- 1Institut de Ciències del Mar (ICM-CSIC), Barcelona, Catalunya, Spain
- 2Departamento de Microbiología y Ecología, Universitat de València, València, Spain
- 3Departament de Genètica i Microbiologia, Facultat de Biociències, Universitat Autònoma de Barcelona, Bellaterra, Spain
Prokaryotes dominate global oceans and shape biogeochemical cycles, yet most taxa remain uncultured and uncharacterized as of today. Here we present the characterization of 26 novel marine bacterial strains from a large isolate collection obtained from Blanes Bay (NW Mediterranean) microcosm experiments made in the four seasons. Morphological, cultural, biochemical, physiological, nutritional, genomic, and phylogenomic analyses were used to characterize and phylogenetically place the novel isolates. The strains represent 23 novel bacterial species and six novel genera: three novel species pertaining to class Alphaproteobacteria (families Rhodobacteraceae and Sphingomonadaceae), six novel species and three new genera from class Gammaproteobacteria (families Algiphilaceae, Salinispheraceae, and Alteromonadaceae), 13 novel species and three novel genera from class Bacteroidia (family Flavobacteriaceae), and one new species from class Rhodothermia (family Rubricoccaceae). The bacteria described here have potentially relevant roles in the cycles of carbon (e.g., carbon fixation or energy production via proteorhodopsin), nitrogen (e.g., denitrification or use of urea), sulfur (oxidation of sulfur compounds), phosphorus (acquisition and use of different forms of phosphorus and remodeling of membrane phospholipids), and hydrogen (oxidation of hydrogen to obtain energy). We mapped the genomes of the presented strains to the Tara Oceans metagenomes to reveal that these strains were globally distributed, with those of the family Flavobacteriaceae being the most widespread and abundant, while Rhodothermia being the rarest and most localized. While molecular-only approaches are also important, our study stresses the importance of culturing as a powerful tool to further understand the functioning of marine bacterial communities.
1 Introduction
Prokaryotes are the most abundant cells in the ocean (ca. ~1029; Whitman et al., 1998), and bacteria have been estimated to represent around ~22% of the ocean’s biomass (Bar-On and Milo, 2019). They possess a wide metabolic diversity, which makes them drive the ocean’s biogeochemical cycles (Falkowski et al., 2008). In fact, prokaryotes are likely responsible for most of the ocean’s respiration (Del Giorgio and Duarte, 2002) and have a major role in key steps of the cycles of carbon, nitrogen, sulfur, phosphorus, hydrogen, iron, and other metals (Gasol and Kirchman, 2018). Microbes are largely responsible for the transformation of inorganic carbon into organic matter via primary production and the transformation of this production into particulate organic matter, supplying carbon and nutrients upwards through the food web (Azam et al., 1983; Farooq, 1998; Fenchel, 2008).
While it has been estimated that there are 1012 microbial species on Earth (Locey and Lennon, 2016), only 22,525 prokaryotic species have been validly published to date (List of Prokaryotic Names with Standing Nomenclature, 11 January 2024), in part because most environmental (including marine) bacteria remain uncultured (Martiny, 2019; Steen et al., 2019).
Isolation is not only a prerequisite for the valid publication of novel taxa (Parker et al., 2019), but it is also an essential tool that allows understanding of the ecology of marine bacteria by providing the possibility to perform physiological experiments to test ecological hypotheses, unveiling their biogeochemical potential by providing access to their whole genomes (Giovannoni and Stingl, 2007), and allowing them to answer complex evolutionary questions (Imachi et al., 2020). Thus, more efforts are needed in culturing marine bacteria and comparing their DNA to environmental sequence data, which provides valuable information about the global context and ecology of the cultured taxa. Moreover, this task has become easier in the last few years with the massive amount of data generated in global sampling expeditions such as Tara Oceans (Pesant et al., 2015) or Malaspina (Duarte, 2015).
We have recently obtained a large culture collection from Blanes Bay (NW Mediterranean) seawater microcosm experiments run in the four astronomical seasons in which we tested the impact of the reduction in grazing and viral pressure, the increase in nutrient availability, and the presence/absence of light on the culturability of marine bacteria (Rey-Velasco et al., 2023). In this culturing effort, we could isolate taxa that represented the top-abundant fractions of the population after some of the manipulations, and we isolated a set of bacteria with less than 97% identity in their 16S rRNA gene sequences to cultured taxa in public databases. Based on that work, we present a comprehensive description of 26 novel marine bacterial strains, including their phenotypic, genomic, and phylogenomic characterization, their potential relevance in biogeochemical cycles, and their distribution across global oceans.
2 Materials and methods
2.1 Isolation of the strains
All strains were isolated from surface seawater samples taken in February, April, July, and November 2017 from the Blanes Bay Microbial Observatory (BBMO) in the NW Mediterranean (41°40′N, 2°48′E), approximately 1 km offshore. The seawater samples were subjected to experimental manipulations to reduce predators and viruses and increase nutrient availability, then incubated for 36–48 h as described in Sánchez et al. (2020). Subsamples of 1 mL were stored in cryovials at −80°C with 75 μL DMSO until the time of isolation. The isolation was carried out between November 2020 and January 2022 using samples from the initial and final times of the experiments, as reported in Rey-Velasco et al. (2023). Briefly, aliquots of 100 μL of undiluted, 1:10 and 1:100 diluted seawater were spread in triplicate on Marine Agar 2216 (MA; Difco) and Marine Reasoner’s 2A Agar (mR2A) plates and incubated at room temperature (20–25°C) until no more colonies appeared (maximum 30 days). Colonies with different morphologies were selected, streaked on new agar plates, transferred to liquid cultures, and stored with 25% glycerol in cryovials at −80°C.
2.2 Identification and selection of the strains
The amplification and sequencing of the 16S rRNA gene were carried out as described in Rey-Velasco et al. (2023). Briefly, the DNA of each isolate was extracted from 100 μL of liquid culture by thermal shock and was used for PCR amplification of the nearly complete 16S rRNA gene with primers 27Fmod (5′-AGR GTT TGA TCM TGG CTC AG-3′) and 1492Rmod (5′-TAC GGY TAC CTT GTT AYG ACT T-3′) from Page et al. (2005). When faint or no bands were observed on agarose gel electrophoresis following PCR, DNA extraction was repeated with the DNeasy Blood & Tissue Kit (Qiagen) following the manufacturer’s recommendations. Purification and OneShot Sanger sequencing of PCR products were carried out by Genoscreen (Lille, France) with the two above-mentioned primers. A total of 1,643 isolates were sequenced in the original study.
These 16S rRNA gene sequences were manually quality-checked, trimmed, and assembled with Geneious software v. 2022.0.1 (Kearse et al., 2012). An initial classification of the near-complete 16S rRNA gene sequences was made with the SINA aligner (Pruesse et al., 2012) against the SILVA database (release 138.1). Isolate sequences were also submitted to BLASTn v. 2.12.0+ (Altschul et al., 1990) against a subset of the RDP database (release 11; Cole et al., 2014) containing only cultured taxa, and another one containing only uncultured taxa, as shown in Figure 1 of Rey-Velasco et al. (2023). The sequences that presented less than 97% identity to cultured taxa according to these data were assessed against type strains using the Identifier tool of EzBioCloud (Yoon et al., 2017). Considering this threshold, we selected for the analyses described here 26 isolates that had less than 96.6% similarity with any type of strain.
2.3 Whole-genome sequencing, assembly, and characterization
The genomic DNA of the 26 selected strains was extracted using the Bacterial DNA Preparation Kit (Jena Bioscience), and its integrity and concentration were checked using DNA gel electrophoresis and a Qubit 1 Fluorometer (Invitrogen), respectively. Further quality control, library preparation, and whole-genome sequencing were carried out using different methodologies: three of the strains were sequenced in the Central Service of Support to Experimental Research (SCSIE) of the University of Valencia (Valencia, Spain): strains S334T and S365 with Illumina MiSeq (2× 250 bp reads) aiming to a coverage of 120× and strain S356T with Sequel PacBio RS II technology (SMRT Link version 7.0) aiming to a coverage of 500×. The other 23 isolates were sequenced in the Center Nacional d’Anàlisi Genòmica (CNAG) with an Illumina MiSeq sequencer (2× 300 bp, Illumina) aiming for a coverage of 120 ×.
The genome of strain S356T was assembled with the Hierarchical Genome Assembly Process (HGAP4) de novo assembly analysis application. The genomes of strains S334T and S365 were assembled as in (Macián et al., 2021). To assemble the other 23 genomes, we used the Nextflow pipeline bacass v.2.0.0 (Peltzer et al., 2021) from the nf-core framework (Ewels et al., 2020). The pipeline runs an automated workflow that uses Skewer (Jiang et al., 2014) to quality trim the reads, performs basic sequencing QC using FastQC,1 and assembles the reads with Unicycler (Wick et al., 2017). Assembly contamination was checked with Kraken2 (Wood et al., 2019), and its quality was assessed using QUAST (Gurevich et al., 2013).
All genomes were quality assessed with CheckM v.1.2.2 (Parks et al., 2015), which also provided basic genomic data such as %GC, genome length, or number of predicted genes. Genomes were annotated using Prokka v.1.14.6 (Seemann, 2014) and then functionally annotated with eggNOG-mapper v.2.1.10 (Cantalapiedra et al., 2021) based on eggNOG orthology data (Huerta-Cepas et al., 2019) and DIAMOND sequence searches (Buchfink et al., 2021). Taxonomic classification was obtained using GTDB-Tk v.2.3.2 (Chaumeil et al., 2020, 2022), relying on release 214 of the GTDB database (Parks et al., 2022). All these pipelines and CheckM were run using custom makefiles available on GitHub.2
2.4 16S rRNA gene sequence analysis
Sequences of 16S rRNA genes were extracted using seqkit v.2.1.0 (Shen et al., 2016) and compared with the sequences initially obtained by Sanger sequencing in order to assure the authenticity of the genomes. Their completion was tested with the Identifier tool of EzBioCloud (Yoon et al., 2017). In the case of strain W364, a part of the 16S rRNA gene sequence obtained by Sanger sequencing was added to the one extracted from the genome in order to increase its completion, after checking their overlap with BLASTn v. 2.12.0+. In the case of strain P117T, the 16S rRNA gene sequence extracted from the genome came in two pieces, which represented two exact halves of the gene according to their alignment with the sequence of the same gene obtained by Sanger sequencing; therefore, they were joined together to form a 100% complete sequence. The final set of 16S rRNA gene sequences was taxonomically classified with GTDB database rs214 (Parks et al., 2022) using the Nextflow pipeline ampliseq v.2.7.1 (Straub et al., 2023) from the nf-core framework (Ewels et al., 2020).
2.5 Phenotypic characterization
Colony morphology and pigmentation were recorded after 24 h, 1 week MA cultures, depending on the growth rate of each strain. Cell morphology and motility were determined by optical microscopy in wet mounts prepared from MA cultures of the same age. In some cases, cell morphology was confirmed by basic fuchsin staining. Gram determinations were made by lysis tests in 3% KOH. For the Flavobacteriaceae strains, flexirubin-type pigmentation was tested with 20% KOH according to the method of Bernadet et al. (2002).
Growth in MA and mR2A was determined by incubating the plates at 26°C in the dark. The temperature ranges for growth (4, 15, 28, 37, and 40°C) were determined in MA incubated for up to 2 days at 40°C and 3 weeks in the rest. Ranges of salinity (0, 0.5, 1, 1.5, 2, 3, 4, 5, 6, 7, 8, 9, 10, 11, 12%) and growth in 2% (w/v) NaCl as sole salt were determined after incubation for up to 2 weeks, first in a medium composed of 15 g/L agar, 5 g/L peptone, and 1 g/L yeast extract supplemented with the appropriate Sea Salts (Sigma) or NaCl quantity and, for some of the strains that did not grow well in this formulation, in Marine Agar supplemented with NaCl to reach the target concentration. Extracellular hydrolytic activities on casein, starch, Tween-80, and DNA were determined as in Pujalte et al. (2018). Extracellular hydrolysis of alginate was determined in MA supplemented with 2 g/L sodium alginate. Sole carbon and energy sources used for growth were tested on simplified Basal Medium Agar (Baumann and Baumann, 1981) composed of 20 g/L Sea Salts (Sigma), 1 g/L NH4Cl, 75 mg/L K2HPO4, 30 mg/L FeSO4·7H2O, 0.1 g/L yeast extract, 12 g Purified Agar, and 2 g/L carbon source. The medium used to test carbon sources in strains S334T, S356T, and S365 did not contain yeast extract. The oxidase test was performed with Oxoid Oxidase Strips, and catalase was tested with 10 vol. H2O2. API 20NE (for all strains except S356) and API ZYM strips (only 10 strains due to the product discontinuation) were inoculated as in Pujalte et al. (2018). Main polar lipids and respiratory quinones were determined by manually searching their synthesis enzymes in their Prokka genome annotation files and on the RAST server (Aziz et al., 2008). Other relevant taxogenomic characteristics were searched using the same methods.
2.6 Inference of phylogeny
We used the Type Strain Genome Server (TYGS; Meier-Kolthoff and Göker, 2019) to compute digital DNA–DNA hybridization (dDDH) values between the novel strains and related type strains, then selected the 25 closest type strains for each isolate and grouped them according to their classification into classes. The accession numbers of these reference strains were obtained from the TYGS output, and their genome sequences were downloaded using NCBI Datasets.3 Average nucleotide identity (ANI) was computed with fastANI (Jain et al., 2018), and average aminoacid identity (AAI) was calculated with EzAAI (Kim D, et al., 2021) between all the strains, the novel and the reference ones, within each group. The Kostas Lab ANI/AAI matrix tool (Rodriguez-R and Konstantinidis, 2016) was used to cross-check some of the AAI values. Phylogenomic trees were computed for each group with UBCG2 (Kim J, et al., 2021), including outgroups and, in some cases, relevant type strains that were missing in the original TYGS search. The trees were graphically represented and customized using the Interactive Tree of Life (iTOL; Letunic and Bork, 2021). Nodes with gene support values lower than 60 (out of 81 core gene trees) were deleted.
2.7 Screening of biogeochemically relevant genes and geographic distribution
We generated a curated list of biogeochemically relevant genes involved in the metabolism of carbon, nitrogen, phosphorus, sulfur, iron, and hydrogen based on previous findings (e.g., Ferrera et al., 2015; Satinsky et al., 2017; Auladell et al., 2023). This list is available in Supplementary Table S1. We searched the KEGG orthologs (KO; Jin et al., 2023), Pfams (Mistry et al., 2021), or Clusters of Orthologous Genes (COGs; Tatusov et al., 2000) that these genes represented in eggNOG-mapper annotations.
The geographic distribution of the strains was computed by calculating the coverages of the novel genomes in Tara Oceans metagenomes (Pesant et al., 2015) with CoverM v.0.6.14 using BWA-MEM v.0.7.17-r1188 (Li, 2013) as the mapping algorithm. Mapping with BWA failed for a few files because they had unmatching read names; therefore, they had to be repaired with BBMap v.38.90 (Bushnell, n.d.) and mapped again. We set 25% breadth of coverage as a threshold to decide whether a strain was present in a given sample.
Output tables were sorted and plots were generated in R software v.4.1.3 (Team, R. C, 2022) and RStudio v.1.3.1093 (Team, R. S, 2020), mainly with packages tidyverse v.1.3.1 (Wickham et al., 2019), qdap v.2.4.3 (Rinker, 2020), magrittr v.2.0.3 (Bache and Wickham, 2022), maps v.3.4.1.1 (Becker and Wilks, 2023), and mapdata v.2.3.1 (Becker and Wilks, 2022).
