- 1Queensland Brain Institute, University of Queensland, St Lucia, QLD, Australia
- 2Life Sciences Institute and Department of Molecular and Integrative Physiology, University of Michigan, Ann Arbor, MI, United States
- 3College of Life Science and Technology, Key laboratory of Molecular Biophysics of MOE, International Research Center for Sensory Biology and Technology of MOST, Huazhong University of Science and Technology, Wuhan, Hubei, China
- 4Department of Microbiology and Physiological Systems, University of Massachusetts Medical School, Worcester, MA, United States
- 5Division of Biological Sciences, University of Montana, Missoula, MT, United States
How neural circuits drive behavior is a central question in neuroscience. Proper execution of motor behavior requires precise coordination of many neurons. Within a motor circuit, individual neurons tend to play discrete roles by promoting or suppressing motor output. How exactly neurons function in specific roles to fine tune motor output is not well understood. In C. elegans, the interneuron RIM plays important yet complex roles in locomotion behavior. Here, we show that RIM both promotes and suppresses distinct features of locomotion behavior to fine tune motor output. This dual function is achieved via the excitation and inhibition of the same motor circuit by electrical and chemical neurotransmission, respectively. Additionally, this bi-directional regulation contributes to motor adaptation in animals placed in novel environments. Our findings reveal that individual neurons within a neural circuit may act in opposing ways to regulate circuit dynamics to fine tune behavioral output.
Introduction
Animals execute a wide range of behavior, which rely on the vast number of neurons in the brain. Control of motor output is an essential feature of the nervous system in nearly all animals, and the successful execution of even the simplest motor behavior requires precise coordination of many individual neurons (Purves et al., 2008). For example, a simple withdrawal behavior in land snails involves different groups of neurons, including sensory, motor, modulatory, and command neurons (Balaban, 2002). Individual neurons within circuits tend to play discrete roles in either promoting or suppressing motor output. For example in the mammalian motor cortex output circuit, distinct neurons release glutamate or GABA, to form a feedforward excitatory or inhibitory circuit, respectively, to regulate motor outputs (Cote et al., 2018). However, how individual neurons coordinate within a functional circuit to generate motor output is not well understood.
C. elegans has emerged as a highly valuable model to investigate the mechanisms by which neural circuits control behavior. C. elegans possess a simple nervous system composed of 302 neurons, approximately 7,000 chemical synapses, and 900 electrical junctions (White et al., 1986). These elements together generate a wide variety of behavior, ranging from simple behavior such as sensory detection and motor output to more complex behavior including mating, sleep, drug-dependency, and learning (de Bono and Maricq, 2005; Feng et al., 2006; Pierce-Shimomura et al., 2008; Hart and Chao, 2010). Furthermore, the connectome of C. elegans nervous system has been mapped in exquisite detail by electron microscopy reconstruction, although this only reveals structural but not functional connections. These features together make C. elegans an excellent model to investigate the neural and genetic mechanisms by which individual neurons function within a circuit to drive motor output.
In order to navigate the environment, C. elegans locomotion is driven by undulations propagating from head to tail. Reorientation via backward locomotion, also called reversal, is a key behavioral strategy in animal navigation and avoidance of aversive stimuli (Hilliard et al., 2002; Gray et al., 2005; Piggott et al., 2011). Despite its simplicity, several elements of this motor program must be elaborately controlled to ensure its proper execution. This includes regulation of timing and strength of the motor output, as well as the likelihood that the behavior is initiated in a specific instance, termed response probability. Many neurons are involved in reversal regulation, ranging from the most upstream sensory neurons down to motor neurons (Gray et al., 2005). Laser ablation studies showed that the interneurons AVA and AVE command reversal execution through the A-type motor neurons (Chalfie et al., 1985). This is further corroborated by calcium imaging studies revealing that the activities of AVA/AVE neurons are tightly coupled with reversals (Kawano et al., 2011; Piggott et al., 2011; Kato et al., 2015). The command interneurons AVA/AVE form a large number of connections with the first layer and second layer interneurons, which are thought to relay sensory information (White et al., 1986). While AVA/AVE command interneurons are essential drivers of reorientation during locomotion, less is understood regarding exactly how these neurons are regulated within the locomotion circuitry to control motor output.
Among the second layer interneurons that connect with AVA/AVE, many reports implicate the pair of RIM interneurons as having an important role in reversal regulation. RIM neurons form both electrical and chemical synapses with AVA/AVE neurons. Laser ablation of RIM neurons has been reported to increase the frequency of reversals, suggesting an inhibitory role of RIM neurons in reversal regulation (Gray et al., 2005; Piggott et al., 2011). Interestingly, RIM-ablated worms also exhibit a reduction in reversal responses to anterior tactile stimulation or osmolarity insult, indicating a promotion role of RIM neurons in reversal regulation (Zheng et al., 1999; Piggott et al., 2011). Furthermore, the calcium activity in RIM is coupled with reversals (Kawano et al., 2011; Kato et al., 2015). While these findings highlight the important role of RIM in regulating reversal behavior, they also reveal a critical knowledge gap in our understanding of how RIM functions in the locomotion circuitry to drive reversal behavior.
In the present study, we investigated how RIM functions and coordinates with AVA/AVE command interneurons to form a functional circuit that properly controls reversal behavior. By combining optogenetics, laser ablation, calcium imaging and molecular genetics, we interrogated the complex roles of RIM in regulating distinct features of reversal behavior. We found that while RIM acutely promotes reversal initiation with AVA/AVE, it chronically suppresses reversal probability via AVA/AVE and A-type motor neurons. At the molecular level, RIM’s promotion of reversal initiation requires gap junctions with AVA/AVE, while its role in suppressing reversal probability relies on chemical neurotransmission with AVA/AVE and A-type motor neurons. At the circuit level, RIM can both promote and suppress AVA/AVE neuronal activities. Additionally, we uncovered that this bi-directional regulation of neural circuits is involved not only in the simple reversal behavior, but also in more complex behavior such as motor adaptation. Our work identifies circuit and molecular mechanisms by which individual neurons within a neural circuit both promote and suppress motor behavior to fine tune motor output.
Methods
Strains
WT: N2.
TQ440: akIs3[Pnmr-1::gfp].
TQ800: lite-1(xu7).
TQ1164: glc-3(ok321).
TQ2225: glc-4(ok212).
TQ2384: glc-2(gk179).
TQ2580: avr-14(ad1302).
TQ2581: avr-15(ad1051).
TQ3032: lite-1(xu7); xuEx1040[Pnmr-1::GCaMP3.0 + Pnmr-1::DsRed2b].
TQ6292: lite-1(xu7); xuEx2167[Pcex-1::GCaMP6f + Pgcy-13::sl2:mcherry].
TQ6346: glc-1(pk54).
TQ6744: lite-1(xu7); xuEx2257[Punc-4::tetx::sl2::yfp].
TQ6745: lite-1(xu7); xuEx2257[Punc-4::tetx::sl2::yfp]; xuEx1932[pgcy-13::TeTx-sl2-YFP;pgcy-13::dsRed2b; pnlp-12::dsRed2b].
TQ6875: xuEx1932[pgcy-13::TeTx-sl2-YFP; pgcy-13::dsRed2b; pnlp-12::dsRed2b]; xuIs219[Podr-2b(3a)::yfp + Punc-122d::gfp].
TQ7103: xuEx2595[Pgcy-13::tdc-1 sense+antisense+Pgcy-13::DsRed].
TQ7119: xuEx1899[Punc-4::DsRed]; xuEx856[pBS-77::5’UTR + avr-14::sl2::yfp].
TQ7283: xuEx2693[Pgcy-13::Chrimson::sl2::yfp].
TQ7313: xuEx2717[Pgcy-13::avr-14(genomic+cDNA)::sl2::YFP]; avr-14(ad1302).
TQ7262: xuEx2675[Pnmr-1::avr-14(genomic+cDNA)::sl2::yfp; avr-14(ad1302)].
TQ7264: xuEx2677[Pnpr-4::avr-14(genomic+cDNA)::sl2::yfp; avr-14(ad1302)].
TQ7267: xuEx2680[Punc-4::avr-14(genomic+cDNA)::sl2::yfp; avr-14(ad1302)].
TQ7269: xuEx2682[Pacr-5::avr-14(genomic+cDNA)::sl2::yfp; avr-14(ad1302)].
TQ7274: xuEx2687[Plgc-55::avr-14(genomic+cDNA)::sl2::yfp; avr-14(ad1302)].
TQ7280: xuEx2593[Pgcy-13::eat-4 sense+antisense+Pgcy-13::DsRed].
TQ7281: avr-14(ad1302)I; xuEx2593[Pgcy-13::eat-4 sense+antisense+Pgcy-13::DsRed].
TQ7324; xuEx2693[Pgcy-13::Chrimson::sl2::YFP]; inx-1(tm3524).
TQ7326: xuEx2693[Pgcy13::chrimson::mcherry; unc-7(e5)].
TQ7325: xuEx2693[Pgcy13::chrimson::mcherry]; unc-9(e101).
TQ7327: xuEx2730[P gcy-13::tetx; Punc-122::GFP]; xuEx2693[Pgcy-13::chrimsom::mcherry].
TQ7365: xuEx2765[Pnmr-1:: avr-14(genomic+cDNA)::sl2::yfp; Punc-4:: avr-14(genomic+cDNA)::sl2::yfp]; avr-14(ad1302).
TQ7332: xuEx2793[Pgcy-13::tetx::YFP; Punc-122::GFP]; xuEx1040[Pnmr-1::GCaMP3.0 + Pnmr-1::DsRed2b].
TQ7399: xuEx2795[Pnmr-1::avr-14::GFP; Punc-4::avr-14::gFP]; xuEx2766[Pgcy-13::eat-4 RNAi; Punc-122::GFP]; avr-14(ad1302).
