- Univ Lyon, Université Claude Bernard Lyon 1, CNRS UMR 5310, INSERM U 1217, Institut NeuroMyoGène, Lyon, France
Inhibitory synapses represent a minority of the total chemical synapses in the mammalian brain, yet proper tuning of inhibition is fundamental to shape neuronal network properties. The neurotransmitter γ-aminobutyric acid (GABA) mediates rapid synaptic inhibition by the activation of the type A GABA receptor (GABAAR), a pentameric chloride channel that governs major inhibitory neuronal transduction in the nervous system. Impaired GABA transmission leads to a variety of neuropsychiatric diseases, including schizophrenia, autism, epilepsy or anxiety. From an evolutionary perspective, GABAAR shows remarkable conservations, and are found in all eukaryotic clades and even in bacteria and archaea. Specifically, bona fide GABAARs are found in the nematode Caenorhabditis elegans. Because of the anatomical simplicity of the nervous system and its amenability to genetic manipulations, C. elegans provide a powerful system to investigate the molecular and cellular biology of GABA synapses. In this mini review article, we will introduce the structure of the C. elegans GABAergic system and describe recent advances that have identified novel proteins controlling the localization of GABAARs at synapses. In particular, Ce-Punctin/MADD-4 is an evolutionarily-conserved extracellular matrix protein that behaves as an anterograde synaptic organizer to instruct the excitatory or inhibitory identity of postsynaptic domains.
Introduction
Neurochemical synapses are the elementary structures that process the directional transfer of electrical signals in neural circuits. Based on their molecular composition, synapses probably emerged early during evolution before the divergence of Cnidarians and Bilaterians, more than 1.2 billion years ago (Sakarya et al., 2007; Emes and Grant, 2012). The molecular composition of the synapse shows high conservation. For example, among Bilaterians, a comparison of mouse genes encoding the postsynaptic proteome indicates that ≈45% have detectable orthologs in the ecdysozoans Caenorhabditis elegans or Drosophila melanogaster (Ryan and Grant, 2009). Although synapses were further diversified in the chordate lineage, it is possible to interrogate the general organization and function of chemical synapses in simple invertebrate organisms, and thereby take advantage of their ease of manipulation and the power of their genetic toolkits. In this mini review article, we outline how this strategy was successful in the nematode C. elegans to identify a novel organizer of inhibitory γ-aminobutyric acid (GABA)ergic synapses.
C. elegans is an anatomically-simple, 1 mm-long, non-parasitic nematode. Stereotyped divisions of the zygote, in combination with fixed programmed cell-death events, generate 959 somatic cells in the adult hermaphrodite and 1,033 in the adult male. The adult hermaphrodite contains 302 neurons, most of which are morphologically simple, extending only a few unbranched neurites. The connectivity of the C. elegans nervous system was reconstructed in the 1970s from serial EM sections (White et al., 1986). Connectivity is relatively sparse since the entire network contains less than 10,000 chemical synapses, including 1,500 neuromuscular junctions (NMJs), and about 800 gap junctions. Based on the reconstruction of few independent specimens and the visualization of specific synapses with fluorescent markers, the overall connectivity of the system appears strikingly reproducible among individuals, yet data are currently being generated using modern connectomic techniques to get a better sense of interindividual variability with single-synapse resolution (Mulcahy et al., 2018; Cook et al., 2019). This anatomical simplicity contrasts with the complexity of the molecular repertoire expressed in the nervous system. Although C. elegans contains 108 times fewer neurons than humans, its genome contains about 22,000 genes, which is very comparable with the human gene content. All classes of neurotransmitter systems found in mammals are present within C. elegans (Hobert, 2018), with a remarkable diversity of peptidergic transmission and the expansion of some receptor families, such as nicotinic and olfactory receptors. Specifically, the machinery to synthesize, release and sense the neurotransmitter GABA is remarkably conserved within mammals (Schuske et al., 2004).
GABAergic Neurotransmission in C. elegans
Early mapping of the GABAergic system by anti-GABA immunostaining identified 26 neurons in the C. elegans nervous system: 19 motoneurons (D-class) that establish NMJs on body-wall muscles, four motoneurons (RMEs) that control head muscles, two neurons (AVL and DVB) that innervate intestinal muscles and the interneuron RIS (McIntire et al., 1993b). A recent study identified 10 additional GABA-positive neurons, out of which three express the glutamic acid decarboxylase (GAD)/UNC-25, while the others might accumulate GABA by re-uptake using the plasma membrane transporter GAT/SNF-11 or some uncharacterized mechanisms (Gendrel et al., 2016).
The prominent phenotype caused by impairing GABA neurotransmission in C. elegans is an abnormal locomotion. Unlike mammals, C. elegans body-wall muscles receive both excitatory input from cholinergic motoneurons and inhibitory input from GABAergic motoneurons. When a cholinergic motoneuron releases acetylcholine (ACh), it triggers both muscle contraction and the activation of a downstream GABAergic motoneuron that projects to the opposite muscles, causing their relaxation (Figure 1A). This ensures local out of phase dorsal/ventral contraction/relaxation, the elementary component of sinusoidal locomotion (Jorgensen and Nonet, 1995). Laser ablation of GABAergic motoneurons causes a specific “shrinker” phenotype due to concomitant hyper contraction of both ventral and dorsal muscles when animals try to move backward. Similarly, RME motoneurons relax head muscles during foraging and impairment of GABA neurotransmission impacts head movements. By contrast, ablation of the AVL and DVB neurons causes a “constipated” phenotype because these neurons directly activate (rather than inhibit) the enteric muscles required for expulsion of the intestinal content (McIntire et al., 1993b). GABA-dependent excitation depends on EXP-1, a GABA-sensitive cation channel with the hallmarks of the Cys-loop receptor superfamily (Thomas, 1990; Beg and Jorgensen, 2003).
