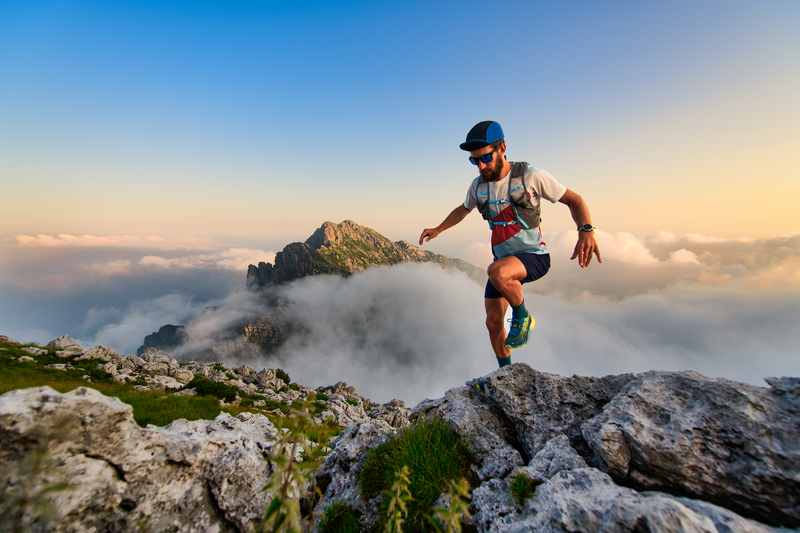
94% of researchers rate our articles as excellent or good
Learn more about the work of our research integrity team to safeguard the quality of each article we publish.
Find out more
REVIEW article
Front. Microbiol. , 29 January 2025
Sec. Microbial Symbioses
Volume 16 - 2025 | https://doi.org/10.3389/fmicb.2025.1504395
This article is part of the Research Topic Regulatory RNAs: Key Modulators in Host-Microbiome Interactions and Disease Pathogenesis View all articles
The gut microbiota plays a pivotal role in human metabolic health by influencing immune responses, digestion, and metabolic homeostasis. Recent research highlights the intricate interactions between gut microbiota and RNA, especially non-coding RNAs, in regulating metabolic processes. Dysbiosis of the gut microbiota has been linked to metabolic disorders such as type 2 diabetes, obesity, metabolic-associated fatty liver disease (MAFLD) and metabolic heart disease. Microbial metabolites, including short-chain fatty acids (SCFAs), modulate RNA expression, influencing lipid metabolism, glucose regulation, and inflammatory responses. Additionally, microRNAs (miRNAs) and long non-coding RNAs (lncRNAs) serve as critical regulators in these processes, with emerging evidence showing that gut-derived metabolites affect post-transcriptional gene regulation. This review synthesizes the current understanding of the gut microbiota-RNA axis and its role in metabolic diseases. By exploring the molecular mechanisms, particularly how gut microbiota-derived signals modulate RNA pathways, the review underscores the potential of targeting this axis for therapeutic interventions. Furthermore, it examines how dysbiosis leads to epigenetic changes such as m6A RNA methylation, contributing to disease pathogenesis. These insights offer a new perspective on the prevention and treatment of metabolic diseases, with potential applications in personalized medicine.
Current epidemiological, pathological, and histological studies at both cellular and animal levels reveal that gut microbiota significantly influence metabolic health and disease risk in humans (Fardi et al., 2023). These microbial communities, which colonize various luminal surfaces of the body, encompass vast numbers of bacteria, fungi, phages, eukaryotic viruses, and other microorganisms, most of which are symbiotic or mutualistic (Carding et al., 2015; Jandhyala et al., 2015; Koutoukidis et al., 2024; Thursby and Juge, 2017). The collective gut microbial genes of an individual represent a gene pool far larger than that of the human genome. The gut microbiota plays a critical role in enhancing host immunity, digestion, endocrine and neural signaling, drug metabolism, endotoxin clearance, and regulating the production of metabolites essential to host health (Flint et al., 2012).
In the past two decades, evidence from observational studies has established a robust link between gut microbiota and host metabolic homeostasis, suggesting that dysbiosis of the gut microbiota is a contributing factor in the pathogenesis of several prevalent metabolic disorders, including type 2 diabetes, obesity, MAFLD and metabolic heart disease (Icaza-Chávez, 2013). For example, the fermentation of dietary fiber by gut microbiota, particularly from the phyla Firmicutes and Bacteroidetes, produces SCFAs such as butyrate, propionate, and acetate, which modulate host metabolism via G-protein-coupled receptors (GPCRs) expressed by intestinal endocrine cells (Scheppach, 1994). Acetate and butyrate stimulate the release of glucagon-like peptide 1 (GLP-1) and peptide YY (PYY), influencing pancreatic insulin biosynthesis (GLP-1 induced) and central appetite regulation (PYY induced satiety) (Drucker, 2022; Pittner et al., 2004; Scheppach, 1994).
RNA serves as a key mediator in the transmission of genetic information and is classified into several major types. Small RNAs such as miRNAs and small interfering RNAs (siRNAs) are essential for regulating gene expression at the post-transcriptional level (Li et al., 2008; Lee et al., 2004). lncRNAs exert significant influence on gene regulation by modulating chromatin architecture, transcription, and translation processes. In the study of metabolic diseases, the expression profile and functional regulation of RNA molecules have become an important research direction (Decatur and Fournier, 2002; Kong et al., 2018; Zhao et al., 2017). Recently, a growing number of researches have focused on the relationship between RNA and gut microbiota in metabolic disorders, for example, The main RNAs associated with metabolic heart disease are miR-21, miR-126, miR-33, lncRNA-H19, lncRNA-H19, lncRNA-ANRIL (Krichevsky and Gabriely, 2009; Olivo-Martínez et al., 2024; Surina et al., 2021).
The intestinal metabolism of phytoestrogens is also associated with metabolic diseases and their amelioration. The gut microbiota metabolizes phytoestrogens, such as isoflavones, ellagic acid derivatives, and lignans, into more bioactive metabolites, including equol, urolithins, and enterolignans. These metabolites, due to their higher lipophilicity and enhanced bioavailability, may exhibit stronger biological activity compared to their precursor compounds. These metabolites can interact with key enzymes involved in cellular processes, reducing DNA damage and lipid peroxidation (Stojanov and Kreft, 2020).
Although most existing reviews focus on the relationship between metabolic diseases and the gut microbiota—primarily highlighting strain abundance or metabolites affecting host receptors and contributing to metabolic disorders, or separately examining the role of RNA in metabolic diseases—limited attention has been given to the integrated effects of gut microbiota and its derivatives with RNA on metabolic diseases. Mechanistic studies in this area are critical for advancing future research. To address this gap, this review synthesizes the intricate interactions between metabolic diseases, gut microbiota, and RNA, with an emphasis on the molecular mechanisms through which gut microbiota modulates host metabolism via RNA pathways. It further examines the specific roles and manifestations of these RNA-mediated mechanisms in the context of metabolic disorders. Through a comprehensive analysis of current research, we aim to offer new insights and directions for future studies, while providing a scientific foundation for the prevention and treatment of metabolic diseases.
Recent studies have revealed that the influence of the gut microbiota extends even to the molecular level, where it can finely regulate the host’s RNA expression, thereby affecting gene function and cellular behavior (Chiba et al., 2024; Wortelboer et al., 2022).
In addition, it was found that gut microbiota produces a short-chain fatty acid butyrate that targets the DNA methylation level of the host miR-378a promoter, thus preventing the development of obesity and glucose intolerance (Krist et al., 2015). Second, in 2019, Virtue et al. found that indole-3-carboxylic acid produced by gut microbes controlled miR-181 family expression in mouse white adipocytes, further negatively regulating energy expenditure and insulin sensitivity (Virtue et al., 2019). This suggests that inhibitory regulation of miRNAs by gut microbe-derived metabolites is the central mechanism. Intestinal flora can affect miRNA expression in intestinal epithelial cells (IEC). Nakata et al. found that gut microbiota induced miR-21-5p expression in IEC, and mmu-miR-21-5p increased intestinal epithelial permeability by down-regulating the expression of ADP ribosylation factor 4 (arf4) in 2017 (Nakata et al., 2017). The above studies suggest that miRNAs may act as intermediate mediators to mediate the regulation of host IEC gene expression by gut microbiota.
When the organism is immunocompromised, pks+ E. coli containing pks gene islands, E. coli can colonize the gut and induce disease. It has been found that the genotoxin Colibactin produced by pks+ E. coli activates the c-Myc pathway by disrupting the DNA double strand, resulting in an increase in downstream miR-20a-5p expression (Cao et al., 2024; Sun et al., 2024).
The miRNAs are partially involved in mediating the expression of miR-20a-5p in E. coli. This suggests that miRNAs are partially involved in mediating the pathogenic effects of pks+ E. coli (Huang et al., 2022). The mechanism through which the gut microbiota regulates miRNA expression is still not fully understood. Is it through the direct regulation of miRNA expression by the host or its cells, or through the indirect regulation of miRNA expression by the metabolites in the intestinal tract? The specific mechanism remains to be studied in depth.
RNA molecules, particularly miRNAs, play a crucial role in maintaining the homeostasis of the gut microbiota. Recent studies have shown that RNA not only plays a key role in host cell proliferation, differentiation, and immune responses but also directly influences the health status of the host by regulating the composition and function of the gut microbiota (Monzel and Desai, 2024). Specifically, certain miRNAs can indirectly affect the composition of the gut microbiota through receptors or secreted substances in host cells. For example, the bacterium Segatella copri is a common member of the human gut microbiota that is associated with both health and disease states. Through extensive transcriptomic analysis of S. copri and human-derived samples, researchers identified and named the S. copri RNA colonization factor (SrcF). Deletion of the SrcF gene significantly affects the ability of S. copri to colonize the gut and disrupts the expression of genes involved in nutrient uptake and utilization, including PULs. SrcF may represent a regulatory hub that integrates nutrient signals essential for S. copri survival in the gut (El Mouali et al., 2024).