TQ7340: xuEx2751[Pnpr-4::chrimson::mcherry]; xuEx2793[Pgcy-13::tetx; Punc-122::GFP].
TQ7441: unc-9(e101); inx-1(tm3524); xuEx2693[Pgcy-13::Chrimson::sl2::yfp].
TQ7348: xuEx2751[Pnpr-4::chrimson::mcherry].
TQ7433: xuEx1040[Pnmr-1::GCaMP3.0 + Pnmr-1::DsRed2b]; xuEx2766[Pgcy-13::eat-4 RNAi; Punc-122::GFP]; lite-1(xu7).
TQ7568: unc-7(e5); inx-1(tm3524); xuEx2693[Pgcy-13::chrimson::sl2::mcherry].
TQ7553: unc-7(e5); inx-1(tm3524); xuEx2751[Pnpr-4::chrimson::sl2::mcherry].
TQ7582: xuEx2860[Pnmr-1::unc-7(cDNA)::sl2::mcherry] + xuEx2823[Pgcy-13::chrimson::sl2::mcherry]; inx-1(tm3524); unc-7(e5).
TQ7710: xuEx2887[Pnmr-1::sl2::mcherry2 + Pinx-1 L::sl2::YFP].
TQ7711: xuEx2888[Pnmr-1::sl2::mcherry2]+ xuEx856[pBS-77::5’UTR + avr-14::sl2::yfp].
TQ8002: xuEx2316[pgcy-13::tdc-1(s + as) + pgcy-13::dsRed2b + pnlp-12::dsRed2b].
TQ8003: xuEx2323[pgcy-13::eat-4RNAi + pgcy-13::sl2::CFP + pnlp-12::dsRed2b].
TQ8004: xuEx2320[pgcy-13::unc-31(s + as) + pgcy-13::sl2-CFP + pnlp-12::dsRed2b].
Promoters used in this research include: Pnpr-4: AVA, AIN; Popt-3: AVE; Pgcy-13: RIM; Pnmr-1: AVA, AVE, AVD, RIM, PVC; Punc-4: a-type motor neurons (include DA and VA neurons, abbreviated as DA/VA); Plgc-55: AVB, SMD, RMD; Pacr-5: DB/VB motor neurons.
Laser ablation: laser ablation was performed on Olympus BX51 upright microscope equipped with a Micropoint system (Bargmann and Avery, 1995). L1 or L2 worms were immobilized on 2% agar pads using 5 mM sodium azide. The transgene Pnmr-1::gfp was included in worms to help identify AVA, AVD, AVE, and RIM. One to two laser pulses were applied to damage the nuclear region of the neuron of interest. Worms were then transferred to freshly seeded NGM plates immediately. Control groups of animals underwent surgical preparation without laser irradiation. Behavioral test and calcium imaging were conducted on day 1 adults.
Optogenetics and behavior: optogenetic interrogation of reversal initiation was performed as previously described (Piggott et al., 2011). Briefly, worms were grown on NGM plates supplied with 5 μM all-trans-retinal. Day 1 adult worms were tested on retinal-free NGM plates spread with a thin layer of OP50 bacteria. Amber light (5 s pulse; 590 nm; ~0.2 mW/mm2) was delivered from a home-made LED light source to activate Chrimson to trigger behavior. Animal behavior were recorded and analyzed using the Wormlab system (MBF Bioscience). Each trial included five animals and at least five trials were performed for each group. Reversals were scored as positive responses if the animal stopped forward movement and initiated a reversal lasting at least half of one head swing upon light stimulation.
Spontaneous reversal frequency was analyzed using an automated single worm tracking system as described previously (Feng et al., 2006; Li et al., 2006; Piggott et al., 2011). Day 1 adult worms were transferred to no food NGM plates for tracking and reversal frequency was recorded for 10 min (Figures 1G–I, 3; Supplementary Figures S4A–C) or 16 min (Figure 5). More than 10 worms were recorded for each group. Reversals were scored as described above.
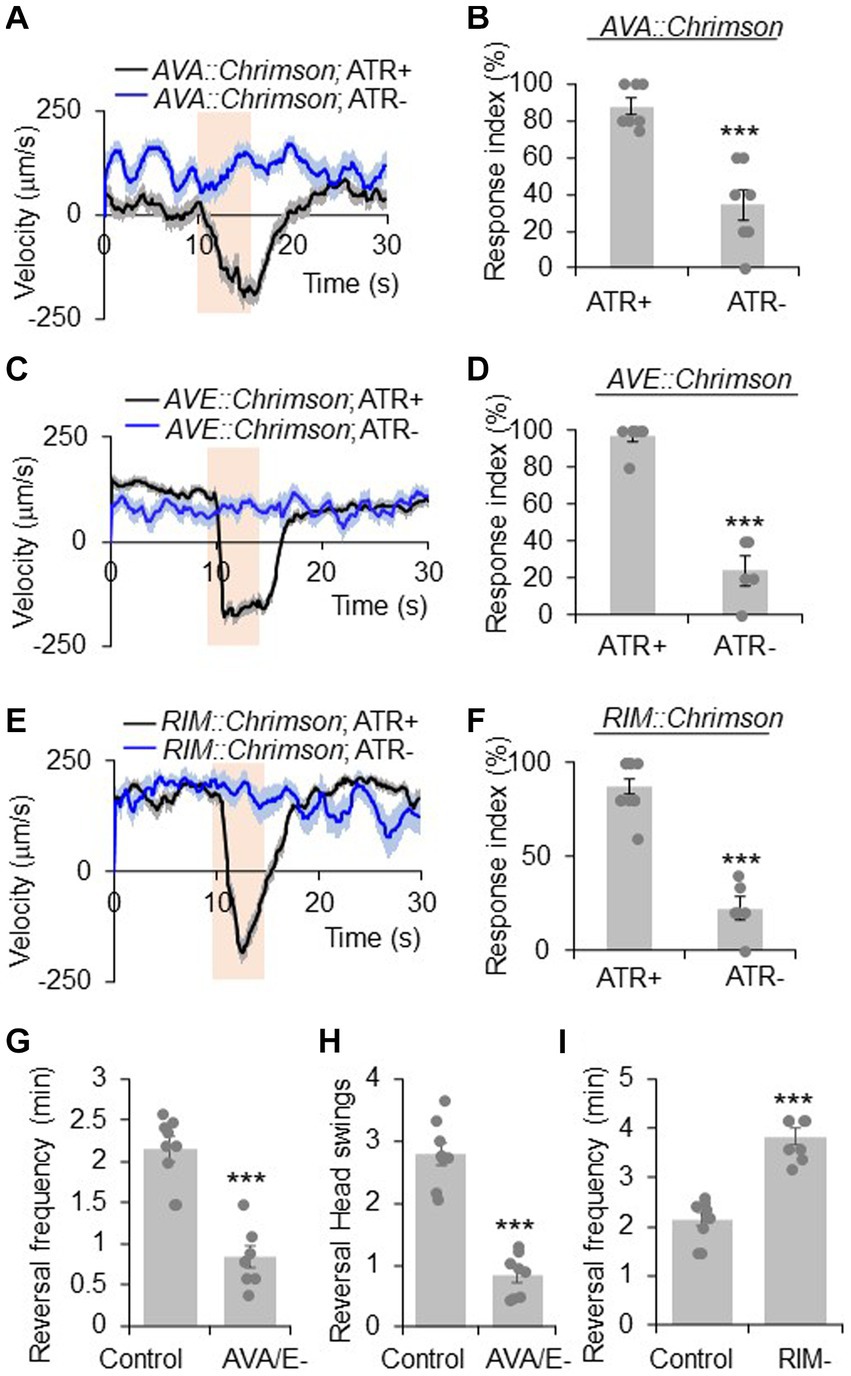
Figure 1. RIM has a complex role in reversal regulation. (A,B) Acute activation of AVA neurons using Chrimson triggers reversals. AVA was stimulated optogenetically by a Chrimson transgene under the npr-4 promoter. (A) Average velocity trace with SEM. n > =35. (B) Bar graph of reversal index quantification. Response index is the percentage of worms showing reversal upon optogenetic stimuli. Error bars: SEM. n ≥ 7. ***p = 0.000106 (unpaired two-sided t-test). ATR: all-trans retinal, which is required for the function of Chrimson. The bar in amber denotes the time window of light illumination. (C,D) Acute activation of AVE neurons using Chrimson trigger reversals. AVE was stimulated optogenetically by a Chrimson transgene under the opt-3 promoter. (C) Average velocity trace with SEM. n > =25. (D) Bar graph of reversal index quantification. Error bars: SEM. n ≥ 5. ***p = 1.18e-6 (unpaired two-sided t-test). (E,F) Acute activation of RIM neurons using Chrimson triggers reversals. RIM was stimulated optogenetically by a Chrimson transgene under the gcy-13 promoter. (E) Average velocity trace with SEM. n > =30. (F) Bar graph of reversal index quantification. Error bars: SEM. n ≥ 6. ***p = 1.063e-7 (unpaired two-sided t-test). (G,H) Ablation of AVA and AVE neurons reduces reversal frequency and reversal head swings. (G) Quantification of reversal frequency. Error bars: SEM. n ≥ 8. ***p = 3.529e-6 (unpaired two-sided t-test). Quantification of reversal headswings. (H) Error bars: SEM. n ≥ 8. ***p = 4.919e-7 (unpaired two-sided t-test). (I) Ablation of RIM neurons increases reversal frequency. Bar graph shows average reversal frequency. Error bars: SEM. n ≥ 8. ***p = 2.39e-5 (unpaired two-sided t-test).
