). Its regulation of the immune system occurs primarily through two key mechanisms: (1) Activation of G-protein coupled receptors for the SCFA receptor family, and (2) Inhibition of histone deacetylases (Tobin et al., 2021). Deficiency of propionate has been linked to an increased risk of asthma and allergies, underscoring its protective role in immune function (Bottcher et al., 2000; Ivashkin et al., 2019; Roduit et al., 2019).
The first step of propionate metabolism is its activation into propionyl-CoA by ACSSs, specifically the ACSS3 isoform (Yoshimura et al., 2017). Propionyl-CoA, an anaplerotic substrate, enters the TCA cycle for complete metabolism. Propionate metabolism is highly efficient, particularly in the liver, maintaining circulating propionate at low levels (0.4–5 μM). However, this metabolism is disrupted when propionyl-CoA carboxylation is impaired, as seen in patients with PA. A reliable biomarker of PA is elevated propionate levels in both blood and urine, as observed in PA patients and mouse models, although propionate is often underreported due to the need for advanced analytical techniques (Wang et al., 2018). The precise mechanism by which PCC deficiency leads to elevated propionate remains unclear.
Our recent work demonstrated that ACSS3 activity in the liver is attenuated in Pcca–/– (A138T) mice (Wang et al., 2018). As the liver is the primary organ in metabolizing propionate derived from the microbiome, the reduction in ACSS3 could contribute to the impaired metabolic disposal of propionate and its subsequent elevation. Another potential source of propionate is the hydrolysis of propionyl-CoA. Under normal conditions, intracellular propionyl-CoA levels are much lower than acetyl-CoA. Thus, the contribution of propionyl-CoA hydrolysis to propionate levels is likely minimal. However, in PA, when propionyl-CoA accumulates to levels comparable to acetyl-CoA, the contribution of propionyl-CoA hydrolysis to propionate production may increase. Despite this, it remains unclear how much propionate is produced from propionyl-CoA hydrolysis in PA. Addressing this question is critical to better understanding the pathophysiology of PA.
If propionyl-CoA hydrolysis were highly efficient, the accumulation of propionyl-CoA might not take place the primary issue, and the elevated propionate or other metabolites could instead be the main disease-causing factor. However, recent studies have shown that increasing coenzyme A synthesis via drug activators alleviates metabolic stress caused by propionyl-CoA accumulation (Subramanian et al., 2021; Subramanian et al., 2023). This suggests that even propionyl-CoA hydrolysis to propionate may occur, it is likely not a major contributor to overall propionate production or the regulation of propionyl-CoA levels.
While propionate has been shown to offer health benefits, its excess levels can be pathological. For instance, individuals with periodontal disease exhibit elevated levels of propionate in their saliva and may be at increased risk for developing Alzheimer’s disease (AD) (Killingsworth et al., 2020). Emerging evidence suggests that excess propionate, along with an increase in propionate-producing bacteria, may play a role in the development of dementia, particularly in AD (Arora et al., 2011).
In PA, where the PCC enzyme is impaired, propionate levels can rise significantly, reaching millimolar concentrations. The metabolic toxicity associated with these elevated propionate levels in PA has been well-documented (Shchelochkov et al., 1993; Marchuk et al., 2023), though the specific mechanisms behind the damage remain largely unclear. PA patients are subject to various complications (Marchuk et al., 2023). In addition to low muscle tone, cardiomyopathy, and pancreatitis, PA patients often present with a variety of neurological symptoms. These include psychomotor retardation, dystonia, developmental and speech delays, dementia, visual hallucinations, psychosis, seizures, stroke, coma, hypotonia, athetosis, optic neuropathy, acute hemiparesis, and other neurological impairments (Scholl-Burgi et al., 2009; Schreiber et al., 2012; Shuaib et al., 2012; Dejean de la Batie et al., 2014; Witters et al., 2016; AlGhamdi et al., 2018; Pfeifer et al., 2018; Almuqbil et al., 2019). Patients may also experience chronic psychological and cognitive sequelae, frequently leading to intellectual disability. Autism spectrum disorder has been reported in approximately 21% of PA patients (Witters et al., 2016; Cotrina et al., 2020).
Neurological impairments are observed more frequently in late-onset PA compared to early-onset cases (Jiang et al., 2022). Diagnosis Diagnostic imaging via magnetic resonance imaging typically reveals bilateral basal ganglia abnormalities, varying degrees of cisternal and sulcal widening, diffusion restriction, asymmetric atrophy, delayed myelination, corpus callosum dysplasia, volume loss in the vermis, gray matter vacuolization, and supratentorial white matter edema (Shuaib et al., 2012; AlGhamdi et al., 2018; Pfeifer et al., 2018; Jiang et al., 2022).
