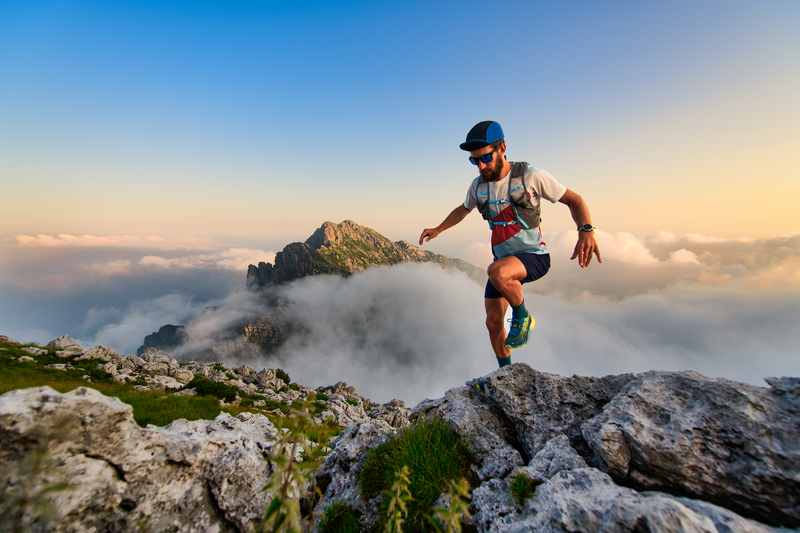
95% of researchers rate our articles as excellent or good
Learn more about the work of our research integrity team to safeguard the quality of each article we publish.
Find out more
ORIGINAL RESEARCH article
Front. Microbiol. , 20 March 2025
Sec. Microorganisms in Vertebrate Digestive Systems
Volume 16 - 2025 | https://doi.org/10.3389/fmicb.2025.1485688
This article is part of the Research Topic Unravelling the Unknown of the Rumen Microbiome: Implications for Animal Health, Productivity, and Beyond View all 20 articles
Both corn gluten feed and bakery by-products are important alternative concentrate feedstuffs for ruminants. Bakery by-products, which are rich in ether extract (EE) and starch, have the potential to be utilized as concentrate feedstuffs for ruminants, with a capacity to reduce ruminal methanogenesis. In the study, fermented corn gluten feed (FCG) and fermented bakery by-products (FBP) were mixed with other feedstuffs to formulate FCG and FBP diets, respectively. Twenty growing Holstein bulls, weighing 241 ± 10.5 kg, were randomly assigned to one of two dietary treatments: FCG or FBP diet. The aim was to investigate effects of replacing FCG with FBP feedstuff on nutrient digestibility, ruminal fermentation, ruminal microbiota, and methanogenesis. Results showed that the bulls feeding FBP diet had greater starch intake (p < 0.01) and digestibility (p = 0.04), EE intake (p < 0.01) and digestibility (p = 0.01), molar proportion of ruminal propionate (p < 0.01), while lower crude protein (CP) (p < 0.01) and neutral detergent fiber (NDF) digestibility (p = 0.01), ruminal dissolved methane concentration (p = 0.02), percentage of ruminal acetate (p < 0.01) and butyrate (p < 0.01), and the ratio of acetate to propionate (p < 0.01), in comparison with those feeding FCG diet. Further investigation on the bacterial community indicated that feeding the FBP diet had greater abundance of Succiniclasticum (p = 0.02), Megasphaera (p < 0.01), Lachnospiraceae_unclassified (p < 0.01) and Lachnospira (p < 0.01), while lower abundance of Christensenellaceae_R-7_group (p < 0.01), Ruminococcus (p < 0.01) and NK4A214_group (p = 0.01). The increases in EE and starch intakes after the substitution of FCG by FBP feedstuff alter fermentation rumen pathway from acetate to propionate production through enriching the propionate producers with net hydrogen incorporation, and reduced ruminal methanogenesis.
By-products that are high in ether extract (EE) and starch offer strategies to meet the nutrient requirements of growing ruminants, while reducing food competition with humans (Humer et al., 2018; Kaltenegger et al., 2020). Bakery by-products, which are leftovers from food processing plants or bakeries, are available in large quantities and have the potential to be used as concentrate feed for ruminants. Recently, a new segment of the feed industry based on bakery by-products has emerged (Humer et al., 2018).
Corn gluten feed is a by-product of corn starch production via the wet milling process and includes bran and steep (Jiao et al., 2022). It is rich in digestible corn fiber and protein, and has been widely used as a concentrate feedstuff for ruminants (Bernard et al., 1991; Siverson et al., 2014). While corn gluten feed has been reported to have no negative effects on the productive performance of dairy cows or steers, replacing grains with corn gluten feed might result in greater methane production due to its high fiber and low starch content.