Calcium imaging: to eliminate intrinsic response to blue light that excites GCaMP (Ward et al., 2008; Liu et al., 2010), strains used for calcium imaging carried a mutation in lite-1 gene that encodes a light-sensing receptor (Gong et al., 2016). Calcium imaging was performed on freely-behaving worms (Piggott et al., 2011) (Figure 4; Supplementary Figures S1, S6A,B). This system consists of an upright fluorescence stereomicroscope (Stemi SV11 M2 BIO), a dual-view beamsplitter, a X-Y motorized stage (Prior H101A) and an Andor EMCCD camera. The genetically-encoded calcium sensor GCaMPs (GCaMP 3.0 or GCaMP6f) were introduced into different neurons using neuron-specific promoters to observe calcium responses, and the red florescent protein DsRed was used as a reference channel for ratiometric imaging. A home-made software was used to coordinate the motorized stage and an Andor iXon EMCCD camera to track animal behavioral as well as capture GCaMP/DsRed signals. Day 1 adult worms were transferred to no food NGM plates for imaging. All experiments were conducted under the standard laboratory conditions (20°C, 30% humidity). Data processing was conducted using home-made software. GCaMP and DsRed ratio was calculated to indicate calcium responses. For calcium imaging with immobized worms (Supplementary Figures S6C,D), worms were immobilized using 3 mM levamisole. After 30 min, worms were transferred onto 2% agar pads. Imaging was conducted on Nikon Discovery Spinning Disc Confocal. Spontaneous calcium activity within AVA neuron was recorded for 10 min. Calcium signals were extracted using ImageJ.
Results
The pair of RIM interneurons both promote and suppress reversal behavior
C. elegans locomotion consists of forward crawling interrupted with reversals. Reversal allows worms to change locomotion direction, a key behavioral strategy in navigation and avoidance of aversive stimuli (Gray et al., 2005). Previous reports indicate a complex role of RIM neurons in the regulation of reversal behavior. To investigate the role of RIM neurons in this behavior, we adopted two behavioral assays. Specifically, we employed optogenetics to evoke reversals acutely to assay reversal initiation. We also recorded the frequency of spontaneous reversal events during worm locomotion in a relatively large time window to assay reversal probability. The onset of reversals in the latter assay is unpredictable. However, the overall reversal probability remains stable with a rate of approximately 2 events per minute.
AVA and AVE neurons act as command interneurons for reversal behavior (Chalfie et al., 1985; Gray et al., 2005; Piggott et al., 2011). Indeed, acute activation of AVA neurons optogenetically with Chrimson, a red light-drivable channelrhodopsin (Klapoetke et al., 2014), triggered reversals immediately (Figures 1A,B), confirming the positive role of AVA neurons in reversal initiation. A similar phenomenon was observed with AVE neurons (Figures 1C,D). When AVA and AVE neurons were ablated, the reversal frequency was greatly reduced (Figure 1G), verifying a critical role of AVA/AVE in promoting reversal probability. Notably, in AVA/AVE-ablated worms, the length (head swings) of the residual reversal events was rather short (Figure 1H). These results support the notion that AVA and AVE neurons play a critical role in driving as well as maintaining reversal behavior.
RIM neurons form both gap junctions and chemical synapses with AVA and AVE neurons (White et al., 1986). To test if RIM neurons share roles similar to AVA/AVE neurons in reversal regulation, we conducted both optogenetic and laser ablation experiments. Similar to AVA/AVE neurons, optogenetic activation of RIM neurons using Chrimson rapidly triggered reversals (Figures 1E,F), confirming a role for RIM neurons in acutely promoting reversals initiation (Zheng et al., 1999; Guo et al., 2009). On the other hand, as reported previously (Gray et al., 2005; Piggott et al., 2011), ablation of RIM resulted in an increase in reversal frequency, indicating a role of RIM neurons in suppressing reversal probability (Figure 1I). Thus, RIM neurons appear to both promote and suppress reversal behavior.
To further interrogate the function of AVA/AVE and RIM neurons in reversal regulation, we recorded the calcium activities of these neurons in freely-behaving worms with the genetic calcium indicator GCaMP6 (Chen et al., 2013), using an automated calcium imaging system. We observed that reversal events were tightly coupled with the rising phase of calcium spikes in AVA/AVE/RIM neurons, supporting the idea that these neurons contribute to reversal initiation (Supplementary Figures S1A,B). Altogether, the above results support the notion that while the command interneurons AVA/AVE are important in promoting reversal, the interneuron RIM plays a more complex role by acutely promoting reversal initiation but chronically suppressing reversal probability.
RIM neurons promote reversal initiation via gap junctions
Having shown that RIM neurons possess roles in both the promotion and suppression of reversals, we next asked how this dual-function is achieved at the circuit and molecular levels. The C. elegans wiring diagram reveals that RIM forms both chemical synapses and electrical gap junctions with AVA and AVE. As AVA/AVE are known to mediate reversal behavior (Chalfie et al., 1985; White et al., 1986), we sought to determine whether RIM promotes reversal initiation through these neurons. A short pulse of red light rapidly triggered a reversal in RIM::Chrimson worms (Figures 2A,B). However, when AVA and AVE neurons were removed by laser ablation, red light was no longer able to trigger reversals in RIM::Chrimson worms, although a decrease in forward speed was still observed (Figures 2A,B). These results suggest that the command interneurons AVA/AVE are required for RIM to acutely promote reversal initiation.
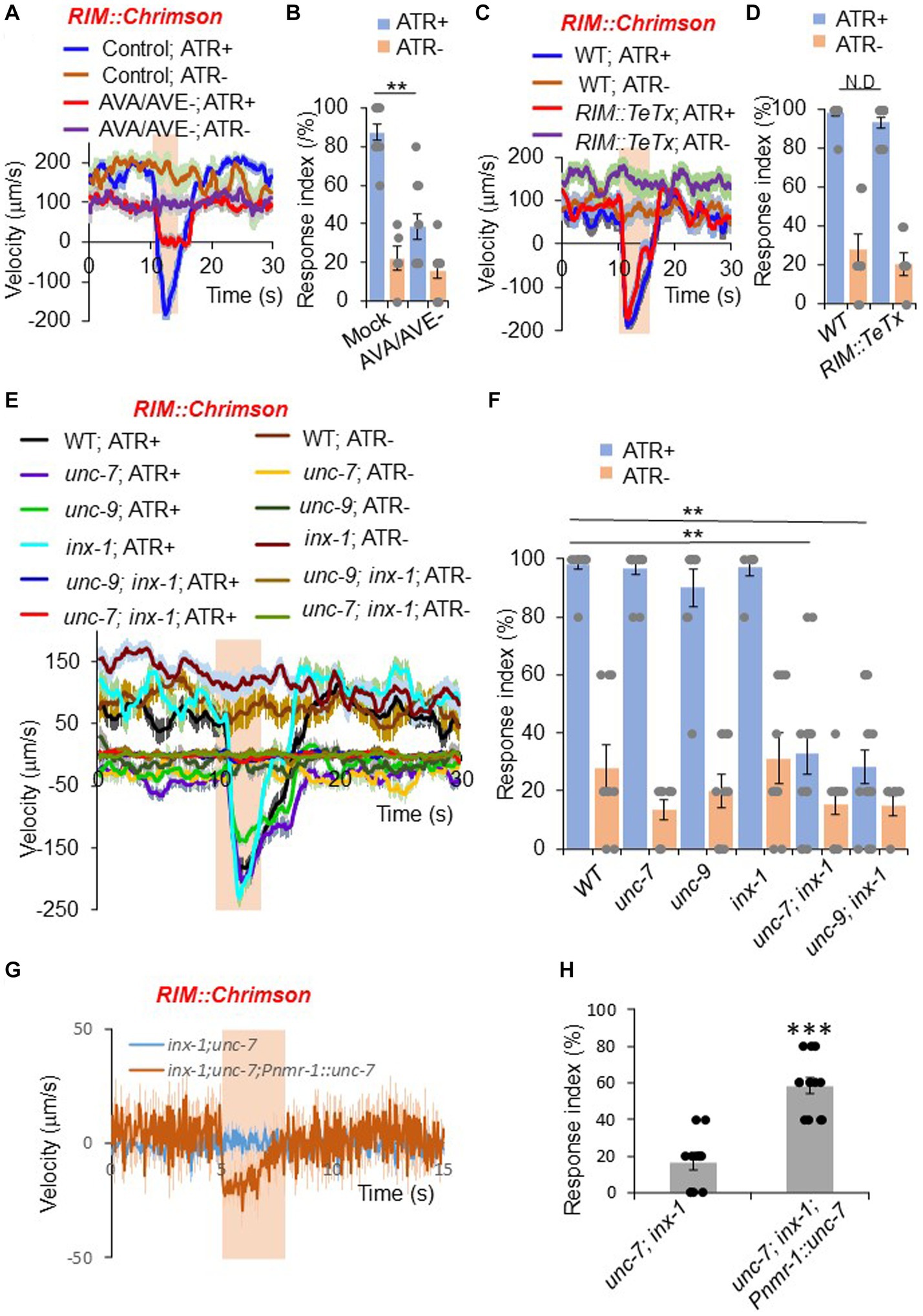
Figure 2. RIM promotes reversals through command interneurons and gap junctions. (A,B) Ablation of AVA/AVE neurons decreases RIM::Chrimson-triggered reversals. RIM was stimulated optogenetically with a Chrimson transgene under the gcy-13 promoter. (A) Traces shows average velocity with SEM. n > =30. ATR: all-trans retinal. The bar in amber denotes the time window of light illumination. (B) Reversal index quantification of (A). Error bars: SEM. n ≥ 6. **p = 1.095e-05 (ANOVA with Tukey’s HSD test). (C,D) Blockade of chemical transmission using Tetanus toxin (TeTx) in RIM does not change RIM::Chrimson-triggered reversals. TeTx was expressed in RIM as a transgene using the gcy-13 promoter. (C) Average velocity traces with SEM. n > =40. (D) Reversal index quantification of (C). Error bars: SEM. n ≥ 8. p = 0.7992 (ANOVA with Tukey’s HSD test). (E,F) Gap junction mutants impair RIM::Chrimson-triggered reversals. (E) Average velocity traces with SEM. n > =40. (F) Reversal index quantification of (E). Error bars: SEM. n ≥ 8. [unc-7; inx-1, p = 1.048e-05; unc-9;inx-1, p = 1.048e-05 (ANOVA with Tukey’s HSD test)]. (G,H) Transgenic expression of unc-7 cDNA within the reversal circuit partially rescues unc-7;inx-1 double mutant defect in RIM::Chrimson triggered reversals. (G) Average velocity traces with SEM. n > =40. (H) Reversal index quantification of (G). Error bars: SEM. n ≥ 12. (ANOVA with Tukey’s HSD test).