3 Results
3.1 Overview of the strains and genome features
The 26 strains characterized here are listed in Table 1, along with their taxonomic affiliation and main genomic features. Fourteen of them pertain to the Flavobacteriaceae (class Bacteroidia); seven are Gammaproteobacteria of families Alteromonadaceae, Salinispheraceae, and Algiphilaceae; three belong to families Roseobacteraceae, Sphingomonadaceae, and Erythrobacteraceae from class Alphaproteobacteria; and two affiliate with Rubrivirga of the Rhodothermia class. Strain S334T has the largest genome (4.57 Mpb), while the smallest (2.84 Mpb) is that of strain W242T, both Flavobacteriaceae. The highest G + C content (73.7%) was observed in strains F394T and S365, while Flavobacteriaceae strains contained the lowest percentages, with strain W332T having the minimum with 32.5%.
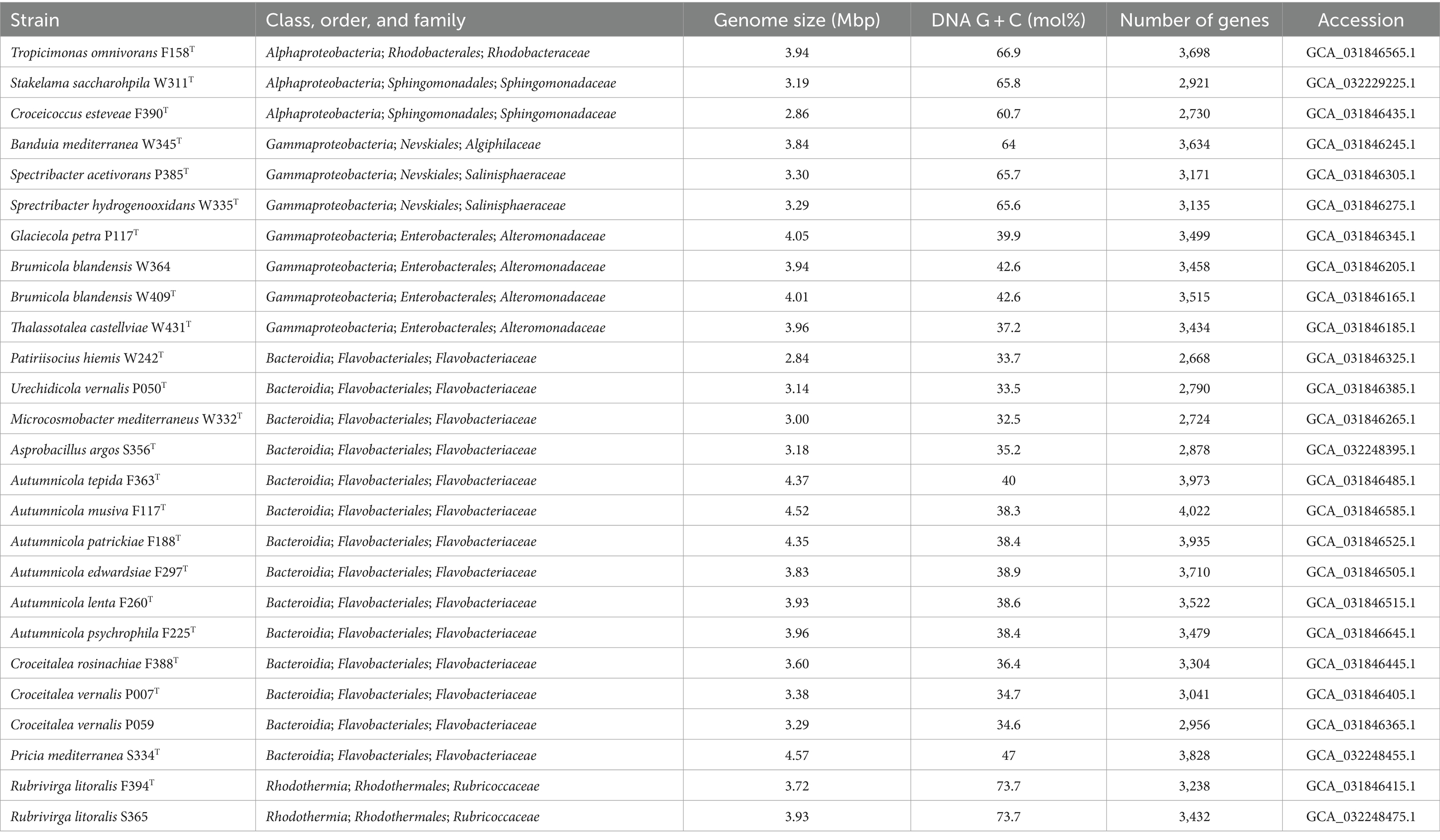
Table 1. Overview of the strains described in this study, their taxonomy, and main genomic characteristics.
Extended genome features and quality values for all the strains are reported in Supplementary Table S2. The genome completeness reported by CheckM was always >96%, with an average of 98.94 ± 0.82%, ranging from 99.89 to 96.72%. Mean contamination was 0.79 ± 0.69% (range 0–3.01%). All the 16S rRNA genes obtained from the genomes matched 100% with those previously obtained by Sanger sequencing, and all but two scored 100% completion in the EzBioCloud identifier. The exceptions were strains W364 and S356T with 95.1 and 98.1% completeness, respectively.
3.2 Phylogeny
The full taxonomic classification of the 16S rRNA genes (with EzBioCloud identifier and GTDB) and of the genomes (with GTDB) for each strain is shown in Supplementary Table S3. Pairwise ANI values of strains belonging to the same species, P007T–P059, W364–W409T, and F394T–S365, are >95% (Supplementary Table S4). The highest ANI value was 99.36% between strains W364T and W409. Phylogenomic comparisons with the closest type strains (Supplementary Table S2 for the closest strains; Supplementary Table S5 for all results) showed no dDDH values higher than 70% nor ANI values higher than 95% for any of the tested genomes. The closest AAI value for strain W345T was 62.95% (Fontimonas thermophila DSM 23609T). In the case of strains W409T and W364, their closest AAI values were, respectively, 76.85 and 76.84%, both to Glaciecola nitratireducens FR1064T. Strains W335T and P385T had, respectively, Salinisphaera halophila YIM 95161T (63.99%) and Salinisphaera orenii MKB5T (64.07%) as their closest strains in terms of AAI. Strain S356T presented an AAI of 72.13% with Polaribacter septentrionalilitoris ANORD1T. The closest AAI for strains F363T, F117T, F188T, F297T, F260T, and F225T ranged from 74.24 to 75.17% with strains Salegentibacter mishustinae KCTC 12263T, Salegentibacter agarivorans DSM 23515T, and Salegentibacter salarius DSM 23401T.
We phylogenomically compared the genomes of the Alphaproteobacteria, where strain F158T formed a tree branch close to Tropicimonas isoalkanivorans DSM 19548T and Tropicimonas sediminicola DSM 29339T inside a node within members of the Roseobacteraceae family (Supplementary Table S5; Figure 1). Strain W311T appeared in a node with other Stakelama species, having Stakelama marina LXI357T as its closest neighbor. Finally, strain F390T formed a relatively long branch in a node shared with Croceicoccus hydrothermalis JLT 1T, which in turn was included in another node with five other Croceicoccus species. In the case of Gammaproteobacteria (Figure 2), strain W345T formed a reasonably long branch inside a clade between members of the Nevskiaceae family and two Algiphilus species, which were together in a different node. Strains P385T and W335T constituted a different node inside a clade with members of the family Salinispheraceae. Strain P117T formed a node with Glaciecola punicea ACAM 611T in the same clade as strains W364 and W409T, which grouped together in a node related to different one which contains Glaciecola nitratireducens FR1064T and Glaciecola pallidula DSM 14239T. In the case of strain W431T, it was placed among other Thalassotalea species, grouping with Thalassotalea insulae KCTC 62186T.
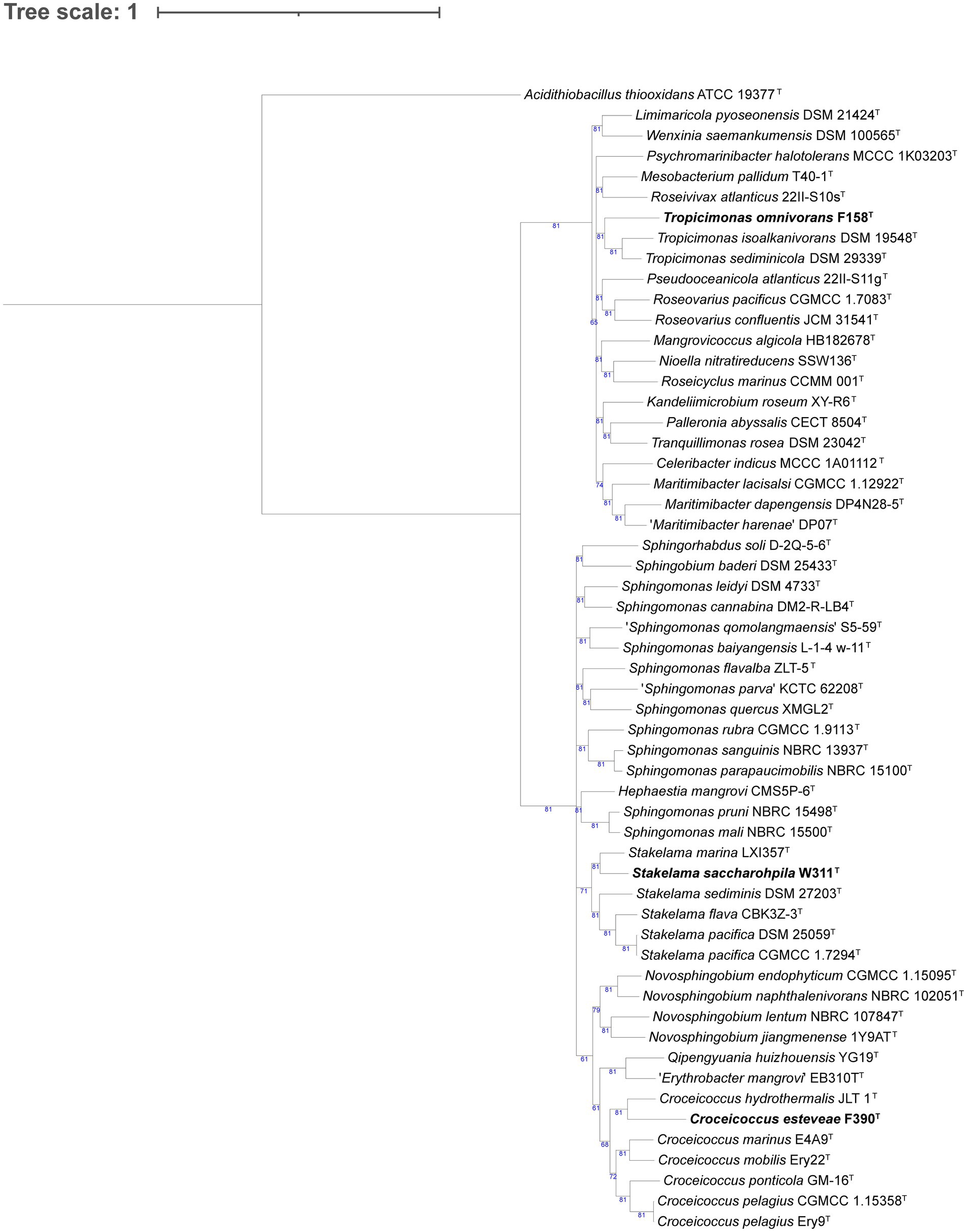
Figure 1. Phylogenomic tree generated with UBCG2 (Kim J, et al., 2021) of the strains belonging to the class Alphaproteobacteria and their closest neighbors according to the TYGS (Type Strain Genome Server). The strains characterized in this study are highlighted in bold. Accession numbers for these genomes can be found in Supplementary Table S5. The numbers at the nodes indicate the gene support index, with 81 being the maximum value.
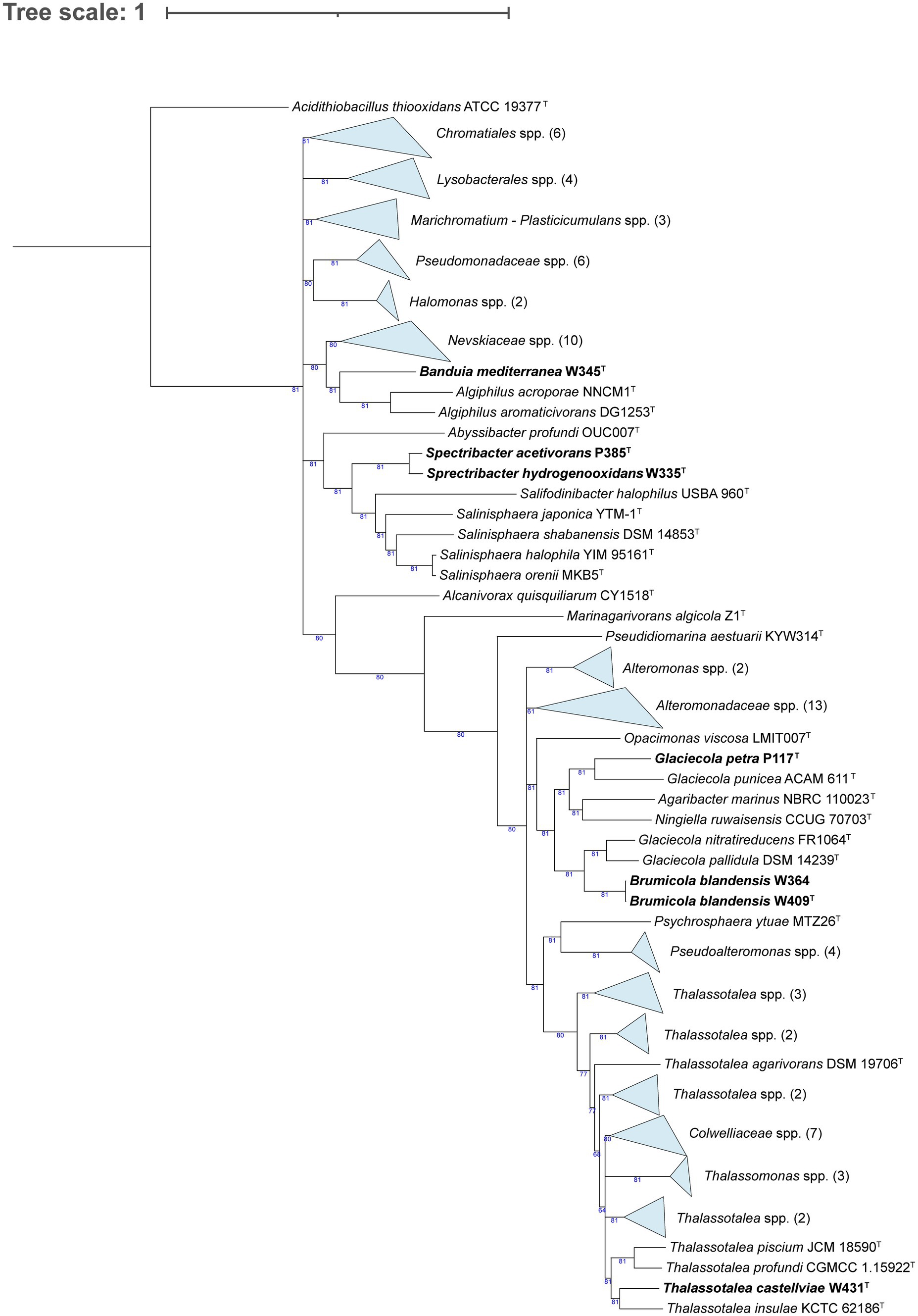
Figure 2. Phylogenomic tree generated with UBCG2 (Kim J, et al., 2021) of the strains belonging to the class Gammaproteobacteria and their closest neighbors according to the TYGS (Type Strain Genome Server). The strains characterized in this study are highlighted in bold. Accession numbers for these genomes can be found in Supplementary Table S5. The numbers at the nodes indicate the gene support index, with 81 being the maximum value.