Archaeal methanogens use hydrogen as an energy source for their own growth and are the primary hydrogen users in the rumen with methane as end products (Wang et al., 2013). Methane production represents a decrease in energetic efficiency, contributes to greenhouse gas emissions and exerts great impact on global climate change (Li et al., 2022; Wang et al., 2022; Wang et al., 2024). Higher levels of starch in the diet would degrade more rapidly into volatile fatty acids (VFAs), leading to a lower ruminal pH, which can inhibit the metabolic activity and numbers of methanogens (Van Kessel and Russell, 1996; Qiu et al., 2023). Additionally, starch fermentation in the rumen favors propionate production, which serves as a hydrogen sink (Hegarty, 1999; Wang et al., 2016a,b). Apart from starch, higher fat concentration in the diet can decrease ruminal methanogenesis by reducing the metabolic activity and numbers of protozoa and/or enhancing hydrogen utilization through biohydrogenation (Henderson, 1973; Zhang et al., 2019). Therefore, it is speculated that high levels of starch and EE in the bakery by-products could reduce ruminal methanogenesis when used in the ruminant diets.
The high sugar and moisture contents of bakery by-products or corn gluten feed make them prone to spoilage, which can be mitigated through solid-state fermentation. Therefore, bakery by-products (a mixture of bread, rolls, biscuits and cakes) and corn gluten feed were fermented to obtain fermented bakery by-products (FBP) and fermented corn gluten feed (FCG) feedstuff, respectively. The substitution of FCG with FBP feedstuff could lead to changes in both ruminal fermentation end-products and the microbial community in bulls, primarily due to an increase in starch and EE content or a reduction in fiber in the diets. It was hypothesized that the ruminal methanogenesis could be suppressed by the greater inclusion of starch and EE following the replacement of FCG with FBP feedstuff. To test this hypothesis, the objective of the study was to compare the effects of FCG feed and FBP on methanogenesis, fermentation pathways, and the bacterial community in the rumen of growing Holstein bulls.
All animals involved in the experiment were cared for in accordance with the Animal Care and Use Guidelines of the Animal Care Committee, Institute of Subtropical Agriculture, the Chinese Academy of Sciences, Changsha, China, with all animal experimental procedures approved by the committee (approval number ISA-WM-202101).
Bakery by-products used in the present study comprised a mixture of 600 g/kg bread crumbs, 200 g/kg cookie crumbs and 200 g/kg cake crumbs, which were sourced from Munters new Co., Ltd (Nanning, China). Semi-dried corn gluten feed was sourced from Nanning Junwu Biological Technology Co., Ltd (Nanning, China). Both of these feedstuffs were sprayed with distilled water to adjust the moisture content to approximately 330 g/kg on a dry matter (DM) basis. They were then fermented in non-anaerobic bags (each weighing approximately 50 kg per bag) at 25°C for 15 d. Samples of FCG and FBP were dried at 65°C for subsequent chemical composition analysis (Table 1).
Table 1. Chemical composition of fermented corn gluten feed (FCG) or fermented by-products (FBP) feedstuff.
Twenty healthy growing Holstein bulls, weighing 241 kg ± 10.53 kg, were employed and randomly assigned to one of the two dietary treatments, with 10 bulls per group. The treatments consisted of diets containing either FCG or FBP feedstuff. The FBP diet was formulated to completely replace FCG by FBP feedstuff. Both diets were designed to meet appropriately 1.2–1.3 times the energy and protein requirements of growing beef bulls (Table 2), and were offered as total mixed rations which were mixed in the TMR machine (9JGW-5A). All bulls were housed in individual pens, and fed equally at 08:00 and 16:00 daily. Feed offered and refused was weighed and recorded each day. The experiment lasted for 67 days, including a 7-d period for environmental and dietary adaption and a 60-d period for measuring animal performance and collecting samples. Sampling was conducted on the last 7 d of the experiment, consisting of a 5-d for digestibility measurements and a 2-d for the collection of rumen contents.
Table 2. Ingredients and chemical composition of diets containing fermented corn gluten feed (FCG) or fermented by-products (FBP) feedstuff.
The total fecal output was collected from each bull from d 61 to d 65. Feces were collected immediately after defecation to avoid contamination by urine, hair, feed, or bedding. The feces were thoroughly mixed multiple times a day into a large plastic garbage container with a black garbage bag covering the top to reduce nitrogen losses before subsampling. One subsample (∽1%) was collected and fixed with 10% (w/w) H2SO4 for CP concentration analyses, and another subsample (∽1%) was collected for analysis of other chemical constituents. The feed provided and any refusals were also weighed and sampled during these 5 consecutive days for the calculation of corresponding feed intake and nutrient digestibility. All samples were dried in a forced oven at 65°C and ground to pass through a 4 mm sieve for subsequent chemical composition analysis.
Rumen contents were collected from the middle part of the rumen using an oral stomach tube before and 2.5 h after the morning feeding from d 66 to d 67 according to Wang et al. (2016b). To minimize saliva contamination, the initial 50 mL of rumen contents were discarded. The ruminal pH was promptly measured with a pH meter (Starter 300, Ohaus Instruments Co., Ltd., Shanghai, China). Two 35-mL subsamples of rumen contents were placed into a 60-mL syringe, which was connected to a 20-mL syringe via a tube with a length of 2–3 cm. The 20-mL syringe was previously filled with 10 mL nitrogen gas. The nitrogen gas was then injected into the 60-mL syringe through the tube, and the dissolved gasses were extracted into nitrogen in a table concentrator at 200 r/min for 5 min. The extracted gasses were injected into the 20-mL syringe and put into 3.7-mL evacuated tubes for the determination of dissolved hydrogen and dissolved methane.