As RIM forms both chemical and electrical synapses with AVA and AVE neurons (Chalfie et al., 1985; White et al., 1986), we asked which type of synapses mediate the transmission between RIM and AVA/AVE. To test whether chemical synapses are required, we employed a genetically-encoded toxin to block chemical synapses. Tetanus toxin (TeTx) specifically cleaves synaptobrevin to impair chemical neurotransmission (Pellizzari et al., 1999). We expressed TeTx as a transgene in RIM using a RIM-specific promoter, and tested whether RIM::Chrimson-triggered reversals were affected. Impairment of RIM chemical transmission with TeTx did not block RIM::Chrimson-triggered reversals (Figures 2C,D), suggesting that chemical synapses are not required for RIM neurons to promote reversal initiation through AVA/AVE neurons.
We next examined whether electrical synapses play a role in RIM-triggered reversal initiation. Previous work reported that two innexin genes unc-7 and unc-9 are expressed in RIM and AVA/AVE neurons (Altun et al., 2009; Bhattacharya et al., 2019). We found that another innexin gene, inx-1, was also highly expressed in these neurons (Supplementary Figure S2). To test whether these innexins mediate the transmission between RIM and AVA/AVE neurons, we activated RIM neurons in unc-7, unc-9 and inx-1 single or double mutant animals using Chrimson. None of the single gap junction mutants showed a defect in RIM::Chrimson-triggered reversals (Figures 2E,F). However, unc-7; inx-1 and unc-9; inx-1 double mutants exhibited largely reduced responses (Figures 2E,F), suggesting that these innexins function in combination to mediate electrical transmission between RIM and AVA/AVE neurons. To test if innexins indeed function in the RIM-AVA/AVE circuit, we focused on the unc-7; inx-1 double mutant for further characterizations. Notably, the double mutant displayed a more severe uncoordinated phenotype than single mutants. To ensure that the reduced responses in the double mutant were not simply caused by uncoordinated movement, we optogenetically activated the downstream command neurons AVA in double mutant worms. We observed that upon activation of AVA neurons, unc-7; inx-1 worms were still able to execute reversals, albeit at a reduced speed and response rate (Supplementary Figures S3A,B). Thus, these double mutant worms retained the ability to execute reversals, though they failed to do so upon activation of RIM. We also found that transgenic expression of wild-type unc-7 gene in AVA/AVE and RIM neurons exhibited a rescuing effect on the reversal defect in unc-7; inx-1 double mutant worms, suggesting that unc-7 functions within the AVA/AVE-RIM circuit to promote reversals (Figures 2G,H). These results demonstrate that gap junction genes contribute to RIM-triggered reversals.
RIM neurons suppress reversal probability via chemical neurotransmission
We next asked how RIM suppresses reversal probability. Previous findings indicate that loss of the first layer interneurons AIB and AIZ, the command interneurons AVA and AVE, and the A-type motor neurons decreases the reversal frequency (Chalfie et al., 1985; Gray et al., 2005; Li et al., 2014). To test whether any of these neurons function downstream of RIM to mediate its suppression effect on reversal probability, we removed these neurons by laser ablation and tested if it eliminated the hyper-reversal phenotype caused by the loss of RIM neurons. Removal of AIB and AIZ neurons did not abolish the hyper-reversal phenotype in RIM-ablated animals, suggesting that AIB and AIZ neurons do not function downstream of RIM to suppress reversal probability (Supplementary Figure S4A). In contrast, when the AVA/AVE or A-type motor neurons (including DA and VA neurons, abbreviated as DA/VA in the figures) were removed by laser ablation or functionally impaired by TeTx, the hyper-reversal phenotype in RIM-ablated worms was largely suppressed, suggesting that RIM suppresses reversal probability via the AVA/AVE-A-type motor neuron circuit (Supplementary Figures S4B,C).
Having characterized the circuit mechanism by which RIM suppresses reversals, we next sought to identify the underlying molecular mechanisms. We again asked whether chemical synaptic transmissions were required. To address this, we specifically expressed TeTx in RIM neurons as a transgene to block their chemical transmission, and recorded the spontaneous reversal frequency. We found that blocking chemical transmission in RIM neurons increased reversal frequency, suggesting that RIM suppresses reversal probability via chemical transmission (Figure 3A).
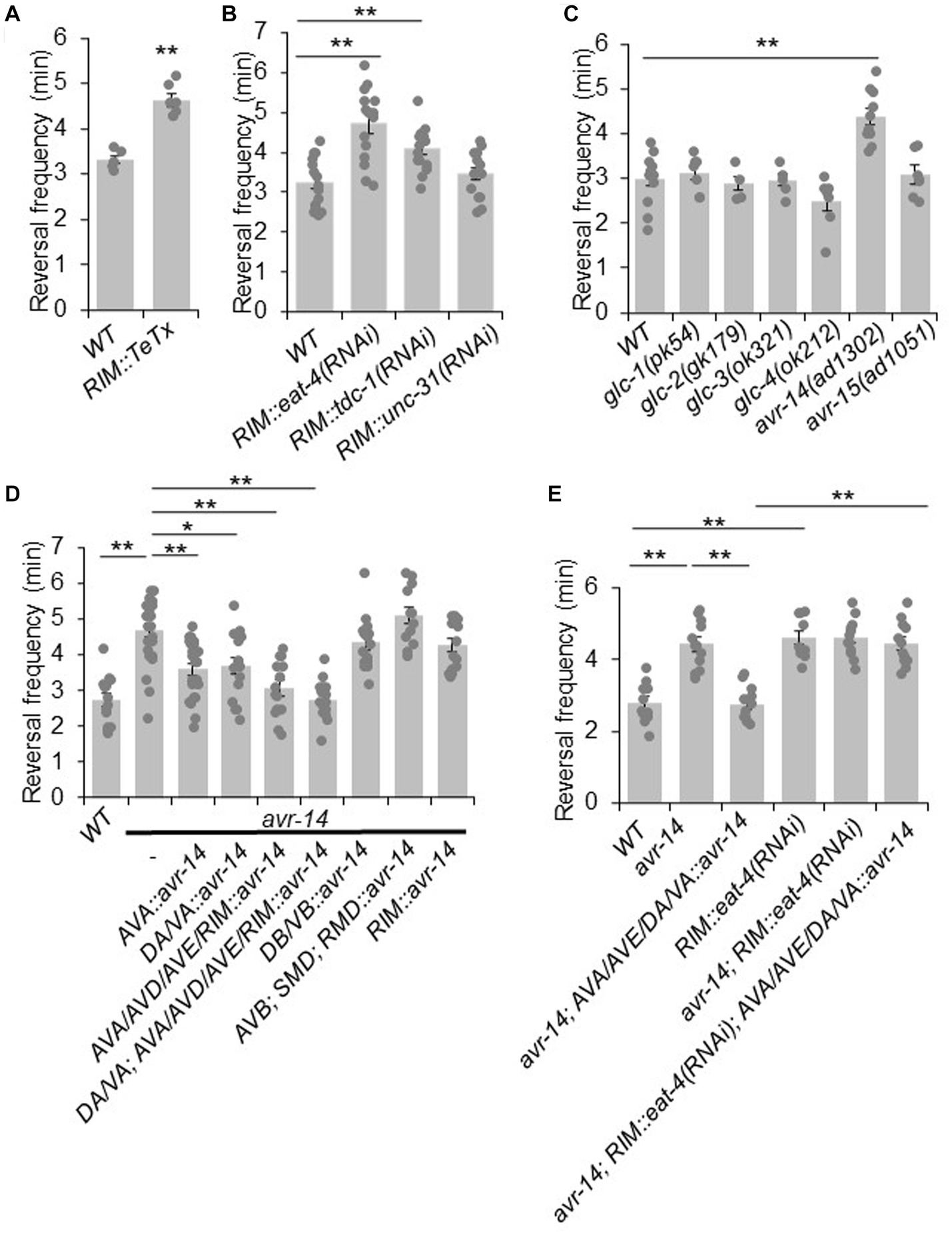
Figure 3. RIM suppresses reversal probability by chemical transmission. (A) Blockage of RIM chemical transmission using Tetanus toxin (TeTx) in RIM increases reversal frequency. Bar graph shows quantification of reversal frequency. Error bars: SEM. n ≥ 6. ***p = 2.73e-05 (unpaired two-sided t-test). (B) Knocking down glutamate and tyramine release by RNAi in RIM increases reversal frequency. RNAi was expressed as a transgene in RIM using the gcy-13 promoter. Bar graph shows quantification of reversal frequency. Error bars: SEM. n ≥ 14. RIM::eat-4(RNAi), **p = 1.171e-05. RIM::tdc-1(RNAi), **p = 0.006846 (ANOVA with Tukey’s HSD test). (C) Reversal frequency of different glutamate-gated chloride channel mutants. Mutation in avr-14 but not other glutamate-gated chloride channel genes increases the reversal frequency. Bar graph shows quantification of reversal frequency. Error bars: SEM. n ≥ 4. **p = 0.0003544 (ANOVA with Tukey’s HSD test). (D) Transgenic expression of wild-type avr-14 gene in AVA/AVE command interneurons and A-type motor neurons rescues the hyper-reversal defect of avr-14 mutant worms. Bar graph shows quantification of reversal frequency. Error bars: SEM. n ≥ 14. **p = 9.89e-06 between WT and avr-14; **p = 0.001245 between avr-14 and AVA::avr-14 rescue; *p = 0.01786 between avr-14 and DA/VA::avr-14 rescue; **p = 1.324e-05 between avr-14 and AVA/AVD/AVE/RIM::avr-14 rescue; **p = 9.887e-06 between avr-14 and DA/VA; AVA/AVD/AVE/RIM::avr-14 rescue. DA/VA is an abbreviation of A-type motor neurons which include DA and VA neurons. (ANOVA with Tukey’s HSD test). (E) Reversal frequency of avr-14 rescue and RIM::eat-4(RNAi) worms. Expression of avr-14 in AVA/AVE and A-type motor neurons rescued the hyper-reversal phenotype of avr-14 mutant worms. Blockage of glutamate release from RIM by RIM::eat-4(RNAi) impaired this avr-14 rescue. Bar graph shows quantification of reversal frequency. Error bars: SEM. n ≥ 10. **p = 1.022e-05 between WT and avr-14; **p = 1.018e-05 between WT and RIM::eat-4(RNAi). **p = 1.019e-05 between avr-14 and avr-14; AVA/AVE/DA/VA::avr-14. **p = 1.018e-05 between avr-14; RIM::eat-4(RNAi); AVA/AVE/DA/VA::avr-14 and avr-14; AVA/AVE/DA/VA::avr-14 (ANOVA with Tukey’s HSD test). Promoters that drive avr-14 transgene expression are Pnpr-4: AVA and AIN; Punc-4: DA, VA neurons; Pnmr-1: AVA, AVE, RIM, AVD and PVC; Pacr-5: DB, VB motor neurons; Plgc-55: AVB, SMD, RMD neurons; Pgcy-13: RIM neurons.