Figure 2 illustrates the current understanding of how propionate and its metabolites contribute to neurological dysfunctions observed in PA patients. The neurological manifestations in PA most likely stem from the metabolic consequences of elevated propionate and its metabolites (Scholl-Burgi et al., 2009; Schreiber et al., 2012). PA-related metabolic stroke, often caused by metabolic acidosis or hyperammonemia, contributes to acute neurological events (Almuqbil et al., 2019). Methylcitrate, a metabolite elevated in PA, has been shown to decrease glutamate oxidation by inhibiting glutamate dehydrogenase, as well as induce mitochondrial permeability transition. The reduction in glutamate oxidation and mitochondrial ATP generation are key factors in the neurological dysfunctions seen in PA (Amaral et al., 2016). In addition to inhibiting glutamate dehydrogenase, propionate and its metabolites also inhibit other TCA cycle enzymes such as pyruvate dehydrogenase, oxoglutarate dehydrogenase, and succinyl-CoA ligase, further compromising energy metabolism (Schreiber et al., 2012). These disruptions in energy metabolism particularly affect areas like the basal ganglia, which are highly energy-dependent and thus more vulnerable to damage from elevated propionate levels (Ji et al., 2022).
Figure 2. Detrimental effects of accumulated propionate and its metabolites in brain in propionic acidemia (PA). SUC-CoA, succinyl-CoA; MM-CoA, methylmalonyl-CoA; GABA, gamma-aminobutyric acid. Accumulated methylcitrate inhibits energy metabolism within the tricarboxylic acid (TCA) cycle, while elevated levels of propionylglutamate impair ammonium disposal via the urea cycle. The various toxic effects of accumulated propionate are highlighted in orange.
Despite lifelong dietary management, some PA patients develop late-onset bilateral optic neuropathy, suggesting a persistent pathological role of propionate in neurological dysfunctions (Williams et al., 2009). The neurotoxic effects of propionate have been observed in both animal models and human patients (Wyse et al., 1998; Colin-Gonzalez et al., 2015). For example, propionate has been shown to inhibit Na+, K+-ATPase activity in rat brain cortex, which may contribute to neurological dysfunctions in PA (Wyse et al., 1998). At concentrations equal to or lower than those found in the blood and brain of PA patients, propionate markedly affects the phosphorylation of cytoskeletal proteins in the cerebral cortex. Alterations in these proteins may disrupt cellular structure, leading to neurodegeneration (de Mattos-Dutra et al., 2000; de Almeida et al., 2006). Elevated propionate levels inhibit GABA transaminase, leading to the accumulation of GABA in the brain. This results in reduced neuronal activity, manifesting as lethargy (Morland et al., 2018).
Propionate also reduces ganglioside content in the cerebral cortex, which could contribute to brain damage in PA. This is similar to the effects seen with methylmalonic acid treatment, reinforcing the notion that brain damage is likely due to propionate rather than propionyl-CoA, which accumulates at much lower levels in methylmalonic acidemia (Trindade et al., 2002). High concentrations of propionate in the brain, as seen in animal models, lead to abnormal behaviors such as delayed habituation and repetitive motor activity (Brusque et al., 1999; Cotrina et al., 2020). These findings suggest that early postnatal propionate exposure results in long-term behavioral deficits.
Oxidative stress is another driver in PA-related neurological damage. Propionate has been shown to stimulate lipid peroxidation in brain tissue, leading to free radical generation that contributes to neurological dysfunctions (Fontella et al., 2000; Rigo et al., 2006; Ribas et al., 2010). In the hippocampus, chronic propionate exposure leads to spatial performance impairments, which are mitigated by ascorbic acid, an antioxidant (Pettenuzzo et al., 2002). Additionally, intrastriatal injection of propionate induces seizures and increases protein carbonyl content in the striatum, effects that are prevented by MK-801, an NMDA receptor antagonist, suggesting a role of NMDA receptors in PA associated oxidative damage and convulsions (Rigo et al., 2006).
Furthermore, propionate may alter neuronal gene expression by increasing histone acetylation (Nguyen et al., 2007). In vitro studies show that while propionate can be metabolized by glial cells, neurons lack this ability, potentially due to a lack of propionyl-CoA synthetase or mitochondrial transporters for propionate. However, exposure to propionate increases histone acetylation in both neurons and astrocytes, suggesting that neurons may be particularly vulnerable to chronically elevated propionate levels in PA (Nguyen et al., 2007). The epigenetic effects of elevated propionate levels remain largely unexplored, presenting a promising area for future research, as both propionyl-CoA and propionate could influence the propionylation, acetylation of proteins and histones and gene transcription (Chapman et al., 2015; Lagerwaard et al., 2021; Park et al., 2023; Yang et al., 2023).
