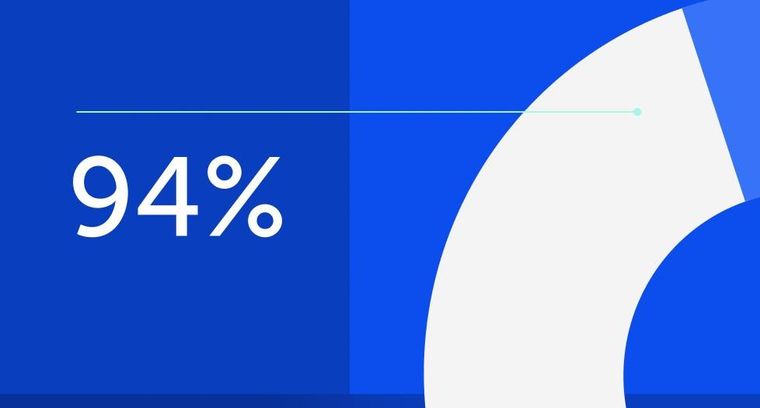
94% of researchers rate our articles as excellent or good
Learn more about the work of our research integrity team to safeguard the quality of each article we publish.
Find out more
MINI REVIEW article
Front. Microbiol., 11 October 2024
Sec. Antimicrobials, Resistance and Chemotherapy
Volume 15 - 2024 | https://doi.org/10.3389/fmicb.2024.1409085
This article is part of the Research TopicOpportunistic pathogens: pathogenesis and multi-drug resistance mechanismsView all 10 articles
Sterols are essential for eukaryotic cells and are crucial in cellular membranes’ structure, function, fluidity, permeability, adaptability to environmental stressors, and host-pathogen interactions. Fungal sterol, such as ergosterol metabolism, involves several organelles, including the mitochondria, lipid droplets, endoplasmic reticulum, and peroxisomes that can be regulated mainly by feedback mechanisms and transcriptionally. The majority of sterol transport in yeast occurs via non-vesicular transport pathways mediated by lipid transfer proteins, which determine the quantity of sterol present in the cell membrane. Pathogenic fungi Candida, Aspergillus, and Cryptococcus species can cause a range of superficial to potentially fatal systemic and invasive infections that are more common in immunocompromised patients. There is a significant risk of morbidity and mortality from these infections, which are very difficult to cure. Several antifungal drugs with different modes of action have received clinical approval to treat fungal infections. Antifungal drugs targeting the ergosterol biosynthesis pathway are well-known for their antifungal activity; however, an imbalance in the regulation and transport of ergosterol could lead to resistance to antifungal therapy. This study summarizes how fungal sterol metabolism and regulation can modulate sterol-targeting antifungal drug resistance.
Invasive fungal infections increased considerably in immunocompromised or critically ill patients, such as HIV-positive patients, cancer patients undergoing chemotherapy, and organ transplant recipients, which poses a significant global threat to human health (Pfaller and Diekema, 2007; Low and Rotstein, 2011; Garnacho-Montero et al., 2024). Worldwide, 150 million immunocompromised individuals suffer from fungal diseases, which claim the lives of about 1.7 million of them annually (Bongomin et al., 2017; Kainz et al., 2020). Usually, Candida, Aspergillus, and Cryptococcus spp., infections account for more than 90% of nosocomial fungal infections, primarily affecting immunocompromised individuals (Brown et al., 2012). Numerous species of Candida can cause invasive candidiasis, a severe infection that can affect the heart, brain, eyes, bones, blood, and other body parts of patients (Pfaller and Diekema, 2007; McCarty and Pappas, 2016; Lass-Flörl et al., 2024). The prevalence of Candida albicans and non-albicans species has grown, particularly in the last two decades (Deorukhkar et al., 2014; Pfaller et al., 2014). This is attributable to a rise in immune-related disorders, the overuse of immunosuppressive medicines, and the prolonged use of medical equipment. The C. albicans mainly cause candidiasis, however, several non-albicans species such as C. parapsilosis, C. glabrata, C. krusei, C. tropicalis, C. dubliniensis, C. lusitaniae also reported from clinical samples of candidiasis patients (Deorukhkar et al., 2014; Hani et al., 2015; Makanjuola et al., 2018).
Several classes of antifungal drugs with different modes of action have been approved for use in clinical settings to treat fungal infections (Fuentefria et al., 2018). These antifungal drugs include azoles, allylamines, morpholines, polyenes, nucleoside analogs, and echinocandins. Azoles (e.g., fluconazole, voriconazole, and isavuconazole) mainly inhibit the ergosterol biosynthesis pathway enzyme lanosterol-14α-demethylase encoded by ERG11 and interrupt the ergosterol biosynthesis (Chen and Sobel, 2005; Allen et al., 2015; Lee et al., 2023). Allylamines (e.g., terbinafine and naftifine) inhibit the squalene epoxidase encoded by ERG1, and morpholine (e.g., amorolfine, fenpropimorph, and tridemorph) inhibits the biosynthesis of sterol by blocking two successive enzymes (a) C-14 sterol reductase (ERG24) and (b) C-8 sterol isomerase (ERG2) (Debieu et al., 2000). Polyene (e.g., amphotericin B, nystatin, and natamycin) interacts with ergosterol in the cell membrane, creating pores and causing cell lysis, while echinocandin (e.g., caspofungin and micafungin) mainly inhibits the enzyme β-1,3-D-glucan synthase encoded by FKS1 (Perlin, 2015; Ahmady et al., 2024). Nucleoside analogs, such as 5′-flucytosine (5-FC), are taken up by cytosine permease, a membrane transporter of fungal cells. Cytosine deaminase then transforms the 5-FC into 5-fluorouracil (5-FU), which is its active form. 5-fluorouracil is metabolized to produce 5-fluorouridine monophosphate (5-FUMP) and 5-fluorodeoxyuridine monophosphate (5-FdUMP), which, respectively, blocks RNA and DNA synthesis (Bhattacharya et al., 2020; Sigera and Denning, 2023). The chemical structure of antifungal drugs that selectively target the ergosterol production pathway of S. cerevisiae is shown in Figures 1A,B.
Figure 1. (a) The chemical structures of various antifungal drug that specifically targets the ergosterol biosynthesis pathway. (b) Schematic representation of ergosterol biosynthesis pathway reported in S. cerevisiae. The production of ergosterol molecules begins with the condensation of acetyl-Co and takes place mainly in the ER, whereas farnesyl-PP biosynthesis occurs in the vacuole. Allylamine inhibitors inhibit squalene epoxidase, which is encoded by ERG1. Azole inhibitors primarily target ERG11, resulting in the generation of hazardous sterols. Morpholines target step catalyzed by ERG24 and ERG2, inhibiting ergosterol production, whereas polyene targets membranes’ ergosterol [Adapted and modified from Onyewu et al., 2003].
The existence of intrinsic, acquired, or clinical resistance poses a significant challenge that limits the potential for the development of novel therapeutics against Candida species (Sanguinetti et al., 2015; Chowdhary et al., 2017; Spivak and Hanson, 2018). The rise in clinical isolates of fungal pathogens that are highly virulent and resistant to drugs poses a severe threat to human health (Taei et al., 2019; McDermott, 2022). Public health worldwide is seriously threatened by the emergence of pan-resistant C. auris clinical isolates (Lockhart, 2019). Therefore, a fundamental comprehension of the molecular pathways that an appropriate drug can target aids in preventing the development of fungal drug resistance.
Ergosterol, a key sterol in fungal membranes, primarily regulates membrane fluidity, membrane-bound enzyme activity, growth, and other cellular processes, which makes them a potentially valuable target for drug development (Iwaki et al., 2008; Rodrigues, 2018). After being produced in the endoplasmic reticulum (ER), ergosterol is transferred to the plasma membrane (PM), which contains an enormous amount of the cell’s free ergosterol pool (Mesmin et al., 2013). Newly produced ergosterol equilibrates with the PM localized sterol pool, with roughly 105 ergosterol molecules entering and exiting the PM per second (Hu et al., 2017). Sterols are insoluble in water, hence equilibration of free/available sterols between organelles needs lipid transfer proteins between membranes. Soluble lipid transfer proteins take a lipid from a donor membrane and deposit it to an acceptor membrane (Wong et al., 2019). Oxysterol-binding protein (OSBP) and OSBP-related proteins (ORPs) are key candidates of the eukaryotic gene family, including ORP in humans and oxysterol-binding homologous (Osh) proteins in yeast that transfer and regulate sterols and phospholipids between organelle membranes (Weber-Boyvat et al., 2013). Although ergosterol production and transport are well understood in model yeast, little is known about how they contribute to antifungal drugs. This study describes the key steps in ergosterol production and transport pathways that lead to antifungal drug resistance.
Ergosterol is mainly synthesized in the ER and transferred to various organelles such as mitochondria, Golgi body, and PM through vesicular and non-vesicular transport mechanisms (Lv et al., 2016; Jordá and Puig, 2020; Zheng Koh and Saheki, 2021). Sterol concentrations are low in the ER but raised in secretory organelles, with the maximum concentration in the PM (Zinser et al., 1993; Hannich et al., 2011). Ergosterol comprises four rings, an acyl side chain, and a hydrophilic hydroxyl group that makes it easy to introduce into membranes. Ergosterol’s structure varies from cholesterol by two additional double bonds (at position C7 and C8) and an extra methyl group (at position C22 and C23) in the side chain (Vanegas et al., 2012).
Sterol biosynthesis in the model yeast Saccharomyces cerevisiae begins with the condensing two acetyl-CoA molecules to produce acetoacetyl-CoA, catalyzed by Erg10p (Liu et al., 2019). Erg13p catalyzes the conversion of a third acetyl-CoA to acetoacetyl-CoA, resulting in 3-hydroxy-3-methylglutarylCoA (HMG-CoA) (Miziorko, 2011). HMG-CoA is then reduced to mevalonate by HMG-CoA reductase encoded by Hmg1p and Hmg2p (Basson et al., 1986). Sterol intermediates block these reductases, making this a critical metabolic checkpoint. Erg12p phosphorylates mevalonate during the subsequent process (Oulmouden and Karst, 1991). Erg8p, a phosphomevalonate kinase, further phosphorylates to produce mevalonate-5-pyrophosphate (Tsay and Robinson, 1991). Next, Erg19p, a mevalonate pyrophosphate decarboxylase, decarboxylates isopentenyl pyrophosphate (IPP) (Berges et al., 1997). IDI1 encodes the IPP isomerase, which converts IPP to dimethylallyl pyrophosphate (DPP). DPP then condenses with another IPP molecule to produce geranyl pyrophosphate, a further addition of IPP results in the formation of farnesyl pyrophosphate (FPP). The geranyl/FPP synthase, Erg20p, catalyzes both processes (Anderson et al., 1989).