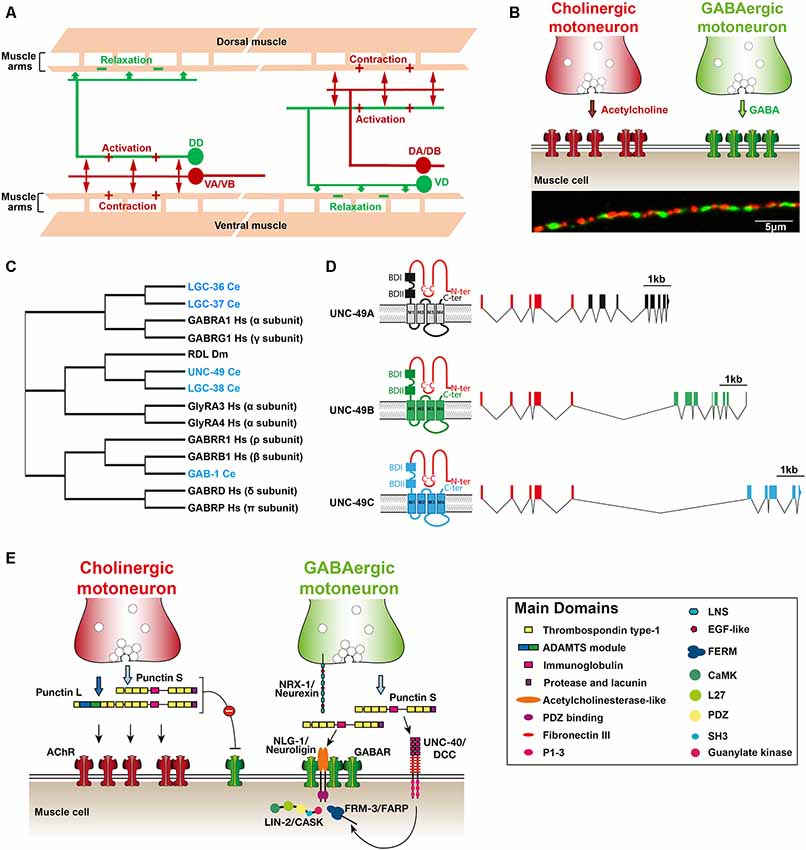
Figure 1. (A) Schematic organization of the C. elegans neuromuscular network. Mononucleated body-wall muscle cells on the ventral and dorsal sides of the worm extend ≈5 muscle arms to contact the axon of cholinergic (red) and γ-aminobutyric acid (GABA)ergic (green) motoneurons along the ventral and dorsal nerve cords, respectively. Cholinergic neurons (VA/VB and DA/DB) form dyadic synapses activating muscle cells and GABAergic motoneurons (DD and VD) that form inhibitory neuromuscular junctions (NMJs) on opposite muscle cells. (B) Distribution of excitatory and inhibitory NMJs along the ventral nerve cord. Upper panel: a schematic drawing showing that each muscle cell receives both cholinergic and GABAergic inputs. Lower panel: immunostaining of cholinergic boutons (anti-UNC-17/VAChT; red) and GABAARs (anti-UNC-49; green) at the dorsal nerve cord. (C) Cladogram showing the phylogenic relationships of the C. elegans genes encoding GABAA receptor subunits (blue). The tree was adapted from Tsang et al. (2007) and Gendrel et al. (2016). Dm, Drosophila melanogaster; Hs, Homo sapiens. (D) Schematic structure of the unc-49 locus encoding the GABAAR present at inhibitory NMJs (adapted from Bamber et al., 1999). The locus generates three distinct subunits by alternative splicing. The first five exons encode most of the extracellular N-terminal, which is common to the three subunits (red). Alternative splicing of 3′ blocks of exons encode the C-terminal part of the A,B and C subunits (black, green and blue, respectively). Putative GABA binding sites (BD) and transmembrane segments are distinct between the different subunits. (E) Working model of GABAAR clustering at NMJ in C. elegans. See the main text for discussion of the model. ADAMTS, a disintegrin and metalloproteinase with thrombospondin; P1-3, protein binding domain 1, 2 and 3; LNS, laminin-neurexin/sex hormone-binding globulin; EGF, epidermal growth factor; FERM, (4.1, ezrin, radixin, moesin) family; SH3, src homology 3 domain; FARP, FERM, ARH/RhoGEF and pleckstrin domain protein; CASK, calcium/calmodulin-dependent serine protein kinase.
The “shrinker” phenotype was used in genetic screens as a proxy to recognize “Uncoordinated” (unc-) mutants with impaired GABA neurotransmission among the initial collection of mutants isolated by Sydney Brenner (Brenner, 1974; Hodgkin, 1983; McIntire et al., 1993a). These included mutants in unc-25, the single gene encoding the GABA-synthetizing enzyme GAD, unc-47, the first gene identified in any species to encode the vesicular GABA Transporter vGAT (McIntire et al., 1997), and unc-49, which codes for the GABAA receptors present at NMJs (Bamber et al., 1999). Interestingly, complete inactivation of GABAergic neurotransmission produces viable mutants that can reproduce under laboratory conditions.
In addition to UNC-49, the C. elegans genome encodes three canonical GABAA receptor subunits, the two alpha-subunit type LGC-36 and LGC-37 and the beta-subunit GAB-1, that are orthologous to GABAAR subunits in mammals (Tsang et al., 2007). UNC-49 and the related receptor LGC-38 are phylogenetically closer to the Drosophila receptor RDL (Figure 1C). Moreover, there are at least two additional bona fide ionotropic GABA receptors, EXP-1 and LGC-35, that are permeable to cations due to specific amino-acid composition of the channel selectivity filter (Beg and Jorgensen, 2003; Jobson et al., 2015). Of the 118 anatomically defined neuron classes of the C. elegans hermaphrodite, 47 neuron classes are innervated by GABAergic neurons (White et al., 1986). Twenty one of these neuron classes express at least one of the aforementioned receptors based on transcriptional reporters. The apparent inability to detect GABAAR expression in the rest of the GABA-innervated neurons might be due to technical limitations. However, it is also likely that additional GABA receptors remain to be characterized because the C. elegans genome contains up to 39 GABA/Glycine receptor-like genes, including Glutamate- and Acetylcholine-gated anion channels (Jones and Sattelle, 2008; Hobert, 2018). Finally, C. elegans expresses two metabotropic GABA receptors for which a comprehensive expression pattern remains to be described (Dittman and Kaplan, 2008; Schultheis et al., 2011). Interestingly, a number of neurons that do not receive direct GABAergic inputs still express GABAA receptors. These receptors may mediate GABA spillover transmission as demonstrated for LGC-35, which activates cholinergic motoneurons when GABA is released by GABAergic motoneurons (Jobson et al., 2015). Notably, our knowledge of the roles of GABAARs beyond the NMJ in C. elegans is rudimentary since the cellular localization and function of every canonical GABAARs still remains to be characterized.