In summary, the metabolic toxicity of elevated propionate in PA involves multiple pathological mechanisms, including mitochondrial dysfunction, oxidative stress, and disruptions in gene expression.
Since chronically elevated propionate is a key factor in the neurological dysfunction associated with PA, treatment strategies should focus on reducing propionate synthesis and enhancing its metabolic disposal.
To reduce propionate synthesis, two main approaches have been reported: (1) Antibiotics: These are used to inhibit gut microbiota, thereby reducing microbial production of propionate, and (2) Carnitine supplementation: Propionyl-CoA can be converted to propionylcarnitine by carnitine acetyltransferase or to propionate by propionyl-CoA hydrolase, both of which help regulate cellular propionyl-CoA levels. Supplementing with L-carnitine supports the conversion of propionyl-CoA to propionylcarnitine, reducing propionate production.
To increase propionate disposal, several strategies have emerged. Firstly, pharmacological activation of CoA synthesis: This strategy has been shown to increase propionyl-CoA flux into the TCA cycle, reducing metabolic stress in PA mice (Armstrong et al., 2021; Subramanian et al., 2021; Subramanian et al., 2023). Secondly, liver transplantation: As the primary site of propionate metabolism, a healthy liver can metabolize 99% of propionate from the portal vein. Liver transplantation has been shown to improve neurological functions in PA patients by preventing metabolic decompensation and reducing propionate-related metabolites, such as methylcitrate and 3-hydroxypropionate (Nagao et al., 2013). Although no direct propionate data was reported, hepatic disposal of propionate is expected to improve. However, propionyl-CoA may still accumulate in other organs deficient in PCC, potentially leading to localized increases in propionate levels. Thirdly, mRNA enzyme replacement therapy: Recent advancements offer the potential to restore PCC activity and improve propionate metabolism. While this strategy shows promise as a future treatment option, current methods still face challenges with side effects (Jiang et al., 2020; Koeberl et al., 2024).
Propionate, a SCFA, is beneficial to health when present at moderate levels and metabolized normally. However, elevated propionate levels or impaired propionate metabolism in PA can lead to harmful effects and various complications, including neurological dysfunctions. Significant progress has been made in understanding the pathological mechanisms linking elevated propionate to neurological dysfunctions in patients with PA. However, several areas require further investigation, including propionate production from propionyl-CoA hydrolysis in the brain, cellular levels of propionate in the brain, and the impact of propionate/propionyl-CoA on protein and histone acylation.
XC: Writing – original draft, Writing – review and editing. QC: Writing – original draft, Writing – review and editing. G-FZ: Writing – original draft, Writing – review and editing.
The author(s) declare that financial support was received for the research and/or publication of this article. This work was supported by the Propionic Acidemia Foundation award to G-FZ; NIH R01 AA030026 and NIIH R21TR005163 to XC and G-FZ; and QC was supported by the RCMI NIMHD U54MD012392, NIA R25AG086122, and Duke/UNC ADRC P30AG072958 grants.
The authors declare that the research was conducted in the absence of any commercial or financial relationships that could be construed as a potential conflict of interest.
All claims expressed in this article are solely those of the authors and do not necessarily represent those of their affiliated organizations, or those of the publisher, the editors and the reviewers. Any product that may be evaluated in this article, or claim that may be made by its manufacturer, is not guaranteed or endorsed by the publisher.