Mechanistic research in this area is still limited, with most studies focusing on the exosome perspective. Exosomes are secreted by almost all cell types via exocytosis and establish cross-talk between the host and the gut microbiota. The major components of exosomes include miRNAs. miRNAs that influence microbial growth in the gut are primarily derived from IECs and homeodomain-only protein homeobox (HOPX)-positive cells. In colitis-tolerant mice, stimulation with DSS induces IECs to secrete miR-142a-3p, which specifically promotes the growth of Lactobacillus reuteri by binding to specific targets in the bacterial genome (Zhang et al., 2023). On the other hand, miR-200b-3p, miR-200b-5p, and miR-26a-5p are negatively correlated with Dubosiella and Lactobacillus but positively correlated with Escherichia-Shigella. The improved microbiota, supplemented with miR-200b-3p, can feedback to the gut microenvironment via exosomes, thus inhibiting the development of intestinal inflammation (He et al., 2022).
Furthermore, RNA molecules also regulate the metabolic environment, and antimicrobial peptide secretion in the gut, directly influencing the growth and proliferation of specific microbial species (Liu et al., 2016). For instance, by culturing the anaerobic bacterium Fusobacterium nucleatum (Fn) and E. coli with synthesized miRNA mimics in vitro, it was found that hsa-miR-515-5p promotes the growth of Fn, while hsa-miR-1226-5p promotes the growth of E. coli. These findings indicate that miRNAs directly influence bacterial growth (Shen et al., 2022). Through these mechanisms, RNA molecules participate in the fine-tuned regulation of the gut microbiota, thereby affecting the host’s immune responses, metabolic functions, and even disease susceptibility.
With changes in modern lifestyles, the incidence of metabolic disease, such as diabetes has significantly increased (de Lima et al., 2024). This condition is complex, having long disease course, and is difficult to cure. The pathological processes involve all aspects of life activities, including epigenetics, proteomics, and metabolomics (Suhre and Zaghlool, 2021). Current studies suggest that the mutual regulatory effects between RNA and the gut microbiota play an irreplaceable role in controlling host metabolism, and their imbalance is likely to contribute to the onset of related metabolic diseases (Mundula et al., 2022; Nie et al., 2019). Further analysis may provide important indicators for the early prediction of metabolic diseases like diabetes and help define future personalized therapeutic targets.
Diabetes includes type 1 diabetes (T1DM), characterized by pancreatic β-cell dysfunction and absolute insulin deficiency, and type 2 diabetes (T2DM), where β-cells have not completely lost the ability to produce insulin, resulting in relative insulin deficiency or resistance (Argano et al., 2023; dos Santos Haber et al., 2023). RNA is considered a crucial determinant of pancreatic β-cell functional integrity, as it regulates β-cell development and dysfunction, controls insulin signaling in peripheral tissues like adipose tissue to maintain glucose homeostasis, and is involved in β-cell proliferation, apoptosis, insulin biosynthesis, and secretion (Benáková et al., 2021; Gao et al., 2022). To date, numerous diabetes-related RNAs have been identified, including miR-21, miR-424-5p, circ-HIPK, lncRNA-MALAT1, circRNA-HIPK3 and miR-30a (Alrefai et al., 2023; Fu et al., 2014; Grieco et al., 2017; Lakhter et al., 2018).
Specifically, the upregulation of miR-21 inhibits the expression of the anti-apoptotic gene Bcl-2, leading to a reduction in Bcl-2 protein synthesis, while simultaneously increasing the pro-apoptotic protein caspase-3, thereby inducing β-cell apoptosis. This results in decreased insulin secretion and elevated blood glucose levels (Nigi et al., 2018; Shi et al., 2010; Ye et al., 2018). miR-424-5p directly interacts with the 3’ UTR sequence of insulin receptor (INSR) mRNA in human hepatocellular carcinoma cell lines, thereby suppressing insulin gene expression and signaling in adipocytes. Additionally, RNA has been implicated in the pathogenesis of diabetic retinopathy. lncRNA-MALAT1 has been shown to promote inflammation and fibrosis in diabetic retinopathy by activating the NF-κB and TGF-β1 signaling pathways (Abedi et al., 2024; Jia and Zhou, 2020; Li et al., 2021; Ren and Wang, 2021). Similarly, circRNA-HIPK3 promotes endothelial cell proliferation and vascular dysfunction in diabetic retinopathy by blocking the function of miR-30a (Pan et al., 2018; Zaiou, 2020; Zhou and Kuang, 2021).
At the same time, an increasing number of studies suggest that the gut microbiota plays an essential role in the development of diabetes driven by RNA (Tanase et al., 2020). In both T1DM and T2DM patients, the abundance and diversity of the gut microbiota are altered to varying degrees. A meta-analysis by Qin et al. on 345 Chinese T2DM patients revealed a decrease in butyrate-producing gut bacteria, such as Clostridium and Butyricicoccus, while the abundance of opportunistic pathogens increased in 2024 (Wu et al., 2024). The gut microbiota assists the host in metabolizing and breaking down dietary intake, converting indigestible polysaccharides in food into SCFAs. The SCFA pathways of the gut microbiota not only provide energy for the host but also maintain the metabolic balance of unbound lipids and glucose in tissues through the activation of their receptor, G protein-coupled receptor 41 (GPR41/43) (Wu et al., 2021). This process interferes with insulin signaling in fat cells, reducing fat accumulation in adipose tissue.
SCFAs, including butyrate, acetate, and propionate, have diverse functions, with butyrate specifically recognized for its antioxidant properties and its ability to suppress autoimmune reactions associated with diabetes (Rasouli-Saravani et al., 2023). Research has shown that butyrate expression is negatively correlated with miR-106a, and reduced miR-106a expression increases the expression of the cell cycle inhibitor p21, partially inhibiting the proliferation of tumor cells. Additionally, miR-21, which was previously mentioned for directly acting on the target gene Bcl-2 to induce β-cell apoptosis, is also thought to be regulated by the gut microbiota during diabetes development (Gao and Zhao, 2020).
Researchers investigating metabolic diseases like obesity and diabetes have discovered that in high-fat diet-induced mouse models, the triglyceride levels in the liver are associated with both the gut microbiota and the expression of liver miRNAs (Wilson et al., 2017). This suggests that the interaction between the gut microbiota and liver miR-21 expression might mediate metabolic effects via the regulation of miR-21 target genes. Studies showed that germ-free mice had lower liver triglyceride levels compared to other models, and miR-18a, miR-666, and miR-21 were all regulated in wild-type mouse hepatocytes by the pro-inflammatory LPS released from Escherichia coli O55 in a LPS-dependent manner (Arunmanee et al., 2016).
Furthermore, liver triglyceride levels were positively correlated with the relative abundance of Firmicutes, and negatively correlated with the expression of liver miR-21 and the relative abundance of Proteobacteria and Bacteroidetes (Shin et al., 2015). The pathological mechanism underlying this process is likely similar to the regulation of miR-21-5p expression by the gut microbiota mentioned earlier. The gut microbiota can highly specifically regulate miR-21-5p through excessive secretion of LPS and peptidoglycan (PGN), activating Toll-like receptor 2/4 (TLR2/4)-related pathways, promoting the expression of pro-inflammatory cytokines and nitric oxide in antigen-presenting cells, and inducing NF-κB activation and interferon upregulation, which triggers an inflammatory immune response (Casado-Bedmar et al., 2024).
In a chronic inflammatory environment, macrophages release TNF-α, IL-1β and IL-18, which interfere with insulin signaling between the liver, fat, and other target tissues, ultimately leading to abnormal hepatocyte apoptosis, non-alcoholic fatty liver disease, insulin resistance, and metabolic endotoxemiat (Lee et al., 2023). Therefore, the composition and metabolic characteristics of the gut microbiota determine its critical role in diabetes onsetk (Cordeiro et al., 2024). Blocking the transmission of specific miRNA regulatory signals from gut microbial metabolites at certain targets may hold great significance in preventing or delaying diabetes-related complications and other metabolic disorders (Prattichizzo et al., 2021).
As the most common post-transcriptional epigenetic modification, m6A exhibits abnormal modification patterns in the β-cells of diabetic islets and in various diabetic complications, disrupting the expression of key target genes and thus influencing disease pathology (Eizirik et al., 2020). Therefore, both gut microbiota dysbiosis and abnormal m6A modification profiles at disease sites reflect the dynamic changes involved in the development of T2DM at the micro level (Benak et al., 2023). Furthermore, recent studies have proposed that gut microbiota, as an environmental factor, can directly or indirectly affect m6A methylation levels in the body, leading to the hypothesis of a gut microbiota-m6A metabolic axis. High-throughput sequencing has shown differences in m6A modification levels across various tissues and organs between germ-free mice and conventional mice, which are associated with changes in transcriptional levels (Jabs et al., 2020; Zhuo et al., 2022). For example, mouse models colonized with single strains like Akkermansia muciniphila and Lactobacillus plantarum exhibit specific alterations in m6A modifications (Guo et al., 2023). In 2022, Chen et al. further confirmed the causal role of gut microbiota in regulating m6A modifications through studies on both animal and human samples (Chen et al., 2023). They found that Fusobacterium nucleatum can activate the YAP signaling pathway, downregulating the transcription of METTL3, significantly reducing m6A modifications in intestinal epithelial tissues, and promoting the expression of serine/threonine-protein kinase 26B, thus enhancing the invasiveness of colorectal cancer (Wang et al., 2020). This indicates that changes in m6A modifications are a crucial mechanism by which the gut microbiota exerts physiological effects (D’Aquila et al., 2020).
Current research suggests two main pathways through which the gut microbiota influences host methylation modifications: direct and indirect pathways (Bhat and Kapila, 2017).
The gut microbiota can directly affect the chromatin and transcriptome states of intestinal cells through structural components such as flagellin, LPS, and peptidoglycan (Wu et al., 2022). Intestinal cells’ leukocyte antigens, major histocompatibility complex gene systems, and pattern recognition receptors can recognize and differentiate bacterial antigens, triggering corresponding responses and forming epigenetic memory (Kmieć et al., 2017). Studies have shown that LPS stimulation alters m6A methylation levels in the liver and activates the nucleotide-binding oligomerization domain (NOD) protein 1/NF-κB pathway, mediating liver inflammation and damage (Satheesan et al., 2024). Additionally, LPS stimulation leads to enhanced m6A modifications in the liver, associated with upregulation of genes like sterol regulatory element-binding protein 1C (SREBP-1C) and stearoyl-CoA desaturase 1 (SCD1), which are involved in liver lipid metabolism disorders (Jeyakumar and Vajreswari, 2022).