The GABAergic Neuromuscular Junction, A Genetically-Tractable Model of Inhibitory Synapse
Paradoxically, the best characterized inhibitory synapse in C. elegans is the GABAergic NMJ, which might relate more closely to neuro-neuronal synapses than to “standard” NMJs. Invertebrates or Drosophila, motoneurons establish a single NMJ with myofibers containing hundreds to thousands of nuclei. This differs from neuronal innervation, where a single neuron typically receives thousands of excitatory and inhibitory inputs, building a mosaic of specialized domains concentrating receptors to match presynaptic inputs. Interestingly, the anatomical organization of the C. elegans neuromuscular system provides a means to interrogate a number of questions which may more closely relate to the innervation of vertebrate neurons.
First, C. elegans body-wall muscle cells do not fuse and remain mononucleated. Second, they send dendrite-like extensions that contact and extend along the motoneurons that run in the ventral and dorsal cords and form “en-passant” synapses. Third, as presented above, each muscle cell receives both excitatory cholinergic and inhibitory GABAergic inputs from distinct classes of motoneurons (Figures 1A,B). Based on functional (Liu et al., 2007) and EM data (White et al., 1986), each synaptic bouton likely activates receptors present on more than one postsynaptic muscle arm facing the presynaptic active zone. Hence, the C. elegans neuromuscular arrangement represents a very simple poly-neuronal innervation system. Specifically, it can be used to interrogate how specific compartments are built on the plasma membrane to concentrate on different neurotransmitter receptors in front of the corresponding neurotransmitter release sites.
C. elegans development is fast. Fourteen hours after fertilization, eggs hatch as the first larval stage. Development then proceeds through four larval stages (called L1 to L4), each separated by a molt, and reach adulthood within 2.5 days at 20°C. At hatching, only six dorsal D-class (DD) motoneurons have been generated and innervate ventral body-wall muscles. The 13 ventral D-class (VD) motoneurons differentiate during the first larval stage. DD neurons rewire at the end of the L1 stage to innervate dorsal muscles while VD neurons innervate ventral muscles (White et al., 1978; Kurup and Jin, 2015). Although the adult is about 10× bigger than L1 larvae, the number of inhibitory NMJs does not increase. Rather, additional active zones form in presynaptic boutons to scale up inhibition (Yeh et al., 2005). Because of the relatively sparse distribution of presynaptic boutons and their highly reproducible patterns across animals, forward genetic screens were successful in identifying mutants with abnormal synapses using fluorescently-tagged presynaptic proteins expressed in GABA motoneurons. These screens were extremely powerful and identified multiple proteins required for the general organization of active zones in neurons such as SYD-2/Liprin, SYD-1, and RPM-1, a founding member of the PHR (Phr1/MYCBP2, highwire and RPM-1) family of proteins (Zhen and Jin, 1999; Zhen et al., 2000; Hallam et al., 2002). However, these screens did not identify proteins specifically involved in the differentiation of inhibitory synapses.
The UNC-49 GABAA receptors are generated from a single complex locus, which generates at least three different subunits (A, B and C) by alternative splicing (Figure 1D). A block of exons encodes most of the extracellular N-terminal domain, which is shared by all subunits, while exons coding for transmembrane regions is specific to each subunit. In Xenopus oocytes, functional GABA receptors can be reconstituted by expressing the B-subunit either alone or in combination with the C-subunit (Bamber et al., 1999). UNC-49B and UNC-49B/C have distinct pharmacology. The positive allosteric regulator diazepam, instead of activating GABAARs, inhibits the GABA-evoked UNC-49B/C current while it has no obvious effect on the UNC-49B homomer. Neurosteroids such as pregnenolone sulfate, that enhances GABA-evoked currents in mammals, have a strong inhibitory effect on the UNC-49B receptor and much weaker effects on UNC-49-B/C. UNC-49B homomers were also found to be sensitive to the broadly-active inhibitor picrotoxin, while UNC-49B/C heteromers are resistant to it (Bamber et al., 2003).
The development of a dissection technique for adult C. elegans enabled stable, whole-cell voltage-clamp recording from ventral medial muscle cells and gave access to native GABAARs (Richmond and Jorgensen, 1999). Spontaneous GABAergic synaptic currents can be isolated either after the pharmacological block of AChRs (Richmond et al., 1999) or by using recording solutions that discriminate excitatory and inhibitory postsynaptic currents (Vashlishan et al., 2008). The total amount of GABAAR present at the muscle cell surface is usually probed by measuring the response to pressure-application of the general agonist muscimol, and the synaptic pool can be activated after optogenetic stimulation of GABA motoneurons (Liewald et al., 2008). in vivo recordings and the pharmacological analyses of endogenous GABAARs indicate that they are likely composed of UNC-49B/C heteromers.
UNC-49 GABAA receptors are clustered in register with presynaptic GABAergic boutons (Figure 1B). Clustering depends on presynaptic innervation and occurs concomitantly with presynaptic differentiation based on the visualization of fluorescently-tagged synaptic proteins (Gally and Bessereau, 2003). However, a detailed longitudinal analysis is still missing to ascertain the precise temporal relationship between pre- and postsynaptic differentiation. Remarkably, in mutants that do not synthesize GABA, both pre- and postsynaptic structures are indistinguishable from wild type, demonstrating that “inhibitory” synapses differentiate in the absence of neurotransmission (Gally and Bessereau, 2003). This situation is not unique since various synaptic types were also reported to differentiate in mammalian cell cultures and in mice in the absence of neurotransmitter release (Misgeld et al., 2002; Varoqueaux et al., 2002; Sigler et al., 2017). Fluorescently-tagged UNC-49 receptors remain functional. Again, because the distribution of these receptors is stereotyped, screens for mutants with abnormal fluorescence distribution identified factors specifically required for the differentiation and organization of GABA NMJs, as described below.