Next, squalene synthase (Farnesyl-diphosphate farnesyl transferase) enzyme Erg9 uses two farnesyl-pyrophosphate (farnesyl-PP) molecules to form squalene. The enzymes squalene epoxidase ERG1 and lanosterol synthase ERG7 work in tandem to convert squalene to lanosterol. Lanosterol is converted into 4,4-dimethyl-cholesta 8,14, 24-trienol by lanosterol 14α-demethylase encoded by ERG11 which is further converted into 4,4-dimethyl-zymosterol which is catalyzed by C14 sterol reductase (ERG24). 4,4-dimethyl-zymosterol is further converted into zymosterol by involving enzymes such as ERG25, ERG26, and ERG27. Finally, the enzyme C24-methyltransferase (Erg6p) converts the zymosterol into fecosterol. In the next step, fecosterol is converted into episterol, a reaction catalyzed by the C8 isomerase enzyme ERG2, and in the last step, episterol is transformed into ergosterol through a complex process involving C5-sterol desaturase (ERG3), C22-sterol desaturase (ERG5) and C24-sterol reductase (ERG4) reactions (Shakoury-Elizeh et al., 2010; Kathiravan et al., 2012; Joshua and Höfken, 2017; Ward et al., 2018; Liu et al., 2019; Vil et al., 2019). The sterol-desaturase enzyme, encoded by CaERG3, is known to utilize 14α-methyl-fecosterol in C. albicans. This enzyme catalyzes the conversion of 14α-methyl-fecosterol to 14α-methyl-ergosta-8,24(28)-dienol-3,6-diol, a toxic sterol linked to the antifungal activity of triazoles. Ergosterol biosynthesis in yeast depends on oxygen and iron at multiple steps such as enzymatic reactions catalyzed by ERG1, ERG3, ERG5, ERG11, and ERG25 use molecular oxygen and heme as the electron acceptor (Jordá et al., 2022).
These days, allylamines such as naftifine and terbinafine are a relatively new class of synthetic antifungal medications against filamentous, dimorphic, yeast-like fungi (Hammoudi Halat et al., 2022). Allylamines act as non-competitive inhibitors mainly by interfering with the initial rate-limiting step of ergosterol biosynthesis catalyzed by squalene epoxidase encoded by ERG1 (Petranyi et al., 1984). C. albicans, and C. parapsilosis squalene epoxidase, are susceptible to allylamine, while mammalian liver squalene epoxidase is significantly less sensitive to allylamines (Ryder, 1987; Ryder and Dupont, 1985). Naftifine is a topical fungicidal that is efficient against dermatophytes and is used to treat cutaneous candidiasis. Terbinafine hypersusceptibility was observed in strains overexpressing ERG1, ERG9, and ERG26. Erg9p converts FPP to produce squalene, a substrate for Erg1p. The overexpression of Erg9p or Erg1p may cause FPP or squalene pools to be diverted toward ergosterol biosynthesis rather than other cellular functions, leading to susceptibility in these strains (Bhattacharya et al., 2018). The C. albicans erg2Δ/Δ and erg24Δ/Δ mutants are also susceptible to terbinafine, due to the enhanced fluidity and permeability of their PMs (Luna-Tapia et al., 2015). Low terbinafine susceptibility was observed in oropharyngeal C. albicans isolates from HIV-positive individuals in a prior investigation (Odds, 2009). Another study also revealed that, except for C. lusitaniae, C. parapsilosis, and C. krusei, other Candida spp., isolates were resistant to terbinafine (MIC >32 mg/L) (Tøndervik et al., 2014).
The azole-antifungal is the most extensive and frequently utilized class of antifungal drugs (Jangir et al., 2023). Azole targets the ergosterol biosynthesis leading to the formation of unusual sterols disrupting the fungal cell membrane. Azoles inhibit the enzyme called lanosterol 14α-demethylase, encoded by ERG11 (CYP51), which is a fungal cytochrome P450-(CYP50) family-dependent enzyme that converts lanosterol into 14α-dimethyl-lanosterol in the ergosterol biosynthesis pathway. The inhibition of this enzyme increases lanosterol and 14α-methyl sterol levels while ergosterol levels decrease. This causes the fungal cell membrane’s typical permeability and fluidity to change, which inhibits the growth of fungal cells (Peyton et al., 2015; Zhang et al., 2019). ERG2 deletion or HMG1 overexpression strains showed increased susceptibility when treated with azoles due to lower ergosterol contents (Donald et al., 1997; Bhattacharya et al., 2018). In S. cerevisiae and C. albicans Δerg3 and Δerg6 deletion strains are resistant to fluconazole due to their inability to manufacture toxic dienol, which accumulates in the wild-type cell after azole treatment (Kodedová and Sychrová, 2015). Fluconazole resistance is also observed in ERG3 deletion/missense mutant strains of Cryptococcus neoformans, C. glabrata, C. parapsilosis, and C. albicans (Branco et al., 2017; Sanglard et al., 2003). Mutation in ERG25 leads to sterol intermediate accumulation and decreases fluconazole’s binding affinity (Kodedová and Sychrová, 2015; Cavassin et al., 2021). Overexpression of ERG11 in C. albicans, C. glabrata, C. krusei, and multidrug resistance C. auris showed higher resistance to azoles (He et al., 2015; Feng et al., 2017; Bhattacharya et al., 2019).
The ergosterol production pathway enzymes, mainly C14-sterol reductase (ERG24), and C8-sterol isomerase (ERG2) are targeted by the morpholine class of antifungals e.g., amorolfine, fenpropimorph (FEN) and tridemorph, which leads to the accumulation of abnormal sterols ignosterol (ergosta-8,14 dieno) (Polak, 1988; Lorenz and Parks, 1992; Parks and Casey, 1995). The observation of FEN resistance in strains overexpressing ERG24 supports that Erg24p is the principal morpholine drug target (Henry et al., 2000). In addition, FEN resistance was observed in disruption mutants of ERG4. FEN hypersusceptibility was detected in the strains that overexpressed NCP1, ERG1, and HMG1 or in Δerg3 and Δerg6 deletion strains (Bhattacharya et al., 2018). It was discovered that C. albicans sensitivity to the morpholines can be decreased by overexpressing the ERG2 or ERG24 genes (Luna-Tapia et al., 2015). The erg2Δ deletion mutant of C. albicans was sensitive to FEN, whereas the erg24Δ deletion mutant was hypersensitive to amorolfine, FEN, and tridemorph. Mutation in the open reading frame of gene FEN2 from S. cerevisiae that encodes a PM H+-pantothenate symporter was shown to be FEN resistance (Stolz and Sauer, 1999). These findings support the theory that the morpholine’s antifungal action depends on the simultaneous inhibition of Erg2p and Erg24p.
Polyene class includes amphotericin B (AmB), nystatin, natamycin, and filipins that target PM ergosterol and create a pore in the membrane (Madaan and Bari, 2023). Changes in ergosterol content or the substitution of sterol intermediates are the causes of polyene resistance (Ahmady et al., 2024). Previous research concluded that C. lusitaniae may become resistant to AmB due to mutations in or changes in the expression of ergosterol biosynthesis genes (Young et al., 2003). Elevated ERG6 transcript levels and decreased ergosterol content were observed in C. lusitaniae resistant to AmB, indicating mutations or dysregulation in the ergosterol biosynthesis pathway (Bhattacharya et al., 2018). In clinical isolates of C. glabrata, ERG6, and ERG2 are important targets associated with reduced susceptibility to AmB (Ahmad et al., 2019). AmB-resistant isolates also showed lower expression of the ERG3 gene, which codes for C5 sterol desaturase, suggesting a possible involvement of ERG3 in the clinical emergence of AmB resistance (Young et al., 2003). C. neoformans strains with sterol compositions corresponding to ERG2 deletion mutant of S. cerevisiae are resistant to AmB (Kelly et al., 1994). A previous study in S. cerevisiae reported that, as compared to the parental strain, the mutant lacking ERG4 was slightly more sensitive to nystatin; moreover, the deletion of ERG2, ERG6, and, to a lesser extent, ERG3, also conferred resistance to this polyene, most likely because of the decreased drug binding affinity for the accumulated fecosterol, zymosterol, and episterol in these mutants (Kodedová and Sychrová, 2015). Natamycin and nystatin-induced loss of inhibition was demonstrated by the loss of double bonds in the B-ring of ergosterol produced by deletions of ERG3 (5,6 position) and particularly ERG2 (7,8 position) (te Welscher et al., 2010).
mRNA expression levels of ERG3 and ERG6 were decreased but increased for ERG11 in AmB-resistant isolates of C. parapsilosis (Lotfali et al., 2017). The loss of function of ERG5 (C22 sterol desaturase) or substitution in ERG11 has been associated with AmB resistance in C. albicans (Martel et al., 2010; Vincent et al., 2013). Inactivation of ERG2 (C8 sterol isomerase) and ERG6 (C24 sterol methyl-transferase) was reported to have a similar impact on C. glabrata (Ahmad et al., 2019). One of the only mechanisms of AmB resistance in C. neoformans that has been described involves a mutation that renders ERG2 inactivated (Kelly et al., 1994). Altered sterol profile due to mutations in several ergosterol biosynthetic pathways genes ERG11, ERG3, ERG2, and ERG6 also cause AmB resistance in Candida species (Vandeputte et al., 2008; Vincent et al., 2013; Carolus et al., 2021; Rybak et al., 2022). Table 1 lists the genes related to ergosterol metabolism and transport pathways that are implicated in resistance to antifungal drugs.
Table 1. A list of ergosterol metabolic and transport pathways genes involved in antifungal drug resistance.
Ergosterol biosynthesis and degradation must be balanced and regulated to prevent the buildup of free sterols, which can be harmful to cells. Yeast cells have evolved distinct regulatory systems that carefully control the ergosterol composition of lipids. Feedback regulation of ergosterol biosynthesis at the biosynthetic level and transcriptional regulation is responsible for regulating the amount of ergosterol (Jordá and Puig, 2020). Furthermore, the ergosterol pathway enzymes exhibit differential localization. For example, ERG1 localizes exclusively to the ER to enhance ergosterol synthesis; ERG6 localizes to the ER, mitochondria, and cytoplasm; however, ERG1 and ERG6 also localize in a lipid particle (Leber et al., 1998; Shakoury-Elizeh et al., 2010).
Several enzymes involved in the process of ergosterol biosynthesis work together to control the level of ergosterol produced. Squalene, epoxy squalene, and polyepoxyl squalene, for instance, increase when ERG27 is inhibited, but not lanosterol, which is identical to that in the erg7∆ mutant and suggests that ERG7 and ERG27 interact genetically (Teske et al., 2008). Subsequent research demonstrated that ERG27 can interact with ERG7 and facilitate a relationship between ERG7 and lipid particles to inhibit ERG7 degradation; additionally, ERG27 regulates ERG7 activity in lipid particles (Layer et al., 2013). Similarly, double deletion mutants of ERG24 and ERG4 cannot grow in either nutrient-rich medium YEPD or a synthetic complete medium in the presence of calcium. This phenomenon is also observed when ERG24 is altered with three additional genes, namely ERG3, ERG5, and ERG6 (Luna-Tapia et al., 2015). Furthermore, the expression of ergosterol synthesis is also regulated by the intracellular transportation of ergosterol.