Gut microbiota metabolic products also play a broad role in regulating the host’s epigenome. As a “microbial endocrine organ,” the gut microbiota produces or transforms active substances such as SCFAs, secondary bile acids, various B vitamins, and S-adenosyl methionine (SAM), a major methyl donor (Monné et al., 2022). These metabolites indirectly induce epigenetic modifications. SAM is the primary methyl donor, while other gut-derived metabolites like methionine, choline, betaine, and folate contribute to the one-carbon cycle, which ultimately produces SAM. Vitamins B2, B6, and B12 from gut bacteria are also involved in the one-carbon cycle, aiding SAM synthesis. SAM is then absorbed into the bloodstream, supplying tissues and organs with the necessary methyl donors for m6A modifications. Other microbial metabolites, such as butyrate, can improve inflammation in granulosa cells in polycystic ovary syndrome by inhibiting METTL3-mediated m6A modifications of FOS-like antigen 2 (Shao et al., 2024). Meanwhile, secondary bile acids like deoxycholic acid can promote the dissociation of the METTL3-METTL14-WTAP complex, thus hindering the progression of gallbladder cancer. Hence, gut microbial metabolites are also important mediators in regulating host m6A modification levels (Figure 1).
Figure 1. Gut microbiota influence the functions of hepatocytes, pancreatic islet β-cells, and enteroendocrine cells through metabolites which are also associated with mRNA methylation processes, impacting gene expression and metabolic regulation. MHC, major histocompatibility complex; TLR, toll-like receptors; ROS, reactive oxygen species; m6A, n6-methyladenosine (created with BioRender.com).
Obesity is a chronic disease characterized by excessive fat accumulation, with an increasing prevalence in recent years. It is also the primary risk factor for MAFLD (Gutiérrez-Cuevas et al., 2021). The causes of obesity are multifactorial, potentially involving interactions between genetic, behavioral, and social factors, and the widespread use of antibiotics may further exacerbate the condition. RNA plays a significant role in obesity. For example, a study using circRNA sequencing and bioinformatics analysis examined the expression of circRNAs in the subcutaneous adipose tissues of Large White and Laiwu pigs, identifying 70 upregulated and 205 downregulated circRNAs (Li et al., 2018). It was hypothesized that circRNAs may regulate adipogenesis and lipid metabolism. Overexpression of circ-SAMD4A has been associated with poor prognosis in obese individuals, as it regulates preadipocyte differentiation by acting as a miR-138-5p sponge (Liu et al., 2020), thereby increasing the expression of EZH2. Another study on visceral and subcutaneous fat deep sequencing revealed that various circRNAs are dynamically regulated during adipogenesis and obesity. Among them, circTshz2-1 and circArhgap5-2 were identified as key regulators of in vitro adipogenesis. What’s more, miRNA-193b-365 is aberrantly expressed in adipocyte differentiation and obesity states, and may be involved in adipocyte differentiation as well as the development of obesity (Goody and Pfeifer, 2019).
Since the discovery in 2006 that transferable obesity-related microbiota can induce weight gain in lean mice, subsequent epidemiological studies have demonstrated differences in gut microbiota between obese and lean individuals. At the species level, twin studies have shown that the abundance of SCFA producers, such as Blautia and Roseburia intestinalis, is associated with obesity, while butyrate producers like Oscillospira spp. (Ejtahed et al., 2020) and the methanogen Methanobrevibacter smithii are inversely correlated with obesity. Another genome-wide association study comparing lean and obese individuals found that in obese individuals, the abundance of Phascolarctobacterium, a glutamate-fermenting symbiont, was significantly lower and negatively correlated with serum glutamate levels (Álvarez-Mercado et al., 2019). In summary, several distinct gut microbiota have been linked to improved metabolic outcomes in mice, although further validation in humans is required. This effect is likely strain-specific and may depend on the presence of other supporting microorganisms, which can vary between individuals.
The gut microbiota influences the transcription of long non-coding RNA (lncRNA) in intestinal epithelial cells, thereby affecting lipid metabolism. Transcriptome sequencing (RNA-seq) results indicate that gut microbiota suppresses the expression of lncRNA Snhg9 (Hashemi et al., 2024). Further research has found that gut microbiota indirectly inhibits the expression of lncRNA Snhg9 through myeloid cells and ILC3. lncRNA Snhg9 enhances the activity of SIRT1 by binding to its inhibitor CCAR2, which is a negative regulator of the lipid metabolism core regulator PPARγ (Zhang et al., 2020). Therefore, lncRNA Snhg9 inhibits lipid uptake through the CCAR2-SIRT1-PPARγ signaling axis, reducing body fat and preventing diet-induced obesity. These findings provide new strategies for treating metabolic diseases by targeting lncRNA Snhg9 and gut microbiota. However, further research is needed to determine which specific components or features of the gut microbiota can activate myeloid cell-ILC3 signaling and how the composition of gut microbiota affects the expression of Snhg9 and lipid metabolisms (Wang et al., 2023; Figure 2).
Figure 2. The role of gut microbiota in regulating fatty acid metabolism, PPARγ signaling, and IL22 production through interactions with immune cells. SIRT1, silent information regulator factor 2-related enzyme 1; CCAR2, cell cycle and apoptosis regulator 2; Snhg9, small nucleolar RNA host gene 9; PPARγ, peroxisome proliferator-activated receptor γ; DC, dendritic cell; IL23, interleukin-23; ILC3, group 3 innate lymphoid cells; IL22, interleukin-22 (created with BioRender.com).
Metabolites from the gut microbiota also play a significant role in promoting obesity. The regulation of white adipose tissue (WAT) by gut microbiota is a critical process in maintaining metabolic health, and dysbiosis of gut microbiota can lead to the development of obesity (Sun et al., 2018). miRNAs are endogenous, non-coding single-stranded RNA molecules approximately 22 nucleotides long, involved in the degradation of target miRNA or inhibition of its translation, thereby negatively regulating post-transcriptional gene expression. The miR-181 family plays an important role in processes such as cell proliferation, apoptosis, and differentiation. Researchers have found that a high-fat diet activates miR-181 in the white adipose tissue of mice, subsequently leading to obesity and insulin resistance (Virtue et al., 2019).
In obese individuals, miR-181 levels are increased in white adipose tissue, while levels of indole are reduced in plasma, suggesting a potential correlation between the gut microbiota-miR-181 axis and human diseases (Eslam et al., 2022). Notably, researchers have observed dysregulation of miR-181 expression in white adipose tissue and the abundance of tryptophan-derived metabolites in a group of obese human children (Virtue et al., 2019). This indicates that the miR-181 family may represent a potential therapeutic target for regulating white adipose tissue function in the context of obesity.
Furthermore, dysregulation of the gut microbiota-miR-181 axis is essential for the development of obesity, insulin resistance, and inflammation in white adipose tissue in mice. In contrast to the AhR agonist Ficz, which improves glucose tolerance without affecting obesity, the administration of indole to high-density lipoprotein-fed mice can prevent obesity and impaired glucose tolerance (Polyzos et al., 2019). This suggests that, at least in mice, indole exerts beneficial effects through the AhR and miR-181. These findings highlight the crucial role of gut microbiota-derived metabolites in regulating miR-181 levels in white adipose tissue, indicating that host metabolism is adjusted in response to dietary and environmental changes (Virtue et al., 2019).
MAFLD is characterized by hepatic steatosis, excess fat accumulation in the liver, and metabolic liver dysfunction (Eslam et al., 2022). Obesity, a chronic metabolic disease closely linked to T2DM, cardiovascular diseases, and osteoarthritis, is the primary risk factor for MAFLD (Polyzos et al., 2019). There is a significant correlation between MAFLD and T2DM—over 70% of T2DM patients have MAFLD, and more than 20% of MAFLD patients either have or are at high risk of developing T2DM (Caussy et al., 2021).
The “gut-liver axis” plays a crucial role in linking the liver and intestines structurally and functionally, involving pathways such as the bile duct, portal vein, and systemic circulation (Rattanaprasert et al., 2019). The liver, receiving about 75% of its blood supply from the portal vein, is the first organ exposed to gut microbiota and their metabolites via the portal circulation (Albillos et al., 2020). A healthy gut barrier prevents the translocation of intestinal microbes and their toxic byproducts, while damage to the barrier can lead to microbial translocation, excessive immune activation, and the onset or progression of liver inflammation (Nicoletti et al., 2019). Consequently, the development of MAFLD can disrupt the gut microbiota, and abnormalities in the gut microbiome and its metabolites can reciprocally affect MAFLD progression (Jiang et al., 2020).
The human gut microbiota predominantly comprises Bacteroidetes, Firmicutes, Proteobacteria, and Actinobacteria, with Bacteroidetes and Firmicutes being the most dominant phyla (Gomaa, 2020; Jiang et al., 2020). Gut microbiota plays a significant role in regulating metabolism, immunity, and disease development, and it is closely associated with MAFLD (Alisi et al., 2024). Various barriers in the gut, including physical, biochemical, and immune defenses, limit the translocation of microbes and their byproducts (Ma et al., 2022). However, unhealthy dietary habits (e.g., high-sugar, high-fat diets, and overeating) can cause dysbiosis, leading to barrier dysfunction and immune homeostasis disruption (Jamar et al., 2021). On one hand, immune activation triggered by gut microbes and their metabolites can exacerbate liver damage, inflammation, and fibrosis, accelerating MAFLD progression (Liu et al., 2022). On the other hand, certain gut microbial metabolites, such as SCFAs and bile acids, can mitigate liver inflammation, oxidative stress, and steatosis (Visekruna and Luu, 2021). Compared to healthy individuals, MAFLD patients exhibit reduced gut microbial diversity and significant compositional alterations, with increased abundance of Gram-negative bacteria (e.g., Bacteroides, Proteobacteria, and Enterobacteria) and decreased levels of Firmicutes, especially SCFA-producing bacteria like Lactobacillus and Ruminococcus (Aron-Wisnewsky et al., 2020). This suggests that the gut microbiota may be a key factor in the pathology of MAFLD.