Molecules Basis of UNC-49 Receptor Clustering in C. elegans
In mammalian neurons, GABAARs clustering mostly relies on the scaffolding protein gephyrin that is hypothesized to form an intracellular lattice providing anchoring sites for synaptic GABAARs (Fritschy et al., 2008; Tyagarajan and Fritschy, 2014). Collybistin, a GTP/GDP exchange factor (GEF) interacts with the synaptic adhesion protein Neuroligin-2 and promotes the clustering of gephyrin and GABAARs (Kins et al., 2000; Tyagarajan et al., 2011). Although Gephyrin acts as a prominent player for GABAARs synaptic clustering, gephyrin-independent GABAAR clustering can occur and the requirement of gephyrin for GABAAR clustering is dependent on neuronal and synapse type (Kneussel et al., 2001; Tretter et al., 2012). Interestingly, gephyrin and collybistin are not conserved in C. elegans, giving access to a different molecular organization for GABAAR clustering.
Ce-Punctin/MADD-4
The postsynaptic assembly of cholinergic and GABAergic NMJs in C. elegans relies on a recently-identified anterograde synaptic organizer Ce-Punctin/MADD-4 (Muscle Arm Development Defective-4). Ce-Punctin belongs to a family of poorly characterized extracellular matrix proteins, the ADAMTS-like proteins, that contain multiple thrombospondin-repeat, immunoglobulin, and structurally-unsolved domains (Apte, 2009). There are two madd-4 orthologs in vertebrates, Punctin1/ADAMTSL1 and Punctin2/ADAMTSL3. The precise function of these genes is unknown. However, a variant of Punctin1 was recently shown to cause a complex phenotype including congenital glaucoma, craniofacial and other systemic features (Hendee et al., 2017). Punctin2 is expressed in the brain and was identified as a susceptibility gene for schizophrenia (Dow et al., 2011). Whether these proteins are involved in synaptic organization has not been determined.
Ce-punctin generates long (Punctin L) and short (Punctin S) isoforms by the use of alternative promoters. Punctin S was initially found to attract muscle arm growth and be required for midline-oriented guidance in C. elegans (Seetharaman et al., 2011). The role of Ce-punctin in synaptic organization was subsequently identified in a visual screen for mutants with abnormal positioning of fluorescently-tagged AChRs at NMJs (Pinan-Lucarré et al., 2014). Punctin L is only expressed in cholinergic motoneurons and secreted in the synaptic cleft where it triggers postsynaptic clustering of AChRs. Punctin S is expressed in both cholinergic and GABAergic neurons (Figure 1E). At cholinergic synapses, Punctin S inhibits the attraction of GABAARs by Punctin L, possibly following heterodimerization of the L and S isoforms. At GABAergic synapses, Punctin S promotes the clustering of GABAARs in front of presynaptic GABA boutons. Genetic inactivation of Punctin S does not alter presynaptic GABA boutons, but GABAARs relocalize at cholinergic synapses. Conversely, forced expression of Punctin L in GABAergic motoneurons in a punctin null mutant triggers the colocalization of AChRs and GABAARs opposed to GABAergic boutons (Pinan-Lucarré et al., 2014). These results demonstrated that the identity of pre- and post-synaptic domains can be genetically uncoupled in vivo.
Interestingly, the expression of Punctin is under direct regulation of the transcription factors that specify the terminal identity of motoneurons. The phylogenetically conserved transcription factor UNC-3 controls the expression of numerous genes required for the cholinergic neurotransmission. It also directly activates the transcription of punctin L and S isoforms in cholinergic motoneurons (Kratsios et al., 2015). Similarly, the homeobox transcription factor UNC-30 controls the GABAergic identity of D-type motoneurons and regulates the expression of punctin S (P. Kratsios and O. Hobert, personal communication). Therefore, coordinated control of motoneuron identity and Punctin expression provides a means to ensure proper coupling between presynaptic identity and postsynaptic differentiation.
NLG-1/Neuroligin
The clustering of GABAARs at C. elegans NMJs requires the synaptic adhesion molecule neuroligin NLG-1. Neuroligins (NLs) are evolutionary ancient proteins that are readily detected in Bilaterians (Lenfant et al., 2014). The human genome encodes 5 NLs that support trans-synaptic adhesive functions at excitatory and inhibitory synapses and contribute to postsynaptic receptor clustering (for review see Südhof, 2008). The C. elegans genome contains only one NL-coding gene, nlg-1, which is expressed in multiple types of neurons and in the muscle (Hunter et al., 2010). NLG-1 shares about 25% identity with human NLs and cannot be related to one specific paralog. However, the core protein organization is conserved between mammals and the nematode (Calahorro, 2014). Three main NLG-1 isoforms are generated by alternative splicing of exons encoding cytoplasmic domains of the protein (Calahorro et al., 2015). This splicing seems developmentally regulated but the precise complement of NLG-1 isoforms expressed in neurons and muscle and its functional relevance remains to be analyzed.
In muscle, NLG-1 is only found at GABAergic NMJs and strictly colocalizes with the UNC-49 GABAARs (Maro et al., 2015; Tu et al., 2015). Disruption of nlg-1 causes a redistribution of the GABAARs out of the GABA receptor domains and a reduction of the frequency and amplitude of spontaneous miniature inhibitory postsynaptic currents (mIPSCs). The synaptic localization of NLG-1 depends on Punctin S, which directly binds the NLG-1 ectodomain. The intracellular moiety of NLG-1 is dispensable for its synaptic localization but is required for its ability to cluster GABAARs (Maro et al., 2015; Tu et al., 2015).