Two endoplasmic reticulum-localized acyl-coenzyme A: sterol acyltransferases, ARE1 and ARE2, which are significantly implicated in sterol esterification, are encoded by S. cerevisiae (Yang et al., 1996). Under normal growth conditions, Are2p esterifies the final product, while Are1p primarily esterifies intermediates in sterol biosynthesis (Valachovic et al., 2001). In S. cerevisiae, neutral lipids are generated by four enzymes: Are1p and Are2p, which generate stearyl esters; and Lro1p and Dga1p, which generate triacylglycerol and are stored as lipid droplets (Jacquier et al., 2011). ARE genes are differently regulated in response to variations in sterol metabolism. The major isoform of the enzyme in a wild-type cell developing aerobically is Are2p. The accumulation of ergosterol pathway intermediates or heme deficiency causes the ARE1 gene to be up-regulated, while ARE2 is repressed under heme deficiency. This suggests that the controlled removal of intermediates in the biosynthesis process before they become toxic or contribute to accumulation in the final product is a novel form of sterol homeostasis (Jensen-Pergakes et al., 2001). Despite altered sterol composition, in an ARE1, ARE2 double mutant, stearyl esters (SE) biosynthesis is blocked without any growth defects (Ploier et al., 2015). The double mutant exhibits an increase in free sterols and a decrease in total sterol biosynthesis, suggesting that the formation of SE can also regulate sterol biosynthesis (Ploier et al., 2015). Optimizing culture conditions and metabolic pathway engineering are the two primary techniques for increasing ergosterol productivity since ergosterol biosynthesis is controlled by genes that regulate the biosynthesis and environmental factors (Náhlík et al., 2017). For example, ergosterol biosynthesis can be markedly increased by overexpressing sterol biosynthesis genes (e.g., ERG1, ERG4, EGR9, and ERG11) or ARE2. Thus, ergosterol biosynthesis regulation is a complicated process influenced by various factors.
Another important metabolic checkpoint for the biosynthesis of ergosterol is the synthesis of HMG-CoA, which is catalyzed by HMG-CoA reductase (HMGR) (Burg and Espenshade, 2011). Excessive sterols in S. cerevisiae can cause HMGR (Hmg2) to be degraded via the ER-related degradation (ERAD) pathway, which lowers mevalonate synthesis and down-regulates sterol production (Espenshade and Hughes, 2007). The ERAD process primarily initiates HMGR degradation with the help of membrane-spanning ubiquitin-protein ligase Hrd1, ubiquitin-conjugating enzyme Ubc7, and the chaperone proteins NSG1 and NSG2 (Hampton and Bhakta, 1997; Burg and Espenshade, 2011; Theesfeld and Hampton, 2013; Figure 2A). ERG1 is also degraded by the ERAD pathway via ubiquitin ligase Doa10 when lanosterol concentration increases to prevent the accumulation of toxic sterol intermediates (Foresti et al., 2013; Huang and Chen, 2023). As a result, ERAD is crucial for preserving cellular sterol homeostasis.
Figure 2. (a) Ergosterol biosynthetic pathway regulatory mechanism contributes to antifungal drug resistance. Overabundance of ergosterols may cause HMG-CoA reductase (HMGR) to be degraded via the proteasome, reducing mevalonate synthesis and down-regulating ergosterol biosynthesis. The ER-related degradation (ERAD) pathway mediates the proteasome recognition process of HMGR. The ERAD process primarily initiates HMGR breakdown by HRD1 and the chaperone proteins NSG1 and NSG2 recognizing sterols (Omelchuk et al., 2018). (1) In excessive sterol conditions, the ergosterol pathway, specifically transcription factor UPC2, binds with ergosterol and Hsp90 and stays in the cytosol as a repressed state. (2) Under low ergosterol, dissociation of ergosterol leads to the relocalization of Upc2 from cytosol to the nucleus by nuclear transport proteins such as importins α. transcription factors UPC2 can bind to the SRE of the sterol biosynthesis genes to promote their expression or Activated Upc2 also triggers the expression of the Adr1 transcription factor, which further serves to direct the expression of ergosterol biosynthesis genes. Activation of Upc2 or Adr1-enhanced azole, AmB, and terbinafine resistance in Candida (Shrivastava et al., 2023). (3) Three members of the heme-binding damage resistance proteins (Dap) family—DapA, DapB, and DapC in A. fumigatus modulate cytochrome P450 enzymes Erg5 and Erg11 in a coordinated manner and influence azole susceptibility (Song et al., 2016). (b) Overview of oxysterol binding protein-mediated sterol transport in S. cerevisiae. (1) Osh1 and Osh4p acts as a sterol-PI4P exchanger where it acquires the sterol from the donor membrane (ER) and exchange it for a PI4P at the acceptor membrane (trans-Golgi) and then carries the PI4P back to the donor membrane, completing the exchange cycle (Mochizuki et al., 2022). Sec14 protein is involved in the transport of PI from ER to Golgi. PI4P is converted into PI at the ER by Sac1p, and PI is phosphorylated into PI4P at the trans-Golgi by Pik1p (De Saint-Jean et al., 2011). (2) OSH2 and OSH3 contain the pleckstrin homology (PH) domain in the N-terminal region, the OSBP-related ligand binding domain (ORD) in the C-terminal region, and the (FFAT) motif. These play a part in the counter-transport of ergosterol and PI4P from the ER to the PM and from the PM to the ER, respectively. PI is phosphorylated into PI4P at the PM by Stt1. (3) Lam1p, Lam2p, Lam3p, and Lam4p is involved in retrograde transport of sterol. (4) LAM5 and LAM6 are involved in retrograde transport from ER to mitochondria (Elbaz-Alon et al., 2015; Gatta et al., 2015).
Most fungi including fission yeast Schizosaccharomyces pombe and opportunistic pathogen C. neoformans contain a homolog of the mammalian sterol regulatory element binding protein (SREBP) known as Sre1, while in Aspergillus fumigatus called SrbA (Brown and Goldstein, 1997; Willger et al., 2008; Chang et al., 2009). SREBP-like proteins are activated upon cleavage by SREBP activating protein (SCAP) known as Scp1, that is absent in A. fumigatus (Willger et al., 2008). Sre1 localizes to the ER and regulates sterol-specific gene expression (Gómez et al., 2020). Under low sterol conditions, Sre1 gets cleaved and enters the nucleus, binds to sterol regulatory elements (SREs), and increases the expression of sterol-synthesizing genes (Hughes et al., 2005). In addition, hypoxic conditions also induce cleavage of Sre1 and lead to the expression of oxygen-dependent enzymes in the ergosterol biosynthesis pathway including Erg3 and Erg25 (Bien and Espenshade, 2010; Tong et al., 2018). Nevertheless, ergosterol production and absorption are regulated differently depending on the kind of yeast. Fission yeast without Sre1 and Scp1 cannot grow in anaerobic conditions as they cannot manufacture ergosterol at low oxygen levels (Hughes et al., 2005; Stewart et al., 2011; Chong and Espenshade, 2013). Similarly, budding yeast does not take up exogenous sterol, under aerobic or normal growth conditions. But in hypoxic/anaerobic environments, budding yeast does take up exogenous sterol; in fact, sterol absorption is critical to the survivability of budding yeast during anaerobic growth when sterol production is restricted by low oxygen supply (da Costa et al., 2018). Under aerobic circumstances or in the presence of azoles, C. glabrata can also absorb cholesterol (Nakayama et al., 2007; Nagi et al., 2013). Moreover, C. glabrata can import cholesterol and use it instead of ergosterol when vital genes ERG1, ERG7, or ERG11 are knocked down, but this is not the case when ERG25 and ERG26 are knocked down (Okamoto et al., 2022).
While budding yeast lacks SREBP homologs, it does have a unique sterol regulatory mechanism that controls ergosterol production. This mechanism involves Upc2 and its paralog Ecm22, both are transcription factors specific to the fungal family able to bind with sterol regulatory elements (SRE) through their amino-terminal Zn2Cys6 DNA binding domain (Vik and Rine, 2001; Yang et al., 2015). Lethality results from the deletion of Ecm22 and Upc2, indicating that both proteins are crucial for controlling sterol metabolism in budding yeast (Shianna et al., 2001). Upc2 has a hydrophobic pocket in its C-terminal domain that binds to sterol and controls the protein’s transition between the cytosol and nucleus (Vik and Rine, 2001; Marie et al., 2008). Under normal conditions, ergosterol binds with the UPC2 carboxy-terminal domain causing repression of the UPC2 transcription factor (Shianna et al., 2001). Under ergosterol depletion or hypoxic conditions, ergosterol ligand dissociation causes conformational changes and the Upc2 transcription factor translocated to the nucleus and activates SRE-containing genes including ergosterol biosynthesis genes, sterol uptake genes (AVS1, PDR11), and DAN1/TIR mannoprotein genes during the anaerobic remodeling of the cell wall (Abramova et al., 2001; Figure 2A).
Dimerization of UPC2 essential for regulatory function, gain of function mutation in UPC2 leads to azole resistance while deletion of UPC2 in C. albicans sensitizes them toward azoles (Whaley et al., 2014; Yang et al., 2015). According to previous research, the transcription factors ECM22 and UPC2, the SRE region of the sterol biosynthesis genes enhance their expression under hypoxic conditions (Davies and Rine, 2006; Woods and Höfken, 2016). AUS1 and PDR11, two ATP-binding transporters, can also be expressed in response to UPC2, which promotes yeast to absorb sterols from its surroundings. Ergosterol production is further impacted by additional environmental variables such as oxidation, ethanol stimulation, and iron availability (Barchiesi et al., 2005).
Sterol transport between organelles and release into the medium rely on vesicular or nonvesicular transport pathway (Jacquier and Schneiter, 2012). Under aerobic conditions, yeast does not incorporate exogenous sterols, however, under hypoxia/anaerobic conditions ability to synthesize sterol is decreased which is compensated by the import of sterol from the medium through nonvesicular intracellular trafficking (Lev, 2010). Newly produced lipids are transported non-selectively from the ER to the PM via secretory vesicle flux (Wong et al., 2019). Lipid transfer proteins and sterol binding proteins are two evolutionarily conserved families of proteins that mediate intracellular sterol distribution (Lin et al., 2023). All eukaryotes have the evolutionarily conserved lipid transport proteins (LTPs) known as Oxysterol-binding protein Homology [Osh] in yeast, and Oxysterol-binding Protein [OSBP] and OSBP-Related Protein [ORP] in mammals (Schulz and Prinz, 2007; Lev, 2010; Ngo et al., 2010). The primary biological functions of OSBP and ORP include signaling, vesicular trafficking, lipid metabolism, and non-vesicular transport (Raychaudhuri and Prinz, 2010; Jackson et al., 2016). It has been demonstrated that these proteins can bind and transport different lipids, such as phosphoinositides (PIPs), and sterols (Ngo et al., 2010; Delfosse et al., 2020; Lin et al., 2023; Nakatsu and Kawasaki, 2021).