AlGhamdi, A., Alrifai, M. T., Al Hammad, A. I., Al Mutairi, F., Alswaid, A., Eyaid, W., et al. (2018). Epilepsy in propionic acidemia: Case series of 14 Saudi patients. J. Child Neurol. 33, 713–717. doi: 10.1177/0883073818786157
Almuqbil, M., Chinsky, J. M., and Srivastava, S. (2019). Metabolic strokes in propionic acidemia: Transient hemiplegic events without encephalopathy. Child Neurol. Open 6, 1–9. doi: 10.1177/2329048X19873242
Amaral, A. U., Cecatto, C., Castilho, R. F., and Wajner, M. (2016). 2-Methylcitric acid impairs glutamate metabolism and induces permeability transition in brain mitochondria. J. Neurochem. 137, 62–75. doi: 10.1111/jnc.13544
Armstrong, A. J., Collado, M. S., Henke, B. R., Olson, M. W., Hoang, S. A., Hamilton, C. A., et al. (2021). A novel small molecule approach for the treatment of propionic and methylmalonic acidemias. Mol. Genet. Metab. 133, 71–82. doi: 10.1016/j.ymgme.2021.03.001
Arora, T., Sharma, R., and Frost, G. (2011). Propionate. Anti-obesity and satiety enhancing factor? Appetite 56, 511–515. doi: 10.1016/j.appet.2011.01.016
Bai, L., Yang, P., Han, B., and Kong, L. (2024). Progress of the acyl-Coenzyme A thioester hydrolase family in cancer. Front. Oncol. 14:1374094. doi: 10.3389/fonc.2024.1374094
Bose, S., Ramesh, V., and Locasale, J. W. (2019). Acetate metabolism in physiology, cancer, and beyond. Trends Cell. Biol. 29, 695–703. doi: 10.1016/j.tcb.2019.05.005
Bottcher, M. F., Nordin, E. K., Sandin, A., Midtvedt, T., and Bjorksten, B. (2000). Microflora-associated characteristics in faeces from allergic and nonallergic infants. Clin. Exp. Allergy 30, 1590–1596. doi: 10.1046/j.1365-2222.2000.00982.x
Brunengraber, H., and Roe, C. R. (2006). Anaplerotic molecules: Current and future. J. Inherit. Metab. Dis. 29, 327–331. doi: 10.1007/s10545-006-0320-1
Brusque, A. M., Mello, C. F., Buchanan, D. N., Terracciano, S. T., Rocha, M. P., Vargas, C. R., et al. (1999). Effect of chemically induced propionic acidemia on neurobehavioral development of rats. Pharmacol. Biochem. Behav. 64, 529–534. doi: 10.1016/s0091-3057(99)00127-6
Burokas, A., Arboleya, S., Moloney, R. D., Peterson, V. L., Murphy, K., Clarke, G., et al. (2017). Targeting the microbiota-gut-brain axis: Prebiotics have anxiolytic and antidepressant-like effects and reverse the impact of chronic stress in mice. Biol. Psychiatry 82, 472–487. doi: 10.1016/j.biopsych.2016.12.031
Byrne, C. S., Chambers, E. S., Alhabeeb, H., Chhina, N., Morrison, D. J., Preston, T., et al. (2016). Increased colonic propionate reduces anticipatory reward responses in the human striatum to high-energy foods. Am. J. Clin. Nutr. 104, 5–14. doi: 10.3945/ajcn.115.126706
Chapman, K. A., Bush, W. S., and Zhang, Z. (2015). Gene expression in cell lines from propionic acidemia patients, carrier parents, and controls. Mol. Genet. Metab. 115, 174–179. doi: 10.1016/j.ymgme.2015.05.004
Colin-Gonzalez, A. L., Paz-Loyola, A. L., Serratos, I., Seminotti, B., Ribeiro, C. A., Leipnitz, G., et al. (2015). Toxic synergism between quinolinic acid and organic acids accumulating in glutaric acidemia type I and in disorders of propionate metabolism in rat brain synaptosomes: Relevance for metabolic acidemias. Neuroscience 308, 64–74. doi: 10.1016/j.neuroscience.2015.09.002
Cotrina, M. L., Ferreiras, S., and Schneider, P. (2020). High prevalence of self-reported autism spectrum disorder in the Propionic acidemia registry. JIMD Rep. 51, 70–75. doi: 10.1002/jmd2.12083
Cummings, J. H., Pomare, E. W., Branch, W. J., Naylor, C. P., and Macfarlane, G. T. (1987). Short chain fatty acids in human large intestine, portal, hepatic and venous blood. Gut 28, 1221–1227. doi: 10.1136/gut.28.10.1221
de Almeida, L. M., Funchal, C., Gottfried, C., Wajner, M., and Pessoa-Pureur, R. (2006). Propionic acid induces cytoskeletal alterations in cultured astrocytes from rat cerebral cortex. Metab. Brain Dis. 21, 51–62. doi: 10.1007/s11011-006-9002-9