Chemical transmission in the nervous system is typically mediated by classic neurotransmitters and neuropeptides. RIM neurons release glutamate, tyramine and possibly neuropeptides (Kim and Li, 2004; Alkema et al., 2005; Serrano-Saiz et al., 2013). To test which of these is required for RIM’s suppression effect on reversal probability, we specifically knocked down the associated pathways in RIM with RNAi of the following genes: eat-4, which encodes a vesicle glutamate transporter (Lee et al., 1999); tdc-1 which encodes a tyrosine decarboxylase required for tyramine biogenesis (Alkema et al., 2005); and unc-31, which is required for neuropeptide release (Speese et al., 2007). No effect was observed in unc-31(RNAi) worms, suggesting that neuropeptide signaling may not play a major role in mediating the suppression effect of RIM on reversal probability (Figure 3B). By contrast, knocking down glutamate release from RIM markedly increased the reversal frequency, suggesting that glutamate release from RIM may suppress reversal probability (Figure 3B). A similar result was obtained with tdc-1 knock down, although the effect was not as robust as that observed with eat-4 knockdown (Figure 3B). Tyramine is known to suppress reversal frequency via tyramine-gated chloride channels (Pirri et al., 2009). However, how glutamatergic signaling suppresses reversal frequency is completely unknown. Given this and the fact that RNAi of eat-4 exhibited a more robust effect, we focused on characterizing the role of glutamate transmission in the suppression of reversal probability.
To identify the glutamate receptor that acts downstream of RIM neurons to mediate the suppression of reversal probability, we examined various glutamate receptor mutants. We focused on glutamate-gated chloride channels as they are known to mediate inhibitory responses (Dent et al., 2000). Six genes in the C. elegans genome encode glutamate-gated chloride channels, including glc-1, glc-2, glc-3, glc-4, avr-14, and avr-15. To ascertain whether they are involved in the regulation of reversal frequency, we recorded locomotion behavior of their mutants. The results showed that mutant worms lacking avr-14 exhibited a hyper-reversal phenotype, similar to that detected in RIM-ablated and RIM::eat-4(RNAi) worms (Figure 3C). In addition, we found that avr-14 was expressed in neurons including AVA/AVE and A-type motor neurons (Supplementary Figure S4D). Furthermore, transgenic expression of wild-type avr-14 gene in AVA/AVE and A-type motor neurons rescued the hyper-reversal phenotype in avr-14 mutant worms (Figure 3D). These results suggest that inhibition of AVA/AVE and A-type motor neurons by AVR-14 suppresses reversal frequency. To test whether glutamate acting on AVR-14 is derived from RIM, we blocked glutamate release specifically from RIM with an RIM::eat-4(RNAi) transgene in the avr-14 rescue background, and found that this abolished the rescue effect of the avr-14 transgene (Figure 3E), indicating that glutamate release from RIM suppressed reversal probability via the glutamate-gated chloride channel AVR-14 in the AVA/AVE-A-type motor neuron circuit.
RIM both promotes and suppresses AVA/AVE activities
Our results show that RIM neurons can both promote reversal initiation as well as suppresses reversal probability through interactions with the interneurons AVA/AVE. We next wondered how RIM regulates the activities of AVA/AVE neurons. To address this, we examined how RIM ablation affects the calcium activities in AVA/AVE neurons in freely-moving worms. In mock-ablated controls, the calcium spikes in AVA/AVE neurons were coupled with reversals, with reversals initiating upon calcium increase in AVA/AVE, and terminating once calcium traces peaked and began to drop (Figure 4A; Supplementary Figures S5B,D). In RIM-ablated animals, we observed that the coupling between reversal events and calcium spikes, as well as the kinetics of calcium spikes, were still preserved (Supplementary Figures S5A–D). However, the amplitude of AVA/AVE calcium spikes was significantly reduced in RIM-ablated worms (Figures 4A–C). This has also been observed in innexin mutant worms (Supplementary Figures S6A,B). These results suggest that RIM promotes AVA/AVE neuronal activities by increasing the amplitude of individual calcium spikes without changing their kinetics. This is further supported by the amplitude distribution pattern of individual calcium spikes (Supplementary Figure S5A). Specifically, in RIM-ablated animals, the amplitude distribution pattern is left-shifted to a narrower window, indicating that the calcium spikes in AVA/AVE neurons became weaker in the absence of RIM neurons (Supplementary Figure S5A). This calcium imaging result is consistent with the behavioral data in which we found the length of reversals (head swings) became shorter in RIM-ablated animals (Figure 4E). On the other hand, the frequency of calcium spikes in AVA/AVE neurons was increased in RIM-ablated worms (Figures 4A,D), indicating that AVA/AVE neurons became more excitable in the absence of RIM. A similar phenomenon was observed in worms in which RIM neurons were deficient in glutamate release (Supplementary Figures S6C,D). Thus, RIM appears to both promote and suppress AVA/AVE activities. These findings also suggest that RIM promotes reversal initiation by potentiating the amplitude of calcium spikes in AVA/AVE neurons, but suppresses reversal probability by inhibiting the frequency of calcium spikes in these neurons, thereby providing a circuit mechanism underlying the dual-role of RIM in regulating reversal behavior.