In recent studies, circRNAs have emerged as potential regulators in MAFLD. For instance, bioinformatics analysis by Ou et al. identified 450 dysregulated circRNAs in a MAFLD mouse model, with 298 upregulated and 152 downregulated circRNAs (Zeng et al., 2024). Guo et al. found that circRNA-0046367 is significantly downregulated in steatotic livers, with its suppression linked to miR-34a/PPARα interaction and lipid peroxidation (Yu et al., 2021). Additionally, circRNA-0046366 was shown to be downregulated during free fatty acid-induced hepatic steatosis, and its upregulation inhibited the miR-34a/PPARα interaction, reducing triglyceride levels and suppressing steatosis (Guo et al., 2018). These findings suggest that circRNA-0046367 and circRNA_0046366 may play critical roles in the pathogenesis of MAFLD (Yepmo et al., 2022). However, the exact mechanisms and roles of m6A-modified non-coding RNAs in MAFLD remain unclear.
Research has demonstrated significant differences in m6A levels across various organs and tissues between germ-free and normal mice, indicating that gut microbiota strongly influences m6A mRNA modification (Jabs et al., 2020). m6A modifications may be crucial in forming the livers specific and complex ‘microenvironment (Pan et al., 2021). Initially, m6A modifications are regulated by methyltransferases (“writers”) and demethylases (“erasers”), with m6A+ binding proteins like YTH domain family 2 (YTHDF2), also known as “readers,” playing roles in RNA translation and autophagy regulation (He and He, 2021). Hence, m6A methylation in non-coding RNAs could serve as an important tool for diagnosing and treating various diseases, including MAFLD (Lin et al., 2020; Figure 3).
Figure 3. Role of epitranscriptomic m6A methylation in regulating miRNA and circRNA dynamics for potential diagnostic and therapeutic applications in MAFLD and liver diseases. m6A, n6-methyladenosine; YTHDF2, YTH N6-methyladenosine RNA-binding protein 2; MAFLD, metabolic-associated fatty liver disease (created with BioRender.com).
Recent studies have also linked nucleotide methylation to inflammation grading, lipid synthesis, and oxidative stress, underscoring its critical role in the progression of MAFLD. As a result, m6A modifications may be a key factor in the pathogenesis of MAFLD, providing new insights into disease mechanisms and potential therapeutic targets.
Metabolic heart disease encompasses a spectrum of cardiovascular disorders closely linked to metabolic dysregulation, primarily driven by disturbances in glucose and lipid metabolism (He and He, 2021). Common metabolic diseases such as diabetes mellitus and its complications, obesity, and fatty liver disease are recognized as significant contributors to this condition (Lin et al., 2020). Numerous studies have identified key risk factors for these metabolic disorders, including unhealthy dietary habits, sedentary lifestyles, and the aging process (Deprince et al., 2020). Notably, these risk factors are also prevalent among patients suffering from various cardiovascular diseases, including myocardial ischemia, atherosclerosis, and related complications (Yki-Järvinen, 2014). Recent projections indicate that by 2030, approximately 27 million individuals will be affected by hypertension, leading to a subsequent rise in coronary artery disease, stroke, and heart failure (Mozaffarian et al., 2008). This alarming trend underscores the high prevalence of metabolic heart disease within the population.
Given the intricate relationship between metabolic dysregulation and cardiovascular health, researchers have increasingly recognized “metabolic heart disease” as a critical term (Roth et al., 2020). This term encapsulates clusters of metabolic disturbances and their secondary effects on cardiovascular physiology (Bugger et al., 2022; Mohebi et al., 2022). In recent years, a wealth of research has emerged focused on these diseases, revealing the profound interplay between metabolic pathways and cardiovascular outcomes (Wang et al., 2022).
Key regulatory ncRNAs implicated in metabolic heart disease include miR-21, miR-126, miR-33, lncRNA H19, and lncRNA-ANRIL (Wishart, 2019). For instance, Surina et al. examined the pathological features of the left ventricle in a 5/6 nephrectomy rat model of chronic kidney disease (CKD), revealing an upregulation of miR-21-5p (Jusic et al., 2020). This upregulation was found to modulate the expression of various transcripts associated with fatty acid oxidation and glycolysis through the targeting of PPARα (Tudurachi et al., 2024). Further studies on H9C2 cells demonstrated that the overexpression of miR-21-5p diminished lipid content and lipid peroxidation, suggesting a regulatory role of miR-21-5p on the dependence of glycolytic and fatty acid oxidation pathways (Li et al., 2015).
Moreover, lncRNA-GAS5 and its target miR-21 have been implicated in regulating lipid levels, macrophage activity, T helper (Th) cell function, and vascular smooth muscle cell behavior, contributing to the progression of atherosclerosis and coronary heart disease (CHD) (Nasci et al., 2019). Investigations by Jiang and Du highlighted the correlation between the circulating levels of lnc-GAS5 and common biochemical markers, the severity of stenosis, and cytokine profiles in CHD patients, revealing a negative association with miR-21 levels. This suggests that lnc-GAS5 and miR-21 may serve as promising therapeutic targets for managing CHD.
In the pathophysiology of atherosclerosis, oxidized low-density lipoprotein (ox-LDL) plays a critical role in endothelial injury, oxidative stress, and inflammatory responses (Wang J. et al., 2019). miR-21 has been shown to mediate several pathophysiological mechanisms associated with atherosclerosis (Khatana et al., 2020). Pei et al. established an in vitro atherosclerosis model using ox-LDL to stimulate human umbilical vein endothelial cells (HUVECs) and vascular smooth muscle cells (VSMCs), investigating the potential functions of the CASC7/miR-21 axis in TLR4/NF-κB and PI3K/AKT signaling pathways (Canfrán-Duque et al., 2017). Their findings indicated that LncRNA-CASC7 regulates these pathways by inhibiting miR-21 expression, thereby influencing the pathological progression of ox-LDL-induced atherosclerosis (Pei et al., 2021).
Additionally, research has uncovered numerous circRNAs derived from genes associated with cardiovascular diseases, such as Ryr2, TTN, and DMD (Jalink et al., 2024). Notably, differential expression of circRNAs has been identified in both healthy individuals and those with cardiovascular conditions. A recent meta-analysis revealed 63 circRNAs with significantly abnormal expression in cardiovascular diseases, with 44 being upregulated and 19 downregulated (Bagheri Moghaddam et al., 2022). Among these, circCDKN2BAS and circMACF1 have been suggested as potential biomarkers for the diagnosis and treatment of cardiovascular diseases (Li et al., 2019). Furthermore, studies have indicated that the circRNA-MICRA, derived from zinc finger protein 609, exhibits lower levels in acute myocardial infarction patients, potentially serving as a biomarker for predicting left ventricular dysfunction (Li et al., 2019).
SCFAs, primarily produced through the fermentation of dietary fiber by gut microbiota, include acetate, propionate, and butyrate (Bayoumi et al., 2018). SCFAs play a vital role in modulating host metabolism and inflammatory responses and can act as signaling molecules influencing miRNA expression (Wang M. et al., 2019). For example, butyrate, as a histone deacetylase (HDAC) inhibitor, alters chromatin structure to regulate gene expression, including that of miRNAs (Yuan et al., 2019). Additionally, SCFAs can activate downstream signaling pathways via G protein-coupled receptors (such as GPR41 and GPR43), subsequently affecting miRNA expression, which may have significant implications for cardiovascular disease progression (Felice et al., 2015).
Moreover, trimethylamine N-oxide (TMAO), produced by gut microbiota through the metabolism of choline and carnitine, has been linked to an increased risk of cardiovascular diseases (Yang and Zhang, 2021). TMAO promotes the development of atherosclerosis by influencing cholesterol and bile acid metabolism (Zhen et al., 2023). Research indicates that TMAO can upregulate the expression of miR-33 in macrophages, a miRNA known to regulate cholesterol metabolism, thereby facilitating foam cell formation—a critical early event in atherosclerosis (Yang et al., 2022).
In conclusion, the exploration of metabolic heart disease encompasses the intricate interplay of metabolic dysregulation, gene expression regulation, and the influence of gut microbiota on cardiovascular health (Xue et al., 2022). These insights provide crucial foundations for understanding the underlying mechanisms and identifying potential therapeutic targets for addressing this pressing health concern (Santos et al., 2017; Tang et al., 2019).
The interaction between RNAs, the gut microbiota and metabolic diseases is a rapidly evolving field that provides new insights into the mechanisms underlying these diseases. Current studies emphasize that the gut microbiota plays a key role in regulating host metabolism and influencing the expression of RNA molecules, especially non-coding RNAs such as miRNAs and lncRNAs. These RNA molecules are key regulators of various metabolic processes, including lipid metabolism, glucose homeostasis, and inflammatory responses, all of which are closely linked to the onset and progression of metabolic diseases such as T2DM, obesity, and MAFLD (Table 1).
The ability of the gut microbiota to regulate RNA expression through the production of metabolites such as SCFAs and TMAOs suggests that the microbiota-RNA axis is an important regulatory mechanism for metabolic health. These metabolites not only regulate gene expression, but also affect post-transcriptional processes such as mRNA stability and translation efficiency. For example, SCFAs can regulate miRNA expression by altering chromatin structure, whereas TMAO can promote atherosclerosis by regulating miR-33.
In addition, the role of the gut microbiota in epigenetic modifications, particularly m6A methylation, further strengthens the link between RNA, gut microbiota, and metabolic diseases. Studies have shown that dysbiosis in the gut microbiota leads to aberrant m6A modifications, which in turn affects the expression of key metabolic genes, leading to metabolic dysfunction. These findings highlight the potential of targeting RNA modifications and the gut microbiota to prevent and treat metabolic diseases.
The complexity of metabolic disease mechanisms is underscored by the inter-regulatory relationship between RNA and the gut microbiota. As researchers continue to unravel the intricate pathways involved, it is clear that both RNA and gut microbiota offer promising targets for therapeutic intervention. Future research should focus on further elucidating these interactions, particularly through large-scale, multicenter clinical trials, in order to identify biomarkers that contribute to the early diagnosis of metabolic diseases and personalized treatment strategies.
By delving into the interactions between the gut microbiota and RNA in the pathogenesis of metabolic diseases, we can gain valuable insights into the diagnosis and prevention of related metabolic diseases. Whether targeting the gut microbiota or RNA alone, or intervening in the communication pathways between the two, these approaches offer unprecedented potential for targeted therapies for metabolic diseases. However, due to differences in RNA secretion patterns-such as variations in composition and levels across race, gender, body fluids, and feces-as well as numerous factors affecting the stability of the gut microbiota, RNA expression and gut microbiota abundance can vary significantly between subjects, even within the same disease context.