In the Flavobacteriaceae tree (Figure 3), strain W242T comprised a branch close to a node containing two Patiriisocius species. Strain P050T groups with Urechidicola croceus LBP0138T. Strain W332T was placed in a node that contained 12 Winogradskyella species. In the case of strain S356T, it formed a single, long root inside a clade that included several Polaribacter and Tenacibaculum species. Strains F363T, F117T, F188T, F297T, F260T, and F225T formed a clearly differentiated group in the tree. Strains F388T, P007T, and P059 also grouped together, close to a root formed by Croceitalea dokdonensis DOKDO 023T. Finally, strain S334T was grouped with Pricia antarctica DSM 23421T. The last tree, formed by species of Rhodothermia (Figure 4), placed strains F394T and S365 in a node with Rubrivirga marina SAORIC-28T.
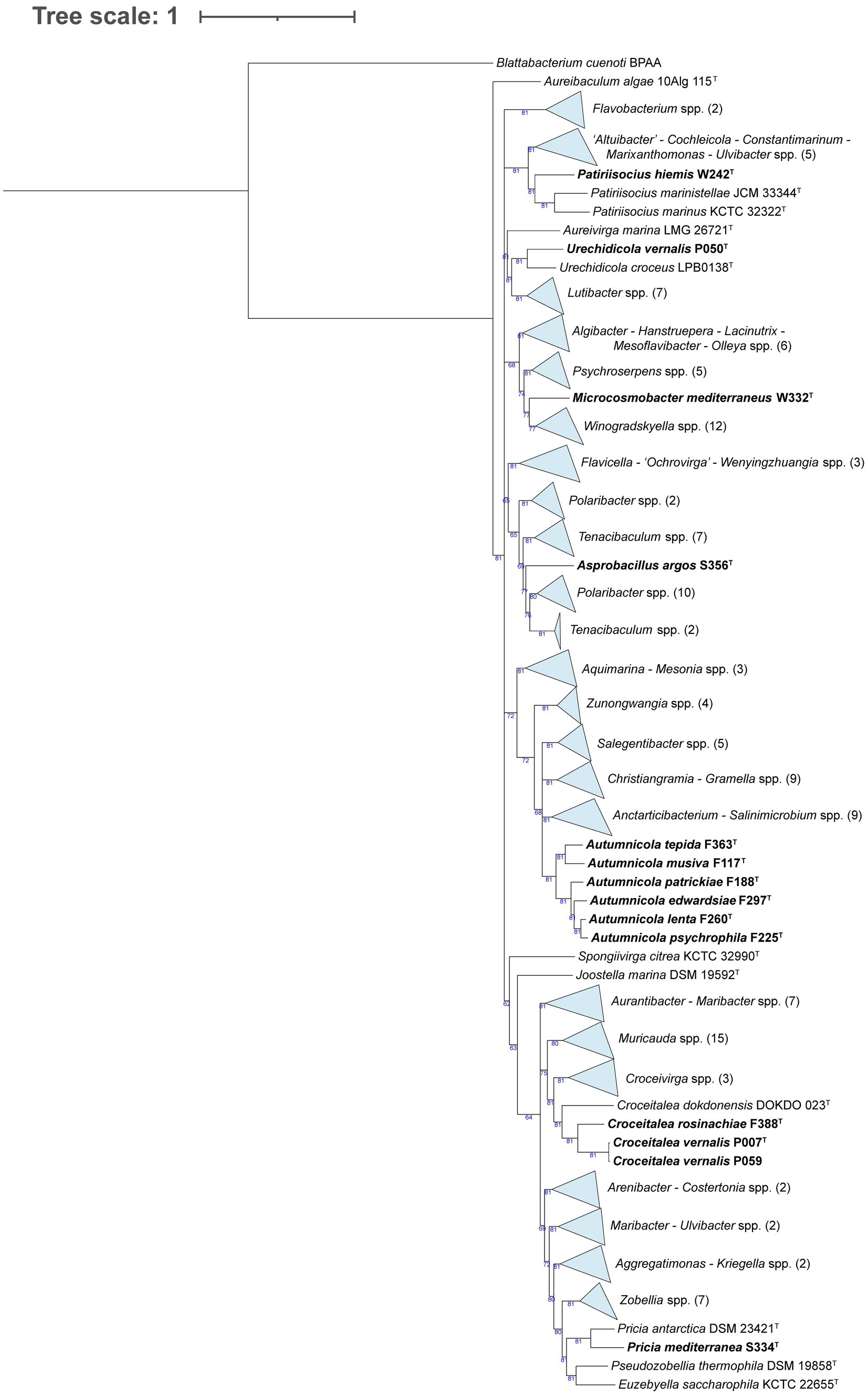
Figure 3. Phylogenomic tree generated with UBCG2 (Kim J, et al., 2021) of the strains belonging to the family Flavobacteriaceae and their closest neighbors according to the TYGS (Type Strain Genome Server). The strains characterized in this study are highlighted in bold. Accession numbers for these genomes can be found in Supplementary Table S5. The numbers at the nodes indicate the gene support index, with 81 being the maximum value.
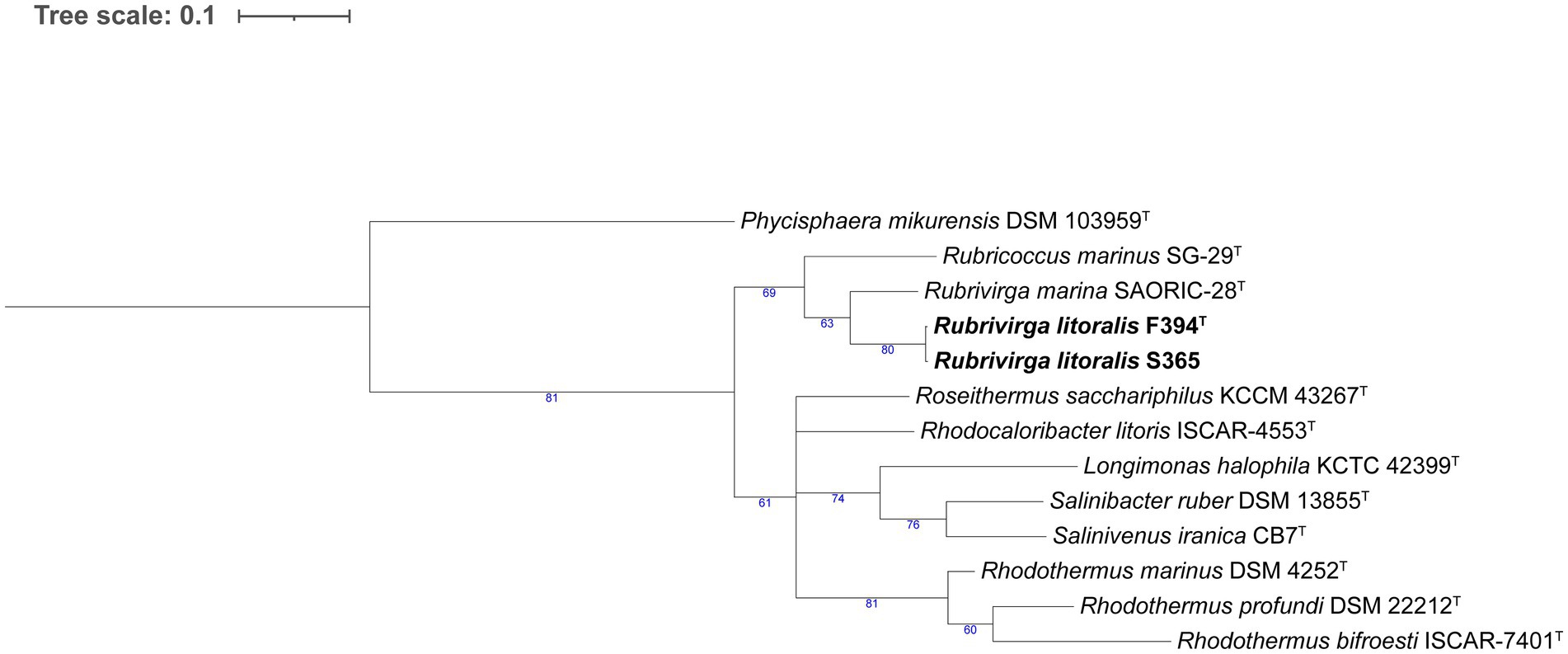
Figure 4. Phylogenomic tree generated with UBCG2 (Kim J, et al., 2021) of the strains belonging to the class Rhodothermia and their closest neighbors according to the TYGS (Type Strain Genome Server). The strains characterized in this study are highlighted in bold. Accession numbers for these genomes can be found in Supplementary Table S5. The numbers at the nodes indicate the gene support index, with 81 being the maximum value.
3.3 Physiology and chemotaxonomy
All strains were Gram-negative, oxidase-positive, and catalase-positive, except for P050T, which was catalase-negative. Flexirubin pigmentation was not detected in any of the strains. The isolates varied in their time of growth to colony detection and maximal biomass density in MA; those pertaining to the Alteromonadaceae family were the most reactive, taking 1–2 days to create visible colonies. They were followed by Flavobacteriaceae strains W242T, F363T, F117T, F188T, F297T, F225T, and S334T; and Alphaproteobaceria strains F158T and W311T, which presented a growth time to detection of 2–4 days. Strains F388T, P007T, and P059 (closely related to Croceitalea species) took 4 days to grow and had short viabilities. Strain P117T also took around 4 days to grow, always in the form of very solid, stone-like colonies, and also presented a short viability. The slowest strains, taking 6–7 days to grow, were the Flavobacteriaceae P050T, W332T, S356T, and F260T; the Alphaproteobacteria F390T; the Gammaproteobacteria W345T, P385T, and W335T; and the Rhodothermia F394T and S365. Strain W345T formed small, flat, and transparent colonies after 6–7 days of growth, but the colonies became bigger, more mucous, and opaque-white over time, while isolates W335T and P385T presented small, transparent, and very thin colonies. Strains F394T and S365 also resulted in small colonies. All the strains could grow in mR2A except F388T, P007T, P059, P050T, W332T (Flavobacteriaceae), P117T (Gammaproteobacteria), F394T, and S365 (Rhodothermia).
The main phenotypic characteristics of the strains used in this study are presented in Table 2. Notably, strains F188T, F225T, W364, W409T, and W431T grew at 4°C. All strains except F388T, W332T, and P117T grew at 15°C, and all of them grew at 28°C. At 37°C, the results were negative for some Flavobacteriaceae and Alteromonadaceae strains, and at 40°C, only strain F363T presented growth. None of the strains grew in 0% (w/v) sea salts or in 2% (w/v) NaCl as the only salt. Some isolates had a broad salinity range: F158T, F188T, F297T, F260T, F225T (closely related among them), S356T, and P385T. On the contrary, strains P059, P050T, P117T, and W332T only grew in 3–4% (w/v) sea salts, 3–5% in the case of P007T, and 1.5–3% for S356T. Additionally, isolates W311T, F390T, F363T, F117T, S334T, W345T, and W335T could grow down to 0.5% (w/v) sea salts, but only W335T grew to more than 6%. Strains F394T and S365 (which are closely related to Rubrivirga) differed in their salt preference: while strain F394T thrived in 3–6% (w/v) sea salts, strain S365 preferred 1.5–3%.
Interestingly, strains F388T, P007T, and P059 showed contrasting results in API ZYM strips: esterase activity was found only in P007T, and α-glucosidase activity was found in F388T and P007T, but not in P059T. API 20NE assimilation assays were considered positive even when low turbidity was observed. Notably, strains F394T, S365 (Rhodothermia), and W431T (related to Thalassotalea species) showed nitrite reductase activity. None of the strains showed glucose fermentation or urease activity. Aesculin hydrolysis was seen in most of the strains, and a few isolates hydrolyzed gelatin.
Most of the isolates presented in this study had weak or no growth in the positive control for carbon sources in a modified Basal Medium (Supplementary Table S6), making it challenging to reach conclusions based on this assay. F158T was the strain that assimilated most carbon sources (28 out of 43, comprising sugars, organic acids, and amino acids). Aside from that, strains F363T, F117T, and W311T showed a preference for sugars, while the Gammaproteobacteria isolates seemed to prefer organic acids and amino acids, although sometimes with weak growth or unclear results. L-leucine was the only compound where no growth by any of the strains could be detected.
The only polar lipid predicted from the genomes of all Flavobacteriaceae was phosphatidylethanolamine (PE), except strain S334T, which also contained diphosphatidylglycerol (DPG; Supplementary Table S7). All Gammaproteobacteria strains presented phosphatidylglycerol (PG) and PE, and W345T, P385T, and W335T genomes also contained DPG. All Alphaproteobacteria strains had enzymes for DPG and PG synthesis. Apart from these, strain F158T contained phosphatidylcholine (PC), strain F390T had PE and phosphatidyl m-inositol (PI), and strain W311T contained PI. Both Rhodothermia genomes present PE and PG synthesis enzymes. As for their respiratory quinones, all Flavobacteriaceae strains presented menaquinone 6 synthesis enzyme in their genomes; Gammaproteobacteria isolates had ubiquinone 8; Alphaproteobacteria possessed ubiquinone 10; and Rhodothermia genomes had menaquinone 7. A screening of gliding motility genes (gld) also indicated that most Flavobacteriaceae genomes contained all the gld genes, yet strains F388T, P007T, and P059 lacked gldI, while P050T, W332T, and S356T lacked gldI and gldH (Supplementary Table S7). In addition, all Gammaproteobacteria strains except W364 and W409T contained gldF and gldA. No gld genes were found in the Alphaproteobacteria or Rhodothermia genomes.
3.4 Biogeochemical relevance
Several genes involved in biogeochemical processes in the cycles of carbon, nitrogen, sulfur, phosphorus, iron, and hydrogen were present among the strains (Figure 5). Concerning the carbon cycle, most characterized strains possessed carbon-monoxide dehydrogenase (coxL), which would permit them to use CO as an energy source. The only exceptions are strains P117T, W242T, and W332T. Interestingly, the closely related strains P385T and W335T carried the genes rbcL and rbcS, which codify for the two subunits of the RuBisCO enzyme, a marker of carbon fixation. Strains P117T (affiliated to Glaciecola), F388T, P007T, P059 (affiliated to Croceitalea), and S365 (affiliated to Rubrivirga) presented proteorhodopsin (prd), indicating that these strains can use light as a complementary energy source. Some Gammaproteobacteria (those affiliating with Alteromonadaceae family) and Flavobacteriaceae (strains W332T, S356T, F388T, P007T, and P059) presented alginate-degrading genes.