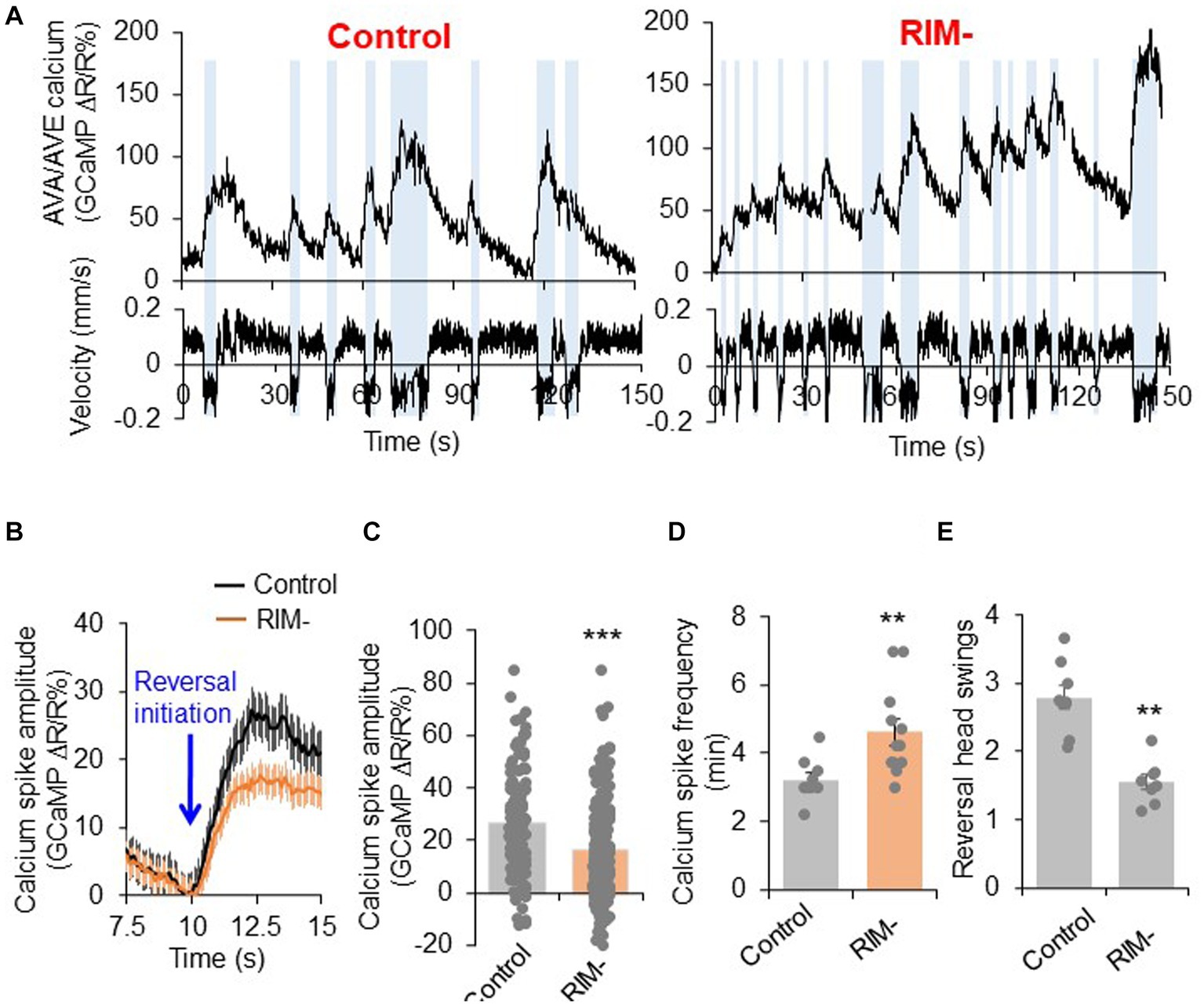
Figure 4. RIM fine tunes AVA/AVE activities. (A) Calcium spikes in AVA/AVE neurons in freely-moving worms are tightly coupled with reversals. Calcium imaging was conducted with freely moving animals using the CARIBN system. Left panels: mock-ablated worms. Right panels: RIM-ablated worms. Upper panels: calcium traces. Lower panels: velocity traces. Amber bars label reversal events. The nmr-1 promoter was used to drive GCaMP3/DsRed expression as a transgene in AVA and AVE neurons. As AVA and AVE neurons are in close proximity, the detected calcium fluorescence signals reflect the overall calcium activity in both neurons, though the calcium signals should be mainly contributed by AVA neurons due to the much stronger expression of GCaMP in AVA than AVE. (B,C) RIM ablation decreases the amplitude of calcium spikes in AVA/AVE neurons. (B) Average traces with SEM. Blue arrow marks the time point of reversal initiation. (C) Bar graph shows quantification of the amplitude of calcium spikes. Error bars: SEM. n ≥ 150. ***p = 5.24e-8 (unpaired two-sided t-test). (D) RIM ablation increases the frequency of calcium spikes in AVA/AVE neurons. Bar graph shows quantification the frequency of calcium spikes. Error bars: SEM. n ≥ 12. **p = 0.005311 (unpaired two-sided t-test). (E) Reversal length (head swings) is reduced in RIM ablated animals. Bar graph shows quantification of reversal head swings. Error bars: SEM. n ≥ 8. ***p = 2.39e-5 (unpaired two-sided t-test).
The dual-role of RIM neurons in motor adaptation
Given our observations that RIM can bi-directionally promote and suppress reversal behavior, we next wondered whether this dual function of RIM contributes to reversal-related complex behavior under more natural conditions. One possible application of this function could be to facilitate motor adaptation after food removal. In the presence of food (time 0 in Figures 5A–C), worms execute mostly short reversals (less than one head swing) (Figure 5B). Upon transfer to a no-food environment (time 1–15 in Figures 5A–C), the reversal length markedly increased to >3 head swings (Figure 5B). Furthermore, the total reversal strength (reversal head swings multiplied by reversal frequency) increased dramatically in the first minute following transfer to a no-food environment (Figure 5C). Constantly maintaining such a high response is not beneficial to animals, as it would be energetically costly to sustain the behavior. Indeed, animals underwent fast motor adaptation following transfer to the no-food environment (Figures 5A–C). Although the number of reversal head swings did not change over time in the no-food environment (Figure 5B), the reversal frequency quickly decreased over time (Figure 5A), resulting in a rapid drop in the total reversal strength over the 15 min time window (Figure 5C), indicating fast motor adaptation. This adaptive behavior represents the transition from local search behavior to dispersal behavior (Gray et al., 2005). This transition features two prominent phases: upon transfer to the no-food environment, worms first exhibited a rapid increase in reversal strength, followed by a progressive decrease in reversal strength over time (Figure 5C).
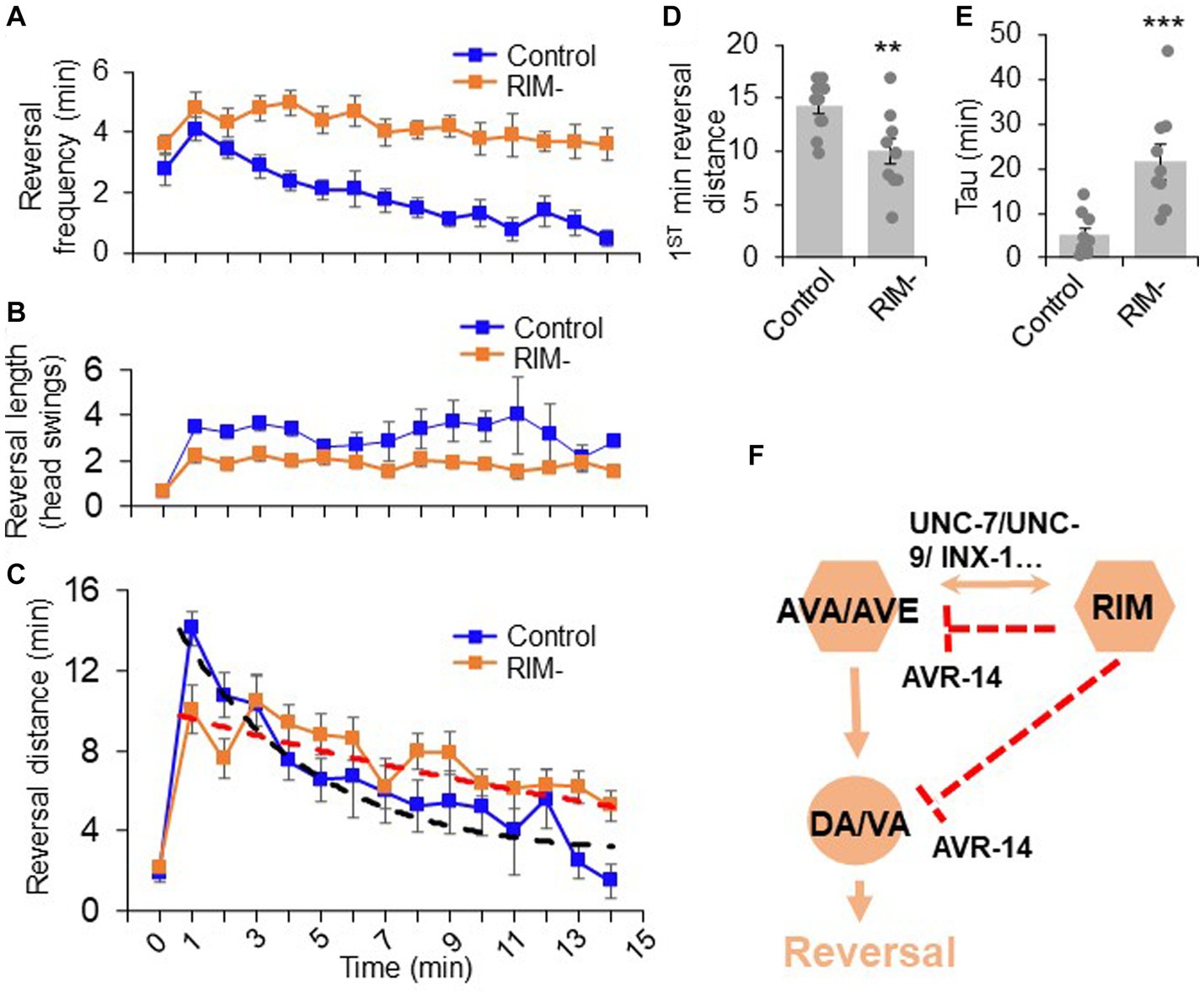
Figure 5. The dual role of RIM neurons in motor adaptation. Quantification of reversal properties in a motor adaptation assay. n = 10. Control groups of animals underwent surgical preparation without laser irradiation. (A) RIM ablation blocks the reversal frequency decline after worms were transferred to no-food environment. Error bars: SEM. n = 10. (B) RIM ablation decreases the reversal length after worms were transferred to no-food environment. SEM. n = 10. (C) Ablation of RIM neurons impairs motor adaptation after worms were transferred to no-food environment. Worms were placed in an environment with food (time = 0) and then transferred to a new environment without food (time = 1–15 min). The reversal strength is the sum of the total reversal distance (reversal head swings) in each minute. Dash lines were the fitting curves for the reversal strength of control and RIM-ablated groups (fitted with exp. function f(x) = y(0) + A*exp.(-invTau*x). Control: y(0) = 3.43, invTau = 0.249, A = 13.461; RIM: y(0) = −0.07, invTau = 0.042, A = 10.467). SEM. n = 10. (D) Ablation of RIM decreases the initial phase of the reversal strength (reversal strength of the 1st minute). Bar graph summarizes the 1st minute data in (C). **p = 0.0066 (unpaired two-sided t-test). (E) Ablation of RIM led to a slower decline in the reversal strength indicated by Tau value. ***p = 0.0006 (unpaired two-sided t-test). Tau values were derived from the fitting lines in (C). (F) Schematic model. RIM neurons acutely promotes reversals by promoting AVA/AVE activity via gap junctions. RIM also chronically inhibits AVA/AVE-A type motor neurons via an inhibitory glutamate pathway, thereby suppressing reversal probability over time.