Therefore, these factors must be considered comprehensively when investigating the interactions between RNA and gut microbiota in relation to pathological changes in the host. Large-scale, multicenter clinical trials should be conducted to further elucidate the regulatory relationship between RNA and the gut microbiota, moving from theoretical proof to more concrete evidence. These studies will help to determine whether the gut microbiota or RNA can serve as a biomarker for metabolic diseases, thereby contributing to the prevention, diagnosis, and monitoring of these diseases. Ultimately, this will provide a solid theoretical basis for the development of new targeted therapies.
In conclusion, the convergence of RNA biology and gut microbiota research opens new avenues for understanding the molecular basis of metabolic diseases. By advancing our knowledge in this area, we can develop innovative approaches to preventing and treating disease, ultimately improving outcomes for patients with these pervasive health problems.
ZH: Visualization, Writing – original draft. QY: Visualization, Writing – original draft. SM: Visualization, Writing – original draft. JZ: Conceptualization, Writing – review & editing. XW: Conceptualization, Writing – review & editing. QM: Conceptualization, Writing – review & editing. YL: Conceptualization, Writing – review & editing. ZY: Conceptualization, Supervision, Writing – review & editing. XC: Conceptualization, Supervision, Writing – review & editing.
The author(s) declare that no financial support was received for the research, authorship, and/or publication of this article.
The authors declare that the research was conducted in the absence of any commercial or financial relationships that could be construed as a potential conflict of interest.
The authors declare that no Generative AI was used in the creation of this manuscript.
All claims expressed in this article are solely those of the authors and do not necessarily represent those of their affiliated organizations, or those of the publisher, the editors and the reviewers. Any product that may be evaluated in this article, or claim that may be made by its manufacturer, is not guaranteed or endorsed by the publisher.
Abedi, Z., Salehi, Z., Zaersabet, M., Mashayekhi, F., Sojoudi, H., Motamed, B., et al. (2024). The Impact of lncRNAs MIR503HG, TTN-AS1, and FEZF1-AS1 and Their Association with miR-107 Expression in Type 2 Diabetes Mellitus. Berlin: Springer.
Albillos, A., De Gottardi, A., and Rescigno, M. (2020). The gut-liver axis in liver disease: Pathophysiological basis for therapy. J. Hepatol. 72, 558–577.
Alisi, A., McCaughan, G., and Grønbæk, H. (2024). Role of gut microbiota and immune cells in metabolic-associated fatty liver disease: Clinical impact. Hepatol. Int. 18(Suppl. 2), 861–872. doi: 10.1007/s12072-024-10674-6
Alrefai, A. A., Khader, H. F., Elbasuony, H. A., Elzorkany, K., and Saleh, A. (2023). Evaluation of the expression levels of lncRNAs H19 and MEG3 in patients with type 2 diabetes mellitus. Mol. Biol. Rep. 50, 6075–6085.
Álvarez-Mercado, A. I., Navarro-Oliveros, M., Robles-Sánchez, C., Plaza-Díaz, J., Sáez-Lara, M., Muñoz-Quezada, S., et al. (2019). Microbial population changes and their relationship with human health and disease. Microorganisms 7:68.
Argano, C, Mirarchi, L, Amodeo, S, Orlando, V, Torres, A, Corrao, S., et al. (2023). The role of Vitamin D and its molecular bases in insulin resistance, diabetes, metabolic syndrome, and cardiovascular disease: State of the art. Int. J. Mol. Sci. 24(20):15485. doi: 10.3390/ijms242015485
Aron-Wisnewsky, J., Vigliotti, C., Witjes, J., Le, P., Holleboom, A. G., Verheij, J., et al. (2020). Gut microbiota and human NAFLD: Disentangling microbial signatures from metabolic disorders. Nat. Rev. Gastroenterol. Hepatol. 17, 279–297. doi: 10.1038/s41575-020-0269-9
Arunmanee, W., Pathania, M., Solovyova, A. S., Le Brun, A., Ridley, H., Baslé, A., et al. (2016). Gram-negative trimeric porins have specific LPS binding sites that are essential for porin biogenesis. Proc. Natl. Acad. Sci. 113, E5034–E5043. doi: 10.1073/pnas.1602382113
Bagheri Moghaddam, M., Maleki, M., Oveisee, M., Mowla, S. J., and Malakootian, M. (2022). Circular RNAs: New players in cardiomyopathy. Genes 13:1537. doi: 10.3390/genes13091537
Bayoumi, A. S., Aonuma, T., Teoh, J., Tang, L., and Kim, M. (2018). Circular noncoding RNAs as potential therapies and circulating biomarkers for cardiovascular diseases. Acta Pharmacol. Sin. 39, 1100–1109.
Benak, D., Benakova, S., Plecita-Hlavata, L., and Hlavackova, M. (2023). The role of m6A and m6Am RNA modifications in the pathogenesis of diabetes mellitus. Front. Endocrinol. 14:1223583. doi: 10.3389/fendo.2023.1223583
Benáková, Š, Holendová, B, and Plecitá-Hlavatá, L. (2021). Redox homeostasis in pancreatic β-cells: From development to failure. Antioxidants 10(4):526.
Bhat, M. I., and Kapila, R. (2017). Dietary metabolites derived from gut microbiota: Critical modulators of epigenetic changes in mammals. Nutr. Rev. 75, 374–389.
Bugger, H., Byrne, N. J., and Abel, E. D. (2022). Animal models of dysregulated cardiac metabolism. Circulation Res. 130, 1965–1993.
Canfrán-Duque, A., Rotllan, N., Zhang, X., Fernández-Fuertes, M., Ramírez-Hidalgo, C., Araldi, E., et al. (2017). Macrophage deficiency of miR-21 promotes apoptosis, plaque necrosis, and vascular inflammation during atherogenesis. EMBO Mol. Med. 9, 1244–1262. doi: 10.15252/emmm.201607492
Cao, X, Ge, J, Ma, Y, Ma, Y, Li, H, Han, W, Lamont, SJ, et al. (2024). MiR-20a-5p targeting the TGFBR2 Gene regulates inflammatory response of chicken macrophages infected with avian pathogenic E. coli. Animals 14(15): 2277. doi: 10.3390/ani14152277
Carding, S., Verbeke, K., Vipond, D. T., Corfe, B., and Owen, L. (2015). Dysbiosis of the gut microbiota in disease. Microb. Ecol. Health Dis. 26:26191.
Casado-Bedmar, M., Roy, M., Berthet, L., Hugot, J., Yang, C., Manceau, H., et al. (2024). Fecal let-7b and miR-21 directly modulate the intestinal microbiota, driving chronic inflammation. Gut Microbes 16:2394249. doi: 10.1080/19490976.2024.2394249
Caussy, C., Aubin, A., and Loomba, R. (2021). The relationship between type 2 diabetes. NAFLD, and cardiovascular risk. Curr. Diabetes Rep. 21, 1–13.
Chen, J., Deng, L. L., Xiao, X. L., Long, S., Deng, Y., Peng, T., et al. (2023). An association between decreased small intestinal RNA modification and disturbed glucagon-like Peptide-1 secretion under high-fat diet stress. Nutrients 15:3707. doi: 10.3390/nu15173707
Chiba, M, Uehara, H, Kuwata, H, and Niiyama, I. (2024). Extracellular miRNAs in the serum and feces of mice exposed to high-dose radiation. Biomed. Rep. 20(3), 1–7. doi: 10.3892/br.2024.1744
Cordeiro, B., Ahn, J. J., Gawde, S., Ucciferri, C., Revelo, X., Stickle, N., et al. (2024). Obesity intensifies sex-specific interferon signaling to selectively worsen central nervous system autoimmunity in females. Cell Metab. 36, 2298–2314.e11. doi: 10.1016/j.cmet.2024.07.017
D’Aquila, P., Lynn Carelli, L., De Rango, F., Passarino, G., and Bellizzi, D. (2020). Gut microbiota as important mediator between diet and DNA methylation and histone modifications in the host. Nutrients 12:597.
de Lima, E P, Moretti, Jr R C, Torres Pomini, K, Laurindo, LF, Sloan, KP, Sloan, LA, et al. (2024). Glycolipid metabolic disorders, metainflammation, oxidative stress, and cardiovascular diseases: Unraveling pathways. Biology 13(7):519. doi: 10.3390/biology13070519
Decatur, W. A., and Fournier, M. J. (2002). rRNA modifications and ribosome function. Trends Biochem. Sci. 27, 344–351.
Deprince, A., Haas, J. T., and Staels, B. (2020). Dysregulated lipid metabolism links NAFLD cardiovascular disease. Mol. Metab. 42:101092.
dos Santos Haber, J F, Barbalho, S M, Sgarbi, J A, Haber, A, Labio, R, Laurindo, F, et al. (2023). The relationship between type 1 diabetes mellitus, TNF-α, and IL-10 gene expression. Biomedicines 11(4): 1120. doi: 10.3390/biomedicines11041120
Drucker, D. J. (2022). GLP-1 physiology informs the pharmacotherapy of obesity. Mol. Metab. 57:101351.
Eizirik, D. L., Pasquali, L., and Cnop, M. (2020). Pancreatic β-cells in type 1 and type 2 diabetes mellitus: Different pathways to failure. Nat. Rev. Endocrinol. 16, 349–362.
Ejtahed, H. S., Angoorani, P., Soroush, A. R., Hasani-Ranjbar, S., Siadat, SD., Larijani, B., et al. (2020). Gut microbiota-derived metabolites in obesity: A systematic review. Biosci. Microbiota Food Health 39, 65–76.
El Mouali, Y., Tawk, C., Huang, K. D., Amend, L., Lesker, T. R., Ponath, F., et al. (2024). The RNA landscape of the human commensal Segatella copri reveals a small RNA essential for gut colonization. Cell Host Microbe 32(11), 1910–1926. e6. doi: 10.1016/j.chom.2024.09.008
Eslam, M., El-Serag, H. B., Francque, S., Sarin, S., Wei, L., Bugianesi, E., et al. (2022). Metabolic (dysfunction)-associated fatty liver disease in individuals of normal weight. Nat. Rev. Gastroenterol. Hepatol. 19, 638–651.