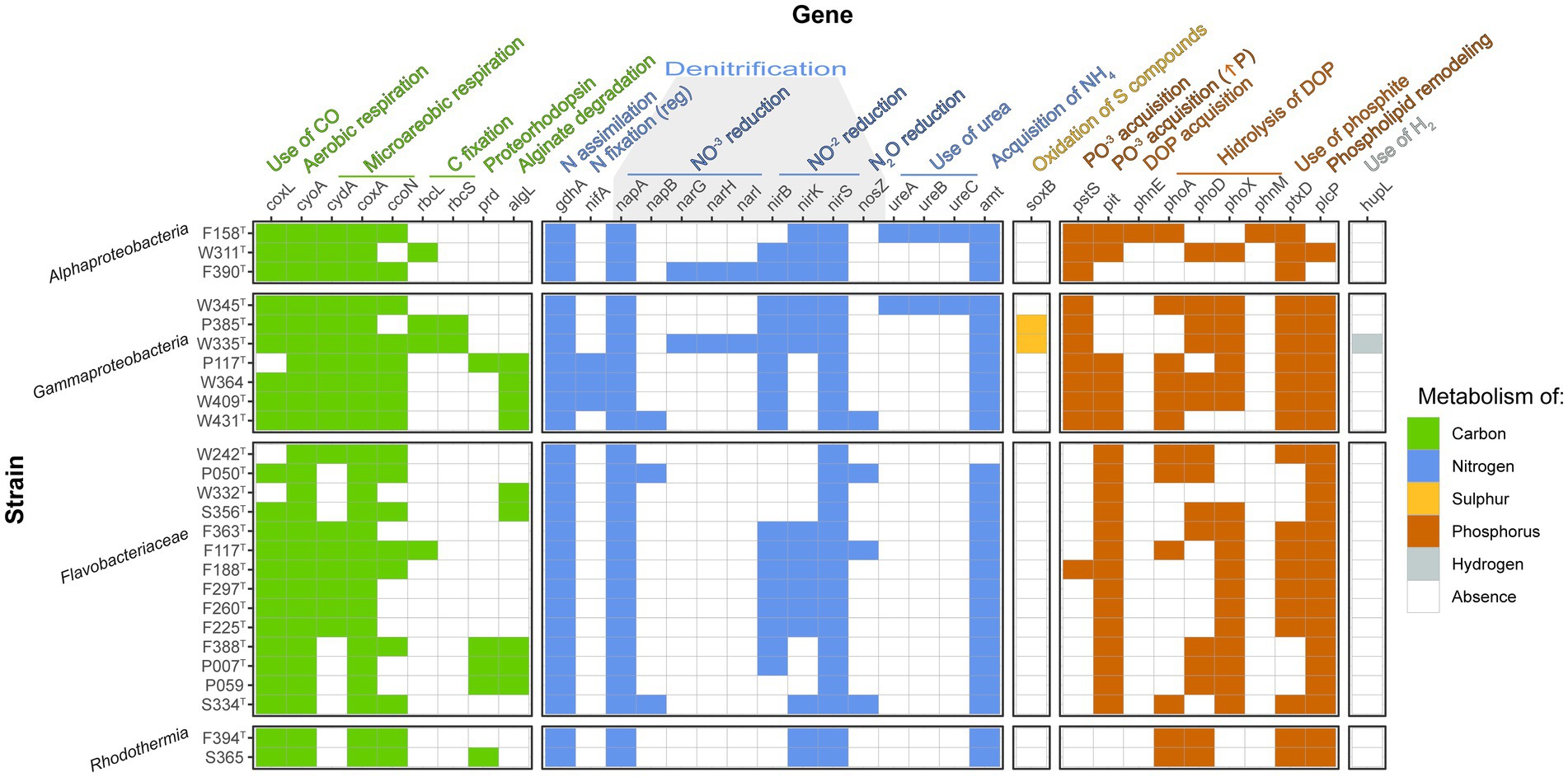
Figure 5. Heatmap indicating the presence/absence of genes relevant to biogeochemical cycles among the novel genomes. Only the genes with at least one positive value are shown.
The gdhA gene encoding glutamate 4ehydrogenase was found in all the strains, while the nifA gene for regulation of nitrogen fixation was detected in the three strains related to Glaciecola (P117T, W364, and W409T), but none of them could be classified as nitrogen fixers since no other nif gene was found. In addition, no genes implied by nitrification were found. We did find several genes related to denitrification in the genomes, but none of them contained the complete pathway. There were, however, three strains that presented the two subunits of the periplasmic nitrate reductase (W431T, P050T, and S334T), two strains that contained the three subunits of the membrane nitrate reductase (F390T and W335T), all strains included at least one nitrite reductase, and four strains (W431T, F117T, P050T, and S334T) contained the nosZ gene codifying for nitrous-oxide reductase. Three subunits of urease were found in strains F158T and W345T, implying that they could use urea as a nitrogen source. Strain W242T seemed to lack the amt ammonium transporter.
Additionally, strains P385T and W335T (closely related Gammaproteobacteria) presented the soxB gene in their genomes and therefore could use sulfur compounds as an energy source. While all Alphaproteobacteria and Gammaproteobacteria strains presented the inorganic phosphate transport pstS gene, Flavobacteriaceae strains had instead the pit gene for acquisition of inorganic phosphate when phosphorus is in high concentrations (F188T had both genes), and the Rhodothermia strains had none. Strain F158T was the only one which presented the phosphonate transport system gene phnE. Most genomes contained at least one alkaline phosphatase gene (phoA, phoD, and phoX), while the ones that lacked them all were strains F390T and W332T. In addition, strains F188T, F297T, F260T, and F225T only presented alkaline phosphatase phoX in their genomes. F158T was the only strain where phnM was detected. All strains had phosphite dehydrogenase ptxD, except P050T, W332T, S356T, F388T, P007T, and P059. Additionally, all strains but F158T and F390T had the plcP gene for phospholipid remodeling under low phosphorus availability. Interestingly, strain W335T presented the NiFe hydrogenase hupL, which would confer the ability to oxidize hydrogen to obtain energy.
3.5 Biogeography
We mapped our genomes to the metagenomic reads sampled by the Tara Oceans expedition to generate a general picture of their presence in different areas and depths of the global oceans. Most of the novel strains here presented had medium relative contribution (0.1–1% of the reads) to the community in various ocean regions (Figure 6), especially the Flavobacteriaceae strains (except for S334T, which seemed to be rarer) and Gammaproteobacteria strains P117T and W431T. Notably, strain S356T reached a relatively high abundance (1.09%) in the Southern Ocean’s surface waters. The rarest strains, which did not surpass the rare biosphere threshold (<0.1% reads) in any region, were W364, W409T (closely related strains from Alteromonadaceae family), F394T, and S365 (pertaining to the same species of Rubrivirga).
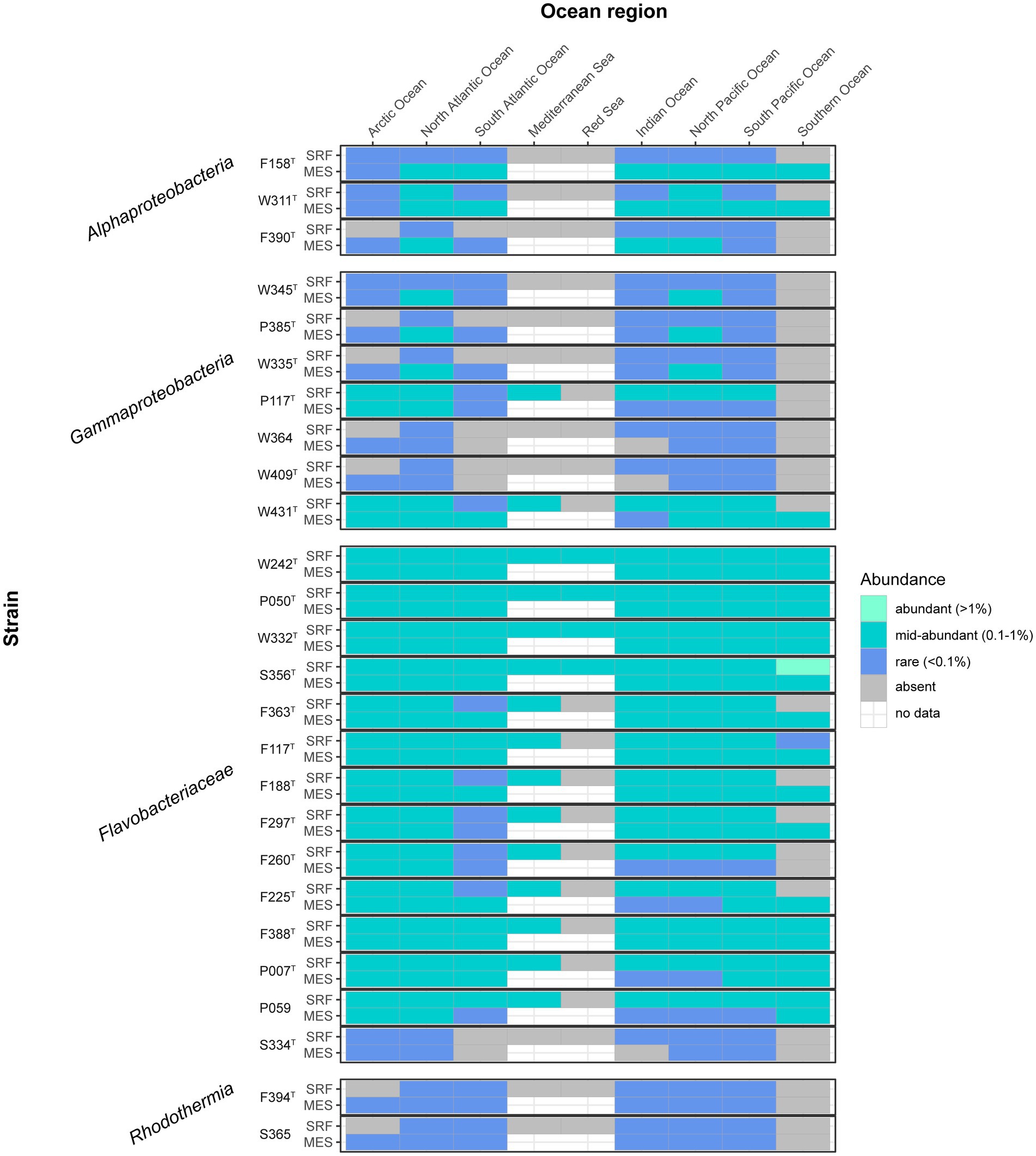
Figure 6. Heatmap indicating the abundance of the described strains in different oceans and layers. The gray color indicates absence, and the white color indicates no data. Data obtained by mapping the genomes to the Tara Oceans metagenomes. SRF, surface; MES, mesopelagic.
As a whole, Flavobacteriaceae strains represented the most widespread and abundant group (they recruited up to 9.49% of the reads in the South Pacific Ocean’s DCM and represented 3.41 ± 1.89% of the total Tara Oceans reads), followed by Gammaproteobacteria, which were frequently more abundant in the mesopelagic (Figure 7), and had a maximum contribution of 2.08% of the reads in the South Pacific Ocean’s DCM and represented 0.46 ± 0.42% of the total dataset. Alphaproteobacteria strains, which summed 0.19 ± 0.29% of the total reads, were also present in most oceans with significant abundances, although the number of samples where they were detected was lower than the previous two groups. They contributed more to the mesopelagic, recruiting up to 1.32% of the reads in the Indian Ocean. The Rhodothermia strains, which represented only 0.03 ± 0.08% of the Tara Oceans reads, were the less abundant, and they were detected in fewer samples, but they were still present in all ocean regions except the Mediterranean and Red Seas and the Southern Ocean.
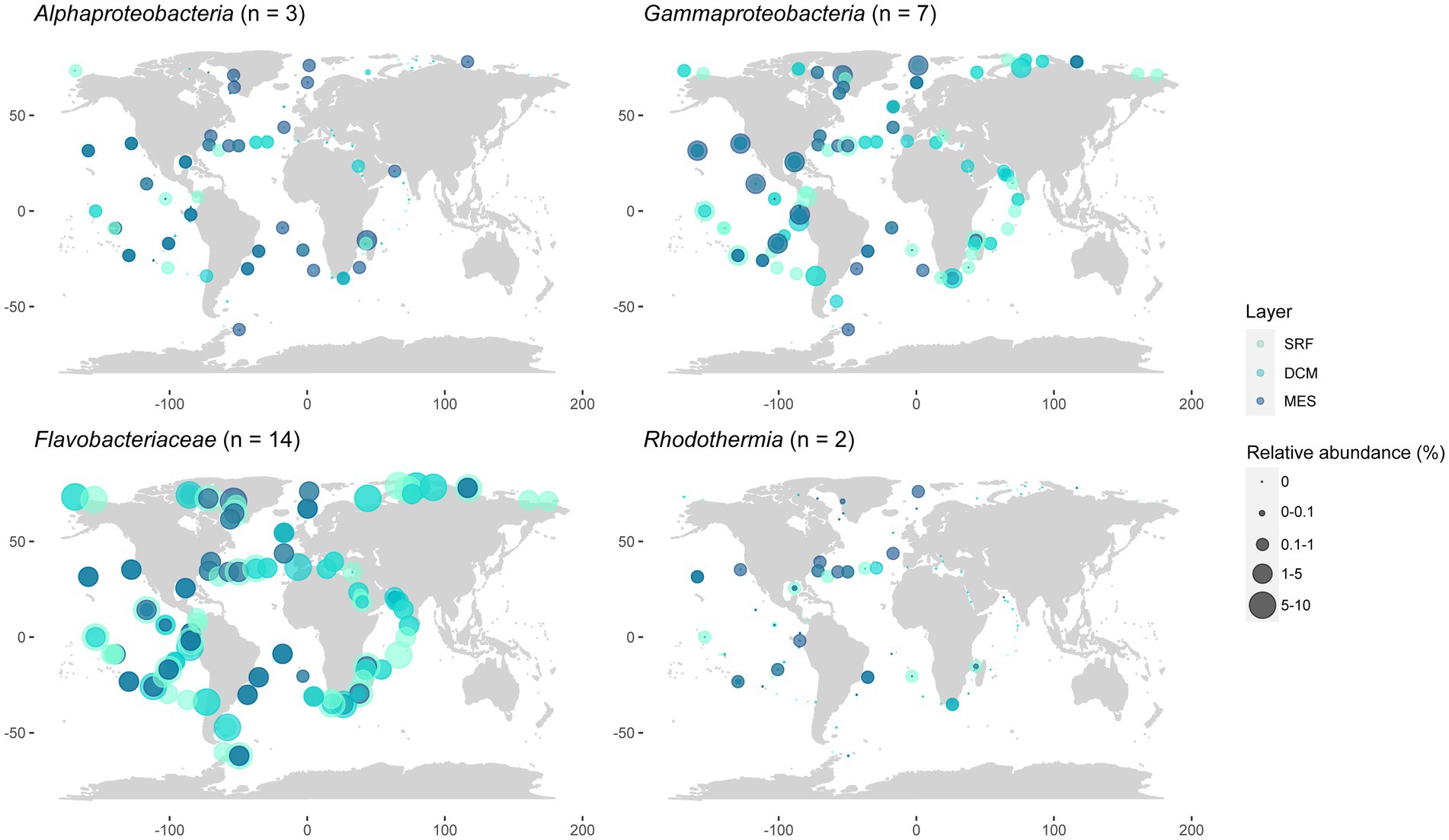
Figure 7. Geographic distribution of the presented strains grouped by class, taking into account different ocean layers. Data matched to the Tara Oceans metagenomes. SRF, surface; DCM, deep chlorophyll maximum; MES, mesopelagic.