We then asked whether RIM neurons contribute to such motor adaptation. In RIM-ablated worms, the number of reversal head swings was decreased compared to controls following transfer to the no-food environment (Figure 5B), resulting in a significant decrease in total reversal strength in the initial phase (e.g., the first minute) of motor adaptation (Figures 5C,D). Despite this, as RIM-ablated worms displayed a much slower decline in the frequency of reversal events in the no-food environment (Figure 5A), the total reversal strength exceeded that observed in mock-ablated control worms in later phases of motor adaptation (e.g., >4 min) (Figures 5C,E). The initial decrease in the reversal strength in RIM-ablated worms is consistent with RIM’s role in promoting reversal initiation, while the elevated reversal strength at later times is in line with RIM’s role in suppressing reversal probability. This biphasic defect in RIM-ablated worms supports the notion that RIM neurons both promote and suppress reversal behavior. Thus, RIM neurons contribute to motor adaptation in a new environment through their modulation of different features of reversal behavior. Taken together, our results provide a model in which RIM neurons function with AVA/AVE/A-type motor neurons to both promote and suppress the reversal circuit to fine tune motor output (Figure 5F).
Discussion
Previous studies reported seemingly conflicting results with respect to the role of RIM neurons in the locomotion circuitry, suggesting a complex role of RIM neurons in regulating locomotion. In the current study, we find that RIM neurons can both promote and suppress reversals during locomotion within a single motor circuit and do so by regulating distinct features of the reversal behavior. RIM neurons acutely promote the initiation of individual reversal events while chronically suppressing reversal probability. This multi-feature regulation is conducted by both electrical and chemical transmissions with the reversal command interneurons AVA/AVE and contributes to motor adaptation.
The electrical and chemical synapses between RIM and AVA/AVE likely play complex roles in regulating their excitability. Here we show that, during reversal initiation, RIM promotes the excitation of AVA/AVE neurons via electrical synapses, which is mediated by innexins including UNC-7, UNC-9, and INX-1. Several first layer interneurons, such as AIB, AIZ, and AIY, form synapses with RIM, which may direct sensory information to RIM. In addition, RIM can still be activated when AVA is silenced, indicating an information flow from upstream first layer interneurons to RIM, and probably then to the command interneurons AVA and AVE via gap junctions. Following RIM activation, glutamate released from RIM may then inhibit AVA/AVE and A-type motor neurons in the reversal circuit synaptically and/or extrasynaptically by turning on the inhibitory glutamate-gated chloride channel AVR-14, leading to the suppression of reversal frequency with time (Figure 5F). Indeed, in RIM-ablated worms, AVA/AVE neurons display a decrease in the amplitude of calcium spikes while exhibiting an increase in the frequency of calcium spikes, indicating that RIM neurons can both promote and suppress AVA/AVE activities. These findings also suggest that RIM promotes reversal initiation by potentiating the amplitude of calcium spikes in AVA/AVE neurons, but suppresses reversal probability by inhibiting the frequency of calcium spikes in these neurons, thereby providing a circuit mechanism underlying the dual-role of RIM in regulating reversal behavior. Notably, in addition to promoting reversal initiation, RIM activation has also been reported to lengthen reversals and does so by stabilizing the reversal circuit activity via gap junctions between AVA/AVE and RIM neurons, as reversals become shorter when RIM is ablated or silenced (Sordillo and Bargmann, 2021). Furthermore, while the active state of RIM appears to promote AVA/AVE activity and thus lengthens reversals, the inactive state of RIM suppresses AVA/AVE activity to lengthen forward runs (Sordillo and Bargmann, 2021). This highlights an intricate role of RIM in regulating multiple features of locomotion behavior.
Our data show that individual neurons in a neural circuit can regulate distinct features of a behavior by using either electrical or chemical transmission to communicate with other neurons in the circuit. Notably, these two modes of transmission are temporally distinct, as electrical transmission via gap junctions is rapid while chemical transmission occurs at a slower pace (Dong et al., 2018). This differential temporal pattern of information processing may explain why RIM initially promotes reversals and subsequently supress them. Our findings suggest that the identified circuit is able to process temporal information to both promote and suppress motor output. Processing differential temporal patterns to fine tune circuit functions offers an excellent coding strategy for behavioral control. In the locust, the dorsal uncrossed bundle (DUB) neurons and the lobula giant movement detector (LGMD) also form similar connections that process time-varying information (Wang et al., 2018), suggesting that similar mechanisms may operate in other species.
Complex brain functions are traditionally believed to depend on a vast number of neurons (Herculano-Houzel, 2012). However, increasing evidence suggests that it may also rely on multiple functions of single neurons. This phenomenon has been observed in both invertebrate and vertebrate brains (Briggman and Kristan, 2008; Rigotti et al., 2013; Li et al., 2014). The observation that RIM regulates multiple features of reversal behavior as well as contributes to motor adaptation in C. elegans indicates that this neuron is multi-functional. In addition to RIM neurons, many other neurons are also multi-functional in C. elegans. For example, AIY interneurons are multi-functional and can both regulate reversals and adjust locomotion speed (Li et al., 2014). The AIB interneuron pair can regulate both locomotion and feeding behavior (Zou et al., 2018). A single pair of PVD sensory neurons are able to detect stretch (proprioception) and touch (harsh touch sensation), as well as sense airborne sound to mediate auditory sensation (Tao et al., 2019; Iliff et al., 2021). SMD neurons are also multifunctional and play roles at multiple hierarchical levels such as fast head casting and omega turn behavior (Kaplan et al., 2020). This growing body of evidence indicates that complex brain functions rely on not only the vast number of neurons, but also multiple functions of individual neurons. Importantly, in the C. elegans connectome, many neurons form similar connection patterns like the circuit described here, indicating that the temporal coding strategy adopted by RIM to relay distinct information within a circuit could be widely employed in neural network integration and behavioral control.
Data availability statement
The original contributions presented in the study are included in the article/Supplementary material, further inquiries can be directed to the corresponding authors.
Author contributions
ZL, JZ, KW, TY, ER, and BP designed and performed the experiments and analyzed the data. ZL, JZ, KW, TY, ER, BP, JL, and XZSX contributed to the interpretation of the results. ZL, ER, and XZSX wrote the manuscript with assistance from all other authors. All authors contributed to the article and approved the submitted version.
Funding
This work was supported by an R35 grant from the NIGMS (to XZSX).
Acknowledgments
We thank Yingjie Wu for technical assistance. Some strains were obtained from the CGC and Knockout Consortiums in the United States and Japan. ER received pre-doctoral training grant support from the NIA and NIDCD.
Conflict of interest
The authors declare that the research was conducted in the absence of any commercial or financial relationships that could be construed as a potential conflict of interest.
Supplementary material
The Supplementary material for this article can be found online at: https://www.frontiersin.org/articles/10.3389/fnmol.2023.1228980/full#supplementary-material
References
Alkema, M. J., Hunter-Ensor, M., Ringstad, N., and Horvitz, H. R. (2005). Tyramine functions independently of octopamine in the Caenorhabditis elegans nervous system. Neuron 46, 247–260. doi: 10.1016/j.neuron.2005.02.024
Altun, Z. F., Chen, B., Wang, Z. W., and Hall, D. H. (2009). High resolution map of Caenorhabditis elegans gap junction proteins. Dev. Dyn. 238, 1936–1950. doi: 10.1002/dvdy.22025
Balaban, P. M. (2002). Cellular mechanisms of behavioral plasticity in terrestrial snail. Neurosci. Biobehav. Rev. 26, 597–630. doi: 10.1016/S0149-7634(02)00022-2
Bargmann, C. I., and Avery, L. (1995). Laser killing of cells in Caenorhabditis elegans. Methods Cell Biol. 48, 225–250. doi: 10.1016/S0091-679X(08)61390-4
Bhattacharya, A., Aghayeva, U., Berghoff, E. G., and Hobert, O. (2019). Plasticity of the electrical connectome of C. elegans. Cells 176, 1174–1189.e16. doi: 10.1016/j.cell.2018.12.024
Briggman, K. L., and Kristan, W. B. (2008). Multifunctional pattern-generating circuits. Annu. Rev. Neurosci. 31, 271–294. doi: 10.1146/annurev.neuro.31.060407.125552
Chalfie, M., Sulston, J. E., White, J. G., Southgate, E., Thomson, J. N., and Brenner, S. (1985). The neural circuit for touch sensitivity in Caenorhabditis elegans. J. Neurosci. 5, 956–964. doi: 10.1523/JNEUROSCI.05-04-00956.1985
Chen, T. W., Wardill, T. J., Sun, Y., Pulver, S. R., Renninger, S. L., Baohan, A., et al. (2013). Ultrasensitive fluorescent proteins for imaging neuronal activity. Nature 499, 295–300. doi: 10.1038/nature12354
Cote, M. P., Murray, L. M., and Knikou, M. (2018). Spinal control of locomotion: individual neurons their circuits and functions. Front. Physiol. 9:784. doi: 10.3389/fphys.2018.00784
de Bono, M., and Maricq, A. V. (2005). Neuronal substrates of complex behaviors in C. elegans. Annu. Rev. Neurosci. 28, 451–501. doi: 10.1146/annurev.neuro.27.070203.144259
Dent, J. A., Smith, M. M., Vassilatis, D. K., and Avery, L. (2000). The genetics of ivermectin resistance in Caenorhabditis elegans. Proc. Natl. Acad. Sci. U. S. A. 97, 2674–2679. doi: 10.1073/pnas.97.6.2674
Dong, A., Liu, S., and Li, Y. (2018). Gap junctions in the nervous system: probing functional connections using new imaging approaches. Front. Cell. Neurosci. 12:320. doi: 10.3389/fncel.2018.00320
Feng, Z., Li, W., Ward, A., Piggott, B. J., Larkspur, E. R., Sternberg, P. W., et al. (2006). A C. elegans model of nicotine-dependent behavior: regulation by TRP-family channels. Cells 127, 621–633. doi: 10.1016/j.cell.2006.09.035
Gong, J., Yuan, Y., Ward, A., Kang, L., Zhang, B., Wu, Z., et al. (2016). The C. elegans taste receptor homolog LITE-1 is a photoreceptor. Cells 167, 1252–1263.e10. doi: 10.1016/j.cell.2016.10.053
Gray, J. M., Hill, J. J., and Bargmann, C. I. (2005). A circuit for navigation in Caenorhabditis elegans. Proc. Natl. Acad. Sci. U. S. A. 102, 3184–3191. doi: 10.1073/pnas.0409009101
Guo, Z. V., Hart, A. C., and Ramanathan, S. (2009). Optical interrogation of neural circuits in Caenorhabditis elegans. Nat. Methods 6, 891–896. doi: 10.1038/nmeth.1397
Hart, A. C., and Chao, M. Y. (2010). From odors to behaviors in Caenorhabditis elegans. The neurobiology of olfaction, A. Menini, (Ed.) (Boca Raton (FL)).