There is evidence supporting the function of a cytoplasmic nonvesicular protein sterol transporter, and the structure of an oxysterol-binding protein homolog (OSH) in yeast (Osh4p/Kes1p) has been solved, without a ligand and in complexes with many oxysterols, cholesterol, and ergosterol, identifying it as a sterol-binding protein (Schulz and Prinz, 2007). A seven-member oxysterol-binding protein family (Osh1-7) in S. cerevisiae performs redundant, overlapping roles in sterol metabolism collectively necessary for maintaining intracellular sterol distribution and homeostasis. All seven proteins are demonstrated to have the highest homology within the restricted region of 150–200 amino acid residues that make up OSBP-related domains (ORD) involved in oxysterol binding and intracellular sterol distribution is significantly changed in mutants lacking any of these proteins, which is consistent with an involvement of Osh proteins in intracellular sterol transport (Beh et al., 2001; Beh and Rine, 2004; Schulz and Prinz, 2007).
All these proteins may have a hydrophobic binding tunnel that is important for interaction with sterol. PH domain located at N- the terminal of Osh1p, Osh2p, and Osh3p proteins may control protein targeting to membranes and function as membrane adaptors by interacting with phospholipids (Powis et al., 2023). Osh1p demonstrated a remarkable dual localization at the Golgi and nucleus-vacuole (NV) junction (Levine and Munro, 2001). According to the deletion mapping of Osh1p, the PH domain is shown to be targeting the Golgi while the ankyrin repeat targets the NV junction (Kvam and Goldfarb, 2004). Osh2p is present in the PM, primarily found in the budding region of G1 phase cells around the mother-daughter bud neck of S-phase cells and in the scattered cytoplasmic pool (Levine and Munro, 2001). Osh3p is distributed throughout the cytoplasm and Osh4p is localized into the Golgi membrane (Li et al., 2002). Osh4p binds to phosphatidyl inositol 4-phosphate (PI4P) and its conserved OSBP domain is crucial for Osh4p localization to the Golgi membrane (Kyte and Doolittle, 1982; Rogaski et al., 2010). Osh5 protein is involved in the regulation of ergosterol biosynthesis and facilitates the transfer of phosphatidylserine (PtdSer) to autophagosome membranes (Muramoto et al., 2024), while Osh6p and Osh7p, located in the membrane contact site between the ER and PM, preferentially transporting PtdSer from the ER to PM (Maeda et al., 2013).
Primarily all Osh proteins contain a conserved OSBP-related domain (ORD) made up of an N-terminal lid and a β-barrel core, which transports lipids including sterol and phospholipids between membranes. Budding yeast mutants that lack all seven Osh (Osh1-7) proteins are not viable; nevertheless, they can become viable again if they express one of the Osh proteins. Moreover, abrupt Osh protein depletion causes a growth arrest and a massive buildup of sterol in cells (Beh and Rine, 2004). This suggests that Osh proteins perform roles in maintaining cell viability, probably by supporting the distribution of sterol throughout the cell.
Specific Osh proteins mediate the directional transport of sterol between two distinct membrane compartments by exchanging PI4P with sterol. Osh4 and other Osh proteins, including Osh3 and Osh5, mediated sterol transport from the PM to the ER. Osh4 localizes to the Golgi and is involved in controlling the amount of PI4P present in this organelle. Osh4 mutually exclusively binds Sterol and PI4P, and Osh4 counter-transports sterol between artificial membranes in vitro in return for PI4P (Rogaski et al., 2010). Previously, it was demonstrated that Osh4p plays a crucial role in maintaining the proper distribution of PI4P in yeast, a function that requires the cooperation of the oxysterol-binding proteins Osh1–Osh7 (LeBlanc and McMaster, 2010; Ling et al., 2014; Figure 2B). Two main PI 4-kinases in budding yeast oversee PI4P production at Golgi and PM. While Stt4 operates at the PM, Pik1 is a lipid kinase in the Golgi apparatus (Audhya et al., 2000). C. albicans contains four Osh proteins (Osh2-4 and Osh7), with Osh4 and Osh7 sharing approximately 60% similarity with their S. cerevisiae counterparts.
A novel evolutionarily conserved family of LTPs, known as Lam proteins belonging to the steroidogenic acute regulatory protein-related lipid transfer (StART) family was discovered in yeast (Gatta et al., 2015). These StART family proteins contain one or two StART-like domains that are conserved in eukaryotes and involved in transporting sterol between intracellular membranes. Six yeast proteins Ysp1/Lam1, Ysp2/Lam2, Sip3/Lam3, Lam4, Lam5, and Lam6 make up this family in budding yeast exhibit a C-terminal transmembrane region that attaches them to the membrane, and N-terminal StART-like domains, and other pleckstrin-homology (PH) superfamily domain (Gatta et al., 2015; Murley et al., 2015). The budding yeast that lacks Ysp1, Ysp2, or Sip3 exhibits reduced sterol trafficking from the PM to the ER and increased susceptibility to AmB and is rescued by multicopy expression of sterol-binding StART domains (Gatta et al., 2015). This suggests a persistent build-up of PM ergosterol in these yeast mutants. These experiments demonstrate that the Lam proteins help to maintain PM sterol homeostasis in yeast by transporting sterol from the PM to the ER (Figure 2B).
Finding inhibitors that target the ergosterol pathways in the fungus and can precisely block them without hurting the host is a significant challenge in the research of antifungals. The extensive use of antifungal drugs to treat fungal disease has led to the emergence of multidrug-resistant clinical isolates. Ergosterol alterations in multidrug resistance isolates can be understood by utilizing high throughput approaches, such as metabolomics of clinical isolate, to analyze changes in metabolic pathways and processes that lead to multi-drug resistance. Despite substantial progress in this area, little is known about the relationship between ergosterol transport control and antifungal drug resistance. Enzymes involved in ergosterol biosynthesis, regulation, and transport are necessary for pathogenic fungi to thrive inside their host species. These enzymes also play a crucial role in the virulence of pathogenic fungi. Thus, the pharmaceutical disruption of the ergosterol biosynthesis and transport would impair their ability to respond appropriately to the environmental stress that host cells experience, restricting the proliferation and pathogenicity of pathogenic fungi. Aspergillosis, candidiasis, and cryptococcosis are severe invasive mycoses that have a high mortality rate in immunocompromised patients. Few antifungal drugs are available to treat such invasive infections, and fungus resistance is increasing quickly. Since fungal ergosterol differs structurally from their mammalian counterparts, the ergosterol biosynthesis and transport pathway provides an opportunity to discover novel antifungal drugs. This review improves our understanding of the synthesis, transport, and regulation of ergosterol, which will aid in creating new inhibitors that specifically target ergosterol metabolism.
ST: Writing – original draft. SK: Formal analysis, Writing – review & editing. VB: Writing – review & editing, Supervision, Project administration.
The author(s) declare that financial support was received for the research, authorship, and/or publication of this article. This study acknowledges the support from the Science and Engineering Research Board (SERB), New Delhi (Award number SRG/2020/000171/LS) awarded to VKB. The funding agencies had no role in the preparation of the manuscript or in the decision to submit the article for publication.
The authors declare that the research was conducted in the absence of any commercial or financial relationships that could be construed as a potential conflict of interest.
All claims expressed in this article are solely those of the authors and do not necessarily represent those of their affiliated organizations, or those of the publisher, the editors and the reviewers. Any product that may be evaluated in this article, or claim that may be made by its manufacturer, is not guaranteed or endorsed by the publisher.