Another two 10-mL subsamples of rumen contents were promptly frozen and stored at −80°C for subsequent DNA extraction and microbial analysis. Additionally, three 2-mL subsamples of rumen contents were centrifuged at 15,000 g for 10 min at 4°C. Aliquots of the supernatants (1.5 mL) were transferred into plastic tubes each containing 0.15 mL of 25% (w/v) metaphosphoric acid, then stored at −20°C for VFA analysis.
The DM (Method 945.15), organic matter (OM) (Method 942.05), CP (Method 954.01), and EE (Method 920.39) were determined according to AOAC (2005). NDF was measured with the inclusion of sodium sulfite and α-amylase according to Van Soest et al. (1991). Gross energy (GE) was determined using an isothermal automatic calorimeter (5E-AC8018; Changsha Kaiyuan Instruments Co., Ltd., Changsha, Hunan). Starch content was determined following the method as described by Karthner and Theurer (1981). The concentration of dissolved hydrogen and dissolved methane were determined and calculated according to Wang et al. (2016a). In detail, concentration of dissolved hydrogen and methane were determined by gas chromatography (Agilent 7890A, Agilent Inc., Palo Alto, CA) using a thermal conductivity and a flame ionization detector, respectively. Hydrogen and methane were separated using a Hayesep Q packed column (2.44 m × 1/8 in. × 2.0 mm ID). Individual VFA was analyzed by gas chromatography (Agilent 7890A, Agilent Inc., Palo Alto, CA) as described by Wang et al. (2014). In detail, after re-centrifuging at 15,000 g, the VFA in the supernatants were measured using gas chromatography. The acids were separated with a DB-FFAP column, and detected with an R flame ionization detector. The carrier gas was nitrogen at a rate of 0.8 mL/min. The analysis was initially isothermal for 2 min at 60°C and then increased to 220°C at a rate of 20°C/min, with a detector temperature of 280°C. VFA were identified and quantified from chromatograph peak areas using calibration with external standards. RNH2 was calculated using the stoichiometric models as described by Wang et al. (2016b).
The DNA was extracted using the RBB + C method as modified by Yu and Morrison (2004). The quantity and quality of the extracted DNA were evaluated using an ND-2000 spectrophotometer (NanoDrop Technologies, Wilmington, DE). Illumina sequencing was performed on the NovaSeq platform at Huayu Gene Technology Co. Ltd. The 16S rRNA V3-V4 hyper variable regions were amplified with a 6 bp barcode unique to each sample by using the universal primers (341F: 5′-CCTAYGGGRBGCASCAG-3′, 806R: 5′-GGACTACNNGGGTATCTAAT-3′), each tagged with a unique 6 bp barcode for each sample. After PCR amplification, all amplicon libraries were sequenced and the barcodes and sequencing primers were removed before data processing. Data were analyzed according to the methodology described by Li et al. (2022) to obtain the alpha diversity indexes and taxonomic relative abundance. Beta diversity was analyzed based on Bray–Curtis similarity distances (Bray and Curtis, 1957).
The SPSS 22.0 software was employed for data analysis. Data were analyzed using a general linear model with the following model:
where μ is the overall mean; Di is the fixed effect of dietary treatment (i = 2, FCG or FBP diet), Bj is the random effect of Holstein bulls (j = 10), and eijk is the random error term. When sampling time was included, a linear mixed model was employed with the following model:
where μ is the overall mean; Di is the fixed effect of dietary treatment (i = 2, FCG or FBP diet); Tk is the repeated measurement of sampling time (k = 2, 0 and 2.5 h relative to the morning feeding); Di × Tk is the interaction between dietary treatment and sampling time; Aj is the random animal effect (j = 10); and eijkm is the random error term.
Microbial community composition with relative abundance of bacteria was derived from amplicon data according to Xu et al. (2023), which were analyzed using the Wilcoxon rank-sum test in JMP Pro software (version 16.1.0, SAS Institute Inc., Cary, NC, United States). Statistical significance of effects was declared at p < 0.05, and a tendency toward significance was noted for 0.05 ≤ p < 0.10.
The FBP feedstuff contained high levels of EE and starch, and low levels of CP and NDF (Table 1), resulting in significant chemical differences between the FBP and FCG diets (Table 2). Despite similar dry matter intake (DMI) between the bulls fed FBP and FCG diets, those on the FBP diet had higher (p < 0.05) intake of EE and starch (Table 3). Compared to the FCG diet, the FBP diet exhibited greater (p < 0.05) apparent total-tract digestibility of EE and starch, but had lower (p < 0.05) digestibility of CP and NDF (Table 3). The results led to similar DM and OM digestibility between the two dietary treatments (Table 3).
Table 3. Feed intake and apparent total-tract digestibility in growing Holstein bulls fed diets containing fermented corn gluten feed (FCG) or fermented by-products (FBP) feedstuff.
Bulls on the FBP diet had lower (p < 0.05) ruminal pH values and dissolved methane concentrations compared to those on the FCG diet (Table 4). Distinct ruminal fermentation profiles were observed between the two diets, with the FBP diet resulting in higher (p < 0.05) molar proportions of propionate, and lower (p < 0.05) molar proportions of acetate, butyrate, the acetate to propionate ratio, and RNH2 compared to the FCG diet (Table 4). There was a significant interaction effect of diet and sampling time on ruminal pH and total VFA concentration, with greater (p < 0.05) total VFA concentration and lower (p < 0.05) pH in the FBP diet compared to the FCG diet after the 2.5 h of morning feeding (Figure 1).