4 Discussion
We compared the phenotypic and genomic characteristics of the novel strains described above to those of their closest relatives in order to resolve their taxonomic classification. None of the new strains had >95% ANI nor >70% dDDH compared to their closest relatives, which confirms their novelty according to the commonly established thresholds (Meier-Kolthoff et al., 2013; Jain et al., 2018). However, there are three pairs of strains that score > 95% ANI between them. Thus, the 26 strains characterized here represent 23 novel marine bacterial species from four different classes: Alphaproteobacteria, Gammaproteobacteria, Bacteroidia (family Flavobacteriaceae), and Rhodothermia.
4.1 Differential characteristics of the Alphaproteobacteria strains
Strain F158T has Tropicimonas isoalkanivorans DSM 19548T and Tropicimonas sediminicola DSM 29339T as its closest neighbors (Figure 1). T. isoalkanivorans is the type species of genus Tropicimonas (Harwati et al., 2009). Their DNA G + C content is similar: strain F158T has 66.9 mol%, T. isoalkanivorans presents 66.5 mol%, and T. sediminicola has 68.5 mol%. They have the same major polar lipids (Supplementary Table S8), but while strain F158T and T. sediminicola (Shin et al., 2012) are catalase positive and non-motile, T. isoalkanivorans is catalase negative and motile. The temperature and salinity ranges also differ: strain F158T grows between 15°C and 37°C and has a broad salinity range (0.5–12% (w/v) sea salts) but cannot grow without salts, T. sediminicola grows at 15–42°C and can thrive in 0–8% (w/v) NaCl, and T. isoalkanivorans grows between 10–46°C and 1–6% (w/v) NaCl. Taking into account the low AAI values that strain F158T has with its closest neighbors (67.06% with T. isoalkanivorans DSM 19548T and 66.82% with T. sediminicola DSM 29339T) and that they are distinct in several phenotypic traits (Supplementary Table S8), it could be considered that this strain represents a novel genus (Nicholson et al., 2020). However, given their placement in the phylogenomic tree, the fact that this genus is already phenotypically diverse, and that they do share some core characteristics (major respiratory quinones, major polar lipids, a highly similar G + C content, and several common substrates), we propose for strain F158T a novel species with the name Tropicimonas omnivorans.
Strain W311T has Stakelama marina LXI357T as its closest neighbor and is also close to Stakelama pacifica DSM 25059T (Figure 1), the type species of genus Stakelama (Chen et al., 2010). The three strains have very similar G + C content: W311T has 65.8 mol%, S. pacifica 66 mol%, and S. marina 64.1 mol% (Wang et al., 2023), but they present some differences in their major polar lipids (Supplementary Table S8). While W311T and S. marina are non-motile, S. pacifica presents motility. Their temperature and salinity ranges are similar: strain W311T survives from 15°C to 37°C and in 0.5–4% (w/v) sea salts, S. pacifica does so in 5–37°C and 0–5% (w/v) NaCl, and S. marina is the most different one, growing between 20–45°C and 0.5–11% (w/v) NaCl. Taking into account these traits, their common and differential assimilated carbon sources and enzymatic activities (Supplementary Table S8), the topology of the phylogenomic tree, and the relatively high AAI values between these strains, especially for S. marina LXI357T (78.8% AAI), we propose for strain W311T a novel species with the name Stakelama saccharophila.
Strain F390T groups close to Croceicoccus hydrothermalis JLT1T in the same clade as the type species, Croceicoccus marinus (Xu et al., 2009; Figure 1). The G + C content is more similar between strain F390T (60.7 mol%) and C. hydrothermalis (63.2 mol%; Chen et al., 2022) than with C. marinus (71.5 mol%), and the same trend applies for their polar lipids (Supplementary Table S8). Contrary to strain F390T and C. hydrothermalis, C. marinus is motile. Temperature ranges for C. hydrothermalis (5–45°C) and C. marinus (4–42°C) are broader than for strain F390T (15–28°C). Similarly, strain F390T thrives in 0.5–5% (w/v) sea salts, but C. hydrothermalis does from 0 to 12% (w/v) NaCl, and C. marinus grows in 0–10% (w/v) NaCl. Only strain F390T is oxidase positive. In terms of carbon source utilization and enzymatic activities, strain F390T is more similar to C. marinus than to C. hydrothermalis (Supplementary Table S8). Taking into account all these common and differential characteristics, the topology of the phylogenomic tree (Figure 1), and the AAI values (74.11% against C. hydrothermalis JLT1T and 70.21% against C. marinus E4A9T), we propose for strain F390T a novel species with the name Croceicoccus esteveae.
4.2 Differential characteristics of the Gammaproteobacteria strains
Strain W345T shares a node with Algiphilus acroporae NNCM1T and Algiphilus aromaticivorans DG1253T (Figure 2). The G + C content of strain W345T (64 mol%), A. acroporae (63.3 mol%; Sun et al., 2022), and A. aromaticivorans (63.6 mol%; Gutierrez et al., 2012) is quite similar. The main polar lipids of the three strains are mostly the same (Supplementary Table S8). We did not observe motility in strain W345T, but the two Algiphilus species are described as motile. Temperature ranges are similar: strain W345T grows at 15–37°C, A. acroporae grows between 18°C and 37°C, and A. aromaticivorans has a broader range: from 4°C to 37°C. While strain W345T survives between 0.5 and 5% (w/v) sea salts, with a weak growth in 6%, A. acroporae grows in 0.5–12% (w/v) NaCl, and A. aromaticivorans does so from 0 to 12% (w/v) NaCl, and thus, it does not require salts for growth. These strains have certain similarities but are quite different in terms of their substrate utilization and extracellular hydrolyses (Supplementary Table S8). In view of these facts and the low AAI between strain W345T and A. acroporae NNCM1T (61.31%) and A. aromaticivorans DG1253T (60.33%), we propose for strain W345T a novel genus and species with the name Banduia mediterranea.
Strains P385T and W335T group together in the phylogenomic tree (Figure 3) and have Salinisphaera orenii MKB5T and Salinisphaera halophila YIM 95161T as their closest neighbors in terms of AAI. Strains P385T (65.7 mol%) and W335T (65.6 mol%) have almost identical G + C content, while S. halophila (69.5 mol%; Zhang et al., 2012) and S. orenii (63.5 mol%; Park et al., 2012) flank them on the upper and lower side, respectively. Strains P385T and W335T have the same major polar lipids and are oxidase positive, but, in contrast, S. halophila contains PC and is oxidase negative (Supplementary Table S8). While we did not observe motility in strains P385T or W335T, both Salinisphaera spp. are motile. The temperature ranges of the four strains are quite similar; strains P385T and W335T grow at 15–37°C, S. halophila at 15–40°C, and S. orenii has the broadest range at 10–40°C. In terms of salinity ranges, strains P385T (1.5–11% w/v sea salts with weak results in 1%) and W335T (0.5–8% w/v salts with weak growth in 9 and 10%) have a much lower tolerance than S. halophila (5–29% w/v NaCl) and S. orenii (5–25% w/v NaCl). Strains P385T and W335T share most enzymatic activities and carbon sources, but they present several differences with S. orenii (especially) and S. halophila (Supplementary Table S8). Considering these characteristics, the low AAI values (around 64%) that both strains share with their closest neighbors (Supplementary Table S5), and the topology of the phylogenomic tree, we propose for strains P385T and W335T a new genus and two novel species with the names Spectribacter acetivorans and Spectribacter hydrogenooxidans, respectively.
Strain P117T clusters with the type species of genus Glaciecola, G. punicea ACAM 611T (Bowman et al., 1998), and has Ningiella ruwaisensis CCUG 70703T as its second closest strain (Figure 2). While strain P117T has 39.9 mol% G + C, G. punicea contains 45.1 mol% (Bowman et al., 1998), and N. ruwaisensis has 43.2 mol% (Fotedar et al., 2020). Contrary to strain P117T, G. punicea and N. ruwaisensis are motile. The temperature ranges of strain P117T and G. punicea are similar: 15–28°C and 15–25°C, respectively, but N. ruwaisensis has a broader range (15–45°C). The salinity range of strain P117T is quite narrow (3–4% (w/v) sea salts), while N. ruwaisensis develops in 0–6% (w/v) NaCl. Strain P117T has more common carbon sources and enzymatic activities with G. punicea than with N. ruwaisensis (Supplementary Table S8). Strain P117T presents an AAI of 70.92% with G. punicea ACAM 611T and 67.84% with N. ruwaisensis CCUG 70703T. With these values, strain P117T could be proposed as a novel genus, but considering that in the phylogenomic tree (Figure 2) this strain clearly clusters with G. punicea, the type species of the genus Glaciecola, and in the absence of the type strain genome for some species of the genus to better assess its taxonomic status, we propose for strain P117T a new species of the genus Glaciecola with the name Glaciecola petra.
In the same section of the tree (Figure 2), we find strains W364 and W409T, which share a node with Glaciecola nitratireducens FR1064T and G. pallidula DSM 14239T. Strains W364 and W409T have 99.25% ANI between them, and thus, they belong to the same species (Jain et al., 2018). Both strains present 42.6 mol% G + C, while G. nitratireducens (Baik et al., 2006) has 44 mol% and G. pallidula (Bowman et al., 1998) contains 40 mol%. While strain W364, G. nitratireducens, and G. pallidula are motile, we did not observe this in strain W409T. The temperature range of the novel strains is 4–37°C, although strain W364 presented weak growth at that maximum temperature. In contrast, G. nitratireducens grows between 15°C and 30°C, and G. pallidula develops at 10–20°C. Strains W364, W409T, and G. nitratireducens do so in 2–9% (w/v) sea salts. Most carbon sources and enzymatic activities displayed by strains W364 and W409T are the same, and they are partly shared with G. nitratireducens and G. pallidula, with certain differences (Supplementary Table S8). Looking at the phylogenomic tree (Figure 2), it is noteworthy that G. nitratireducens and G. pallidula cluster with strains W364 and W409T rather than with G. punicea, the type species of the genus Glaciecola. Moreover, Agaribacter marinus and Ningiella ruwaisensis are closer to G. punicea, making a polyphyletic branch. We wanted to explore this in more detail, using the Kostas Lab ANI/AAI matrix tool (Rodriguez-R and Konstantinidis, 2016) to compute an AAI matrix with all these strains and P117T. The matrix showed AAI values of 64–65% when comparing G. punicea ACAM 611T to G. nitratireducens FR1064T and G. pallidula DSM 14239T, while these two had 73–74% AAI with strains W364 and W409T (Supplementary Figure S1). Strains W364 and W409T also have a low AAI (63%) with G. punicea ACAM 611T. In view of all these data, we propose the reclassification of Glaciecola pallidula and Glaciecola nitratireducens into a new genus, resulting in the new combinations Brumicola pallidula and Brumicola nitratireducens, and for strain W409T, a new species with the name Brumicola blandensis.
At the bottom of the phylogenomic tree (Figure 2), we observe strain W431T clustering with Thalassotalea insulae KCTC 62186T and, more distantly, with the type species of the genus, T. ganghwensis (Zhang et al., 2014). Strain W431T has a lower G + C (37.2 mol%) than T. insulae (41.3 mol%; Park et al., 2018) and T. ganghwensis (42 mol%). The temperature ranges of strain W431T (4–37°C), T. insulae (10–37°C), and T. ganghwensis (15–40°C) are quite similar. The salinity ranges of strain W431T and T. ganghwensis are almost identical (1.5–8% (w/v) sea salts and 1–8% (w/v) NaCl, respectively), while T. insulae can grow without salt and up to 6% (w/v) NaCl. Strain W431T displays certain differences with T. insulae and T. ganghwensis in terms of assimilation of carbon sources and enzymatic activities (Supplementary Table S8). With this information, the phylogenomic tree that clearly places W431T within the Thalassotalea species (Figure 2) and the AAI values (Supplementary Table S5), we propose for strain W431T a new species with the name Thalassotalea castellviae.
4.3 Differential characteristics of the Flavobacteriaceae strains
As it is usual in the Flavobacteriaceae (Bernadet et al., 2002), all novel strains from that family had PE as their major polar lipid, menaquinone 6 as their predominant respiratory quinone, and are non-motile. In addition, they did not present flexirubin-type pigments, which are common in marine strains from this family (Bernadet et al., 2002). Thus, unless there is a difference between the novel strains and those used for the comparisons, these characteristics will not be mentioned below.
Strain W242T is placed close to Patiriisocius marinistellae JCM 33344T and P. marinus KCTC 32322T in the phylogenomic tree (Figure 3). They are the only two characterized species of that recent genus, P. marinistellae, its type species (Kawano et al., 2020). The three strains have very similar G + C content: 33.7 mol% for W242T, 33.4 mol% for P. marinistellae (Kawano et al., 2020), and 33.3 mol% for P. marinus. Strain W242T (15–37°C), P. marinistellae (4–30°C), and P. marinus (4–35°C) have relatively similar temperature ranges, and while strain W242T develops in 2–8% (w/v) sea salts, the others do it in 0.5–5% (w/v) NaCl. Although strain W242T differs in several carbon sources and enzymatic activities from P. marinus and P. marinistellae (Supplementary Table S8), in view of their clear placement in the phylogenomic tree (Figure 3), and also considering the AAI values (Supplementary Table S5), we propose for strain W242T a new species with the name Patiriisocius hiemis.
Strain P050T clearly clusters with Urechidicola croceus (Figure 3), the only species of that genus to date (Shin and Yi, 2020). Strain P050T has a higher G + C content (33.5 mol%) than U. croceus (30.4 mol%). Their temperature ranges are almost identical: 4–28°C for strain P050T and 4–28°C for U. croceus, and while strain P050T has a narrow salinity range (only 3–4% (w/v) sea salts), U. croceus can grow between 1 and 5% (w/v) NaCl. Strain P050T is oxidase-positive and catalase-negative, while U. croceus presents the opposite profile. Both strains coincide in their capacity to hydrolyze DNA and their negative results in indole production and citrate assimilation in API 20NE. However, strain P050T was also positive (in API 20NE strips but not on agar plates) in the assimilation of several compounds for which U. croceus gave negative results (Supplementary Table S8). Another difference is that U. croceus reduces nitrate to nitrite, but strain P050T does not. In view of these data, the AAI values in Supplementary Table S5, and the topology of the phylogenomic tree, we propose for strain P050T a new species with the name Urechidicola vernalis.
Following the Flavobacteriaceae phylogenomic tree, strain W332T shares a node with 12 Winogradskyella species, from which it is separated by a relatively long branch (Figure 3). It is close to W. echinorum but also quite close to the type species W. thalassocola (Nedashkovskaya et al., 2005). Strain W332T has 32.5 mol% G + C, W. thalassocola presents 34.6 mol%, and W. echinorum has 33.6 mol% (Nedashkovskaya et al., 2009). While the temperature (28°C) and salinity ranges (3–4% (w/v) sea salts) of strain W332T are pretty narrow, W. thalassocola develops at 4–33°C and 1–8% (w/v) NaCl, and W. echinorum grows at 4–37°C and in 1–6% (w/v) NaCl. For those characteristics for which data exists, some differences between strain W332T, W. echinorum, and W. thalassocola in terms of assimilated carbon sources and enzymatic activities could be identified (Supplementary Table S8). Considering that strain W332T is relatively distant to its closest Winogradskyella neighbor (72.62% AAI to W. echinorum; Supplementary Table S5) and that it is differentiated from all the Winogradskyella strains by a long branch in the phylogenomic tree (Figure 3), we propose for strain W332T a new genus and species with the name Microcosmobacter mediterraneus.