Abramova, N. E., Cohen, B. D., Sertil, O., Kapoor, R., Davies, K. J. A., and Lowry, C. V. (2001). Regulatory mechanisms controlling expression of the DAN/TIR mannoprotein genes during anaerobic remodeling of the cell wall in Saccharomyces cerevisiae. Genetics 157, 1169–1177. doi: 10.1093/genetics/157.3.1169
Ahmad, S., Joseph, L., Parker, J. E., Asadzadeh, M., Kelly, S. L., Meis, J. F., et al. (2019). ERG6 and ERG2 are major targets conferring reduced susceptibility to amphotericin B in clinical Candida glabrata isolates in Kuwait. Antimicrob. Agents Chemother. 63:18. doi: 10.1128/AAC.01900-18
Ahmady, L., Gothwal, M., Mukkoli, M. M., and Bari, V. K. (2024). Antifungal drug resistance in Candida: a special emphasis on amphotericin B. APMIS 132, 291–316. doi: 10.1111/apm.13389
Allen, D., Wilson, D., Drew, R., and Perfect, J. (2015). Azole antifungals: 35 years of invasive fungal infection management. Expert Rev. Anti-Infect. Ther. 13, 787–798. doi: 10.1586/14787210.2015.1032939
Anderson, M. S., Yarger, J. G., Burck, C. L., and Poulter, C. D. (1989). Farnesyl diphosphate synthetase. Molecular cloning, sequence, and expression of an essential gene from Saccharomyces cerevisiae. J. Biol. Chem. 264, 19176–19184. doi: 10.1016/s0021-9258(19)47284-0
Anderson, J. B., Sirjusingh, C., Parsons, A. B., Boone, C., Wickens, C., Cowen, L. E., et al. (2003). Mode of selection and experimental evolution of antifungal drug resistance in Saccharomyces cerevisiae. Genetics 163, 1287–98. doi: 10.1093/genetics/163.4.1287
Audhya, A., Foti, M., and Emr, S. D. (2000). Distinct roles for the yeast phosphatidylinositol 4-kinases, Stt4p and Pik1p, in secretion, cell growth, and organelle membrane dynamics. Mol Bio Cell 11, 2673–2689. doi: 10.1091/mbc.11.8.2673
Barchiesi, F., Spreghini, E., Tomassetti, S., Arzeni, D., Giannini, D., and Scalise, G. (2005). Comparison of the fungicidal activities of caspofungin and amphotericin B against Candida glabrata. Antimicrob. Agents Chemother. 49, 4989–4992. doi: 10.1128/AAC.49.12.4989-4992.2005
Basson, M. E., Thorsness, M., and Rine, J. (1986). Saccharomyces cerevisiae contains two functional genes encoding 3-hydroxy-3-methylglutaryl-coenzyme a reductase. Proc. Natl. Acad. Sci. USA 83, 5563–5567. doi: 10.1073/pnas.83.15.5563
Beh, C. T., Cool, L., Phillips, J., and Rine, J. (2001). Overlapping functions of the yeast oxysterol-binding protein homologues. Genetics 157, 1117–1140. doi: 10.1093/genetics/157.3.1117
Beh, C. T., and Rine, J. (2004). A role for yeast oxysterol-binding protein homologs in endocytosis and in the maintenance of intracellular sterol-lipid distribution. J. Cell Sci. 117, 2983–2996. doi: 10.1242/jcs.01157
Berges, T., Guyonnet, D., and Karst, F. (1997). The Saccharomyces cerevisiae mevalonate diphosphate decarboxylase is essential for viability, and a single Leu-to-Pro mutation in a conserved sequence leads to thermosensitivity. J. Bacteriol. 179, 4664–4670. doi: 10.1128/jb.179.15.4664-4670.1997
Bhattacharya, S., Esquivel, B. D., and White, T. C. (2018). Overexpression or deletion of Ergosterol biosynthesis genes alters doubling time, response to stress agents, and drug susceptibility in Saccharomyces cerevisiae. mBio 9, e01291–e01218. doi: 10.1128/mBio.01291-18
Bhattacharya, S., Holowka, T., Orner, E. P., and Fries, B. C. (2019). Gene duplication associated with increased fluconazole tolerance in Candida auris cells of advanced generational age. Sci. Rep. 9:5052. doi: 10.1038/s41598-019-41513-6
Bhattacharya, S., Sae-Tia, S., and Fries, B. C. (2020). Candidiasis and mechanisms of antifungal resistance. Antibiotics 9:312. doi: 10.3390/antibiotics9060312
Bien, C. M., and Espenshade, P. J. (2010). Sterol regulatory element binding proteins in fungi: hypoxic transcription factors linked to pathogenesis. Eukaryot. Cell 9, 352–359. doi: 10.1128/EC.00358-09
Bojsen, R., Regenberg, B., Gresham, D., and Folkesson, A. (2016). A common mechanism involving the TORC1 pathway can lead to amphotericin B-persistence in biofilm and planktonic Saccharomyces cerevisiae populations. Sci. Rep. 21874. doi: 10.1038/srep21874
Bongomin, F., Gago, S., Oladele, R. O., and Denning, D. W. (2017). Global and multi-national prevalence of fungal diseases—estimate precision. J Fungi (Basel) 3:57. doi: 10.3390/jof3040057
Branco, J., Fonseca, E., Gomes, N. C., Martins-Cruz, C., Silva, A. P., Silva-Dias, A., et al. (2017). Impact of ERG3 mutations and expression of ergosterol genes controlled by UPC2 and NDT80 in Candida parapsilosis azole resistance. Clin. Microbiol. Infect. 23, 575.e1–575.e8. doi: 10.1016/j.cmi.2017.02.002
Brown, G. D., Denning, D. W., Gow, N. A., Levitz, S. M., Netea, M. G., and White, T. C. (2012). Hidden killers: human fungal infections. Sci. Transl. Med. 4:165rv13. doi: 10.1126/scitranslmed.3004404
Brown, M. S., and Goldstein, J. L. (1997). The SREBP pathway: regulation of cholesterol metabolism by proteolysis of a membrane-bound transcription factor. Cell 89, 331–340. doi: 10.1016/S0092-8674(00)80213-5
Bühler, N., Hagiwara, D., and Takeshita, N. (2015). Functional analysis of sterol transporter orthologues in the filamentous fungus Aspergillus nidulans. Eukaryot. Cell 14, 908–921. doi: 10.1128/EC.00027-15
Burg, J. S., and Espenshade, P. J. (2011). Regulation of HMG-CoA reductase in mammals and yeast. Prog. Lipid Res. 50, 403–410. doi: 10.1016/j.plipres.2011.07.002
Carolus, H., Pierson, S., Muñoz, J. F., Subotić, A., Cruz, R. B., Cuomo, C. A., et al. (2021). Genome-wide analysis of experimentally evolved Candida auris reveals multiple novel mechanisms of multidrug resistance. MBio 12:333. doi: 10.1128/mBio.03333-20
Cavassin, F. B., Baú-Carneiro, J. L., Vilas-Boas, R. R., and Queiroz-Telles, F. (2021). Sixty years of amphotericin B: an overview of the Main antifungal agent used to treat invasive fungal infections. Infect. Dis. Ther. 10, 115–147. doi: 10.1007/s40121-020-00382-7
Chang, Y. C., Ingavale, S. S., Bien, C., Espenshade, P., and Kwon-Chung, K. J. (2009). Conservation of the sterol regulatory element-binding protein pathway and its pathobiological importance in Cryptococcus neoformans. Eukaryot. Cell 8, 1770–1779. doi: 10.1128/EC.00207-09
Chen, A., and Sobel, J. D. (2005). Emerging azole antifungals. Expert Opin. Emerg. Drugs 10, 21–33. doi: 10.1517/14728214.10.1.21
Chong, R., and Espenshade, P. J. (2013). Structural requirements for sterol regulatory element-binding protein (SREBP) cleavage in fission yeast. J. Biol. Chem. 288, 20351–20360. doi: 10.1074/jbc.M113.482224
Chowdhary, A., Sharma, C., and Meis, J. F. (2017). Candida auris: a rapidly emerging cause of hospital-acquired multidrug-resistant fungal infections globally. PLoS Pathog. 13:e1006290. doi: 10.1371/journal.ppat.1006290
Choy, H. L., Gaylord, E. A., and Doering, T. L. (2003). Ergosterol distribution controls surface structure formation and fungal pathogenicity. mBio 14:e0135323. doi: 10.1128/mbio.01353-23
da Costa, B. L. V., Basso, T. O., Raghavendran, V., and Gombert, A. K. (2018). Anaerobiosis revisited: growth of Saccharomyces cerevisiae under extremely low oxygen availability. Appl. Microbiol. Biotechnol. 102, 2101–2116. doi: 10.1007/s00253-017-8732-4
Davies, B. S. J., and Rine, J. (2006). A role for sterol levels in oxygen sensing in Saccharomyces cerevisiae. Genetics 174, 191–201. doi: 10.1534/genetics.106.059964
De Saint-Jean, M., Delfosse, V., Douguet, D., Chicanne, G., Payrastre, B., Bourguet, W., et al. (2011). Osh4p exchanges sterols for phosphatidylinositol 4-phosphate between lipid bilayers. J. Cell Biol. 195, 965–978. doi: 10.1083/jcb.201104062
Debieu, D., Bach, J., Arnold, A., Brousset, S., Gredt, M., Taton, M., et al. (2000). Inhibition of ergosterol biosynthesis by morpholine, piperidine, and spiroketalamine fungicides in Microdochium nivale: effect on sterol composition and sterol Δ8 → Δ7 isomerase activity. Pestic. Biochem. Physiol. 67, 85–94. doi: 10.1006/pest.2000.2485
Delfosse, V., Bourguet, W., and Drin, G. (2020). Structural and functional specialization of OSBP-related proteins. Contact 3:251525642094662. doi: 10.1177/2515256420946627
Deorukhkar, S. C., Saini, S., and Mathew, S. (2014). Non-albicans Candida infection: an emerging threat. Interdiscip Perspect Infect Dis 2014:7. doi: 10.1155/2014/615958
Donald, K. A., Hampton, R. Y., and Fritz, I. B. (1997). Effects of overproduction of the catalytic domain of 3-hydroxy-3-methylglutaryl coenzyme a reductase on squalene synthesis in Saccharomyces cerevisiae. Appl. Environ. Microbiol. 63, 3341–3344. doi: 10.1128/aem.63.9.3341-3344.1997
Elbaz-Alon, Y., Eisenberg-Bord, M., Shinder, V., Stiller, S. B., Shimoni, E., Wiedemann, N., et al. (2015). Lam6 regulates the extent of contacts between organelles. Cell Rep. 12, 7–14. doi: 10.1016/j.celrep.2015.06.022
Espenshade, P. J., and Hughes, A. L. (2007). Regulation of sterol synthesis in eukaryotes. Annu. Rev. Genet. 41, 401–427. doi: 10.1146/annurev.genet.41.110306.130315
Feng, W., Yang, J., Xi, Z., Qiao, Z., Lv, Y., Wang, Y., et al. (2017). Mutations and/or Overexpressions of ERG4 and ERG11 genes in clinical azoles-resistant isolates of Candida albicans. Microb. Drug Resist. 23, 563–570. doi: 10.1089/mdr.2016.0095
Foresti, O., Ruggiano, A., Hannibal-Bach, H. K., Ejsing, C. S., and Carvalho, P. (2013). Sterol homeostasis requires regulated degradation of squalene monooxygenase by the ubiquitin ligase Doa10/Teb4. eLife 2:e00953. doi: 10.7554/eLife.00953