Table 4. Ruminal dissolved gasses and fermentation end products in growing Holstein bulls fed with diets containing fermented corn gluten feed (FCG) or fermented by-products (FBP) feedstuff.
Figure 1. Ruminal pH and total VFA concentration at 0 and 2.5 h after the morning feeding in the rumen of growing Holstein bulls fed with diets containing fermented corn gluten feed (FCG) or fermented by-products (FBP) feedstuff. *Indicates significant difference as P < 0.05.
Bulls on the FBP diet had distinct ruminal bacterial communities compared to those on the FCG diet, as samples were clearly separated by PC1 (explaining 47.5% of the variation) (p < 0.05, Figure 2). Feeding the FBP diet enhanced bacterial diversity, as indicated by the greater (p < 0.05) Shannon index and lower Simpson index compared to feeding FCG diet (Figure 3). A total of 16 phyla were identified with Bacteroidetes and Firmicutes being the most abundant (Table 5). Bulls on the FBP diet had a greater (p < 0.05) ruminal relative abundance of Firmicutes, Proteobacteria and Actinobacteriota, and a lower (p < 0.05) abundance of Bacteroidetes compared to those on the FCG diet. In total, 219 genera were identified, with Prevotella being the most abundant (Table 5). The FBP diet resulted in a greater (p < 0.05) relative abundance of Succiniclasticum, Lachnospiraceae_unclassified, Selenomonadaceae_unclassified, Lachnospiraceae_NK3A20_group, Megasphaera, and Prevotellaceae_unclassified, and a lower (p < 0.05) abundance of Rikenellaceae_RC9_gut_group, F082_unclassified, Christensenellaceae_R-7_group and Ruminococcus compared to the FCG diet (Table 5).
Figure 2. PCoA analyses of bacterial communities in the rumen of growing Holstein bulls fed with diets containing fermented corn gluten feed (FCG) or fermented by-products (FBP) feedstuff (p = 0.001, R2 = 0.46).
Figure 3. Alpha diversity indices of bacteria community in the rumen of growing Holstein bulls fed with diets containing fermented corn gluten feed (FCG) or fermented by-products (FBP) feedstuff. *Indicates significant difference as P < 0.05.
Table 5. The relative abundance (>1%) of bacteria in the rumen of growing Holstein bulls fed diets containing fermented corn gluten feed (FCG) or fermented by-products (FBP) feedstuff.
Although replacing FCG with FBP feedstuff had little influence on nutrient intake, it increased the intake and digestibility of EE and starch, and decreased CP and NDF digestibility. The reduction of NDF digestibility could be attributed to the greater inclusion of starch or fat, as these components are known to negatively affect ruminal fibrolytic bacteria and protozoa, thus impairing fiber digestibility (NRC, 2001; Patra, 2013; Bhatt et al., 2011). Furthermore, the heating process of bakery by-products can activate the Maillard reactions of CP, hindering ruminal CP degradability (Purlis, 2010), which results in lower CP digestibility in FBP dietary treatment. These distinct changes in the digestibility of starch, EE, NDF, and CP contributed to the similar total feed digestibility of DM observed between the two dietary treatments.
Methane is a major end product for methanogenesis during the rumen fermentation of ingested carbohydrates (Wang et al., 2015). Carbohydrates with higher starch content degrade more rapidly in the rumen, often resulting in a rapid increase in ruminal dissolved methane concentration (Wang et al., 2016b). However, it is not the case in present study. Lower dissolved methane concentrations were observed despite higher starch intake in the FBP diet, along with a decreased pH value at 2.5 h after the morning feeding. A rapid pH drop below 6.0 can inhibit ruminal methanogenesis, as the activities of methanogens decrease at pH below 6.0 (Van Kessel and Russell, 1996; Qiu et al., 2023). Moreover, the increased EE intake with the FBP diet likely contributed to the reduced ruminal methanogenesis. Bakery by-products are rich in EE with greater percentages of unsaturated fatty acids (Humer et al., 2018), which have been reported to have negative effects on methanogens by adhering to the surface or smearing the cell walls and thus impeding the essential nutrient passage (Henderson, 1973; Prins et al., 1972; Van Nevel and Demeyer, 1996). Therefore, the reduced ruminal methanogenesis could be attributed to the combined effects of lower ruminal pH and higher EE intake after replacing FCG with FBP feedstuff.
Hydrogen is formed during the ruminal fermentation of carbohydrates into VFA, and is primarily utilized by archaeal methanogens to form methane (Janssen, 2010; van Lingen et al., 2016). When methanogen activity is inhibited, hydrogen partial pressure accumulates in the rumen (Van Kessel and Russell, 1996; Wang et al., 2013). However, the dissolved hydrogen concentration remained unchanged despite the inhibition of ruminal methanogenesis in the FBP diet. It could be due to the reduced hydrogen accumulation, which is determined by the balance of hydrogen production and utilization (Wang et al., 2016b). The reduced rumen pH and increased EE intake likely inhibited the growth of protozoa (Janssen, 2010), which are important hydrogen producers in the rumen microbial ecosystem (Muller, 1980; Paul et al., 1990), leading to reduced hydrogen accumulation in the FBP diet.