de Mattos-Dutra, A., Meirelles, R., Bevilaqua da Rocha, B., Kommers, T., and Wofchuk, S. T. (2000). Methylmalonic and propionic acids increase the in vitro incorporation of 32P into cytoskeletal proteins from cerebral cortex of young rats through NMDA glutamate receptors. Brain Res. 856, 111–118. doi: 10.1016/s0006-8993(99)02380-x
De Vadder, F., Kovatcheva-Datchary, P., Goncalves, D., Vinera, J., Zitoun, C., Duchampt, A., et al. (2014). Microbiota-generated metabolites promote metabolic benefits via gut-brain neural circuits. Cell 156, 84–96. doi: 10.1016/j.cell.2013.12.016
Dejean de la Batie, C., Barbier, V., Valayannopoulos, V., Touati, G., Maltret, A., Brassier, A., et al. (2014). Acute psychosis in propionic acidemia: 2 case reports. J. Child Neurol. 29, 274–279. doi: 10.1177/0883073813508812
Delzenne, N. M., and Williams, C. M. (2002). Prebiotics and lipid metabolism. Curr. Opin. Lipidol. 13, 61–67. doi: 10.1097/00041433-200202000-00009
Deng, S., Zhang, G. F., Kasumov, T., Roe, C. R., and Brunengraber, H. (2009). Interrelations between C4 ketogenesis, C5 ketogenesis, and anaplerosis in the perfused rat liver. J. Biol. Chem. 284, 27799–27807. doi: 10.1074/jbc.M109.048744
Fontella, F. U., Pulrolnik, V., Gassen, E., Wannmacher, C. M., Klein, A. B., Wajner, M., et al. (2000). Propionic and L-methylmalonic acids induce oxidative stress in brain of young rats. Neuroreport 11, 541–544. doi: 10.1097/00001756-200002280-00023
He, W., Marchuk, H., Koeberl, D., Kasumov, T., Chen, X., and Zhang, G. F. (2024). Fasting alleviates metabolic alterations in mice with propionyl-CoA carboxylase deficiency due to Pcca mutation. Commun. Biol. 7:659. doi: 10.1038/s42003-024-06362-8
He, W., Wang, Y., Xie, E. J., Barry, M. A., and Zhang, G. F. (2021). Metabolic perturbations mediated by propionyl-CoA accumulation in organs of mouse model of propionic acidemia. Mol. Genet. Metab. 134, 257–266. doi: 10.1016/j.ymgme.2021.09.009
Hosseini, E., Grootaert, C., Verstraete, W., and Van de Wiele, T. (2011). Propionate as a health-promoting microbial metabolite in the human gut. Nutr. Rev. 69, 245–258. doi: 10.1111/j.1753-4887.2011.00388.x
Hunt, M. C., and Alexson, S. E. (2002). The role Acyl-CoA thioesterases play in mediating intracellular lipid metabolism. Prog. Lipid Res. 41, 99–130. doi: 10.1016/s0163-7827(01)00017-0
Ivashkin, V., Zolnikova, O., Potskherashvili, N., Trukhmanov, A., Kokina, N., Dzhakhaya, N., et al. (2019). Metabolic activity of intestinal microflora in patients with bronchial asthma. Clin. Pract. 9:1126. doi: 10.4081/cp.2019.1126
Ji, G., Liu, Y., Song, X., and Li, Z. (2022). Case report: Novel mutations in the PCCB gene causing late-onset Propionic acidemia. Front. Genet. 13:807822. doi: 10.3389/fgene.2022.807822
Jiang, L., Park, J. S., Yin, L., Laureano, R., Jacquinet, E., Yang, J. S., et al. (2020). Dual mRNA therapy restores metabolic function in long-term studies in mice with propionic acidemia. Nat. Commun. 11:5339. doi: 10.1038/s41467-020-19156-3
Jiang, Z., Fu, Y., Wei, X., Wang, Z., and Yu, X. (2022). Case report: A unusual case of delayed propionic acidemia complicated with subdural hematoma. Front. Neurol. 13:1010636. doi: 10.3389/fneur.2022.1010636
Jin, Z., Bian, F., Tomcik, K., Kelleher, J. K., Zhang, G. F., and Brunengraber, H. (2015). Compartmentation of Metabolism of the C12-, C9-, and C5-n-dicarboxylates in Rat liver, investigated by Mass Isotopomer analysis: Anaplerosis from dodecanedioate. J. Biol. Chem. 290, 18671–18677. doi: 10.1074/jbc.M115.651737
Jones, J. G., Naidoo, R., Sherry, A. D., Jeffrey, F. M., Cottam, G. L., and Malloy, C. R. (1997). Measurement of gluconeogenesis and pyruvate recycling in the rat liver: A simple analysis of glucose and glutamate isotopomers during metabolism of [1,2,3-(13)C3]propionate. FEBS Lett. 412, 131–137. doi: 10.1016/s0014-5793(97)00764-3
Kiasat, A., Rautiainen, S., Prast-Nielsen, S., Engstrand, L., Schuppe-Koistinen, I., Gustafsson, U. O., et al. (2023). Evaluation of plasma Short chain fatty acid levels as markers for Inflammatory bowel disease. Scand. J. Gastroenterol. 58, 1246–1252. doi: 10.1080/00365521.2023.2219357