Fuentefria, A. M., Pippi, B., Dalla Lana, D. F., Donato, K. K., and de Andrade, S. F. (2018). Antifungals discovery: an insight into new strategies to combat antifungal resistance. Lett. Appl. Microbiol. 66, 2–13. doi: 10.1111/lam.12820
Garnacho-Montero, J., Barrero-García, I., and León-Moya, C. (2024). Fungal infections in immunocompromised critically ill patients. J. Intensive Med. 4, 299–306. doi: 10.1016/j.jointm.2024.01.005
Gatta, A. T., Wong, L. H., Sere, Y. Y., Calderón-Noreña, D. M., Cockcroft, S., Menon, A. K., et al. (2015). A new family of StART domain proteins at membrane contact sites has a role in ER-PM sterol transport. eLife 4:e07253. doi: 10.7554/eLife.07253
Gómez, M., Campusano, S., Gutiérrez, M. S., Sepúlveda, D., Barahona, S., Baeza, M., et al. (2020). Sterol regulatory element-binding protein Sre1 regulates carotenogenesis in the red yeast Xanthophyllomyces dendrorhous. J. Lipid Res. 61, 1658–1674. doi: 10.1194/jlr.RA120000975
Hammoudi Halat, D., Younes, S., Mourad, N., and Rahal, M. (2022). Allylamines, Benzylamines, and fungal cell permeability: a review of mechanistic effects and usefulness against fungal pathogens. Membranes 12:1171. doi: 10.3390/membranes12121171
Hampton, R. Y., and Bhakta, H. (1997). Ubiquitin-mediated regulation of 3-hydroxy-3-methylglutaryl-CoA reductase. Proc. Natl. Acad. Sci. USA 94, 12944–12948. doi: 10.1073/pnas.94.24.12944
Hani, U., Shivakumar, H. G., Vaghela, R., Osmani, R. A. M., and Shrivastava, A. (2015). Candidiasis: a fungal infection- current challenges and Progress in prevention and treatment. Infect. Disord. Drug Targets 15, 42–52. doi: 10.2174/1871526515666150320162036
Hannich, J. T., Umebayashi, K., and Riezman, H. (2011). Distribution and functions of sterols and sphingolipids. Cold Spring Harb. Perspect. Biol. 3:a004762. doi: 10.1101/cshperspect.a004762
He, X., Zhao, M., Chen, J., Wu, R., Zhang, J., Cui, R., et al. (2015). Overexpression of both ERG11 and ABC2 genes might be responsible for itraconazole resistance in clinical isolates of Candida krusei. PLoS One 10:e0136185. doi: 10.1371/journal.pone.0136185
Henry, K. W., Nickels, J. T., and Edlind, T. D. (2000). Upregulation of ERG genes in Candida species by azoles and other sterol biosynthesis inhibitors. Antimicrob. Agents Chemother. 44, 2693–2700. doi: 10.1128/AAC.44.10.2693-2700.2000
Hu, Z., He, B., Ma, L., Sun, Y., Niu, Y., and Zeng, B. (2017). Recent advances in Ergosterol biosynthesis and regulation mechanisms in Saccharomyces cerevisiae. Indian J. Microbiol. 57, 270–277. doi: 10.1007/s12088-017-0657-1
Huang, L. J., and Chen, R. H. (2023). Lipid saturation induces degradation of squalene epoxidase for sterol homeostasis and cell survival. Life Sci. Alliance 6:e202201612. doi: 10.26508/lsa.202201612
Hughes, A. L., Todd, B. L., and Espenshade, P. J. (2005). SREBP pathway responds to sterols and functions as an oxygen sensor in fission yeast. Cell 120, 831–842. doi: 10.1016/j.cell.2005.01.012
Iwaki, T., Iefuji, H., Hiraga, Y., Hosomi, A., Morita, T., Giga-Hama, Y., et al. (2008). Multiple functions of ergosterol in the fission yeast Schizosaccharomyces pombe. Microbiol 154, 830–841. doi: 10.1099/mic.0.2007/011155-0
Jackson, C. L., Walch, L., and Verbavatz, J. M. (2016). Lipids and their trafficking: an integral part of cellular organization. Dev. Cell 39, 139–153. doi: 10.1016/j.devcel.2016.09.030
Jacquier, N., Choudhary, V., Mari, M., Toulmay, A., Reggiori, F., and Schneiter, R. (2011). Lipid droplets are functionally connected to the endoplasmic reticulum in Saccharomyces cerevisiae. J. Cell Sci. 124, 2424–2437. doi: 10.1242/jcs.076836
Jacquier, N., and Schneiter, R. (2012). Mechanisms of sterol uptake and transport in yeast. J. Steroid Biochem. Mol. Biol. 129, 70–78. doi: 10.1016/j.jsbmb.2010.11.014
Jangir, P., Kalra, S., Tanwar, S., and Bari, V. K. (2023). Azole resistance in Candida auris: mechanisms and combinatorial therapy. APMIS 131, 442–462. doi: 10.1111/apm.13336
Jensen-Pergakes, K., Guo, Z., Giattina, M., Sturley, S. L., and Bard, M. (2001). Transcriptional regulation of the two sterol esterification genes in the yeast Saccharomyces cerevisiae. J. Bacteriol. 183, 4950–4957. doi: 10.1128/JB.183.17.4950-4957.2001
Jordá, T., Barba-Aliaga, M., Rozès, N., Alepuz, P., Martínez-Pastor, M. T., and Puig, S. (2022). Transcriptional regulation of ergosterol biosynthesis genes in response to iron deficiency. Environ. Microbiol. 24, 5248–5260. doi: 10.1111/1462-2920.16157
Jordá, T., and Puig, S. (2020). Regulation of Ergosterol biosynthesis in Saccharomyces cerevisiae. Genes 11:795. doi: 10.3390/genes11070795
Joshua, I., and Höfken, T. (2017). From lipid homeostasis to differentiation: old and new functions of the zinc cluster proteins Ecm22, Upc2, Sut1 and Sut2. Int. J. Mol. Sci. 18:772. doi: 10.3390/ijms18040772
Kainz, K., Bauer, M. A., Madeo, F., and Carmona-Gutierrez, D. (2020). Fungal infections in humans: the silent crisis. Microb Cell 7, 143–145. doi: 10.15698/mic2020.06.718
Kathiravan, M. K., Salake, A. B., Chothe, A. S., Dudhe, P. B., Watode, R. P., Mukta, M. S., et al. (2012). The biology and chemistry of antifungal agents: a review. Bioorg. Med. Chem. 20, 5678–5698. doi: 10.1016/j.bmc.2012.04.045
Kelly, S. L., Lamb, D. C., Taylor, M., Corran, A. J., Baldwin, B. C., and Powderly, W. G. (1994). Resistance to amphotericin B associated with defective sterol Δ8→7 isomerase in a Cryptococcus neoformans strain from an AIDS patient. FEMS Microbiol. Lett. 122, 39–42. doi: 10.1111/j.1574-6968.1994.tb07140.x
Kodedová, M., and Sychrová, H. (2015). Changes in the sterol composition of the plasma membrane affect membrane potential, salt tolerance and the activity of multidrug resistance pumps in Saccharomyces cerevisiae. PLoS One 10:e0139306. doi: 10.1371/journal.pone.0139306
Kvam, E., and Goldfarb, D. S. (2004). Nvj1p is the outer-nuclear-membrane receptor for oxysterol-binding protein homolog Osh1p in Saccharomyces cerevisiae. J. Cell Sci. 117, 4959–4968. doi: 10.1242/jcs.01372
Kyte, J., and Doolittle, R. F. (1982). A simple method for displaying the hydropathic character of a protein. J. Mol. Biol. 157, 105–132. doi: 10.1016/0022-2836(82)90515-0
Lass-Flörl, C., Kanj, S. S., Govender, N. P., Thompson, G. R. III, Ostrosky- Zeichner, L., and Govrins, M. A. (2024). Invasive candidiasis. Nat. Rev. Dis. Primers 10, 1–18. doi: 10.1038/s41572-024-00503-3
Layer, J. V., Barnes, B. M., Yamasaki, Y., Barbuch, R., Li, L., Taramino, S., et al. (2013). Characterization of a mutation that results in independence of oxidosqualene cyclase (Erg7) activity from the downstream 3-ketoreductase (Erg27) in the yeast ergosterol biosynthetic pathway. Biochim. Biophys. Acta Mol. Cell Biol. Lipids 1831, 361–369. doi: 10.1016/j.bbalip.2012.09.012
Leber, R., Landl, K., Zinser, E., Ahorn, H., Spök, A., Kohlwein, S. D., et al. (1998). Dual localization of squalene epoxidase, Erg1p, in yeast reflects a relationship between the endoplasmic reticulum and lipid particles. Mol. Biol. Cell 9, 375–386. doi: 10.1091/mbc.9.2.375
LeBlanc, M. A., and McMaster, C. R. (2010). Lipid binding requirements for oxysterol-binding protein Kes1 inhibition of autophagy and endosome-trans-Golgi trafficking pathways. J. Biol. Chem. 285, 33875–33884. doi: 10.1074/jbc.M110.147264
Lee, Y., Robbins, N., and Cowen, L. E. (2023). Molecular mechanisms governing antifungal drug resistance. Antimicrob Resist 1:5. doi: 10.1038/s44259-023-00007-2
Lev, S. (2010). Non-vesicular lipid transport by lipid-transfer proteins and beyond. Nat. Rev. Mol. Cell Biol. 11, 739–750. doi: 10.1038/nrm2971
Levine, T. P., and Munro, S. (2001). Dual targeting of Osh1p, a yeast homologue of oxysterol-binding protein, to both the Golgi and the nucleus-vacuole junction. Mol. Biol. Cell 12, 1633–1644. doi: 10.1091/mbc.12.6.1633
Li, X., Rivas, M. P., Fang, M., Marchena, J., Mehrotra, B., Chaudhary, A., et al. (2002). Analysis of oxysterol binding protein homologue Kes1p function in regulation of Sec14p-dependent protein transport from the yeast Golgi complex. J. Cell Biol. 157, 63–78. doi: 10.1083/jcb.200201037
Lin, Y., Ran, L., Du, X., Yang, H., and Wu, Y. (2023). Oxysterol-binding protein: new insights into lipid transport functions and human diseases. Biochim. Biophys. Acta Mol. Cell Biol. Lipids 1868:159365. doi: 10.1016/j.bbalip.2023.159365
Ling, Y., Hayano, S., and Novick, P. (2014). Osh4p is needed to reduce the level of phosphatidylinositol-4-phosphate on secretory vesicles as they mature. Mol. Biol. Cell 25, 3389–3400. doi: 10.1091/mbc.e14-06-1087
Liu, J. F., Xia, J. J., Nie, K. L., Wang, F., and Deng, L. (2019). Outline of the biosynthesis and regulation of ergosterol in yeast. World J. Microbiol. Biotechnol. 35:98. doi: 10.1007/s11274-019-2673-2
Lockhart, S. R. (2019). Candida auris and multidrug resistance: defining the new normal. Fungal Genet. Biol. 131:103243. doi: 10.1016/j.fgb.2019.103243
Lorenz, R. T., and Parks, L. W. (1992). Cloning, sequencing, and disruption of the gene encoding sterol C-14 reductase in Saccharomyces cerevisiae. DNA Cell Biol. 11, 685–692. doi: 10.1089/dna.1992.11.685
Lotfali, E., Ghajari, A., Kordbacheh, P., Zaini, F., Mirhendi, H., Mohammadi, R., et al. (2017). Regulation of ERG3, ERG6, and ERG11 genes in antifungal-resistant isolates of Candida parapsilosis. Iran. Biomed. J. 21, 275–281. doi: 10.18869/acadpub.ibj.21.4.275