Starch fermentation favors propionate production over acetate production, while fiber fermentation produces more acetate than propionate (Bannink et al., 2006; Song et al., 2018). Propionate synthesis serves as a hydrogen sink whereas acetate and butyrate production are accompanied by net hydrogen generated (Janssen, 2010; Ungerfeld, 2015; Li et al., 2024). The FBP diet showed higher molar percentages of propionate and lower molar percentages of acetate and butyrate. Increased propionate production indicates reduced hydrogen produced per mole of VFA, as is consistent with the reduced RNH2 observed. The decreased efficiency of hydrogen production aligns with the reduced ruminal methanogenesis in the FBP diet and may partially explain the unchanged dissolved hydrogen concentration.
Furthermore, the introduction of FBP feedstuff into the diet likely alters the fermentation dynamics in the rumen, which in turn could influence microbial populations. Specifically, the high starch content in the FBP diet leads to a decrease in ruminal pH, creating an environment more favorable to starch-degrading bacteria. The shift in microbial community composition could increase populations of Succiniclasticum species, which are involved in the fermentation of starch and production of succinate (Bi et al., 2018; Cancino-Padilla et al., 2021). Additionally, the abundance of Megasphaera, which plays a major role in the production of propionate, is likely to increase (Pitta et al., 2014; Humer et al., 2018).
Such a shift in microbial community could result in a reduction in hydrogen availability for methanogenic archaea, thereby altering hydrogen metabolism in rumen. Prevotella, known for degrading wide ranges of proteins, starch, hemicellulose and pectin to produce VFA and acting as a hydrogen consumer (Mickdam et al., 2017; Betancur-Murillo et al., 2022), was unexpectedly reduced in the FBP diet, possibly due to high levels of fat in the FBP diet (Cremonesi et al., 2018). Further studies are needed to understand the role of Prevotella on ruminal lipid metabolism. Instead, Succiniclasticum and Megasphaera were enriched in the rumen of bulls on the FBP diet. Oil supplementation and elevated feed energy levels have been reported to promote the growth of Succiniclasticum, which converts succinate to propionate, providing an alternative pathway of hydrogen utilization for propionate formation (Bi et al., 2018; Cancino-Padilla et al., 2021). Megasphaera, a major soluble sugar fermenter, utilizes lactate (almost up to 80%) to produce propionate and is therefore boosted by diets high in digestible starch or sugar (Pitta et al., 2014; Humer et al., 2018). Thus, the enrichment of Succiniclasticum and Megasphaera likely promoted hydrogen incorporation for propionate synthesis in the FBP dietary treatment.
Cellulose-degrading bacteria are major producers of acetate with net hydrogen production (Chaucheyras-Durand et al., 2010; Pang et al., 2022). Feeding the FBP diet had lower abundance of fibrolytic bacteria, such as Christensenellaceae_R-7_group, Ruminococcus and NK4A214_group, which was consistent with lower ruminal acetate percentage and RNH2. Biohydrogenating bacteria, such as Lachnospiraceae_unclassified and Lachnospira (Boeckaert et al., 2008), were greatly enriched in FBP dietary treatment, which was consistent with its elevated EE intake with enhancement of net hydrogen incorporation.
Replacing FCG with FBP increases EE and starch digestibility but decreases NDF and CP digestibility, with minimal impact on DM or OM digestibility. The increase in starch and EE intakes after replacing FCG by FBP feedstuff decreases ruminal methanogenesis by shifting rumen fermentation from acetate to propionate production through enriching Succiniclasticum, Megasphaera, Lachnospiraceae_unclassified, and Lachnospira which could reduce the reliance on hydrogen for methane synthesis by methanogenic archaea, and inhibiting Christensenellaceae_R-7_group, Ruminococcus and NK4A214_group. Consequently, FBP feedstuff can serve as an effective alternative to FCG with the additional benefit for inhibiting methanogenesis in the rumen.
The original contributions presented in the study are publicly available. This data can be found here: https://www.ncbi.nlm.nih.gov/bioproject/PRJNA1151939.
All animals involved in the experiment were cared for in accordance with the Animal Care and Use Guidelines of the Animal Care Committee, Institute of Subtropical Agriculture, the Chinese Academy of Sciences, Changsha, China, with all animal experimental procedures approved by the committee (approval number ISA-WM-202101).
XP: Investigation, Methodology, Writing – original draft. WZ: Investigation, Methodology, Writing – original draft. FY: Investigation, Methodology, Writing – original draft. XZ: Conceptualization, Investigation, Methodology, Resources, Writing – review & editing. RW: Data curation, Software, Writing – review & editing. QL: Data curation, Software, Writing – review & editing. XY: Data curation, Investigation, Writing – review & editing. DC: Methodology, Resources, Writing – review & editing. JH: Formal Analysis, Methodology, Software, Writing – review & editing. XS: Formal Analysis, Writing – review & editing. ZT: Conceptualization, Supervision, Writing – review & editing. BL: Conceptualization, Resources, Supervision, Writing – review & editing. MW: Conceptualization, Data curation, Investigation, Project administration, Software, Writing – review & editing.