Killingsworth, J., Sawmiller, D., and Shytle, R. D. (2020). Propionate and Alzheimer’s disease. Front. Aging Neurosci. 12:580001. doi: 10.3389/fnagi.2020.580001
Koeberl, D., Schulze, A., Sondheimer, N., Lipshutz, G. S., Geberhiwot, T., Li, L., et al. (2024). Interim analyses of a first-in-human phase 1/2 mRNA trial for propionic acidaemia. Nature 628, 872–877. doi: 10.1038/s41586-024-07266-7
Kundra, P., Greppi, A., Duppenthaler, M., Pluss, S., Pugin, B., Lacroix, C., et al. (2024). Vitamin B12 analogues from gut microbes and diet differentially impact commensal propionate producers of the human gut. Front. Nutr. 11:1360199. doi: 10.3389/fnut.2024.1360199
Lagerwaard, B., Pougovkina, O., Bekebrede, A. F., Te Brinke, H., Wanders, R. J. A., Nieuwenhuizen, A. G., et al. (2021). Increased protein propionylation contributes to mitochondrial dysfunction in liver cells and fibroblasts, but not in myotubes. J. Inherit. Metab. Dis. 44, 438–449. doi: 10.1002/jimd.12296
Langfeld, L. Q., Du, K., Bereswill, S., and Heimesaat, M. M. (2021). A review of the antimicrobial and immune-modulatory properties of the gut microbiota-derived short chain fatty acid propionate - What is new? Eur. J. Microbiol. Immunol. 11, 50–56. doi: 10.1556/1886.2021.00005
Louis, P., and Flint, H. J. (2017). Formation of propionate and butyrate by the human colonic microbiota. Environ. Microbiol. 19, 29–41. doi: 10.1111/1462-2920.13589
Marchuk, H., Wang, Y., Ladd, Z. A., Chen, X., and Zhang, G. F. (2023). Pathophysiological mechanisms of complications associated with propionic acidemia. Pharmacol. Ther. 249:108501. doi: 10.1016/j.pharmthera.2023.108501
Marin-Valencia, I., Kocabas, A., Rodriguez-Navas, C., Miloushev, V. Z., Gonzalez-Rodriguez, M., Lees, H., et al. (2024). Imaging brain glucose metabolism in vivo reveals propionate as a major anaplerotic substrate in pyruvate dehydrogenase deficiency. Cell Metab. 36, 1394–1410.e1312. doi: 10.1016/j.cmet.2024.05.002
Mithieux, G., and Gautier-Stein, A. (2014). Intestinal glucose metabolism revisited. Diabetes Res. Clin. Pract. 105, 295–301. doi: 10.1016/j.diabres.2014.04.008
Mitsui, R., Ono, S., Karaki, S., and Kuwahara, A. (2005). Propionate modulates spontaneous contractions via enteric nerves and prostaglandin release in the rat distal colon. Jpn. J. Physiol. 55, 331–338. doi: 10.2170/jjphysiol.RP000205
Morland, C., Froland, A. S., Pettersen, M. N., Storm-Mathisen, J., Gundersen, V., Rise, F., et al. (2018). Propionate enters GABAergic neurons, inhibits GABA transaminase, causes GABA accumulation and lethargy in a model of propionic acidemia. Biochem. J. 475, 749–758. doi: 10.1042/BCJ20170814
Morrison, D. J., and Preston, T. (2016). Formation of short chain fatty acids by the gut microbiota and their impact on human metabolism. Gut Microbes 7, 189–200. doi: 10.1080/19490976.2015.1134082
Nagao, M., Tanaka, T., Morii, M., Wakai, S., Horikawa, R., and Kasahara, M. (2013). Improved neurologic prognosis for a patient with propionic acidemia who received early living donor liver transplantation. Mol. Genet. Metab. 108, 25–29. doi: 10.1016/j.ymgme.2012.10.022
Nguyen, N. H., Morland, C., Gonzalez, S. V., Rise, F., Storm-Mathisen, J., Gundersen, V., et al. (2007). Propionate increases neuronal histone acetylation, but is metabolized oxidatively by glia. Relevance for propionic acidemia. J. Neurochem. 101, 806–814. doi: 10.1111/j.1471-4159.2006.04397.x
Park, K. C., Crump, N. T., Louwman, N., Krywawych, S., Cheong, Y. J., Vendrell, I., et al. (2023). Disrupted propionate metabolism evokes transcriptional changes in the heart by increasing histone acetylation and propionylation. Nat. Cardiovasc. Res. 2, 1221–1245. doi: 10.1038/s44161-023-00365-0
Pettenuzzo, L. F., Schuck, P. F., Fontella, F., Wannmacher, C. M., Wyse, A. T., Dutra-Filho, C. S., et al. (2002). Ascorbic acid prevents cognitive deficits caused by chronic administration of propionic acid to rats in the water maze. Pharmacol. Biochem. Behav. 73, 623–629. doi: 10.1016/s0091-3057(02)00856-0