Strain S356T lies between several Tenacibaculum and Polaribacter spp., with Polaribacter septentrionalilitoris ANORD1T and P. pectinis L12M9T as its closest neighbors. Strain S356T has a G + C content (35.2 mol%) more similar to P. pectinis (36.4 mol%) than to P. septentrionalilitoris (30.6 mol%), and it has a narrow temperature range (15–28°C) compared to its relatives (4–25°C for P. pectinis, 0–45°C for P. septentrionalilitoris). While strain S356T grows only on 1.5–3% (w/v) sea salts, P. pectinis grows in 0.5–6% (w/v) NaCl and P. septentrionalilitoris in 2–10% (w/v) NaCl. P. pectinis and P. septentrionalilitoris assimilate more compounds and have more positive results for enzymatic activities than strain S356T (Supplementary Table S8). Given the evident phenotypic differences and the long branch formed by strain S356T on the phylogenomic tree (Figure 3), we explored the AAI values of this genome with its closest neighbors using the ANI/AAI matrix tool (Rodriguez-R and Konstantinidis, 2016). Supplementary Figure S2 shows that the AAI values between S356T and its closest strains range from 64.2 to 68.6%, evidencing that it belongs to a novel genus. The matrix also raises doubts about the classification of some species, such as Polaribacter pacificus or Tenacibaculum agarivorans, and those placed below in the table, but that observation is out of the scope of this study. Thus, we propose for strain S356T a new genus and species with the name Asprobacillus argos.
A series of our isolates (F363T, F117T, F188T, F297T, F260T, and F225T) were placed together in the phylogenomic tree in a clearly differentiated node (Figure 3). Their closest neighbors are the type strains of Salegentibacter mishustinae, S. agarivorans, and S. salarius. We also included in the comparison S. salegens, the type species of the genus (McCammon and Bowman, 2000). The G + C content of these strains (38.3–40 mol%) is similar to that of Salegentibacter spp. (37.5–41 mol%), and all of them are catalase and oxidase-positive. While S. mishustinae, S. agarivorans, and S. salarius can grow at 4°C, only strains F188T and F225T can. Strain F363T, S. agarivorans, and S. salarius grow up to 40–41°C. Notably, strains F297T and F260T have a narrower temperature range compared to the rest. Regarding salinity, all strains grow at low salt percentages, but Salegentibacter species seem to tolerate higher salt concentrations (up to 18–20% w/v NaCl) than the novel isolates (up to 11% w/v sea salts). Assimilated carbon sources and enzymatic activities vary between these strains (Supplementary Table S8). Considering these data, the fact that none of our isolates have an AAI >75% with any Salegentibacter strain (Supplementary Table S5), and, particularly, accounting for the topology of the phylogenomic tree (Figure 3), we propose for strains F117T, F188T, F225T, F260T, F297T, and F363T a new genus, Autumnicola, with six new species named: A. musiva, A. patrickiae, A. psychrophila, A. lenta, A. edwardsiae, and A. tepida, respectively. In addition to listing the main characteristics of the Autumnicola strains in Table 2 and Supplementary Table S8, we have made a drawing summarizing their roles in biogeochemical cycles (Supplementary Figure S3).
The next group of strains included F388T, P007T, and P059, which formed a node close to Croceitalea dokdonensis DOKDO 023T (Figure 3). The type species of the genus Croceitalea is C. eckloniae (Lee et al., 2008), which we include in the comparison. The G + C content of strains P007T and P059 is almost identical (34.7 and 34.6 mol%, respectively) and similar to strain F388T (36.4 mol%), but in the case of C. dokdonensis and C. eckloniae, it is much higher: 59.4 and 66.5 mol%, respectively. Strains F388T (28°C), P007T, and P059 (15–28°C) have a narrower temperature range than C. dokdonensis (12–38°C) and C. eckloniae (10–34°C). Salinity ranges are also narrow for these strains: none of them grows in less than 2% (w/v) sea salts nor in more than 5%. However, C. dokdonensis grows at 1–7% (w/v) sea salts, while C. eckloniae does at 0.5–7% (w/v) sea salts. While our isolates are oxidase-positive, C. dokdonensis and C. eckloniae are negative. Strains P007T and P059 share most of the tested carbon sources and enzymatic activities, and they are fairly similar in these terms to F388T but not to C. dokdonensis and C. eckloniae (Supplementary Table S8). Strains P007T and P059 have an ANI of 98.95% (Supplementary Table S4), therefore they pertain to the same species (Jain et al., 2018). Given the topology of the phylogenomic tree (Figure 3) and the AAI values of almost 76% that the novel strains have with C. dokdonensis DOKDO 023T (Supplementary Table S5), we propose two new species of the genus Croceitalea: C. rosinachiae for strain F388T and C. vernalis for strain P007T.
The last strain of the Flavobacteriaceae phylogenomic tree is S334T, which clusters with Pricia antarctica, the only species of the genus Pricia (Yu et al., 2012). Strain S334T has a higher G + C content (47 mol%) than P. antarctica (43.9 mol%). While strain S334T thrives at 15–28°C, P. antarctica can survive at lower temperatures (0–25°C). Their salinity ranges are similar: 0.5–5% (w/v) sea salts for strain S334T and 0.6–6% (w/v) NaCl for P. antarctica. Strain S334T seems to assimilate many more carbon sources and have a broader set of enzymatic activities than P. antarctica (Supplementary Table S8). Taking into account these common and differential traits, considering the topology of the phylogenomic tree (Figure 3) and the AAI value of 80.87% (Supplementary Table S5) between S334T and P. antartica DSM 2342T, we propose for strain S334T a new species of Pricia with the name Pricia mediterranea.
4.4 Differential characteristics of the Rhodothermia strains
Strains F394T and S365 appear close to Rubrivirga marina in the phylogenomic tree (Figure 4), which is the type species of the genus Rubrivirga (Park et al., 2013). We include in the comparison the only other species of that genus, R. profundi (Song et al., 2016), which was not included in the tree since the genome of its type strain is not available in public repositories. Strains F394T and S365 have a higher G + C content (73.7 mol%) than R. marina (64.8–65.8 mol%) and R. profundi (66.2 mol%). Strains F394T and S365 present PE and PG as their major polar lipids, but R. marina and R. profundi also include DPG. All of them display menaquinone 7 as their predominant respiratory quinone and do not contain flexirubin-type pigmentation. The temperature range of strains F394T and S365 is 15–37°C, R. marina survives from 10°C to 37°C, and R. profundi has a broader range (4–42°C). While strain F394T grows between 3 and 6% (w/v) sea salts, S365 does from 1.5 to 3% (w/v) sea salts, and R. marina and R. profundi develop between 1 and 5% (w/v) NaCl. All strains are oxidase and catalase positive except R. marina, which is catalase negative. Our strains have several differential results in API 20NE strip assimilation tests and present enzymatic profiles that diverge from R. marina and R. profundi (Supplementary Table S8). Interestingly, strains F394T and S365 reduced nitrate to nitrite, but R. profundi did not show this capacity, and R. marina has not been reported to do so. F394T and S365 have 98.88% ANI between them (Supplementary Table S4), and they are thus two strains of the same species (Jain et al., 2018). Taking into account all these data and the AAI values in Supplementary Table S5, we conclude that strain F394T is a new species of Rubrivirga, with the proposed name Rubrivirga mediterranea.
4.5 Ecological relevance of the new strains
The strains characterized in this study contain several genes with important roles in carbon, nitrogen, sulfur, phosphorus, and hydrogen cycling in the oceans (Figure 5). The gene coxL, which encodes for the large subunit of CO dehydrogenase, is found in almost all the strains. The capacity of obtaining energy from CO through its oxidation is widespread and has been proposed as a relevant mechanism for long-term survival of heterotrophic bacteria in oligotrophic environments (Cordero et al., 2019). Strains P385T and W335T, which have been characterized here as two members of the same novel genus, bear genes encoding for RuBisCO small and large subunits (genes rbc). They are, thus, potential important contributors to the carbon cycle via anaplerotic CO2 fixation (Newton et al., 2010). Interestingly, these two bacteria were isolated in winter and spring, and the rbcL gene was more abundant at the site of the study (Auladell et al., 2023). On the other hand, the prd gene (which encodes for proteorhodopsin) was found in strains P117T (Gammaproteobacteria), F388T, P007T, P059 (three Bacteroidia grouping into the same genus), and S365 (Rhodothermia). Proteorhodopsin allows bacteria to obtain energy from light (Beja et al., 2000), and it is a relevant gene for long-term survival in low-nutrient environments such as the BBMO (Gómez-Consarnau et al., 2010). Bacteroidia and Gammaproteobacteria have been reported to contain this proton pump (Beja et al., 2000; Yoshizawa et al., 2014).
Furthermore, the gene algL could be detected in strains P117T, W364, W409T, W431T (Alteromonadaceae), W332T, S356T (Flavobacteriaceae), F388T, P007T, and P059 (closely related Flavobacteriaceae). This gene encodes for alginate lyase, which catalyzes the degradation of alginate (Wong et al., 2000). Alginate is a complex polysaccharide mainly found in the walls of brown algae and constitutes an important marine carbon source (Mabeau and Kloareg, 1987). Bacteria that degrade complex algal polysaccharides can make them available for fast-growing opportunistic bacteria to grow and potentially develop bacterial blooms (Teeling et al., 2012). Despite the genomic predictions, strains W409T, W431T, and W332T were not seen to hydrolyze alginate experimentally, and W311T hydrolyzed it weakly (Table 2), although it was not predicted to do so. In fact, detecting a gene in a genome does not necessarily correlate with its expression in the environment.
All the strains characterized here contain the gdhA gene encoding for glutamate dehydrogenase. This enzyme allows N assimilation through NH4 at the cost of α-ketoglutarate, and its expression has been seen to be enhanced when the N/C ratio is high (Hoch et al., 2006), acting as a balance between the carbon and nitrogen cycles. As commented above, although we found the nifA gene involved in the regulation of nitrogen fixation (Lei et al., 1999), we did not find among the novel strains the most relevant marker genes encoding for the different nitrogenases implicated in nitrogen fixation (nifD, nifH, or nifK). Several of the strains had potential for partial denitrification, but only three of them were seen to experimentally reduce nitrate to nitrite (Table 2): W431T, which contains periplasmic nitrate reductase genes napA and napB, F394T and S365, for which no nitrate reduction genes were detected. All in all, several of the novel strains theoretically have the capacity to undergo partial denitrification, and we have confirmed the reduction of nitrate to nitrite in three of them, which makes them relevant members of the marine bacterial community in terms of the nitrogen cycle (Ferrera et al., 2015). Concerning other nitrogen compounds, although strains F158T and W345T contain the genes for the three subunits of urease, they gave negative results in API 20NE strips for this test (Table 2). In addition, strain W242T lacked the amt gene encoding for a widespread ammonium transporter (Alonso-Sáez et al., 2020), and it is thus likely that these bacteria acquire N by other means that were not tested here.
The only gene related to the sulfur cycle that was found in these new isolates was soxB, a marker for the oxidation of sulfur compounds (Meyer et al., 2007), in the two strains pertaining to the novel genus Spectribacter. The dmdA gene, involved in dimethyl sulfoxide (DMSP) oxidation and widespread in marine bacteria (Moran et al., 2012), was not found in the novel strains. Moreover, the pstS gene, which is the marker for a phosphate transport system, was found mainly in Alphaproteobacteria and Gammaproteobacteria. Precisely these two groups have been reported to contain this gene, which seems to lack seasonality in the BBMO (Auladell et al., 2023) and has been proposed to be a general P uptake mechanism (Orchard et al., 2009). On the contrary, the Flavobacteriaceae strains exclusively contained the pit gene (except for F188T, which had both pit and pstS). The pit gene expression is enhanced under high P conditions, and it is related to copiotrophic Gammaproteobacteria and Bacteroidia, which would use it to cover their high energy demands (Alonso-Sáez et al., 2020). Among the novel strains, this gene was also found in some Gammaproteobacteria (precisely the ones affiliating with the copiotrophic family Alteromonadaceae) and two out of three Alphaproteobacteria. Interestingly, no phosphate acquisition genes were found in the Rhodothermia strains.
The only strain that seems to be capable of acquiring dissolved organic phosphorus (DOP) via a phosphonate transport system encoded by phnE is F158T, pertaining to the Roseobacter clade. Marine bacteria often rely on DOP to meet their phosphorus requirements, and Rhodobacteraceae have been characterized as important members of marine DOP cycling (Lockwood et al., 2022). While the most classical alkaline phosphatase phoA was found in 12 of the novel genomes, the marine extracellular phosphatases phoD and phoX (Luo et al., 2009; Sebastian and Ammerman, 2009) were found in 15 and 18 strains, respectively. Another protein used for DOP hydrolysis, the alkylphosphonate utilization protein encoded by phnM, was found only in strain F158T. Rhodobacteraceae was one of the groups reported to contain this gene in the BBMO (Auladell et al., 2023). In addition, the ptxD gene was present in all the strains except for six of the Flavobacteriaceae, indicating that phosphite could be a relevant P source for these bacteria (Martínez et al., 2012). Phospholipid remodeling is an important strategy in P-depleted environments such as the Mediterranean Sea, especially among Flavobacteriaceae and during the summer (Auladell et al., 2023). In concordance with these observations, the plcP gene was found in all strains except two of them (both Alphaproteobacteria). Finally, there was one strain, W335T, which contained the hupL gene. Hydrogen has recently drawn the attention of researchers as a notable energy source for marine bacteria, especially in environments with low primary production (Lappan et al., 2023).
The novel strains seem to be widespread in the global ocean (Figures 6, 7) and frequently constitute notorious proportions of the bacterial community, especially those belonging to the Flavobacteriaceae. Surprisingly, some of the strains were not detected in the Mediterranean Sea, where they were actually isolated. It could be that they had very low abundances during the Tara Oceans sampling, that took place in November 2009, quite separated from the sampling that yielded the novel isolates, occurring during the whole year in 2017.
4.6 Concluding remarks
Here, we have presented the full characterization of 23 novel bacterial species and six novel genera with potential relevant roles in biogeochemical cycling and widespread presence throughout the oceans. This study points to the need of revising the taxonomy of the genera Glaciecola, Polaribacter, and Tenacibaculum. In this study, the combination of bacterial cultures with sequencing-based approaches has proven to be a powerful tool to unveil new members of the marine microbial community with hypothetical ecological relevance. More studies combining cultures with sequencing are needed to describe the high number of uncharacterized bacterial species in the environment.
4.7 Description of Tropicimonas omnivorans sp. nov.
Tropicimonas omnivorans (om.ni.vo’rans. L. neut. adj. omne, everything; L. inf. v. vorare, to devour; N.L. part. adj. omnivorans, eating everything).