GABA motoneurons also express NRX-1, the sole ortholog of the mammalian neurexins that are presynaptic ligands of neuroligins (reviewed in Südhof, 2008). NRX-1 is present at presynaptic sites of GABAergic NMJs but is not required for the synaptic localization of NLG-1. Based on genetic evidence, NRX-1 was proposed to work in parallel with Punctin to promote the clustering of GABAARs (Maro et al., 2015). The nrx-1 mutant used in this study no longer expressed the NRX-1 γ isoform, which was recently shown to be important for the presynaptic organization (Kurshan et al., 2018). However, most of the ectodomain of the NRX-1 α isoform potentially remained synthesized. Therefore, the positive interaction between NRX-1 and Punctin at GABA synapses remains to be further investigated in this system.
UNC-40/DCC
At the C. elegans NMJ, the synaptic content of GABAARs depends on the netrin receptor UNC-40/DCC (deleted in colorectal cancer; Tu et al., 2015). This receptor has been implicated in a wide range of developmental events involving cellular migration and axonal navigation (Chan et al., 1996; Keino-Masu et al., 1996). It is a single transmembrane domain protein that does not contain any obvious catalytic domain. Upon netrin binding, UNC-40 is believed to dimerize, causing the intracellular domains to serve as a signaling platform to recruit or activate numerous downstream targets, including several signal transduction molecules that regulate cytoskeletal dynamics (for reviews see Finci et al., 2015; Boyer and Gupton, 2018).
In C. elegans, UNC-40 plays a specific role in the neuromuscular system. First, it promotes the growth of muscle arms (Alexander et al., 2009). At the early larval stage, the Punctin S localizes UNC-40 at the tip of the muscle arms and, together with the guidance cue UNC-6/netrin, activates UNC-40. Thus, the number of muscle arms that project to the ventral and dorsal nerve cords is drastically reduced in unc-40 mutants. However, the number of GABAergic boutons is unaffected and NLG-1 postsynaptic clusters remain readily detected. In addition, UNC-40 controls the amount of GABAARs at synapses. In unc-40 mutants, there is a 60% reduction of receptors at GABAergic NMJs. A constitutively-activated version of UNC-40, which only contains the intracellular moiety of UNC-40 targeted to the plasma membrane, rescues the synaptic clustering of GABAARs (Tu et al., 2015). This suggests that upon activation by Punctin, UNC-40 promotes the recruitment of GABAARs onto NLG-1 clusters.
FRM-3/FARP and LIN-2/Cask
Recently, the intracellular proteins FRM-3 and LIN-2 were reported to regulate GABAARs at NMJs (Tong et al., 2015). FRM-3 was initially described as a band 4.1 (EPB4.1) paralog (Tong et al., 2015), but the recently annotated FRM-3B isoform appears to be the unambiguous ortholog of the mammalian FARP1 and FARP2 proteins. FARPs are able to modulate F-actin assembly and regulate neuronal development and synaptogenesis by interacting with cell-surface proteins such as SynCAM1 and class A Plexins (Cheadle and Biederer, 2012, 2014). In C. elegans, FRM-3 binds the intracellular loop of the UNC-49B subunit (Tong et al., 2015). It also binds LIN-2, the ortholog of CASK, a membrane-associated guanylate kinase (MAGUK) multidomain scaffolding molecule (Hata et al., 1996) that plays important role in presynaptic assembly at mammalian synapses. At GABAergic NMJs, disruption of frm-3 or lin-2 causes about 25% reduction of mIPSC amplitude, while pressure-applied response to the GABAARs agonist muscimol was unchanged, indicating that receptors were expressed but not properly clustered at synapses. FRM-3 and LIN-2 were proposed to stabilize GABAARs at the synapse, in parallel to a Neuroligin-dependent scaffold (Tong et al., 2015). The precise interplay between these different proteins remains to be characterized further.
Conclusion
GABAergic inhibitory neurotransmission is an evolutionarily ancient system that has been conserved over ≈1,000 million years of evolution. Genetic analysis in C. elegans identified a novel anterograde synaptic organizer, Ce-Punctin, which specifies the position of post-synaptic domains by localizing neuroligin in register with synaptic boutons, and controls the number of postsynaptic receptors through the activation of UNC-40/DCC (Figure 1E). The conservation of this system still remains to be tested in mammals. Even at the C. elegans NMJ, several questions remain unanswered: how is Punctin secreted and confined at synapses? What are the mechanisms that differentiate Punctin function at cholinergic and GABAergic synapses? To what extent is this system regulated by synaptic activity? Most surprisingly, it is amazing to see that the cellular and molecular basis of synaptic neuro-neuronal GABA transmission in C. elegans remains terra incognita. Lots remain to be learned.
Author Contributions
XZ and J-LB wrote the manuscript.
Funding
This work was supported by the Programme Avenir Lyon Saint-Etienne, the Agence Nationale de la Recherche (ANR) grant GABAREL ANR-15-CE11-0016-01 and the European Research Council (ERC_Adg C.NAPSE #695295).
Conflict of Interest
The authors declare that the research was conducted in the absence of any commercial or financial relationships that could be construed as a potential conflict of interest.
Acknowledgments
We thank Maëlle Jospin and Thomas Boulin for critical reading of the manuscript and Camilla Luccardini for helping in figure design.