Herculano-Houzel, S. (2012). The remarkable, yet not extraordinary, human brain as a scaled-up primate brain and its associated cost. Proc. Natl. Acad. Sci. U. S. A. 109, 10661–10668. doi: 10.1073/pnas.1201895109
Hilliard, M. A., Bargmann, C. I., and Bazzicalupo, P. (2002). C. elegans responds to chemical repellents by integrating sensory inputs from the head and the tail. Curr. Biol. 12, 730–734. doi: 10.1016/S0960-9822(02)00813-8
Iliff, A. J., Wang, C., Ronan, E. A., Hake, A. E., Guo, Y., Li, X., et al. (2021). The nematode C. elegans senses airborne sound. Neuron 109, 3633–3646.e7. doi: 10.1016/j.neuron.2021.08.035
Kaplan, H. S., Salazar Thula, O., Khoss, N., and Zimmer, M. (2020). Nested neuronal dynamics orchestrate a behavioral hierarchy across timescales. Neuron 105, 562–576.e9. doi: 10.1016/j.neuron.2019.10.037
Kato, S., Kaplan, H. S., Schrodel, T., Skora, S., Lindsay, T. H., Yemini, E., et al. (2015). Global brain dynamics embed the motor command sequence of Caenorhabditis elegans. Cells 163, 656–669. doi: 10.1016/j.cell.2015.09.034
Kawano, T., Po, M. D., Gao, S., Leung, G., Ryu, W. S., and Zhen, M. (2011). An imbalancing act: gap junctions reduce the backward motor circuit activity to bias C. elegans for forward locomotion. Neuron 72, 572–586. doi: 10.1016/j.neuron.2011.09.005
Kim, K., and Li, C. (2004). Expression and regulation of an FMRFamide-related neuropeptide gene family in Caenorhabditis elegans. J. Comp. Neurol. 475, 540–550. doi: 10.1002/cne.20189
Klapoetke, N. C., Murata, Y., Kim, S. S., Pulver, S. R., Birdsey-Benson, A., Cho, Y. K., et al. (2014). Independent optical excitation of distinct neural populations. Nat. Methods 11, 338–346. doi: 10.1038/nmeth.2836
Lee, R. Y., Sawin, E. R., Chalfie, M., Horvitz, H. R., and Avery, L. (1999). EAT-4, a homolog of a mammalian sodium-dependent inorganic phosphate cotransporter, is necessary for glutamatergic neurotransmission in caenorhabditis elegans. J. Neurosci. 19, 159–167. doi: 10.1523/JNEUROSCI.19-01-00159.1999
Li, W., Feng, Z., Sternberg, P. W., and Xu, X. Z. (2006). A C. elegans stretch receptor neuron revealed by a mechanosensitive TRP channel homologue. Nature 440, 684–687. doi: 10.1038/nature04538
Li, Z. Y., Liu, J., Zheng, M. H., and Xu, X. Z. S. (2014). Encoding of both analog- and digital-like behavioral outputs by one C. elegans interneuron. Cells 159, 751–765. doi: 10.1016/j.cell.2014.09.056
Liu, J., Ward, A., Gao, J., Dong, Y., Nishio, N., Inada, H., et al. (2010). C. elegans phototransduction requires a G protein-dependent cGMP pathway and a taste receptor homolog. Nat. Neurosci. 13, 715–722. doi: 10.1038/nn.2540
Pellizzari, R., Rossetto, O., Schiavo, G., and Montecucco, C. (1999). Tetanus and botulinum neurotoxins: mechanism of action and therapeutic uses. Philos. Trans. R. Soc. London B 354, 259–268. doi: 10.1098/rstb.1999.0377
Pierce-Shimomura, J. T., Chen, B. L., Mun, J. J., Ho, R., Sarkis, R., and McIntire, S. L. (2008). Genetic analysis of crawling and swimming locomotory patterns in C. elegans. Proc. Natl. Acad. Sci. U. S. A. 105, 20982–20987. doi: 10.1073/pnas.0810359105
Piggott, B. J., Liu, J., Feng, Z., Wescott, S. A., and Xu, X. Z. (2011). The neural circuits and synaptic mechanisms underlying motor initiation in C. elegans. Cells 147, 922–933. doi: 10.1016/j.cell.2011.08.053
Pirri, J. K., McPherson, A. D., Donnelly, J. L., Francis, M. M., and Alkema, M. J. (2009). A tyramine-gated chloride channel coordinates distinct motor programs of a Caenorhabditis elegans escape response. Neuron 62, 526–538. doi: 10.1016/j.neuron.2009.04.013
Purves, D., Augustine, G. J., Fitzpatrck, D., Hall, W. C., LaMantia, A. S., McNamara, J. O., et al., (2008). Movement and its central control. Neuroscience (Sunderland: Sinauer Associates, Inc.), 397–541.
Rigotti, M., Barak, O., Warden, M. R., Wang, X. J., Daw, N. D., Miller, E. K., et al. (2013). The importance of mixed selectivity in complex cognitive tasks. Nature 497, 585–590. doi: 10.1038/nature12160
Serrano-Saiz, E., Poole, R. J., Felton, T., Zhang, F., De La Cruz, E. D., and Hobert, O. (2013). Modular control of glutamatergic neuronal identity in C. elegans by distinct homeodomain proteins. Cells 155, 659–673. doi: 10.1016/j.cell.2013.09.052
Sordillo, A., and Bargmann, C. I. (2021). Behavioral control by depolarized and hyperpolarized states of an integrating neuron. elife 10:67723. doi: 10.7554/eLife.67723
Speese, S., Petrie, M., Schuske, K., Ailion, M., Ann, K., Iwasaki, K., et al. (2007). UNC-31 (CAPS) is required for dense-core vesicle but not synaptic vesicle exocytosis in Caenorhabditis elegans. J. Neurosci. 27, 6150–6162. doi: 10.1523/JNEUROSCI.1466-07.2007
Tao, L., Porto, D., Li, Z., Fechner, S., Lee, S. A., Goodman, M. B., et al. (2019). Parallel processing of two mechanosensory modalities by a single neuron in C. elegans. Dev. Cell 51, 617–631.e3. doi: 10.1016/j.devcel.2019.10.008
Wang, H., Dewell, R. B., Zhu, Y., and Gabbiani, F. (2018). Feedforward inhibition conveys time-varying stimulus information in a collision detection circuit. Curr. Biol. 28:e1503, 1509–1521.e3. doi: 10.1016/j.cub.2018.04.007
Ward, A., Liu, J., Feng, Z., and Xu, X. Z. (2008). Light-sensitive neurons and channels mediate phototaxis in C. elegans. Nat. Neurosci. 11, 916–922. doi: 10.1038/nn.2155
White, J. G., Southgate, E., Thomson, J. N., and Brenner, S. (1986). The structure of the nervous system of the nematode Caenorhabditis elegans. Philos. Trans. R. Soc. London B 314, 1–340. doi: 10.1098/rstb.1986.0056
Zheng, Y., Brockie, P. J., Mellem, J. E., Madsen, D. M., and Maricq, A. V. (1999). Neuronal control of locomotion in C. elegans is modified by a dominant mutation in the GLR-1 ionotropic glutamate receptor. Neuron 24, 347–361. doi: 10.1016/S0896-6273(00)80849-1
Keywords: motor behavior, motor control, neural circuit, C. elegans, glutamate
Citation: Li Z, Zhou J, Wani KA, Yu T, Ronan EA, Piggott BJ, Liu J and Xu XZS (2023) A C. elegans neuron both promotes and suppresses motor behavior to fine tune motor output. Front. Mol. Neurosci. 16:1228980. doi: 10.3389/fnmol.2023.1228980
Edited by:
Angeles Badell Ribera, University of Colorado Denver, United StatesReviewed by:
Jie Liu, Monash University, AustraliaJinhyun Kim, Korea Institute of Science and Technology (KIST), Republic of Korea
Copyright © 2023 Li, Zhou, Wani, Yu, Ronan, Piggott, Liu and Xu. This is an open-access article distributed under the terms of the Creative Commons Attribution License (CC BY). The use, distribution or reproduction in other forums is permitted, provided the original author(s) and the copyright owner(s) are credited and that the original publication in this journal is cited, in accordance with accepted academic practice. No use, distribution or reproduction is permitted which does not comply with these terms.
*Correspondence: Zhaoyu Li, zhaoyu.li@uq.edu.au; X.Z. Shawn Xu, shawnxu@umich.edu
†These authors have contributed equally to this work