The author(s) declare that financial support was received for the research, authorship, and/or publication of this article. The work was supported by National Natural Science Foundation of China (Grant No. U22A20512, 32161143028), the National Key Research and Development Program of China (Grant No. 2023YFD1300902), Strategic Priority Research Program of the Chinese Academy of Sciences Grant No. XDA26040203), The science and technology innovation Program of Hunan Province (2022RC3058).
The authors declare that the research was conducted in the absence of any commercial or financial relationships that could be construed as a potential conflict of interest.
All claims expressed in this article are solely those of the authors and do not necessarily represent those of their affiliated organizations, or those of the publisher, the editors and the reviewers. Any product that may be evaluated in this article, or claim that may be made by its manufacturer, is not guaranteed or endorsed by the publisher.
Bannink, A., Kogut, J., Dijkstra, J., France, J., Kebreab, E., van, A. M., et al. (2006). Estimation of the stoichiometry of volatile fatty acid production in the rumen of lactating cows. J. Theor. Biol. 238, 36–51. doi: 10.1016/j.jtbi.2005.05.026
Bernard, J. K., Delost, R. C., Mueller, F. J., Miller, J. K., and Miller, W. M. (1991). Effect of wet or dry corn gluten feed on nutrient digestibility and milk yield and composition. J. Dairy Sci. 74, 3913–3919. doi: 10.3168/jds.S0022-0302(91)78584-6
Betancur-Murillo, C. L., Aguilar-Marín, S. B., and Jovel, J. (2022). Prevotella: a key player in ruminal metabolism. Microorganisms 11:1. doi: 10.3390/microorganisms11010001
Bhatt, R., Soren, N. M., Tripathi, M. K., and Karim, S. A. (2011). Effects of different levels of coconut oil supplementation on performance, digestibility, rumen fermentation and carcass traits of Malpura lambs. Anim. Feed Sci. Technol. 164, 29–37. doi: 10.1016/j.anifeedsci.2010.11.021
Bi, Y., Zeng, S., Zhang, R., Diao, Q., and Tu, Y. (2018). Effects of dietary energy levels on rumen bacterial community composition in Holstein heifers under the same forage to concentrate ratio condition. BMC Microbiol. 18:69. doi: 10.1186/s12866-018-1213-9
Boeckaert, C., Vlaeminck, B., Fievez, V., Maignien, L., Dijkstra, J., and Boon, N. (2008). Accumulation of trans C18:1 fatty acids in the rumen after dietary algal supplementation is associated with changes in the Butyrivibrio community. Appl. Environ. Microbiol. 74, 6923–6930. doi: 10.1128/AEM.01473-08
Bray, J. R., and Curtis, J. T. (1957). An ordination of the upland Forest communities of southern Wisconsin. Ecol. Monogr. 27, 325–349. doi: 10.2307/1942268
Cancino-Padilla, N., Catalan, N., Siu-Ting, K., Creevey, C. J., Huws, S. A., and Romero, J. (2021). Long-term effects of dietary supplementation with olive oil and hydrogenated vegetable oil on the rumen microbiome of dairy cows. Microorganisms 9:1121. doi: 10.3390/microorganisms9061121
Chaucheyras-Durand, F., Masséglia, S., Fonty, G., and Forano, E. (2010). Influence of the composition of the cellulolytic flora on the development of hydrogenotrophic microorganisms, hydrogen utilization, and methane production in the rumens of gnotobiotically reared lambs. Appl. Environ. Microbiol. 76, 7931–7937. doi: 10.1128/AEM.01784-10
Cremonesi, P., Conte, G., Severgnini, M., Turri, F., Monni, A., Capra, E., et al. (2018). Evaluation of the effects of different diets on microbiome diversity and fatty acid composition of rumen liquor in dairy goat. Animal 12, 1856–1866. doi: 10.1017/S1751731117003433
Hegarty, R. S. (1999). Reducing rumen methane emissions through elimination of rumen protozoa. Aust. J. Agric. Res. 50, 1321–1328. doi: 10.1071/AR99008
Henderson, C. (1973). The effects of fatty acids on pure cultures of rumen bacteria. J. Agric. Sci. 81, 107–112. doi: 10.1017/S0021859600058378
Humer, E., Aditya, S., Kaltenegger, A., Klevenhusen, F., Petri, R. M., and Zebeli, Q. (2018). Graded substitution of grains with BP modulates ruminal fermentation, nutrient degradation, and microbial community composition in vitro. J. Dairy Sci. 101, 3085–3098. doi: 10.3168/jds.2017-14051
Janssen, P. H. (2010). Influence of hydrogen on rumen methane formation and fermentation balances through microbial growth kinetics and fermentation thermodynamics. Anim. Feed Sci. Technol. 160, 1–22. doi: 10.1016/j.anifeedsci.2010.07.002
Jiao, Y., Chen, H. D., Han, H., and Chang, Y. (2022). Development and utilization of corn processing by-products: a review. Food Secur. 11:3709. doi: 10.3390/foods11223709
Kaltenegger, A., Humer, E., Stauder, A., and Zebeli, Q. (2020). Feeding of BP in the replacement of grains enhanced milk performance, modulated blood metabolic profile, and lowered the risk of rumen acidosis in dairy cows. J. Dairy Sci. 103, 10122–10135. doi: 10.3168/jds.2020-18425
Karthner, R. J., and Theurer, B. (1981). Comparison of hydrolysis methods used in feed, digesta, and fecal starch analysis. J. Agric. Food Chem. 29, 8–11. doi: 10.1021/jf00103a003
Li, Q., Ma, Z., Huo, J., Zhang, X., Wang, R., Zhang, Z. G., et al. (2024). Ecological niches and assembly dynamics of diverse microbial consortia in the gastrointestine of goat kids. ISME J. 18:wrae002. doi: 10.1093/ismejo/wrae002
Li, Q. S., Wang, R., Ma, Z. Y., Zhang, X. M., Jiao, J. Z., Zhang, Z. G., et al. (2022). Dietary selection of metabolically distinct microorganisms drives hydrogen metabolism in ruminants. ISME J. 16, 2535–2546. doi: 10.1038/s41396-022-01294-9
Mickdam, E., Khiaosa-ard, R., Metzler-Zebeli, B. U., Humer, E., Harder, H., Khol-Parisini, A., et al. (2017). Modulation of ruminal fermentation profile and microbial abundance in cows fed diets treated with lactic acid, without or with inorganic phosphorus supplementation. Anim. Feed Sci. Technol. 230, 1–12. doi: 10.1016/j.anifeedsci.2017.05.017
Muller, M. (1980). “The hydrogenosome” in The eukaryotic microbial cell. eds. G. W. Gooday, D. Lloyd, and A. P. J. Trinci (Cambridge: Cambridge University Press), 127–142.