Low, C. Y., and Rotstein, C. (2011). Emerging fungal infections in immunocompromised patients. F1000 Med. Rep. 3:14. doi: 10.3410/M3-14
Luna-Tapia, A., Peters, B. M., Eberle, K. E., Kerns, M. E., Foster, T. P., Marrero, L., et al. (2015). ERG2 and ERG24 are required for Normal vacuolar physiology as well as Candida albicans pathogenicity in a murine model of disseminated but not vaginal candidiasis. Eukaryot. Cell 14, 1006–1016. doi: 10.1128/EC.00116-15
Lv, Q. Z., Yan, L., and Jiang, Y. Y. (2016). The synthesis, regulation, and functions of sterols in Candida albicans: well-known but still lots to learn. Virulence 7, 649–659. doi: 10.1080/21505594.2016.1188236
Madaan, K., and Bari, V. K. (2023). Emerging role of sphingolipids in amphotericin B drug resistance. Microb. Drug Resist. 29, 319–332. doi: 10.1089/mdr.2022.0353
Maeda, K., Anand, K., Chiapparino, A., Kumar, A., Poletto, M., Kaksonen, M., et al. (2013). Interactome map uncovers phosphatidylserine transport by oxysterol-binding proteins. Nature 501, 257–261. doi: 10.1038/nature12430
Makanjuola, O., Bongomin, F., and Fayemiwo, S. A. (2018). An update on the roles of non-albicans Candida species in Vulvovaginitis. J. Fungi 4:121. doi: 10.3390/jof4040121
Marie, C., Leyde, S., and White, T. C. (2008). Cytoplasmic localization of sterol transcription factors Upc2p and Ecm22p in S. cerevisiae. Fungal Genet. Biol. 45, 1430–1438. doi: 10.1016/j.fgb.2008.07.004
Martel, C. M., Parker, J. E., Bader, O., Weig, M., Gross, U., Warrilow, A. G. S., et al. (2010). A clinical isolate of Candida albicans with mutations in ERG11 (encoding sterol 14α-demethylase) and ERG5 (encoding C22 desaturase) is cross resistant to azoles and amphotericin B. Antimicrob. Agents Chemother. 54, 3578–3583. doi: 10.1128/AAC.00303-10
McCarty, T. P., and Pappas, P. G. (2016). Invasive Candidiasis. Infect. Dis. Clin. N. Am. 30, 103–124. doi: 10.1016/j.idc.2015.10.013
McDermott, A. (2022). Drug-resistant fungi on the rise. Proc. Natl. Acad. Sci. USA 119:e2217948119. doi: 10.1073/pnas.2217948119
Mesmin, B., Antonny, B., and Drin, G. (2013). Insights into the mechanisms of sterol transport between organelles. Cell. Mol. Life Sci. 70, 3405–3421. doi: 10.1007/s00018-012-1247-3
Miziorko, H. M. (2011). Enzymes of the mevalonate pathway of isoprenoid biosynthesis. Arch. Biochem. Biophys. 505, 131–143. doi: 10.1016/j.abb.2010.09.028
Mochizuki, S., Miki, H., Zhou, R., and Noda, Y. (2022). The involvement of oxysterol-binding protein-related protein (ORP) 6 in the counter-transport of phosphatidylinositol-4-phosphate (PI4P) and phosphatidylserine (PS) in neurons. Biochem Biophys Rep 30:101257. doi: 10.1016/j.bbrep.2022.101257
Muramoto, M., Mineoka, N., Fukuda, K., Kuriyama, S., Masatani, T., and Fujita, A. (2024). Coordinated regulation of phosphatidylinositol 4-phosphate and phosphatidylserine levels by Osh4p and Osh5p is an essential regulatory mechanism in autophagy. Biochim. Biophys. Acta Biomembr. 1866:184308. doi: 10.1016/j.bbamem.2024.184308
Murley, A., Sarsam, R. D., Toulmay, A., Yamada, J., Prinz, W. A., and Nunnari, J. (2015). Ltc1 is an ER‐localized sterol transporter and a component of ER‐mitochondria and ER‐vacuole contacts. J. Cell. Biol. 209, 539–548. doi: 10.1083/jcb.201502033
Nagi, M., Tanabe, K., Ueno, K., Nakayama, H., Aoyama, T., Chibana, H., et al. (2013). The Candida glabrata sterol scavenging mechanism, mediated by the ATP-binding cassette transporter Aus1p, is regulated by iron limitation. Mol. Microbiol. 88, 371–381. doi: 10.1111/mmi.12189
Náhlík, J., Hrnčiřík, P., Mareš, J., Rychtera, M., and Kent, C. A. (2017). Towards the design of an optimal strategy for the production of ergosterol from Saccharomyces cerevisiae yeasts. Biotechnol. Prog. 33, 838–848. doi: 10.1002/btpr.2436
Nakatsu, F., and Kawasaki, A. (2021). Functions of oxysterol-binding proteins at membrane contact sites and their control by phosphoinositide metabolism. Front. Cell Dev. Biol. 9:664788. doi: 10.3389/fcell.2021.664788
Nakayama, H., Tanabe, K., Bard, M., Hodgson, W., Wu, S., Takemori, D., et al. (2007). The Candida glabrata putative sterol transporter gene CgAUS1 protects cells against azoles in the presence of serum. J. Antimicrob. Chemother. 60, 1264–1272. doi: 10.1093/jac/dkm321
Ngo, M. H., Colbourne, T. R., and Ridgway, N. D. (2010). Functional implications of sterol transport by the oxysterol-binding protein gene family. Biochem. J. 429, 13–24. doi: 10.1042/BJ20100263
Odds, F. C. (2009). In Candida albicans, resistance to flucytosine and terbinafine is linked to MAT locus homozygosity and multilocus sequence typing clade 1. FEMS Yeast Res. 9, 1091–1101. doi: 10.1111/j.1567-1364.2009.00577.x
Okamoto, M., Takahashi-Nakaguchi, A., Tejima, K., Sasamoto, K., Yamaguchi, M., Aoyama, T., et al. (2022). Erg25 controls host-cholesterol uptake mediated by Aus1p-associated sterol-rich membrane domains in Candida glabrata. Front. Cell Dev. Biol. 10:820675. doi: 10.3389/fcell.2022.820675
Omelchuk, O. A., Tevyashova, A. N., and Shchekotikhin, A. E. (2018). Recent advances in antifungal drug discovery based on polyene macrolide antibiotics. Rus Chem Rev 87, 1206–1225. doi: 10.1070/RCR4841
Onyewu, C., Blankenship, J. R., Del Poeta, M., and Heitman, J. (2003). Ergosterol biosynthesis inhibitors become fungicidal when combined with calcineurin inhibitors against Candida albicans, Candida glabrata, and Candida krusei. Antimicrob. Agents Chemother. 47, 956–964. doi: 10.1128/AAC.47.3.956-964.2003
Oulmouden, A., and Karst, F. (1991). Nucleotide sequence of the ERG12 gene of Saccharomyces cerevisiae encoding mevalonate kinase. Curr. Genet. 19, 9–14. doi: 10.1007/BF00362081
Parks, L. W., and Casey, W. M. (1995). Physiological implications of sterol biosynthesis in yeast. Ann. Rev. Microbiol. 49, 95–116. doi: 10.1146/annurev.mi.49.100195.000523
Perlin, D. S. (2015). Mechanisms of echinocandin antifungal drug resistance. Ann. N. Y. Acad. Sci. 1354, 1–11. doi: 10.1111/nyas.12831
Petranyi, G., Ryder, N. S., and Stütz, A. (1984). Allylamine derivatives: new class of synthetic antifungal agents inhibiting fungal squalene epoxidase. Science 224, 1239–1241. doi: 10.1126/science.6547247
Peyton, L. R., Gallagher, S., and Hashemzadeh, M. (2015). Triazole antifungals: a review. Drugs Today 51, 705–718. doi: 10.1358/dot.2015.51.12.2421058
Pfaller, M. A., Andes, D. R., Diekema, D. J., Horn, D. L., Reboli, A. C., Rotstein, C., et al. (2014). Epidemiology and outcomes of invasive candidiasis due to non-albicans species of Candida in 2,496 patients: data from the prospective antifungal therapy (PATH) registry 2004-2008. PLoS One 9:e101510. doi: 10.1371/journal.pone.0101510
Pfaller, M. A., and Diekema, D. J. (2007). Epidemiology of invasive candidiasis: a persistent public health problem. Clin. Microbiol. Rev. 20, 133–163. doi: 10.1128/CMR.00029-06
Ploier, B., Korber, M., Schmidt, C., Koch, B., Leitner, E., and Daum, G. (2015). Regulatory link between steryl ester formation and hydrolysis in the yeast Saccharomyces cerevisiae. Biochim Biophys Acta-Mol Cell Bio Lipids 1851, 977–986. doi: 10.1016/j.bbalip.2015.02.011
Polak, A. (1988). Mode of action of Morpholine derivatives. Ann. N. Y. Acad. Sci. 544, 221–228. doi: 10.1111/j.1749-6632.1988.tb40406.x
Powis, G., Meuillet, E. J., Indarte, M., Booher, G., and Kirkpatrick, L. (2023). Pleckstrin homology [PH] domain, structure, mechanism, and contribution to human disease. Biomed. Pharmacother. 165:115024. doi: 10.1016/j.biopha.2023.115024
Raychaudhuri, S., and Prinz, W. A. (2010). The diverse functions of oxysterol-binding proteins. Ann Rev Cell Dev Biol 26, 157–177. doi: 10.1146/annurev.cellbio.042308.113334
Rodrigues, M. L. (2018). The multifunctional fungal Ergosterol. MBio 9, e01755–e01718. doi: 10.1128/mBio.01755-18
Rogaski, B., Lim, J. B., and Klauda, J. B. (2010). Sterol binding and membrane lipid attachment to the Osh4 protein of yeast. J. Phys. Chem. B 114, 13562–13573. doi: 10.1021/jp106890e
Rybak, J. M., Barker, K. S., Muñoz, J. F., Parker, J. E., Ahmad, S., Mokaddas, E., et al. (2022). In vivo, emergence of high-level resistance during treatment reveals the first identified mechanism of amphotericin B resistance in Candida auris. Clin. Microbiol. Infect. 28, 838–843. doi: 10.1016/j.cmi.2021.11.024
Ryder, N. S. (1987). Squalene epoxidase as the target of antifungal allylamines. Pest Sci 21, 281–288. doi: 10.1002/ps.2780210405
Ryder, N. S., and Dupont, M. C. (1985). Inhibition of squalene epoxidase by allylamine antimycotic compounds. A comparative study of the fungal and mammalian enzymes. Biochem. J. 230, 765–770. doi: 10.1042/bj2300765
Sanglard, D., Ischer, F., Parkinson, T., Falconer, D., and Bille, J. (2003). Candida albicans mutations in the Ergosterol biosynthetic pathway and resistance to several antifungal agents. Antimicrob. Agents Chemother. 47, 2404–2412. doi: 10.1128/AAC.47.8.2404-2412.2003
Sanguinetti, M., Posteraro, B., and Lass-Flörl, C. (2015). Antifungal drug resistance among Candida species: mechanisms and clinical impact. Mycoses 58, 2–13. doi: 10.1111/myc.12330
Schulz, T. A., and Prinz, W. A. (2007). Sterol transport in yeast and the oxysterol binding protein homologue (OSH) family. Biochim. Biophys. Acta Mol. Cell Biol. Lipids 1771, 769–780. doi: 10.1016/j.bbalip.2007.03.003
Shakoury-Elizeh, M., Protchenko, O., Berger, A., Cox, J., Gable, K., Dunn, T. M., et al. (2010). Metabolic response to Iron deficiency in Saccharomyces cerevisiae. J. Biol. Chem. 285, 14823–14833. doi: 10.1074/jbc.M109.091710