Fardi, F., Khasraghi, L. B., Shahbakhti, N., Salami Naseriyan, A., and Najafi, S. (2023). An interplay between non-coding RNAs and gut microbiota in human health. Diabetes Res. Clin. Pract. 201:110739. doi: 10.1016/j.diabres.2023.110739
Felice, C., Lewis, A., Armuzzi, A., Lindsay, J. O., and Silver, A. (2015). selective histone deacetylase isoforms as potential therapeutic targets in inflammatory bowel diseases. Alimentary Pharmacol. Therapeutics 41, 26–38.
Flint, H. J., Scott, K. P., Louis, P., and Duncan, S. (2012). The role of the gut microbiota in nutrition and health. Nat. Rev. Gastroenterol. Hepatol. 9, 577–589.
Fu, C., Dong, W., Wang, Z., Li, H., Qin, Q., Li, B., et al. (2014). The expression of miR-21 and miR-375 predict prognosis of esophageal cancer. Biochem. Biophys. Res. Commun. 446, 1197–1203.
Gao, D., Jiao, J., Wang, Z., Huang, X., Ni, X., Fang, S., et al. (2022). The roles of cell-cell and organ-organ crosstalk in the type 2 diabetes mellitus associated inflammatory microenvironment. Cytokine Growth Factor Rev. 66, 15–25. doi: 10.1016/j.cytogfr.2022.04.002
Gao, X., and Zhao, S. (2020). miRNA-16-5p inhibits the apoptosis of high glucose-induced pancreatic β cells via targeting of CXCL10: Potential biomarkers in type 1 diabetes mellitus. Endokrynol. Polska 71, 404–410.
Gomaa, E. Z. (2020). Human gut microbiota/microbiome in health and diseases: A review. Antonie Van Leeuwenhoek 113, 2019–2040. doi: 10.1007/s10482-020-01474-7
Goody, D., and Pfeifer, A. (2019). MicroRNAs in brown and beige fat. Biochim. Biophys. Acta Mol. Cell Biol. Lipids 1864, 29–36.
Grieco, F. A., Sebastiani, G., Juan-Mateu, J., Villate, O., Marroqui, L., Ladrière, L., et al. (2017). MicroRNAs miR-23a-3p, miR-23b-3p, and miR-149-5p regulate the expression of proapoptotic BH3-only proteins DP5 and PUMA in human pancreatic β-cells. Diabetes 66, 100–112.
Guo, M., Lu, M., Chen, K., Xu, R., Xia, Y., Liu, X., et al. (2023). Akkermansia muciniphila and Lactobacillus plantarum ameliorate systemic lupus erythematosus by possibly regulating immune response and remodeling gut microbiota. Msphere 8:e0007023. doi: 10.1128/msphere.00070-23
Guo, X. Y., Sun, F., Chen, J. N., Wang, Y., Pan, Q., Fan, J., et al. (2018). circRNA_0046366 inhibits hepatocellular steatosis by normalization of PPAR signaling. World J. Gastroenterol. 24:323. doi: 10.3748/wjg.v24.i3.323
Gutiérrez-Cuevas, J., Santos, A., and Armendariz-Borunda, J. (2021). Pathophysiological molecular mechanisms of obesity: A link between MAFLD and NASH with cardiovascular diseases. Int. J. Mol. Sci. 22:11629. doi: 10.3390/ijms222111629
Hashemi, M., Khosroshahi, E. M., Asadi, S., Tanha, M., Ghatei Mohseni, F., Abdolmohammad Sagha, R., et al. (2024). Emerging roles of non-coding RNAs in modulating the PI3K/Akt pathway in cancer. Non-coding RNA Res. 10, 1–15.
He, L., Zhou, X., Liu, Y., Zhou, L., and Li, F. (2022). Fecal miR-142a-3p from dextran sulfate sodium-challenge recovered mice prevents colitis by promoting the growth of Lactobacillus reuteri. Mol. Therapy 30(1), 388–399. doi: 10.1016/j.ymthe.2021.08.025
He, P. C., and He, C. (2021). m6A RNA methylation: From mechanisms to therapeutic potential. EMBO J. 40:e105977.
Huang, W., Wu, X., Xiang, S., Qiao, M., Cen, X., Pan, X., et al. (2022). Regulatory mechanism of miR-20a-5p expression in Cancer. Cell Death Discov. 8(1):262.
Icaza-Chá,vez, M. E. (2013). Gut microbiota in health and disease. Rev. Gastroenterol. México (English Edition) 78, 240–248.
Jabs, S., Biton, A., Bécavin, C., Nahori, M., Ghozlane, A., Pagliuso, A., et al. (2020). Impact of the gut microbiota on the m6A epitranscriptome of mouse cecum and liver. Nat. Commun. 11:1344. doi: 10.1038/s41467-020-15126-x
Jalink, E. A., Schonk, A. W., Boon, R. A., and Juni, P. (2024). Non-coding RNAs in the pathophysiology of heart failure with preserved ejection fraction. Front. Cardiovasc. Med. 10:1300375. doi: 10.3389/fcvm.2023.1300375
Jamar, G., Ribeiro, D. A., and Pisani, L. P. (2021). High-fat or high-sugar diets as trigger inflammation in the microbiota-gut-brain axis. Crit. Rev. Food Sci. Nutr. 61, 836–854.
Jandhyala, S. M., Talukdar, R., Subramanyam, C., Vuyyuru, H., Sasikala, M., Nageshwar Reddy, D., et al. (2015). Role of the normal gut microbiota. World J. Gastroenterol. 21:8787.
Jeyakumar, S. M., and Vajreswari, A. (2022). Stearoyl-CoA desaturase 1: A potential target for non-alcoholic fatty liver disease?-perspective on emerging experimental evidence. World J. Hepatol. 14:168. doi: 10.4254/wjh.v14.i1.168
Jia, Y., and Zhou, Y. (2020). Involvement of lncRNAs and macrophages: Potential regulatory link to angiogenesis. J. Immunol. Res. 2020:1704631. doi: 10.1155/2020/1704631
Jiang, X., Zheng, J., Zhang, S., Wang, B., Wu, C., Guo, X., et al. (2020). Advances in the involvement of gut microbiota in pathophysiology of NAFLD. Front. Med. 7:361. doi: 10.3389/fmed.2020.00361
Jusic, A., and Devaux, Y., and Eu-CardioRNA Cost Action (CA17129) (2020). Mitochondrial noncoding RNA-regulatory network in cardiovascular disease. Basic Res. Cardiol. 115:23. doi: 10.1007/s00395-020-0783-5
Khatana, C., Saini, N. K., Chakrabarti, S., Saini, V., Sharma, A., Saini, R. V., et al. (2020). Mechanistic insights into the oxidized low-density lipoprotein-induced atherosclerosis. Oxidative Med. Cell. Longevity 2020:5245308. doi: 10.1155/2020/5245308
Kmieć, Z., Cyman, M., and Ślebioda, T. J. (2017). Cells of the innate and adaptive immunity and their interactions in inflammatory bowel disease. Adv. Med. Sci. 62, 1–16.
Kong, Y., Hsieh, C. H., and Alonso, L. C. (2018). ANRIL: A lncRNA at the CDKN2A/B locus with roles in cancer and metabolic disease. Front. Endocrinol. 9:405. doi: 10.3389/fendo.2018.00405
Koutoukidis, D., Yen, S., Gomez Castro, P., Misheva, M., Jebb, S., Aveyard, P., et al. (2024). Changes in intestinal permeability and gut microbiota following diet-induced weight loss in patients with metabolic dysfunction-associated steatohepatitis and liver fibrosis. Gut Microbes 16:2392864. doi: 10.1080/19490976.2024.2392864
Krichevsky, A. M., and Gabriely, G. (2009). miR-21: A small multi-faceted RNA. J. Cell. Mol. Med. 13, 39–53. doi: 10.1111/j.1582-4934.2008.00556.x
Krist, B., Florczyk, U., Pietraszek-Gremplewicz, K., Józkowicz, A., and Dulak, J. (2015). The role of miR-378a in metabolism, angiogenesis, and muscle biology. Int. J. Endocrinol. 2015(1):281756. doi: 10.1155/2015/281756
Lakhter, A. J., Pratt, R. E., Moore, R. E., Doucette, K., Maier, B., DiMeglio, L., et al. (2018). Beta cell extracellular vesicle miR-21-5p cargo is increased in response to inflammatory cytokines and serves as a biomarker of type 1 diabetes. Diabetologia 61, 1124–1134. doi: 10.1007/s00125-018-4559-5
Lee, R., Feinbaum, R., and Ambros, V. (2004). A short history of a short RNA. Cell 116(2 Suppl.), S89–S92.
Lee, W. H., Najjar, S. M., Kahn, C. R., and Hinds, T. (2023). Hepatic insulin receptor: New views on the mechanisms of liver disease. Metabolism 145:155607. doi: 10.1016/j.metabol.2023.155607
Li, A., Huang, W., Zhang, X., Xie, L., and Miao, X. (2018). Identification and characterization of CircRNAs of two pig breeds as a new biomarker in metabolism-related diseases. Cell. Physiol. Biochem. 47, 2458–2470. doi: 10.1159/000491619
Li, C., Hu, J., Hu, X., Zhao, C., Mo, M., Zu, X., et al. (2021). LncRNA SNHG9 is a prognostic biomarker and correlated with immune infiltrates in prostate cancer. Transl. Androl. Urol. 10:215. doi: 10.21037/tau-20-1134
Li, J. J. H., Wang, W., Wang, X. Q., He, Y., Wang, S. S., Yan, Y. X., et al. (2019). A novel strategy of identifying circRNA biomarkers in cardiovascular disease by meta-analysis. J. Cell. Physiol. 234, 21601–21612. doi: 10.1002/jcp.28817
Li, J., Huang, Q., Long, X., Zhang, J., Huang, X., Aa, J., et al. (2015). CD147 reprograms fatty acid metabolism in hepatocellular carcinoma cells through Akt/mTOR/SREBP1c and P38/PPARα pathways. J. Hepatol. 63, 1378–1389.
Li, P. T., Vieregg, J., and Tinoco, I. (2008). How RNA unfolds and refolds. Annu. Rev. Biochem. 77, 77–100.