NRC (2001). Nutrient requirements of dairy cattle. 7th rev. ed. Washington, DC: National Academies Press.
Pang, K., Chai, S., Yang, Y., Wang, X., Liu, S., and Wang, S. (2022). Dietary forage to concentrate ratios impact on yak ruminal microbiota and metabolites. Front. Microbiol. 13:964564. doi: 10.3389/fmicb.2022.964564
Patra, A. K. (2013). The effect of dietary fats on methane emissions, and its other effects on digestibility, rumen fermentation and lactation performance in cattle: a meta-analysis. Livest. Sci. 155, 244–254. doi: 10.1016/j.livsci.2013.05.023
Paul, R. G., Williams, A. G., and Butler, R. D. (1990). Hydrogenosomes in the rumen entodiniomorphid ciliate Polyplastron multivesiculatum. J. Gen. Microbiol. 136, 1981–1989. doi: 10.1099/00221287-136-10-1981
Pitta, D. W., Kumar, S., Vecchiarelli, B., Shirley, D. J., Bittinger, K., Baker, L. D., et al. (2014). Temporal dynamics in the ruminal microbiome of dairy cows during the transition period. J. Anim. Sci. 92, 4014–4022. doi: 10.2527/jas.2014-7621
Prins, R. A., van Nevel, C. J., and Demeyer, D. I. (1972). Pure culture studies of inhibitors for methanogenic bacteria. Antonie Van Leeuwenhoek 38, 281–287. doi: 10.1007/BF02328099
Purlis, E. (2010). Browning development in bakery products-a review. J. Food Eng. 99, 239–249. doi: 10.1016/j.jfoodeng.2010.03.008
Qiu, S., Zhang, X., Xia, W., Li, Z., Wang, L., Chen, Z., et al. (2023). Effect of extreme pH conditions on methanogenesis: methanogen metabolism and community structure. Sci. Total Environ. 877:162702. doi: 10.1016/j.scitotenv.2023.162702
Siverson, A. V., Titgemeyer, E. C., Montgomery, S. P., Oleen, B. E., Preedy, G. W., and Blasi, D. A. (2014). Effects of corn processing and dietary wet corn gluten feed inclusion on performance and digestion of newly received growing cattle. J. Anim. Sci. 92, 1604–1612. doi: 10.2527/jas.2013-6839
Song, S. D., Chen, G. J., Guo, C. H., Rao, K. Q., Gao, Y. H., Peng, Z. L., et al. (2018). Effects of exogenous fibrolytic enzyme supplementation to diets with different NFC/NDF ratios on the growth performance, nutrient digestibility and ruminal fermentation in Chinese domesticated black goats. Anim. Feed Sci. Technol. 236, 170–177. doi: 10.1016/j.anifeedsci.2017.12.008
Ungerfeld, E. M. (2015). Shifts in metabolic hydrogen sinks in the methanogenesis-inhibited ruminal fermentation: Ameta-analysis. Front. Microbiol. 6:37. doi: 10.3389/fmicb.2015.00037
Van Kessel, J. A. S., and Russell, J. B. (1996). The effect of pH on ruminal methanogenesis. FEMS Microbiol. Ecol. 20, 205–210. doi: 10.1016/0168-6496(96)00030-X
van Lingen, H. J., Plugge, C. M., Fadel, J. G., Kebreab, E., Bannink, A., and Dijkstra, J. (2016). Thermodynamic driving force of hydrogen on rumen microbial metabolism: a theoretical investigation. PLoS One 11:e0161362. doi: 10.1371/journal.pone.0161362
Van Nevel, C. J., and Demeyer, D. I. (1996). Control of rumen methanogenesis. Environ. Monit. Assess. 42, 73–97. doi: 10.1007/BF00394043
Van Soest, P. J., Robertson, J. B., and Lewis, B. A. (1991). Methods for dietary fiber, neutral detergent fiber, and nonstarch polysaccharides in relation to animal nutrition. J. Dairy Sci. 74, 3583–3597. doi: 10.3168/jds.S0022-0302(91)78551-2
Wang, R., Bai, Z., Chang, J., Li, Q., Hristov, A. N., Smith, P., et al. (2022). China’s low-emission pathways toward climate-neutral livestock production for animal-derived foods. Innovation 3:100220. doi: 10.1016/j.xinn.2022.100220
Wang, M., Janssen, P. H., Sun, X. Z., Muetzel, S., Tavendale, M., Tan, Z. L., et al. (2013). A mathematical model to describe in vitro kinetics of H2 gas accumulation. Anim. Feed Sci. Technol. 184, 1–16. doi: 10.1016/j.anifeedsci.2013.05.002