References
Alexander, M., Chan, K. K. M., Byrne, A. B., Selman, G., Lee, T., Ono, J., et al. (2009). An UNC-40 pathway directs postsynaptic membrane extension in Caenorhabditis elegans. Development 136, 911–922. doi: 10.1242/dev.030759
Apte, S. S. (2009). A disintegrin-like and metalloprotease (reprolysin-type) with thrombospondin type 1 motif (ADAMTS) superfamily: functions and mechanisms. J. Biol. Chem. 284, 31493–31497. doi: 10.1074/jbc.r109.052340
Bamber, B. A., Beg, A. A., Twyman, R. E., and Jorgensen, E. M. (1999). The Caenorhabditis elegans unc-49 locus encodes multiple subunits of a heteromultimeric GABA receptor. J. Neurosci. 19, 5348–5359. doi: 10.1523/JNEUROSCI.19-13-05348.1999
Bamber, B. A., Twyman, R. E., and Jorgensen, E. M. (2003). Pharmacological characterization of the homomeric and heteromeric UNC-49 GABA receptors in C. elegans. Br. J. Pharmacol. 138, 883–893. doi: 10.1038/sj.bjp.0705119
Beg, A. A., and Jorgensen, E. M. (2003). EXP-1 is an excitatory GABA-gated cation channel. Nat. Neurosci. 6, 1145–1152. doi: 10.1038/nn1136
Boyer, N. P., and Gupton, S. L. (2018). Revisiting netrin-1: one who guides (Axons). Front. Cell. Neurosci. 12:221. doi: 10.3389/fncel.2018.00221
Calahorro, F. (2014). Conserved and divergent processing of neuroligin and neurexin genes: from the nematode C. elegans to human. Invert. Neurosci. 14, 79–90. doi: 10.1007/s10158-014-0173-5
Calahorro, F., Holden-Dye, L., and O’Connor, V. (2015). Analysis of splice variants for the C. elegans orthologue of human neuroligin reveals a developmentally regulated transcript. Gene Expr. Patterns 17, 69–78. doi: 10.1016/j.gep.2015.02.002
Chan, S. S.-Y., Zheng, H., Su, M.-W., Wilk, R., Killeen, M. T., Hedgecock, E. M., et al. (1996). UNC-40, a C. elegans homolog of DCC (deleted in colorectal cancer), is required in motile cells responding to UNC-6 netrin cues. Cell 87, 187–195. doi: 10.1016/s0092-8674(00)81337-9
Cheadle, L., and Biederer, T. (2012). The novel synaptogenic protein Farp1 links postsynaptic cytoskeletal dynamics and transsynaptic organization. J. Cell Biol. 199, 985–1001. doi: 10.1083/jcb.201205041
Cheadle, L., and Biederer, T. (2014). Activity-dependent regulation of dendritic complexity by semaphorin 3A through Farp1. J. Neurosci. 34, 7999–8009. doi: 10.1523/jneurosci.3950-13.2014
Cook, S. J., Jarrell, T. A., Brittin, C. A., Wang, Y., Bloniarz, A. E., Yakovlev, M. A., et al. (2019). Whole-animal connectomes of both Caenorhabditis elegans sexes. Nature 571, 63–71. doi: 10.1038/s41586-019-1352-7
Dittman, J. S., and Kaplan, J. M. (2008). Behavioral impact of neurotransmitter-activated GPCRs: muscarinic and GABAB receptors regulate C. elegans locomotion. J. Neurosci. 28, 7104–7112. doi: 10.1523/jneurosci.0378-08.2008
Dow, D. J., Huxley-Jones, J., Hall, J. M., Francks, C., Maycox, P. R., Kew, J. N. C., et al. (2011). ADAMTSL3 as a candidate gene for schizophrenia: gene sequencing and ultra-high density association analysis by imputation. Schizophr. Res. 127, 28–34. doi: 10.1016/j.schres.2010.12.009
Emes, R. D., and Grant, S. G. N. (2012). Evolution of synapse complexity and diversity. Ann. Rev. Neurosci. 35, 111–131. doi: 10.1146/annurev-neuro-062111-150433
Finci, L., Zhang, Y., Meijers, R., and Wang, J.-H. (2015). Signaling mechanism of the netrin-1 receptor DCC in axon guidance. Prog. Biophys. Mol. Biol. 118, 153–160. doi: 10.1016/j.pbiomolbio.2015.04.001
Fritschy, J.-M., Harvey, R. J., and Schwarz, G. (2008). Gephyrin: where do we stand, where do we go? Trends Neurosci. 31, 257–264. doi: 10.1016/j.tins.2008.02.006
Gally, C., and Bessereau, J.-L. (2003). GABA is dispensable for the formation of junctional GABA receptor clusters in Caenorhabditis elegans. J. Neurosci. 23, 2591–2599. doi: 10.1523/jneurosci.23-07-02591.2003
Gendrel, M., Atlas, E. G., and Hobert, O. (2016). A cellular and regulatory map of the GABAergic nervous system of C. elegans. Elife 5:e17686. doi: 10.7554/eLife.17686
Hallam, S. J., Goncharov, A., McEwen, J., Baran, R., and Jin, Y. (2002). SYD-1, a presynaptic protein with PDZ, C2 and rhoGAP-like domains, specifies axon identity in C. elegans. Nat. Neurosci. 5, 1137–1146. doi: 10.1038/nn959
Hata, Y., Butz, S., and Südhof, T. C. (1996). CASK: a novel dlg/PSD95 homolog with an N-terminal calmodulin-dependent protein kinase domain identified by interaction with neurexins. J. Neurosci. 16, 2488–2494. doi: 10.1523/jneurosci.16-08-02488.1996
Hendee, K., Wang, L. W., Reis, L. M., Rice, G. M., Apte, S. S., and Semina, E. V. (2017). Identification and functional analysis of an ADAMTSL1 variant associated with a complex phenotype including congenital glaucoma, craniofacial and other systemic features in a three generation human pedigree. Hum. Mutat. 38, 1485–1490. doi: 10.1002/humu.23299
Hobert, O. (2018). The neuronal genome of Caenorhabditis elegans. WormBook 13, 1–106. doi: 10.1895/wormbook.1.161.1
Hodgkin, J. (1983). Male phenotypes and mating efficiency in Caenorhabditis elegans. Genetics 103, 43–64.