Lin, H. Y., Yang, Y. L., Wang, P. W., Wang, F. S., and Huang, Y. H. (2020). The emerging role of microRNAs in NAFLD: Highlight of microRNA-29a in modulating oxidative stress, inflammation, and beyond. Cells 9:1041. doi: 10.3390/cells9041041
Liu, S., Da Cunha, A. P., Rezende, R. M., Cialic, R., Wei, Z., Bry, L., et al. (2016). The host shapes the gut microbiota via fecal microRNA. Cell Host Microbe 19(1), 32–43.
Liu, J., Wu, A., Cai, J., She, Z., and Li, H. (2022). The contribution of the gut-liver axis to the immune signaling pathway of NAFLD. Front. Immunol. 13:968799. doi: 10.3389/fimmu.2022.968799
Liu, Y., Liu, H., Li, Y., Mao, R., Yang, H., Zhang, Y., et al. (2020). Circular RNA SAMD4A controls adipogenesis in obesity through the miR-138-5p/EZH2 axis. Theranostics 10:4705. doi: 10.7150/thno.42417
Ma, J., Piao, X., Mahfuz, S., Long, S., and Wanh, J. (2022). The interaction among gut microbes, the intestinal barrier and short chain fatty acids. Anim. Nutr. 9, 159–174.
Mohebi, R., Chen, C., Ibrahim, N. E., McCarthy, C. P., Gaggin, H. K., Singer, D. E., et al. (2022). Cardiovascular disease projections in the United States based on the 2020 census estimates. J. Am. Coll. Cardiol. 80, 565–578.
Monné, M., Marobbio, C. M., Agrimi, G., Palmieri, L., and Palmieri, F. (2022). Mitochondrial transport and metabolism of the major methyl donor and versatile cofactor S-adenosylmethionine, and related diseases: A review. IUBMB Life 74, 573–591. doi: 10.1002/iub.2658
Monzel, E., and Desai, M. S. (2024). Bacterial small RNA makes a big impact for gut colonization. Cell Host Microbe 32(11), 1875–1877. doi: 10.1016/j.chom.2024.10.010
Mozaffarian, D., Wilson, P. W. F., and Kannel, W. B. (2008). Beyond established and novel risk factors: Lifestyle risk factors for cardiovascular disease. Circulation 117, 3031–3038.
Mundula, T., Russo, E., Curini, L., Giudici, F., Piccioni, A., Franceschi, F., et al. (2022). Chronic systemic low-grade inflammation and modern lifestyle: The dark role of gut microbiota on related diseases with a focus on COVID-19 pandemic. Curr. Med. Chem. 29(33), 5370–5396. doi: 10.2174/0929867329666220430131018
Nakata, K., Sugi, Y., Narabayashi, H., Narabayashi, H., Kobayakawa, T., Nakanishi, Y., et al. (2017). Commensal microbiota-induced microRNA modulates intestinal epithelial permeability through the small GTPase ARF4. J. Biol. Chem. 292(37), 15426–15433. doi: 10.1074/jbc.M117.788596
Nasci, V. L., Chuppa, S., Griswold, L., Goodreau, K. A., Dash, R. K., and Kriegel, A. J. (2019). miR-21-5p regulates mitochondrial respiration and lipid content in H9C2 cells. Am. J. Physiol. Heart Circ. Physiol. 316, H710–H721.
Nicoletti, A., Ponziani, F. R., Biolato, M., Valenza, V., Marrone, G., Sganga, G., et al. (2019). Intestinal permeability in the pathogenesis of liver damage: From non-alcoholic fatty liver disease to liver transplantation. World J. Gastroenterol. 25:4814.
Nie, P., Li, Z., Wang, Y., Zhang, Y., Zhao, M., Luo, J., et al. (2019). Gut microbiome interventions in human health and diseases. Med. Res. Rev. 39(6), 2286–2313. doi: 10.1002/med.21584
Nigi, L., Grieco, G. E., Ventriglia, G., Brusco, N., Mancarella, F., Formichi, C., et al. (2018). MicroRNAs as regulators of insulin signaling: Research updates and potential therapeutic perspectives in type 2 diabetes. Int. J. Mol. Sci. 19:3705. doi: 10.3390/ijms19123705
Olivo-Martínez, Y., Martínez-Ruiz, S., Cordero, C., Badia, J., and Baldoma, L. (2024). Extracellular vesicles of the probiotic Escherichia coli Nissle 1917 reduce PepT1 levels in IL-1β-Treated Caco-2 cells via Upregulation of miR-193a-3p. Nutrients 16(16):2719.
Pan, L., Lian, W., Zhang, X., Han, S., Cao, C., Li, X., et al. (2018). Human circular RNA-0054633 regulates high glucose-induced vascular endothelial cell dysfunction through the microRNA-218/roundabout 1 and microRNA-218/heme oxygenase-1 axes. Int. J. Mol. Med. 42, 597–606. doi: 10.3892/ijmm.2018.3625
Pan, X., Huang, C., and Li, J. (2021). The emerging roles of m6A modification in liver carcinogenesis. Int. J. Biol. Sci. 17:271.
Pei, X., Wen, Y., Cui, F., Yang, Z., and Xie, Z. (2021). lncRNA CASC7 regulates pathological progression of ox-LDL-stimulated atherosclerotic cell models via sponging miR-21 and regulating PI3K/Akt and TLR4/NF-κB signaling pathways. Aging (Albany NY) 13:25408.
Pittner, R. A., Moore, C. X., Bhavsar, S. P., Gedulin, B., Smith, P., Jodka, C., et al. (2004). Effects of PYY [3–36] in rodent models of diabetes and obesity. Int. J. Obesity 28, 963–971.
Polyzos, S. A., Kountouras, J., and Mantzoros, C. S. (2019). Obesity and nonalcoholic fatty liver disease: From pathophysiology to therapeutics. Metabolism 92, 82–97.
Prattichizzo, F., Matacchione, G., Giuliani, A., Sabbatinelli, J., Olivieri, F., de Candia, P., et al. (2021). Extracellular vesicle-shuttled miRNAs: A critical appraisal of their potential as nano-diagnostics and nano-therapeutics in type 2 diabetes mellitus and its cardiovascular complications. Theranostics 11:1031. doi: 10.7150/thno.51605
Rasouli-Saravani, A., Jahankhani, K., Moradi, S., Gorgani, M., Shafaghat, Z., Mirsanei, Z., et al. (2023). Role of microbiota short-chain fatty acids in the pathogenesis of autoimmune diseases. Biomed. Pharmacother. 162:114620.
Rattanaprasert, M., van Pijkeren, J., Ramer-Tait, A., Quintero, M., Kok, C., Walter, J., et al. (2019). Genes involved in galactooligosaccharide Metabolism in Lactobacillus reuteri and their ecological role in the gastrointestinal tract. Appl. Environ. Microbiol. 85:e01788-19. doi: 10.1128/AEM.01788-19
Ren, H., and Wang, Q. (2021). Non-coding RNA and diabetic kidney disease. DNA Cell Biol. 40, 553–567.
Roth, G. A., Mensah, G. A., Johnson, C. O., Addolorato, G., Ammirati, E., Baddour, L. M., et al. (2020). Global burden of cardiovascular diseases and risk factors, 1990–2019: Update from the GBD 2019 study. J. Am. Coll. Cardiol. 76, 2982–3021.
Santos, R., Ursu, O., Gaulton, A., Bento, A. P., Donadi, R. S., Bologa, C. G., et al. (2017). A comprehensive map of molecular drug targets. Nat. Rev. Drug Discov. 16, 19–34.
Satheesan, A., Kumar, J., Leela, K. V., Murugesan, R., Chaithanya, V., Angelina, M., et al. (2024). Review on the role of nucleotide-binding oligomerization domain-like receptor protein 3 (NLRP3) inflammasome pathway in diabetes: Mechanistic insights and therapeutic implications. Inflammopharmacology 32, 2753–2779. doi: 10.1007/s10787-024-01556-2
Scheppach, W. (1994). Effects of short chain fatty acids on gut morphology and function. Gut 35(1 Suppl.), S35–S38.
Shao, Y., Dong, Y., Zhou, J., Lu, Z., Chen, C., Yuan, X., et al. (2024). Advances in the study of antisense long-stranded non-coding RNAs in tumors. Int. J. Oncol. 64, 1–17. doi: 10.3892/ijo.2024.5610
Shen, Q., Huang, Z., Ma, L., Yao, J., Luo, T., Zhao, Y., et al. (2022). Extracellular vesicle miRNAs promote the intestinal microenvironment by interacting with microbes in colitis. Gut Microbes 14(1):2128604. doi: 10.1080/19490976.2022.2128604
Shi, L., Chen, J., Yang, J., Pan, T., Zhang, S., Wang, Z., et al. (2010). MiR-21 protected human glioblastoma U87MG cells from chemotherapeutic drug temozolomide induced apoptosis by decreasing Bax/Bcl-2 ratio and caspase-3 activity. Brain Res. 1352, 255–264. doi: 10.1016/j.brainres.2010.07.009
Shin, N. R., Whon, T. W., and Bae, J. W. (2015). Proteobacteria: Microbial signature of dysbiosis in gut microbiota. Trends Biotechnol. 33, 496–503. doi: 10.1016/j.tibtech.2015.06.011
Stojanov, S., and Kreft, S. (2020). Gut microbiota and the metabolism of phytoestrogens. Rev. Brasileira de Farmacognosia 30, 145–154.
Suhre, K., and Zaghlool, S. (2021). Connecting the epigenome, metabolome and proteome for a deeper understanding of disease. J. Internal Med. 290(3), 527–548. doi: 10.1111/joim.13306
Sun, H., Ma, Y., Cao, X., Li, H., Han, W., Qu, L. J., et al. (2024). PTEN regulated by gga-miR-20a-5p is involved in chicken macrophages inflammatory response to APEC infection via autophagy. Poultry Sci. 103(11):104170. doi: 10.1016/j.psj.2024.104170
Sun, L., Ma, L., Ma, Y., Zhang, F., Zhao, C., Nie, Y., et al. (2018). Insights into the role of gut microbiota in obesity: Pathogenesis, mechanisms, and therapeutic perspectives. Protein Cell 9, 397–403.