Wang, M., Sun, X. Z., Janssen, P. H., Tang, S. X., and Tan, Z. L. (2014). Responses of methane production and fermentation pathways to the increased dissolved hydrogen concentration generated by eight substrates in in vitro ruminal cultures. Anim. Feed Sci. Technol. 194, 1–11. doi: 10.1016/j.anifeedsci.2014.04.012
Wang, M., Wan, D., Xie, X., Bai, Z., Wang, R., Zhang, X., et al. (2024). Crop-livestock integration: implications for food security, resource efficiency and greenhouse gas mitigation. Innovation Life 2:100103. doi: 10.59717/j.xinn-life.2024.100103
Wang, M., Wang, R., Janssen, P. H., Zhang, X. M., Sun, X. Z., Pacheco, D., et al. (2016a). Sampling procedure for the measurement of dissolved hydrogen and volatile fatty acids in the rumen of dairy cows. J. Anim. Sci. 94, 1159–1169. doi: 10.2527/jas.2015-9658
Wang, M., Wang, R., Sun, X. Z., Chen, L., Tang, S. X., Zhou, C., et al. (2015). A mathematical model to describe the diurnal pattern of enteric methane emissions from non-lactating dairy cows post-feeding. Anim. Nutr. 1, 329–338. doi: 10.1016/j.aninu.2015.11.009
Wang, M., Wang, R., Xie, T. Y., Janssen, P. H., Sun, X. Z., Beauchemin, K. A., et al. (2016b). Shifts in rumen fermentation and microbiota are associated with dissolved ruminal hydrogen concentrations in lactating dairy cows fed different types of carbohydrates. J. Nutr. 146, 1714–1721. doi: 10.3945/jn.116.232462
Xu, S., Zhan, L., Tang, W., Wang, Q., Dai, Z., Zhou, L., et al. (2023). MicrobiotaProcess: a comprehensive R package for deep mining microbiome. Innovation (Camb.) 4:100388. doi: 10.1016/j.xinn.2023.100388
Yu, Z., and Morrison, M. (2004). Improved extraction of PCR-quality community DNA from digesta and fecal samples. BioTechniques 36, 808–812. doi: 10.2144/04365ST04
Zhang, X. M., Medrano, R. F., Wang, M., Beauchemin, K. A., Ma, Z. Y., Wang, R., et al. (2019). Corn oil supplementation enhances hydrogen use for biohydrogenation, inhibits methanogenesis, and alters fermentation pathways and the microbial community in the rumen of goats. J. Anim. Sci. 97, 4999–5008. doi: 10.1093/jas/skz352
Keywords: fermented bakery by-products, nutrient digestibility, dissolved methane, rumen fermentation, bacteria
Citation: Pu X, Zhang W, Yang F, Zhang X, Wang R, Li Q, Yang X, Cai D, Huo J, Sun X, Tan Z, Lin B and Wang M (2025) Evaluating the potential of fermented bakery by-products as a replacement for corn gluten feed in cattle diets to suppress methanogenesis and alter rumen fermentation in growing Holstein bulls. Front. Microbiol. 16:1485688. doi: 10.3389/fmicb.2025.1485688
Received: 27 August 2024; Accepted: 03 February 2025;
Published: 20 March 2025.
Edited by:
Grzegorz Bełżecki, Polish Academy of Sciences, PolandReviewed by:
Kang Zhan, Yangzhou University, ChinaCopyright © 2025 Pu, Zhang, Yang, Zhang, Wang, Li, Yang, Cai, Huo, Sun, Tan, Lin and Wang. This is an open-access article distributed under the terms of the Creative Commons Attribution License (CC BY). The use, distribution or reproduction in other forums is permitted, provided the original author(s) and the copyright owner(s) are credited and that the original publication in this journal is cited, in accordance with accepted academic practice. No use, distribution or reproduction is permitted which does not comply with these terms.
*Correspondence: Min Wang, bXdhbmdAaXNhLmFjLmNu
Disclaimer: All claims expressed in this article are solely those of the authors and do not necessarily represent those of their affiliated organizations, or those of the publisher, the editors and the reviewers. Any product that may be evaluated in this article or claim that may be made by its manufacturer is not guaranteed or endorsed by the publisher.
Research integrity at Frontiers
Learn more about the work of our research integrity team to safeguard the quality of each article we publish.