Hunter, J. W., Mullen, G. P., McManus, J. R., Heatherly, J. M., Duke, A., and Rand, J. B. (2010). Neuroligin-deficient mutants of C. elegans have sensory processing deficits and are hypersensitive to oxidative stress and mercury toxicity. Dis. Model. Mech. 3, 366–376. doi: 10.1242/dmm.003442
Jobson, M. A., Valdez, C. M., Gardner, J., Garcia, L. R., Jorgensen, E. M., and Beg, A. A. (2015). Spillover transmission is mediated by the excitatory GABA receptor LGC-35 in C. elegans. J. Neurosci. 35, 2803–2816. doi: 10.1523/jneurosci.4557-14.2015
Jones, A., and Sattelle, D. (2008). The cys-loop ligand-gated ion channel gene superfamily of the nematode, Caenorhabditis elegans. Invert. Neurosci. 8, 41–47. doi: 10.1007/s10158-008-0068-4
Jorgensen, E. M., and Nonet, M. L. (1995). Neuromuscular junctions in the nematode C. elegans. Semin. Dev. Biol. 6, 207–220. doi: 10.1016/s1044-5781(06)80030-7
Keino-Masu, K., Masu, M., Hinck, L., Leonardo, E. D., Chan, S. S.-Y., Culotti, J. G., et al. (1996). Deleted in colorectal cancer (DCC) encodes a netrin receptor. Cell 87, 175–185. doi: 10.1016/s0092-8674(00)81336-7
Kins, S., Betz, H., and Kirsch, J. (2000). Collybistin, a newly identified brain-specific GEF, induces submembrane clustering of gephyrin. Nat. Neurosci. 3, 22–29. doi: 10.1038/71096
Kneussel, M., Helmut Brandstätter, J., Gasnier, B., Feng, G., Sanes, J. R., and Betz, H. (2001). Gephyrin-independent clustering of postsynaptic GABAA receptor subtypes. Mol. Cell. Neurosci. 17, 973–982. doi: 10.1006/mcne.2001.0983
Kratsios, P., Pinan-Lucarré, B., Kerk, S. Y., Weinreb, A., Bessereau, J.-L., and Hobert, O. (2015). Transcriptional coordination of synaptogenesis and neurotransmitter signaling. Curr. Biol. 25, 1282–1295. doi: 10.1016/j.cub.2015.03.028
Kurshan, P. T., Merrill, S. A., Dong, Y., Ding, C., Hammarlund, M., Bai, J., et al. (2018). γ-neurexin and frizzled mediate parallel synapse assembly pathways antagonized by receptor endocytosis. Neuron 100, 150.e4–166.e4. doi: 10.1016/j.neuron.2018.09.007
Kurup, N., and Jin, Y. (2015). Neural circuit rewiring: insights from DD synapse remodeling. Worm 5:e1129486. doi: 10.1080/21624054.2015.1129486
Lenfant, N., Hotelier, T., Bourne, Y., Marchot, P., and Chatonnet, A. (2014). Tracking the origin and divergence of cholinesterases and neuroligins: the evolution of synaptic proteins. J. Mol. Neurosci. 53, 362–369. doi: 10.1007/s12031-013-0194-2
Liewald, J. F., Brauner, M., Stephens, G. J., Bouhours, M., Schultheis, C., Zhen, M., et al. (2008). Optogenetic analysis of synaptic function. Nat. Methods 5, 895–902. doi: 10.1038/nmeth.1252
Liu, Q., Chen, B., Hall, D. H., and Wang, Z.-W. (2007). A quantum of neurotransmitter causes minis in multiple postsynaptic cells at the Caenorhabditis elegans neuromuscular junction. Dev. Neurobiol. 67, 123–128. doi: 10.1002/dneu.20307
Maro, G. S., Gao, S., Olechwier, A. M., Hung, W. L., Liu, M., Özkan, E., et al. (2015). MADD-4/punctin and neurexin Organize C. elegans GABAergic postsynapses through neuroligin. Neuron 86, 1420–1432. doi: 10.1016/j.neuron.2015.05.015
McIntire, S. L., Jorgensen, E., and Horvitz, H. R. (1993a). Genes required for GABA function in Caenorhabditis elegans. Nature 364, 334–337. doi: 10.1038/364334a0
McIntire, S. L., Jorgensen, E., Kaplan, J., and Horvitz, H. R. (1993b). The GABAergic nervous system of Caenorhabditis elegans. Nature 364, 337–341. doi: 10.1038/364337a0
McIntire, S. L., Reimer, R. J., Schuske, K., Edwards, R. H., and Jorgensen, E. M. (1997). Identification and characterization of the vesicular GABA transporter. Nature 389, 870–876. doi: 10.1038/39908
Misgeld, T., Burgess, R. W., Lewis, R. M., Cunningham, J. M., Lichtman, J. W., and Sanes, J. R. (2002). Roles of neurotransmitter in synapse formation: development of neuromuscular junctions lacking choline acetyltransferase. Neuron 36, 635–648. doi: 10.1016/s0896-6273(02)01020-6
Mulcahy, B., Witvliet, D., Holmyard, D., Mitchell, J., Chisholm, A. D., Meirovitch, Y., et al. (2018). A pipeline for volume electron microscopy of the Caenorhabditis elegans nervous system. Front. Neural Circuits 12:94. doi: 10.3389/fncir.2018.00094
Pinan-Lucarré, B., Tu, H., Pierron, M., Cruceyra, P. I., Zhan, H., Stigloher, C., et al. (2014). C. elegans Punctin specifies cholinergic versus GABAergic identity of postsynaptic domains. Nature 511, 466–470. doi: 10.1038/nature13313
Richmond, J. E., Davis, W. S., and Jorgensen, E. M. (1999). UNC-13 is required for synaptic vesicle fusion in C. elegans. Nat. Neurosci. 2, 959–964. doi: 10.1038/14755
Richmond, J. E., and Jorgensen, E. M. (1999). One GABA and two acetylcholine receptors function at the C. elegans neuromuscular junction. Nat. Neurosci. 2, 791–797. doi: 10.1038/12160
Ryan, T. J., and Grant, S. G. N. (2009). The origin and evolution of synapses. Nat. Rev. Neurosci. 10, 701–712. doi: 10.1038/nrn2717
Sakarya, O., Armstrong, K. A., Adamska, M., Adamski, M., Wang, I.-F., Tidor, B., et al. (2007). A post-synaptic scaffold at the origin of the animal kingdom. PLoS One 2:e506. doi: 10.1371/journal.pone.0000506
Schultheis, C., Brauner, M., Liewald, J. F., and Gottschalk, A. (2011). Optogenetic analysis of GABAB receptor signaling in Caenorhabditis elegans motor neurons. J. Neurophysiol. 106, 817–827. doi: 10.1152/jn.00578.2010
Schuske, K., Beg, A. A., and Jorgensen, E. M. (2004). The GABA nervous system in C. elegans. Trends in Neurosci. 27, 407–414. doi: 10.1016/j.tins.2004.05.005
Seetharaman, A., Selman, G., Puckrin, R., Barbier, L., Wong, E., D’Souza, S. A., et al. (2011). MADD-4 is a secreted cue required for midline-oriented guidance in Caenorhabditis elegans. Dev. Cell 21, 669–680. doi: 10.1016/j.devcel.2011.07.020
Sigler, A., Oh, W. C., Imig, C., Altas, B., Kawabe, H., Cooper, B. H., et al. (2017). Formation and maintenance of functional spines in the absence of presynaptic glutamate release. Neuron 94, 304.e4–311.e4. doi: 10.1016/j.neuron.2017.03.029
Südhof, T. C. (2008). Neuroligins and neurexins link synaptic function to cognitive disease. Nature 455, 903–911. doi: 10.1038/nature07456
Thomas, J. H. (1990). Genetic analysis of defecation in Caenorhabditis elegans. Genetics 124, 855–872.