Cells are rod-shaped, short and thicker by the sides, and non-motile. Colonies on Marine Agar are small, opaque, pink-colored, and non-mucous; they grow between 15°C and 37°C and between 0.5 and 12% (w/v) sea salts; they do not grow in 2% (w/v) NaCl as the only salt; they are Gram-negative, oxidase-positive, and catalase-positive; they hydrolyze starch weakly and do not hydrolyze casein, alginate, DNA, or cellulose; and they do not grow in medium containing Tween-80. Among the carbon sources tested on simplified Basal Medium Agar grows on D-ribose, D-xylose, L-arabinose, D-glucose, D-mannose, D-trehalose, L-rhamnose, maltose, cellobiose, lactose, sucrose, melibiose, N-acetylglucosamine, glycerol, D-mannitol, D-glucuronate, acetate, pyruvate, citrate, 2-ketoglutarate, succinate, fumarate, malate, lactate, L-serine, L-alanine, L-glutamate, L-aspartate, and GABA. In API ZYM, it is positive for alkaline phosphatase, leucine and valine arylamidases, acid phosphatase, and naphtol AS-BI-phosphohydrolase. In API 20NE, it is positive for the assimilation of glucose, arabinose, mannose, mannitol, N-acetylglucosamine, maltose, gluconate, caprate, adipate, and malate. The predicted major polar lipids are DPG, PG, and PC. The predicted main respiratory quinone is ubiquinone 10.
The type strain is F158T (= CECT 30792T = CCM 9343T), which was isolated from microcosm experiments made with seawater from Blanes Bay. The DNA G + C content of the strain is 66.9 mol%, and its genome size is 3.94 Mbp. The GenBank accession numbers for the whole genome and 16S rRNA gene sequences are GCA_031846565 and OP342988, respectively.
4.8 Description of Stakelama saccharophila sp. nov.
Stakelama saccharophila (sac.cha.ro’phi.la. Gr. neut. n. sakcharon, sugar; N.L. fem. adj. suff. -phila, friend, loving; from Gr. fem. adj. philê, loving; N.L. fem. adj. saccharophila, sugar-loving).
Cells are thick, short rods often found in pairs. Motility is not observed. Colonies on Marine Agar are small, circular, convex, translucent, non-mucous, and pale yellow in color; they grow between 15°C and 37°C and between 0.5 and 4% (w/v) sea salts; they do not grow in 2% (w/v) NaCl as the only salt; they are Gram-negative, oxidase-positive, and catalase-positive; they hydrolyze Tween-80 and alginate (weakly), but not casein, starch, DNA, or cellulose. Among the carbon sources tested on simplified Basal Medium Agar grows on D-xylose, L-arabinose, D-glucose, D-fructose, D-mannose, D-trehalose, L-rhamnose, maltose, cellobiose, lactose, sucrose, melibiose, D-glucuronate, D-galacturonate, fumarate, malate, L-alanine, L-glutamate, and L-tyrosine. They grow weakly on acetate. In API 20NE, it is positive for aesculin hydrolysis and for the assimilation of glucose, arabinose, mannose, mannitol, N-acetylglucosamine, maltose, gluconate, adipate, malate, and phenylacetate. The predicted major polar lipids are DPG, PG, and PI. The predicted main respiratory quinone is ubiquinone 10.
The type strain is W311T (= CECT 30796T = CCM 9337T), which was isolated from microcosm experiments made with seawater from Blanes Bay. The DNA G + C content of the strain is 65.8 mol%, and its genome size is 3.19 Mbp. The GenBank accession numbers for the whole genome and 16S rRNA gene sequences are GCA_032229225 and OP344261, respectively.
4.9 Description of Croceicoccus esteveae sp. nov.
Croceicoccus esteveae, named after Isabel Esteve (1947–2020) in honor of her relevant career in microbiology teaching and research at the Universitat Autònoma de Barcelona (es.te’ve.ae. N.L. gen. fem. n. esteveae).
Cells are cocci or coccobacilli surrounded by fibrous material. Motility is not observed. Colonies on Marine Agar are small, circular, convex, translucent, non-mucous, and bright yellow in color; they grow between 15°C and 28°C and between 0.5 and 5% (w/v) sea salts; they do not grow in 2% (w/v) NaCl as the only salt; they are Gram-negative, oxidase-positive, and catalase-positive; they weakly hydrolyze starch and do not hydrolyze alginate, casein, DNA, or cellulose; they do not grow in Agar Tween-80; and they do not grow in any of the carbon sources tested on simplified Basal Medium Agar. In API 20NE, it is positive for the assimilation of glucose, arabinose, mannose, mannitol, N-acetylglucosamine, gluconate, caprate, adipate, malate, and phenylacetate. The predicted major polar lipids are PE, DPG, PG, and PI. The predicted main respiratory quinone is ubiquinone 10.
The type strain is F390T (= CECT 30821T = CCM 9349T), which was isolated from microcosm experiments made with seawater from Blanes Bay. The DNA G + C content of the strain is 60.7 mol%, and its genome size is 2.86 Mbp. The GenBank accession numbers for the whole genome and 16S rRNA gene sequences are GCA_031846435 and OP343189, respectively.
4.10 Description of Banduia gen. nov.
Banduia (Ban.du’ia. N.L. fem. n. Banduia, named after the Gallaecian and Lusitanian goddess of water Bandua).
Cells are Gram-negative bacilli; they are oxidase-positive and catalase-positive; they are mesophilic and slightly halophilic; they require sea salts for their growth; and they are aerobic and chemoorganotrophic. The predicted major polar lipids are DPG, PG, and PE. The predicted main respiratory quinone is ubiquinone 8. The DNA G + C content is 64 mol%.
Affiliated to family Algiphilaceae in class Gammaproteobacteria. The type species is Banduia mediterranea.
4.11 Description of Banduia mediterranea sp. nov.
Banduia mediterranea (me.di.ter.ra’ne.a. L. fem. adj. mediterranea, belonging to the Mediterranean Sea).
The description is as for the genus, with the following additions: cells are thick bacilli. Motility is not observed. Colonies on Marine Agar are small, circular, convex, transparent, and non-mucous, turning opaque, mucous, and white after 2 weeks; they grow between 15°C and 37°C and between 0.5 and 5% (w/v) sea salts, with weak growth in 6%; they do not grow in 2% (w/v) NaCl as the only salt; they are Gram-negative, oxidase-positive, and catalase-positive; they do not hydrolyze any of the tested compounds. Among the carbon sources tested on simplified Basal Medium Agar grows on maltose, N-acetylglucosamine, and butyrate; they grow weakly on acetate, 3-hydroxybutyrate, and L-citrulline. In API 20NE, it is positive for the assimilation of glucose, arabinose, mannose, mannitol, N-acetylglucosamine, maltose, gluconate, caprate, adipate, malate, and phenylacetate.
The type strain is W345T (= CECT 30811T = CCM 9345T), which was isolated from microcosm experiments made with Blanes Bay seawater. The DNA G + C content of the strain is 64 mol%, and its genome size is 3.84 Mbp. The GenBank accession numbers for the whole genome and 16S rRNA gene sequences are GCA_031846245 and OP344286, respectively.
4.12 Description of Spectribacter gen. nov.
Spectribacter (Spec.tri.bac’ter. L. neut. n. spectrum, as in specter, ghost; L. vowel -i-, connecting vowel; N.L. masc. n. bacter, rod or staff and, in biology, a bacterium; Spectribacter, a bacterium of a ghostly appearance, referring to the transparent, flat aspect of its colonies).
Cells are Gram-negative bacilli; they are oxidase-positive and catalase-positive; they are mesophilic and slightly halophilic; they require sea salts for their growth; they are aerobic and chemoorganotrophic. The predicted major polar lipids are PE, DPG, and PG. The predicted main respiratory quinone is ubiquinone 8. The DNA G + C content is 67.5–67.6 mol%.
They belong to the family Salinispheraceae in the class Gammaproteobacteria. The type species is Spectribacter hydrogenooxidans.
4.13 Description of Spectribacter hydrogenooxidans sp. nov.
Spectribacter hydrogenooxidans (hy.dro.ge.no.o’xi.dans. N.L. neut. n. hydrogenum, hydrogen; N.L. pres. part. oxidans, oxidizing; N.L. part. adj. hydrogenooxidans, hydrogen-oxidizing).
The description is as for the genus, with the following additions. Cells are curved bacilli sometimes forming small chains. Motility is not observed. Colonies on Marine Agar are small, circular, plain, non-mucous, and transparent that turn whiter with time; they grow between 15°C and 37°C and between 0.5 and 8% (w/v) sea salts, with weak growth in 9 and 10%; they do not grow in 2% (w/v) NaCl as the only salt; they are Gram-negative, oxidase-positive, and catalase-positive; they do not hydrolyze any of the tested compounds, and they do not grow in Tween-80 or DNA hydrolysis plates. Among the carbon sources tested on simplified Basal Medium Agar, grows on N-acetylglucosamine, acetate, pyruvate, malate, lactate, 3-hydroxybutyrate, L-arginine, L-glutamate, and L-ornitine. They grow weakly on D-trehalose, L-rhamnose, melibiose, glycerol, D-mannitol, D-sorbitol, D-gluconate, citrate, 2-ketoglutarate, succinate, fumarate, L-alanine, L-aspartate, L-citrulline, and GABA. In API 20NE, it is positive for the assimilation of arabinose, mannose, mannitol, N-acetylglucosamine, gluconate, adipate, malate, and phenylacetate. Its genome contains the hupL gene for hydrogen oxidation.
The type strain is W335T (= CECT 30818T = CCM 9350T), which was isolated from microcosm experiments with Blanes Bay seawater. The DNA G + C content of the strain is 65.6 mol%, and its genome size is 3.29 Mbp. The GenBank accession numbers for the whole genome and 16S rRNA gene sequences are GCA_031846275 and OP344279, respectively.
4.14 Description of Spectribacter acetivorans sp. nov.
Spectribacter acetivorans (a.ce.ti.vo’rans. L. neut. n. acetum, vinegar; L. pres. part. vorans, devouring; N.L. part. adj. acetivorans, acetate-consuming).
The description is as for the genus, with the following additions. Cells are curved bacilli, sometimes found in pairs. Motility is not observed. Colonies on Marine Agar are small, circular, plain, non-mucous, transparent-white in color; they grow between 15°C and 37°C and between 1.5 and 11% (w/v) sea salts, with weak growth in 1%; they do not grow in 2% (w/v) NaCl as the only salt; they are Gram-negative, oxidase-positive, and catalase-positive; they do not hydrolyze any of the tested compounds, and they do not grow in Tween-80 agar. Among the carbon sources tested on simplified Basal Medium Agar grows on saccharose, N-acetylglucosamine, acetate, pyruvate, 3-hydroxybutyrate, L-arginine, L-aspartate, L-glutamate, L-histidine, L-citrulline, and L-ornithine. They grow weakly on D-trehalose, L-rhamnose, maltose, lactose, melibiose, D-mannitol, D-sorbitol, D-gluconate, citrate, 2-ketoglutarate, succinate, fumarate, malate, lactate, L-alanine, and GABA. In API 20NE, it is positive for the assimilation of arabinose, mannose, mannitol, N-acetylglucosamine, gluconate, adipate, malate, and phenylacetate.
The type strain is P385T (= CECT 30819T = CCM 9351T), which was isolated from microcosm experiments with Blanes Bay seawater. The DNA G + C content of the strain is 65.7 mol%, and its genome size is 3.3 Mbp. The GenBank accession numbers for the whole genome and 16S rRNA gene sequences are GCA_031846305 and OP343522, respectively.
4.15 Description of Glaciecola petra sp. nov.
Glaciecola petra (pe’tra. L. gen. fem. n. petra, stone, referring to the aspect of its colonies).
Cells are rods that form occasional chains. Motility is not observed. Colonies on Marine Agar are medium-sized, spherical, opaque, hard, and cream-colored; they grow between 15°C (weakly) and 28°C and between 3 and 4% (w/v) sea salts; they do not grow in 2% (w/v) NaCl as the only salt; they are Gram-negative, oxidase-positive, and catalase-positive; they hydrolyze alginate, but not cellulose; they do not grow in medium with casein, starch, Tween-80, or DNA. Among the carbon sources tested on simplified Basal Medium Agar, it grows weakly on 2-ketoglutarate, succinate, fumarate, malate, 3-hydroxybutyrate, and L-citrulline. In API 20NE, it is positive for hydrolysis of aesculin, β-galactosidase activity, and the assimilation of glucose, arabinose, mannose, mannitol, N-acetylglucosamine, maltose, gluconate, caprate, adipate, malate, and phenylacetate. The predicted major polar lipids are PE and PG. The predicted main respiratory quinone is ubiquinone 8.
The type strain is P117T (= CECT 30809T = CCM 9332T), which was isolated from microcosm experiments with Blanes Bay seawater. The DNA G + C content of the strain is 39.9 mol%, and its genome size is 4.05 Mbp. The GenBank accession numbers for the whole genome and 16S rRNA gene sequences are GCA_031846345 and OP343310, respectively.
4.16 Description of Brumicola gen. nov.
Brumicola (Bru.mi’co.la. L. fem. n. bruma, winter; L. masc./fem. n. suff. -cola, an inhabitant; from L. masc./fem. n. incola, dweller; N.L. fem. n. Brumicola, a dweller of winter, after the season of isolation).
Cells are Gram-negative bacilli; they are oxidase-positive and catalase-positive; they are mesophilic and slightly halophilic; they require sea salts for their growth; they are aerobic and chemoorganotrophic. The predicted major polar lipids are PE and PG. The predicted main respiratory quinone is ubiquinone 8. The DNA G + C content is 39.8–44 mol%.
They belong to the family Alteromonadaceae in the class Gammaproteobacteria. The type species is Brumicola pallidula.
4.17 Description of Brumicola pallidula comb. nov.
Brumicola pallidula (pal.lid’u.la. L. fem. adj. pallidula, somewhat pale, referring to the weak pigmentation of the species when growing on plates).
Basonym: Glaciecola pallidula Bowman et al., 1998.
The description is as given for the basonym in Bowman et al. (1998).
The type strain is IC079T (= ACAM 615T = ATCC 700757T = CIP 105819T = DSM 14239T). The GenBank accession numbers for the whole genome and 16S rRNA gene sequences are GCA_000428905 and FR746107, respectively.
4.18 Description of Brumicola nitratireducens comb. nov.
Brumicola nitratireducens (ni.tra.ti.re.du’cens. N.L. masc. n. nitras, nitrate; L. pres. part. reducens, converting to a different state; N.L. part. adj. nitratireducens, reducing nitrate).
Basonym: Glaciecola nitratireducens Baik et al., 2006.
The description is as given for the basonym in Baik et al. (2006).
The type strain is FR1064T (= JCM 12485T = KCTC 12276T). The GenBank accession numbers for the whole genome and 16S rRNA gene sequences are GCA_000226565 and AY787042, respectively.
4.19 Description of Brumicola blandensis sp. nov.
Brumicola blandensis (blan.den’sis. N.L. masc./fem. adj. blandensis, pertaining to Blande or Blanda, the name the Romans used for the city of Blanes, which has given its name to the Bay of Blanes, where the type strain was isolated).
The description is as for the genus, with the following additions. Cells are rod-shaped. Motility is not observed. Colonies on Marine Agar are big, circular, convex, translucent, mucous, and cream-colored; they grow between 4°C and 37°C (one of the strains grows weakly at this temperature) and between 2 and 9% (w/v) sea salts; they do not grow in 2% (w/v) NaCl as the only salt; they are Gram-negative, oxidase-positive, and catalase-positive; they hydrolyze casein, starch, DNA, and alginate (strain W364), but not cellulose. Among the carbon sources tested on simplified Basal Medium Agar grows on acetate, pyruvate, butyrate, citrate, 2-ketoglutarate, succinate, fumarate, lactate, 3-hydroxybutyrate, L-arginine, L-serine, L-histidine, L-serine, and L-citrulline. Cellobiose, melibiose, and malate are assimilated by one of the two strains. It grows weakly on glycerol, D-mannitol, D-sorbitol, D-gluconate, L-aspartate, GABA, and L-threonine. Arabinose, lactose, sucrose, propionate, and L-glutamate are weakly assimilated by one of the two strains. In API ZYM, it is positive for alkaline phosphatase, leucine arylamidase, trypsin, α-chemotrypsin, acid phosphatase, and naphtol AS-BI phosphohydrolase. In API 20NE, it is positive for hydrolysis of aesculin and gelatin (strain W364), β-galactosidase activity, and the assimilation of glucose (varies among strains), arabinose, mannose, mannitol, N-acetylglucosamine, maltose, gluconate, caprate (varies among strains), adipate, malate, and phenylacetate.