Pfeifer, C. M., Van Tassel, D. C., and Miller, J. H. (2018). Unique neuroradiological findings in propionic acidemia. Radiol. Case Rep. 13, 1207–1211. doi: 10.1016/j.radcr.2018.08.011
Ribas, G. S., Manfredini, V., de Mari, J. F., Wayhs, C. Y., Vanzin, C. S., Biancini, G. B., et al. (2010). Reduction of lipid and protein damage in patients with disorders of propionate metabolism under treatment: A possible protective role of L-carnitine supplementation. Int. J. Dev. Neurosci. 28, 127–132. doi: 10.1016/j.ijdevneu.2010.01.002
Rigo, F. K., Pasquetti, L., Malfatti, C. R., Fighera, M. R., Coelho, R. C., Petri, C. Z., et al. (2006). Propionic acid induces convulsions and protein carbonylation in rats. Neurosci. Lett. 408, 151–154. doi: 10.1016/j.neulet.2006.08.075
Roduit, C., Frei, R., Ferstl, R., Loeliger, S., Westermann, P., Rhyner, C., et al. (2019). High levels of butyrate and propionate in early life are associated with protection against atopy. Allergy 74, 799–809. doi: 10.1111/all.13660
Scholl-Burgi, S., Haberlandt, E., Gotwald, T., Albrecht, U., Baumgartner Sigl, S., Rauchenzauner, M., et al. (2009). Stroke-like episodes in propionic acidemia caused by central focal metabolic decompensation. Neuropediatrics 40, 76–81. doi: 10.1055/s-0029-1231065
Schreiber, J., Chapman, K. A., Summar, M. L., Ah Mew, N., Sutton, V. R., MacLeod, E., et al. (2012). Neurologic considerations in propionic acidemia. Mol. Genet. Metab. 105, 10–15. doi: 10.1016/j.ymgme.2011.10.003
Shchelochkov, O. A., Carrillo, N., and Venditti, C. (1993). “Propionic acidemia,” in GeneReviews(R), eds M. P. Adam, J. Feldman, and G. M. Mirzaa (Seattle, WA: University of Washington).
Shuaib, T., Al-Hashmi, N., Ghaziuddin, M., Megdad, E., Abebe, D., Al-Saif, A., et al. (2012). Propionic acidemia associated with visual hallucinations. J. Child Neurol. 27, 799–803. doi: 10.1177/0883073811426929
Subramanian, C., Frank, M. W., Tangallapally, R., Yun, M. K., Edwards, A., White, S. W., et al. (2021). Pantothenate kinase activation relieves coenzyme A sequestration and improves mitochondrial function in mice with propionic acidemia. Sci. Transl. Med. 13:eabf5965. doi: 10.1126/scitranslmed.abf5965
Subramanian, C., Frank, M. W., Tangallapally, R., Yun, M. K., White, S. W., Lee, R. E., et al. (2023). Relief of CoA sequestration and restoration of mitochondrial function in a mouse model of propionic acidemia. J. Inherit. Metab. Dis. 46, 28–42. doi: 10.1002/jimd.12570
Sunkara, L. T., Jiang, W., and Zhang, G. (2012). Modulation of antimicrobial host defense peptide gene expression by free fatty acids. PLoS One 7:e49558. doi: 10.1371/journal.pone.0049558
Thompson, G. N., Walter, J. H., Bresson, J. L., Ford, G. C., Lyonnet, S. L., Chalmers, R. A., et al. (1990). Sources of propionate in inborn errors of propionate metabolism. Metabolism 39, 1133–1137. doi: 10.1016/0026-0495(90)90084-p
Tobin, D., Vige, R., and Calder, P. C. (2021). Review: The nutritional management of multiple sclerosis with propionate. Front. Immunol. 12:676016. doi: 10.3389/fimmu.2021.676016
Trindade, V. M., Brusque, A. M., Raasch, J. R., Pettenuzzo, L. E., Rocha, H. P., Wannmacher, C. M., et al. (2002). Ganglioside alterations in the central nervous system of rats chronically injected with methylmalonic and propionic acids. Metab. Brain Dis. 17, 93–102. doi: 10.1023/a:1015464028616
Wang, Y., Christopher, B. A., Wilson, K. A., Muoio, D., McGarrah, R. W., Brunengraber, H., et al. (2018). Propionate-induced changes in cardiac metabolism, notably CoA trapping, are not altered by l-carnitine. Am. J. Physiol. Endocrinol. Metab. 315, E622–E633. doi: 10.1152/ajpendo.00081.2018
Wang, Y., Zhu, S., He, W., Marchuk, H., Richard, E., Desviat, L. R., et al. (2024). The attenuated hepatic clearance of propionate increases cardiac oxidative stress in propionic acidemia. Basic Res. Cardiol. 119, 1045–1062. doi: 10.1007/s00395-024-01066-w