Surina, S., Fontanella, R. A., Scisciola, L., Marfella, R., Paolisso, G., Barbieri, M., et al. (2021). miR-21 in human cardiomyopathies. Front. Cardiovascular Med. 8:767064.
Tanase, D. M., Gosav, E. M., Neculae, E., Costea, C., Ciocoiu, M., Hurjui, L., et al. (2020). Role of gut microbiota on onset and progression of microvascular complications of type 2 diabetes (T2DM). Nutrients 12:3719. doi: 10.3390/nu12123719
Tang, W. H. W., Li, D. Y., and Hazen, S. L. (2019). Dietary metabolism, the gut microbiome, and heart failure. Nat. Rev. Cardiol. 16, 137–154. doi: 10.1038/s41569-018-0108-7
Thursby, E., and Juge, N. (2017). Introduction to the human gut microbiota. Biochem. J. 474, 1823–1836.
Tudurachi, B. S., Anghel, L., Tudurachi, A., Sascau, R., Zanfirescu, R., Statescu, C., et al. (2024). Unraveling the cardiac matrix: From diabetes to heart failure, exploring pathways and potential medications. Biomedicines 12:1314. doi: 10.3390/biomedicines12061314
Virtue, A. T., McCright, S. J., Wright, J. M., Jimenez, M. T., Mowel, W. K., Kotzin, J. J., et al. (2019). The gut microbiota regulates white adipose tissue inflammation and obesity via a family of microRNAs. Sci. Transl. Med. 11(496):eaav1892. doi: 10.1126/scitranslmed.aav1892
Visekruna, A., and Luu, M. (2021). The role of short-chain fatty acids and bile acids in intestinal and liver function, inflammation, and carcinogenesis. Front. Cell Dev. Biol. 9:703218. doi: 10.3389/fcell.2021.703218
Wang, J., Duan, Y., Sluijter, J. P. G., and Xiao, J. (2019). Lymphocytic subsets play distinct roles in heart diseases. Theranostics 9:4030.
Wang, M., Wichienchot, S., He, X., Fu, X., Huang, Q., Zhang, B., et al. (2019). In vitro colonic fermentation of dietary fibers: Fermentation rate, short-chain fatty acid production and changes in microbiota. Trends Food Sci. Technol. 88, 1–9.
Wang, Q., Chen, C., Ding, Q., Zhao, Y., Wang, Z., Chen, J., et al. (2020). METTL3-mediated m6A modification of HDGF mRNA promotes gastric cancer progression and has prognostic significance. Gut 69, 1193–1205. doi: 10.1136/gutjnl-2019-319639
Wang, Q., He, Y., Li, X., Zhang, T., Liang, M., Wang, G., et al. (2022). Lactobacillus reuteri CCFM8631 Alleviates hypercholesterolaemia caused by the paigen atherogenic diet by regulating the gut microbiota. Nutrients 14:1272. doi: 10.3390/nu14061272
Wang, Y., Wang, M., Chen, J., Li, Y., Kuang, Z., Dende, C., et al. (2023). The gut microbiota reprograms intestinal lipid metabolism through long noncoding RNA Snhg9. Science 381, 851–857. doi: 10.1126/science.ade0522
Wilson, R. A., Deasy, W., Hayes, A., and Cooke, M. (2017). High fat diet and associated changes in the expression of micro-RNAs in tissue: Lessons learned from animal studies. Mol. Nutr. Food Res. 61:1600943. doi: 10.1002/mnfr.201600943
Wishart, D. S. (2019). Metabolomics for investigating physiological and pathophysiological processes. Physiol. Rev. 99, 1819–1875.
Wortelboer, K., Bakker, G. J., Winkelmeijer, M., van Riel, N., Levin, E., Nieuwdorp, M., et al. (2022). Fecal microbiota transplantation as tool to study the interrelation between microbiota composition and miRNA expression. Microbiol. Res. 257: 126972. doi: 10.1016/j.micres.2022.126972
Wu, J., Zhao, Y., Wang, X., Kong, L., Johnston, L., Lu, L., et al. (2022). Dietary nutrients shape gut microbes and intestinal mucosa via epigenetic modifications. Crit. Rev. Food Sci. Nutr. 62, 783–797. doi: 10.1080/10408398.2020.1828813
Wu, X. Q., Zhao, L., Zhao, Y. L., He, X., Zou, L., Zhao, Y., et al. (2024). Traditional Chinese medicine improved diabetic kidney disease through targeting gut microbiota. Pharm. Biol. 62, 423–435.
Wu, Y., Xu, H., Tu, X., and Gao, Z. (2021). The role of short-chain fatty acids of gut microbiota origin in hypertension. Front. Microbiol. 12:730809. doi: 10.3389/fmicb.2021.730809
Xue, H., Chen, X., Yu, C., Deng, Y., Zhang, Y., Chen, S., et al. (2022). Gut microbially produced indole-3-propionic acid inhibits atherosclerosis by promoting reverse cholesterol transport and its deficiency is causally related to atherosclerotic cardiovascular disease. Circulation Res. 131, 404–420. doi: 10.1161/CIRCRESAHA.122.321253
Yang, J. Y., Zhang, T. T., Yu, Z. L., Wang, C. C., Zhao, Y. C., Wang, Y. M., et al. (2022). Taurine alleviates trimethylamine N-oxide-induced atherosclerosis by regulating bile acid metabolism in ApoE–/–mice. J. Agric. Food Chem. 70, 5738–5747. doi: 10.1021/acs.jafc.2c01376
Yang, M., and Zhang, C. Y. (2021). G protein-coupled receptors as potential targets for nonalcoholic fatty liver disease treatment. World J. Gastroenterol. 27:677.
Ye, D., Zhang, T., Lou, G., Xu, W., Dong, F., Chen, G., et al. (2018). Plasma miR-17, miR-20a, miR-20b and miR-122 as potential biomarkers for diagnosis of NAFLD in type 2 diabetes mellitus patients. Life Sci. 208, 201–207. doi: 10.1016/j.lfs.2018.07.029
Yepmo, M., Potier, J. B., Pinget, M., Grabarz, A., Bouzakri, K., Bourie, A., et al. (2022). Discussing the role of circular RNA in the pathogenesis of non-alcoholic fatty liver disease and its complications. Front. Endocrinol. 13:1035159. doi: 10.3389/fendo.2022.1035159
Yki-Järvinen, H. (2014). Non-alcoholic fatty liver disease as a cause and a consequence of metabolic syndrome. Lancet Diabetes Endocrinol. 2, 901–910. doi: 10.1016/S2213-8587(14)70032-4
Yu, G., Yang, Z., Peng, T., and Lu, Y. (2021). Circular RNAs: Rising stars in lipid metabolism and lipid disorders. J. Cell. Physiol. 236, 4797–4806. doi: 10.1002/jcp.30200
Yuan, C., Steer, C. J., and Subramanian, S. (2019). Host–MicroRNA–microbiota interactions in colorectal cancer. Genes 10:270. doi: 10.3390/genes10040270
Zaiou, M. (2020). circRNAs signature as potential diagnostic and prognostic biomarker for diabetes mellitus and related cardiovascular complications. Cells 9:659. doi: 10.3390/cells9030659
Zeng, Q., Liu, C. H., Ampuero, J., Wu, D., Jiang, W., Zhou, L., et al. (2024). Circular RNAs in non-alcoholic fatty liver disease: Functions and clinical significance. RNA Biol. 21, 1–15. doi: 10.1080/15476286.2023.2290769
Zhang, C., Liu, H., Sun, L., Wang, Y., Chen, X., Du, J., et al. (2023). An overview of host-derived molecules that interact with gut microbiota. Imeta 2(2):e88.
Zhang, H., Li, J., Shao, W., and Shen, N. (2020). LncRNA SNHG9 is downregulated in osteoarthritis and inhibits chondrocyte apoptosis by downregulating miR-34a through methylation. BMC Musculoskeletal Disord. 21:511. doi: 10.1186/s12891-020-03497-7
Zhao, Y., Wu, J., Liangpunsakul, S., and Wang, L. (2017). Long non-coding RNA in liver metabolism and disease: Current status. Liver Res. 1, 163–167.
Zhen, J., Zhou, Z., He, M., Han, M., Lv, H., Wen, P., et al. (2023). The gut microbial metabolite trimethylamine N-oxide and cardiovascular diseases. Front. Endocrinol. 14:1085041. doi: 10.3389/fendo.2023.1085041
Zhou, H., and Kuang, H. (2021). Circular RNAs: Novel target of diabetic retinopathy. Rev. Endocrine Metab. Disorders 22, 205–216.
Keywords: RNA, metabolic diseases, gut microbiota, metabolism, microbial metabolites
Citation: Huang Z, Yao Q, Ma S, Zhou J, Wang X, Meng Q, Liu Y, Yu Z and Chen X (2025) The synergistic role of gut microbiota and RNA in metabolic diseases: mechanisms and therapeutic insights. Front. Microbiol. 16:1504395. doi: 10.3389/fmicb.2025.1504395
Received: 30 September 2024; Accepted: 09 January 2025;
Published: 29 January 2025.
Edited by:
Marina Burgos Da Silva, Memorial Sloan Kettering Cancer Center, United StatesReviewed by:
Spase Stojanov, Institut Jožef Stefan (IJS), SloveniaCopyright © 2025 Huang, Yao, Ma, Zhou, Wang, Meng, Liu, Yu and Chen. This is an open-access article distributed under the terms of the Creative Commons Attribution License (CC BY). The use, distribution or reproduction in other forums is permitted, provided the original author(s) and the copyright owner(s) are credited and that the original publication in this journal is cited, in accordance with accepted academic practice. No use, distribution or reproduction is permitted which does not comply with these terms.
*Correspondence: Zihan Yu, yuzihan@tmu.edu.cn; orcid.org/0000-0002-2696-2297; Xin Chen, xchen03@tmu.edu.cn; orcid.org/0000-0003-3024-9053
†These authors have contributed equally to this work
Disclaimer: All claims expressed in this article are solely those of the authors and do not necessarily represent those of their affiliated organizations, or those of the publisher, the editors and the reviewers. Any product that may be evaluated in this article or claim that may be made by its manufacturer is not guaranteed or endorsed by the publisher.
Research integrity at Frontiers
Learn more about the work of our research integrity team to safeguard the quality of each article we publish.