Shianna, K. V., Dotson, W. D., Tove, S., and Parks, L. W. (2001). Identification of a UPC2 homolog in Saccharomyces cerevisiae and its involvement in aerobic sterol uptake. J. Bacteriol. 183, 830–834. doi: 10.1128/JB.183.3.830-834.2001
Shrivastava, M., Kouyoumdjian, G. S., Kirbizakis, E., Ruiz, D., Henry, M., Vincent, A. T., et al. (2023). The Adr1 transcription factor directs regulation of the ergosterol pathway and azole resistance in Candida albicans. MBio 14, e01807–e01823. doi: 10.1128/mbio.01807-23
Sigera, L. S. M., and Denning, D. W. (2023). Flucytosine and its clinical usage. Ther Adv Infect Dis. 10:20499361231161387. doi: 10.1177/20499361231161387
Sokolov, S. S., Vorobeva, M. A., Smirnova, A. I., Smirnova, E. A., Trushina, N. I., Galkina, K. V., et al. (2020). LAM genes contribute to environmental stress tolerance but sensibilize yeast cells to azoles. Front. Microbiol. 11:38. doi: 10.3389/fmicb.2020.00038
Song, J., Zhai, P., Zhang, Y., Zhang, C., Sang, H., Han, G., et al. (2016). The Aspergillus fumigatus damage resistance protein family coordinately regulates Ergosterol biosynthesis and azole susceptibility. MBio 7:e01919. doi: 10.1128/mBio.01919-15
Spivak, E. S., and Hanson, K. E. (2018). Candida auris: an emerging fungal pathogen. J. Clin. Microbiol. 56, e01588–e01517. doi: 10.1128/JCM.01588-17
Stewart, E. V., Nwosu, C. C., Tong, Z., Roguev, A., Cummins, T. D., Kim, D. U., et al. (2011). Yeast SREBP cleavage activation requires the Golgi Dsc E3 ligase complex. Mol. Cell. 42, 160–171. doi: 10.1016/j.molcel.2011.02.035
Stolz, J., and Sauer, N. (1999). The fenpropimorph resistance gene FEN2 from Saccharomyces cerevisiae encodes a plasma membrane H+-pantothenate symporter. J. Biol. Chem. 274, 18747–18752. doi: 10.1074/jbc.274.26.18747
Taei, M., Chadeganipour, M., and Mohammadi, R. (2019). An alarming rise of non-albicans Candida species and uncommon yeasts in the clinical samples; a combination of various molecular techniques for identification of etiologic agents. BMC. Res. Notes 12:779. doi: 10.1186/s13104-019-4811-1
te Welscher, Y. M., Jones, L., van Leeuwen, M. R., Dijksterhuis, J., de Kruijff, B., Eitzen, G., et al. (2010). Natamycin inhibits vacuole fusion at the priming phase via a specific interaction with ergosterol. Antimicrob. Agents Chemother. 54, 2618–2625. doi: 10.1128/AAC.01794-09
Teske, B., Taramino, S., Bhuiyan, M. S. A., Kumaraswami, N. S., Randall, S. K., Barbuch, R., et al. (2008). Genetic analyses involving interactions between the ergosterol biosynthetic enzymes, lanosterol synthase (Erg7p) and 3-ketoreductase (Erg27p), in the yeast Saccharomyces cerevisiae. Biochim. Biophys. Acta Mol. Cell Biol. Lipids 1781, 359–366. doi: 10.1016/j.bbalip.2008.04.017
Theesfeld, C. L., and Hampton, R. Y. (2013). Insulin-induced gene protein (INSIG)-dependent sterol regulation of Hmg2 endoplasmic reticulum-associated degradation (ERAD) in yeast. J. Biol. Chem. 288, 8519–8530. doi: 10.1074/jbc.M112.404517
Tøndervik, A., Sletta, H., Klinkenberg, G., Emanuel, C., Powell, L. C., Pritchard, M. F., et al. (2014). Alginate oligosaccharides inhibit fungal cell growth and potentiate the activity of antifungals against Candida and Aspergillus spp. PLoS One 9:e112518. doi: 10.1371/journal.pone.0112518
Tong, J., Manik, M. K., and Im, Y. J. (2018). Structural basis of sterol recognition and nonvesicular transport by lipid transfer proteins anchored at membrane contact sites. Proc. Natl. Acad. Sci. USA 115, E856–E865. doi: 10.1073/pnas.1719709115
Tsay, Y. H., and Robinson, G. W. (1991). Cloning and characterization of ERG8 an essential gene of Saccharomyces cerevisiae that encodes phosphomevalonate kinase. Mol. Cell. Biol. 11, 620–631. doi: 10.1128/mcb.11.2.620-631.1991
Valachovic, M., Hronská, L., and Hapala, I. (2001). Anaerobiosis induces complex changes in sterol esterification pattern in the yeast Saccharomyces cerevisiae. FEMS Microbiol. Lett. 197, 41–45. doi: 10.1111/j.1574-6968.2001.tb10580.x
Vandeputte, P., Tronchin, G., Larcher, G., Ernoult, E., Bergès, T., Chabasse, D., et al. (2008). A nonsense mutation in the ERG6 gene leads to reduced susceptibility to polyenes in a clinical isolate of Candida glabrata. Antimicrob. Agents Chemother. 52, 3701–3709. doi: 10.1128/AAC.00423-08
Vanegas, J. M., Contreras, M. F., Faller, R., and Longo, M. L. (2012). Role of unsaturated lipid and ergosterol in ethanol tolerance of model yeast biomembranes. Biophys. J. 102, 507–516. doi: 10.1016/j.bpj.2011.12.038
Vik, Å., and Rine, J. (2001). Upc2p and Ecm22p, dual regulators of sterol biosynthesis in Saccharomyces cerevisiae. Mol. Cell. Biol. 21, 6395–6405. doi: 10.1128/mcb.21.19.6395-6405.2001
Vil, V., Gloriozova, T. A., Poroikov, V. V., Terentev, A. O., Savidov, N., and Dembitsky, V. M. (2019). Naturally occurring of α, β-diepoxy-containing compounds: origin, structures, and biological activities. Appl. Microbiol. Biotechnol. 103, 3249–3264. doi: 10.1007/s00253-019-09711-4
Vincent, B. M., Lancaster, A. K., Scherz-Shouval, R., Whitesell, L., and Lindquist, S. (2013). Fitness trade-offs restrict the evolution of resistance to amphotericin B. PLoS Biol. 11:e1001692. doi: 10.1371/journal.pbio.1001692
Ward, D. M., Chen, O. S., Li, L., Kaplan, J., Bhuiyan, S. A., Natarajan, S. K., et al. (2018). Altered sterol metabolism in budding yeast affects mitochondrial iron–sulfur (Fe-S) cluster synthesis. J. Biol. Chem. 293, 10782–10795. doi: 10.1074/jbc.RA118.001781
Weber-Boyvat, M., Zhong, W., Yan, D., and Olkkonen, V. M. (2013). Oxysterol-binding proteins: functions in cell regulation beyond lipid metabolism. Biochem. Pharmacol. 86, 89–95. doi: 10.1016/j.bcp.2013.02.016
Whaley, S. G., Caudle, K. E., Vermitsky, J. P., Chadwick, S. G., Toner, G., Barker, K. S., et al. (2014). UPC2A is required for high-level azole antifungal resistance in Candida glabrata. Antimicrob. Agents Chemother. 58, 4543–4554. doi: 10.1128/AAC.02217-13
Willger, S. D., Puttikamonkul, S., Kim, K. H., Burritt, J. B., Grahl, N., Metzler, L. J., et al. (2008). A sterol-regulatory element binding protein is required for cell polarity, hypoxia adaptation, azole drug resistance, and virulence in Aspergillus fumigatus. PLoS Pathog. 4:e1000200. doi: 10.1371/journal.ppat.1000200
Wong, L. H., Gatta, A. T., and Levine, T. P. (2019). Lipid transfer proteins: the lipid commute via shuttles, bridges and tubes. Nat. Rev. Mol. Cell Biol. 20, 85–101. doi: 10.1038/s41580-018-0071-5
Woods, K., and Höfken, T. (2016). The zinc cluster proteins Upc2 and Ecm22 promote filamentation in Saccharomyces cerevisiae by sterol biosynthesis-dependent and -independent pathways. Mol. Microbiol. 99, 512–527. doi: 10.1111/mmi.13244
Yang, H., Bard, M., Bruner, D. A., Gleeson, A., Deckelbaum, R. J., Aljinovic, G., et al. (1996). Sterol esterification in yeast: a two-gene process. Science 272, 1353–1356. doi: 10.1126/science.272.5266.1353
Yang, H., Tong, J., Lee, C. W., Ha, S., Eom, S. H., and Im, Y. J. (2015). Structural mechanism of ergosterol regulation by fungal sterol transcription factor Upc2. Nat. Commun. 6:6129. doi: 10.1038/ncomms7129
Young, L. Y., Hull, C. M., and Heitman, J. (2003). Disruption of Ergosterol biosynthesis confers resistance to amphotericin B in Candida lusitaniae. Antimicrob. Agents Chemother. 47, 2717–2724. doi: 10.1128/AAC.47.9.2717-2724.2003
Zhang, J., Li, L., Lv, Q., Yan, L., Wang, Y., and Jiang, Y. (2019). The fungal CYP51s: their functions, structures, related drug resistance, and inhibitors. Front. Microbiol. 10:691. doi: 10.3389/fmicb.2019.00691
Zheng Koh, D. H., and Saheki, Y. (2021). Regulation of plasma membrane sterol homeostasis by nonvesicular lipid transport. Contact 4:251525642110424. doi: 10.1177/25152564211042451
Keywords: ergosterol metabolism, oxysterol binding proteins, sterol transfer proteins, sterol regulation, pathogenic fungi
Citation: Tanwar S, Kalra S and Bari VK (2024) Insights into the role of sterol metabolism in antifungal drug resistance: a mini-review. Front. Microbiol. 15:1409085. doi: 10.3389/fmicb.2024.1409085
Received: 29 March 2024; Accepted: 26 September 2024;
Published: 11 October 2024.
Edited by:
Lisheng Liao, South China Agricultural University, ChinaReviewed by:
Eugene A. Rogozhin, Institute of Bioorganic Chemistry (RAS), RussiaCopyright © 2024 Tanwar, Kalra and Bari. This is an open-access article distributed under the terms of the Creative Commons Attribution License (CC BY). The use, distribution or reproduction in other forums is permitted, provided the original author(s) and the copyright owner(s) are credited and that the original publication in this journal is cited, in accordance with accepted academic practice. No use, distribution or reproduction is permitted which does not comply with these terms.
*Correspondence: Vinay Kumar Bari, dmluYXkuYmFyaUBjdXAuZWR1Lmlu; dmluYXlpbXRAZ21haWwuY29t
†ORCID: Vinay Kumar Bari, https://orcid.org/0000-0002-5670-3798
Disclaimer: All claims expressed in this article are solely those of the authors and do not necessarily represent those of their affiliated organizations, or those of the publisher, the editors and the reviewers. Any product that may be evaluated in this article or claim that may be made by its manufacturer is not guaranteed or endorsed by the publisher.
Research integrity at Frontiers
Learn more about the work of our research integrity team to safeguard the quality of each article we publish.