Tong, X.-J., Hu, Z., Liu, Y., Anderson, D., and Kaplan, J. M. (2015). A network of autism linked genes stabilizes two pools of synaptic GABAA receptors. ELife 4:e09648. doi: 10.7554/eLife.09648
Tretter, V. E., Mukherjee, J., Maric, H. M., Schindelin, H., Sieghart, W., and Moss, S. J. (2012). Gephyrin, the enigmatic organizer at GABAergic synapses. Front. Cell. Neurosci. 6:23. doi: 10.3389/fncel.2012.00023
Tsang, S.-Y., Ng, S.-K., Xu, Z., and Xue, H. (2007). The evolution of GABAA receptor-like genes. Mol. Biol. Evol. 24, 599–610. doi: 10.1093/molbev/msl188
Tu, H., Pinan-Lucarré, B., Ji, T., Jospin, M., and Bessereau, J.-L. (2015). C. elegans punctin clusters GABAA receptors via neuroligin binding and UNC-40/DCC recruitment. Neuron 86, 1407–1419. doi: 10.1016/j.neuron.2015.05.013
Tyagarajan, S. K., and Fritschy, J.-M. (2014). Gephyrin: a master regulator of neuronal function? Nat. Rev. Neurosci. 15, 141–156. doi: 10.1038/nrn3670
Tyagarajan, S. K., Ghosh, H., Harvey, K., and Fritschy, J.-M. (2011). Collybistin splice variants differentially interact with gephyrin and Cdc42 to regulate gephyrin clustering at GABAergic synapses. J. Cell Sci. 124, 2786–2796. doi: 10.1242/jcs.086199
Varoqueaux, F., Sigler, A., Rhee, J.-S., Brose, N., Enk, C., Reim, K., et al. (2002). Total arrest of spontaneous and evoked synaptic transmission but normal synaptogenesis in the absence of Munc13-mediated vesicle priming. Proc. Natl. Acad. Sci. U S A 99, 9037–9042. doi: 10.1073/pnas.122623799
Vashlishan, A. B., Madison, J. M., Dybbs, M., Bai, J., Sieburth, D., Ch’ng, Q., et al. (2008). An RNAi screen identifies genes that regulate GABA synapses. Neuron 58, 346–361. doi: 10.1016/j.neuron.2008.02.019
White, J. G., Albertson, D. G., and Anness, M. A. R. (1978). Connectivity changes in a class of motoneurone during the development of a nematode. Nature 271, 764–766. doi: 10.1038/271764a0
White, J. G., Southgate, E., Thomson, J. N., and Brenner, S. (1986). The structure of the nervous system of the nematode Caenorhabditis elegans. Philos. Trans. R. Soc. Lond. B Biol. Sci. 314, 1–340. doi: 10.1098/rstb.1986.0056
Yeh, E., Kawano, T., Weimer, R. M., Bessereau, J.-L., and Zhen, M. (2005). Identification of genes involved in synaptogenesis using a fluorescent active zone marker in Caenorhabditis elegans. J. Neurosci. 25, 3833–3841. doi: 10.1523/JNEUROSCI.4978-04.2005
Zhen, M., Huang, X., Bamber, B., and Jin, Y. (2000). Regulation of presynaptic terminal organization by C. elegans RPM-1, a putative guanine nucleotide exchanger with a RING-H2 finger domain. Neuron 26, 331–343. doi: 10.1016/s0896-6273(00)81167-8
Keywords: GABAA receptor, C. elegans, neuromuscular junction, punctin, neuroligin
Citation: Zhou X and Bessereau J-L (2019) Molecular Architecture of Genetically-Tractable GABA Synapses in C. elegans. Front. Mol. Neurosci. 12:304. doi: 10.3389/fnmol.2019.00304
Received: 05 August 2019; Accepted: 26 November 2019;
Published: 12 December 2019.
Edited by:
Shiva K. Tyagarajan, University of Zurich, SwitzerlandReviewed by:
Jasmina N. Jovanovic, UCL School of Pharmacy, United KingdomAngelo Keramidas, University of Queensland, Australia
Copyright © 2019 Zhou and Bessereau. This is an open-access article distributed under the terms of the Creative Commons Attribution License (CC BY). The use, distribution or reproduction in other forums is permitted, provided the original author(s) and the copyright owner(s) are credited and that the original publication in this journal is cited, in accordance with accepted academic practice. No use, distribution or reproduction is permitted which does not comply with these terms.
*Correspondence: Jean-Louis Bessereau, jean-louis.bessereau@univ-lyon1.fr