Weidemann, M. J., Hems, R., Williams, D. L., Spray, G. H., and Krebs, H. A. (1970). Gluconeogenesis from propionate in kidney and liver of the vitamin B12-deficient rat. Biochem. J. 117, 177–181. doi: 10.1042/bj1170177
Williams, Z. R., Hurley, P. E., Altiparmak, U. E., Feldon, S. E., Arnold, G. L., Eggenberger, E., et al. (2009). Late onset optic neuropathy in methylmalonic and propionic acidemia. Am. J. Ophthalmol. 147, 929–933. doi: 10.1016/j.ajo.2008.12.024
Wilson, K. A., Han, Y., Zhang, M., Hess, J. P., Chapman, K. A., Cline, G. W., et al. (2017). Inter-relations between 3-hydroxypropionate and propionate metabolism in rat liver: Relevance to disorders of propionyl-CoA metabolism. Am. J. Physiol. Endocrinol. Metab. 313, E413–E428. doi: 10.1152/ajpendo.00105.2017
Witters, P., Debbold, E., Crivelly, K., Vande Kerckhove, K., Corthouts, K., Debbold, B., et al. (2016). Autism in patients with propionic acidemia. Mol. Genet. Metab. 119, 317–321. doi: 10.1016/j.ymgme.2016.10.009
Wolever, T. M., Spadafora, P., and Eshuis, H. (1991). Interaction between colonic acetate and propionate in humans. Am. J. Clin. Nutr. 53, 681–687. doi: 10.1093/ajcn/53.3.681
Wyse, A. T., Brusque, A. M., Silva, C. G., Streck, E. L., Wajner, M., and Wannmacher, C. M. (1998). Inhibition of Na+,K+-ATPase from rat brain cortex by propionic acid. Neuroreport 9, 1719–1721. doi: 10.1097/00001756-199806010-00009
Yang, Z., He, M., Austin, J., Sayed, D., and Abdellatif, M. (2023). Reducing branched-chain amino acids improves cardiac stress response in mice by decreasing histone H3K23 propionylation. J. Clin. Invest. 133:e169399. doi: 10.1172/JCI169399
Yoshimura, Y., Araki, A., Maruta, H., Takahashi, Y., and Yamashita, H. (2017). Molecular cloning of rat acss3 and characterization of mammalian propionyl-CoA synthetase in the liver mitochondrial matrix. J. Biochem. 161, 279–289. doi: 10.1093/jb/mvw067
Zhang, Q., Koser, S. L., and Donkin, S. S. (2016). Propionate induces mRNA expression of gluconeogenic genes in bovine calf hepatocytes. J. Dairy Sci. 99, 3908–3915. doi: 10.3168/jds.2015-10312
Zhang, Y., Li, X., Huang, G., Wang, H., Chen, H., Su, Y., et al. (2022). Propionate stimulates the secretion of satiety hormones and reduces acute appetite in a cecal fistula pig model. Anim. Nutr. 10, 390–398. doi: 10.1016/j.aninu.2022.06.003
Keywords: propionic acidemia, propionate, neurological dysfunction, microbiome, short-chain fatty acids, rare metabolic diseases, metabolism
Citation: Chen X, Cheng Q and Zhang G-F (2025) Elevated propionate and its association with neurological dysfunctions in propionic acidemia. Front. Mol. Neurosci. 18:1499376. doi: 10.3389/fnmol.2025.1499376
Received: 23 September 2024; Accepted: 03 March 2025;
Published: 19 March 2025.
Edited by:
Annelise E. Barron, Stanford University, United StatesReviewed by:
Akihito Yasuoka, Seitoku University, JapanCopyright © 2025 Chen, Cheng and Zhang. This is an open-access article distributed under the terms of the Creative Commons Attribution License (CC BY). The use, distribution or reproduction in other forums is permitted, provided the original author(s) and the copyright owner(s) are credited and that the original publication in this journal is cited, in accordance with accepted academic practice. No use, distribution or reproduction is permitted which does not comply with these terms.
*Correspondence: Xiaoxin Chen, bGNoZW5AY29yaWVsbC5vcmc=; Guo-Fang Zhang, Z3VvZmFuZy56aGFuZ0BkdWtlLmVkdQ==
Disclaimer: All claims expressed in this article are solely those of the authors and do not necessarily represent those of their affiliated organizations, or those of the publisher, the editors and the reviewers. Any product that may be evaluated in this article or claim that may be made by its manufacturer is not guaranteed or endorsed by the publisher.
Research integrity at Frontiers
Learn more about the work of our research integrity team to safeguard the quality of each article we publish.