The type strain is W409T (= CECT 30798T = CCM 9336T), which was isolated from microcosm experiments with Blanes Bay seawater. The DNA G + C content of the strain is 42.6 mol%, and its genome size is 4.01 Mbp. The GenBank accession numbers for the whole genome and 16S rRNA gene sequences are GCA_031846165 and OP344346, respectively. Strain W364 also belongs to this species.
4.20 Description of Thalassotalea castellviae sp. nov.
Thalassotalea castellviae (cas.tell’vi.ae, N.L. gen. fem. n. castellviae, in honor of Josefina Castellví, prominent biologist and oceanographer from the Institut de Ciències del Mar, CSIC).
Cells are rod-shaped with observed polar motility. Colonies on Marine Agar are big, circular, convex, translucent, mucous, and cream-colored; they grow between 4°C and 28°C with weak results at 37°C, and between 1.5 and 8% (w/v) sea salts; they do not grow in 2% (w/v) NaCl as the only salt; they are Gram-negative, oxidase-positive, and catalase positive; they hydrolyze casein and starch, but not alginate or cellulose; and they do not grow in medium with Tween-80 nor DNA. Among the carbon sources tested on simplified Basal Medium Agar grows on pyruvate and propionate, and weakly assimilated D-fructose. API 20NE, it is positive for reduction of nitrate to nitrite, hydrolysis of aesculin and gelatin, and assimilation of glucose, arabinose, mannose, mannitol, N-acetylglucosamine, maltose, gluconate, caprate, adipate, and malate.
The type strain is W431T (= CECT 30799T = CCM 9335T), which was isolated from microcosm experiments made with Blanes Bay seawater. The DNA G + C content of the strain is 37.2 mol%, and its genome size is 3.96 Mbp. The GenBank accession numbers for the whole genome and 16S rRNA gene sequences are GCA_031846185 and OP344367, respectively.
4.21 Description of Patiriisocius hiemis sp. nov.
Patiriisocius hiemis (hi’e.mis. L. gen. masc. n. hiemis, from the cold, from winter, related to the season of isolation).
Cells are thin rods that group in long chains. Motility is not observed. Colonies on Marine Agar are small, circular, convex, translucent, non-mucous, and intense yellow in color; they do not produce flexirubin-type pigments; they grow between 15°C and 37°C and between 3 and 8% (w/v) sea salts, with weak results in 2%; they do not grow in 2% (w/v) NaCl as the only salt; they are Gram-negative, oxidase-positive, and catalase positive; they hydrolyze casein and DNA, but not alginate, starch, or cellulose; and they do not grow in Agar Tween-80. Among the carbon sources tested on simplified Basal Medium Agar grows on L-arginine and grows weakly on acetate. In API 20NE, it is positive for hydrolysis of gelatin and assimilation of glucose, arabinose, mannose, mannitol, N-acetylglucosamine, maltose, gluconate, caprate, adipate, and malate. The predicted major polar lipid is PE. The predicted main respiratory quinone is menaquinone 6.
The type strain is W242T (= CECT 30795T = CCM 9333T), which was isolated from microcosm experiments made with Blanes Bay seawater. The DNA G + C content of the strain is 33.7 mol%, and its genome size is 2.84 Mbp. The GenBank accession numbers for the whole genome and 16S rRNA gene sequences are GCA_031846325 and OP344205, respectively.
4.22 Description of Urechidicola vernalis sp. nov.
Urechidicola vernalis (ver.na’lis. L. fem. adj. vernalis, belonging to the spring, the season when the type strain was isolated).
Cells are thin rods forming very long and sinuous chains. Motility is not observed. Colonies on Marine Agar are medium-sized, circular, convex, translucent, mucous, and yellow in color; they do not produce flexirubin-type pigments; they grow between 4°C and 28°C and between 3 and 4% (w/v) sea salts; they do not grow in 2% (w/v) NaCl as the only salt; they are Gram-negative, oxidase-positive, and catalase negative; they hydrolyze starch, but not casein, alginate, or cellulose; they do not grow in medium with Tween-80 or DNA; and they do not grow in any of the carbon sources tested on simplified Basal Medium Agar. In API 20NE, it is positive for assimilation of glucose, arabinose, mannose, mannitol, N-acetylglucosamine, maltose, gluconate, caprate, adipate, malate, and phenylacetate. The predicted major polar lipid is PE. The predicted main respiratory quinone is menaquinone 6.
The type strain is P050T (= CECT 30802T = CCM 9340T), which was isolated from microcosm experiments made with Blanes Bay seawater. The DNA G + C content of the strain is 33.5 mol%, and its genome size is 3.14 Mbp. The GenBank accession numbers for the whole genome and 16S rRNA gene sequences are GCA_031846385 and OP343250, respectively.
4.23 Description of Microcosmobacter gen. nov.
Microcosmobacter (Mi.cro.cos.mo.bac’ter. Gr. masc. adj. mikros, small; N.L. masc. n. Gr. masc. n. kosmos, universe or world; N.L. masc. n. bacter, a rod; N.L. masc. n. Microcosmobacter, a rod from a microcosm, in reference to the isolation site, a microcosm experiment).
Cells are Gram-negative bacilli; they are oxidase-positive and catalase-positive; they are mesophilic and slightly halophilic; they require sea salts for their growth; and they are aerobic and chemoorganotrophic. The predicted major polar lipid is PE. The predicted main respiratory quinone is menaquinone 6. They do not produce flexirubin-type pigments. The DNA G + C content is 32.5 mol%.
They belong to the family Flavobacteriaceae in the class Bacteroidia. The type species is Microcosmobacter mediterraneus.
4.24 Description of Microcosmobacter mediterraneus sp. nov.
Microcosmobacter mediterraneus (me.di.ter.ra’ne.us. L. masc. adj. mediterraneus, from the Mediterranean Sea).
The description is as for the genus, with the following additions. Cells are small, short rods. Motility is not observed. Colonies on Marine Agar are small, circular, convex, translucent, non-mucous, and yellow-orange in color; they do not produce flexirubin-type pigments; they grow at 28°C only and between 3 and 4% (w/v) sea salts; they do not grow in 2% (w/v) NaCl as the only salt; they are Gram-negative, oxidase-positive, and catalase positive; they hydrolyze casein, but not starch, alginate, or cellulose; do not grow in medium with Tween-80 or DNA; and they do not grow in any of the carbon sources tested on simplified Basal Medium Agar. In API 20NE, it is positive for assimilation of glucose, arabinose, mannose, mannitol, N-acetylglucosamine, maltose, gluconate, caprate, adipate, malate, and phenylacetate. The predicted major polar lipid is PE. The predicted main respiratory quinone is menaquinone 6.
The type strain is W332T (= CECT 30810T = CCM 9339T), which was isolated from microcosm experiments made with Blanes Bay seawater. The DNA G + C content of the strain is 32.5 mol%, and its genome size is 3 Mbp. The GenBank accession numbers for the whole genome and 16S rRNA gene sequences are GCA_031846265 and OP344277, respectively.
4.25 Description of Asprobacillus gen. nov.
Asprobacillus (As.pro.ba.ci’llus. Gr. adj. Aspros, white; L. masc. n. bacillus, small rod, N.L. masc. n. Asprobacillus, white rod).
Cells are Gram-negative bacilli; they are oxidase-positive and catalase-positive; they are mesophilic and slightly halophilic; they require sea salts for their growth; they are aerobic and chemoorganotrophic. The predicted major polar lipid is PE. The predicted main respiratory quinone is menaquinone 6. They do not produce flexirubin-type pigments. The DNA G + C content is 35.2 mol%.
They belong to the family Flavobacteriaceae in the class Bacteroidia. The type species is Asprobacillus argos.
4.26 Description of Asprobacillus argos sp. nov.
Asprobacillus argos (ar’gos. Gr. masc. adj. argos, slow, referring to its slow growth).
The description is as for the genus, with the following additions. Cells are thin rods. Motility is not observed. Colonies on Marine Agar are circular, convex, mucous, opaque, and white in color; they grow between 15°C and 28°C and between 1.5 and 3% (w/v) sea salts; they do not grow in 2% (w/v) NaCl as the only salt; they are Gram-negative, oxidase-positive, and catalase weakly positive; they hydrolyze starch, but not cellulose; they do not grow in medium with casein or Tween-80; they do not grow in any of the carbon sources tested on simplified Basal Medium Agar. In API ZYM, it is positive for alkaline phosphatase, leucine and valine arylamidases, acid phosphatase, and naphtol AS-BI phosphohydrolase.
The type strain is S356T (= CECT 30474T = CCM 9388T), which was isolated from microcosm experiments made in Blanes Bay seawater. The DNA G + C content of the strain is 35.2 mol%, and its genome size is 3.18 Mbp. The GenBank accession numbers for the whole genome and 16S rRNA gene sequences are GCA_032248395 and OP343904, respectively.
4.27 Description of Autumnicola gen. nov.
Autumnicola (Au.tum.ni’co.la. L. masc. n. autumnus, fall, autumn; L. masc./fem. n. suff. -cola, an inhabitant; from L. masc./fem. n. incola, dweller; N.L. fem. n. Autumnicola, autumn dweller, in reference of the season when the strains of this genus were isolated).
Cells are Gram-negative bacilli; they are oxidase-positive and catalase-positive; they are mesophilic and slightly halophilic; they require sea salts for their growth; they are aerobic and chemoorganotrophic. The predicted major polar lipid is PE. The predicted main respiratory quinone is menaquinone 6. They do not produce flexirubin-type pigments. The DNA G + C content is 38.3–40 mol%.
They belong to the family Flavobacteriaceae in the class Bacteroidia. The type species is Autumnicola musiva.
4.28 Description of Autumnicola musiva sp. nov.
Autumnicola musiva (mu’si.va, L. fem. adj. musiva, mosaic, related to the aspect of the cells observed through an optical microscope).
The description is as for the genus, with the following additions. Cells are short bacilli, often forming sinuous chains. Motility is not observed. Colonies on Marine Agar are small, circular, convex, translucid, mucous, and yellowish in color; they grow between 15°C and 37°C and between 0.5 and 7% (w/v) sea salts, with weak growth in 8 and 9%; they do not grow in 2% (w/v) NaCl as the only salt; they are Gram-negative, oxidase-positive, and catalase-positive; they hydrolyze DNA and weakly hydrolyze casein and starch; they do not hydrolyze alginate or cellulose; and they do not grow on medium with Tween-80. Among the carbon sources tested on simplified Basal Medium Agar grows on D-trehalose, L-rhamnose, cellobiose, lactose, sucrose, N-acetylglucosamine, and L-glutamate. In API 20NE, it is positive for hydrolysis of aesculin and assimilation of glucose, arabinose, mannose, mannitol, N-acetylglucosamine, maltose, gluconate, caprate, adipate, malate, citrate, and phenylacetate.
The type strain is F117T (= CECT 30804T = CCM 9344T), which was isolated from microcosm experiments made with Blanes Bay seawater. The DNA G + C content of the strain is 38.3 mol%, and its genome size is 4.52 Mbp. The GenBank accession numbers for the whole genome and 16S rRNA gene sequences are GCA_031846585 and OP342948, respectively.
4.29 Description of Autumnicola tepida sp. nov.
Autumnicola tepida (te’pi.da, L. fem. adj. tepida, warm).
The description is as for the genus, with the following additions. Cells are thin rods, frequently forming sinuous chains. Motility is not observed. Colonies on Marine Agar are small, circular, convex, translucent, non-mucous, orange in the center, and yellow in the margins; they grow between 15°C and 40°C and between 0.5 and 4% (w/v) sea salts; they do not grow in 2% (w/v) NaCl as the only salt; they are Gram-negative, oxidase-positive, and catalase-positive; they hydrolyze casein, Tween-80, and DNA and weakly hydrolyze starch; and they do not hydrolyze alginate or cellulose. Among the carbon sources tested on simplified Basal Medium Agar grows on D-trehalose, maltose, cellobiose, lactose, sucrose, melibiose, N-acetylglucosamine, and D-gluconate. In API 20NE, it is positive for hydrolysis of aesculin and gelatin, β-galactosidase activity, and assimilation of glucose, arabinose, mannose, mannitol, N-acetylglucosamine, maltose, adipate, malate, and phenylacetate.
The type strain is F363T (= CECT 30820T = CCM 9348T), which was isolated from microcosm experiments made with Blanes Bay seawater. The DNA G + C content of the strain is 40 mol%, and its genome size is 4.37 Mbp. The GenBank accession numbers for the whole genome and 16S rRNA gene sequences are GCA_031846485 and OP343172, respectively.
4.30 Description of Autumnicola patrickiae sp. nov.
Autumnicola patrickiae (pa.trick’i.ae, N.L. gen. fem. n. patrickiae, in honor of Ruth Myrtle Patrick (1907–2013), a botanist and limnologist specializing in diatoms and freshwater ecology).
The description is as for the genus, with the following additions. Cells are long rods, sometimes forming pairs or sinuous chains. Motility is not observed. Colonies on Marine Agar are small, circular, convex, translucent, non-mucous, and yellow in color; they grow between 4°C and 37°C and between 1.5 and 11% (w/v) sea salts, with weak growth in 1%; they do not grow in 2% (w/v) NaCl as the only salt; they are Gram-negative, oxidase-positive, and catalase positive; they hydrolyze DNA and weakly hydrolyze starch; they do not hydrolyze casein, alginate, or cellulose; and they do not grow in medium with Tween-80. Among the carbon sources tested on simplified Basal Medium Agar only grows on D-trehalose. In API 20NE, it is positive for hydrolysis of aesculin, β-galactosidase activity, and assimilation of glucose, arabinose, mannose, mannitol, N-acetylglucosamine, maltose, gluconate, caprate, adipate, malate, and phenylacetate.
The type strain is F188T (= CECT 30805T = CCM 9341T), which was isolated from microcosm experiments made with Blanes Bay seawater. The DNA G + C content of the strain is 38.4 mol%, and its genome size is 4.35 Mbp. The GenBank accession numbers for the whole genome and 16S rRNA gene sequences are GCA_031846525 and OP343012, respectively.
4.31 Description of Autumnicola edwardsiae sp. nov.
Autumnicola edwardsiae (ed.ward’si.ae, N.L. gen. fem. n. edwardsiae, in honor of Katrina Jane Edwards (1968–2014), pioneering geomicrobiologist also called ‘Mistress of the Dark World’ known for her studies on the organisms living below the ocean floor).
The description is as for the genus, with the following additions. Cells are short, thick rods, often found in small or long sinuous chains. Motility is not observed. Colonies on Marine Agar are small, circular, convex, translucent, non-mucous, and yellow in color; they grow between 15°C and 28°C and between 1 and 11% (w/v) sea salts, with weak growth in 12%; they do not grow in 2% (w/v) NaCl as the only salt; they Gram-negative, oxidase-positive, and catalase-positive; they hydrolyze DNA and weakly hydrolyze starch; they do not hydrolyze casein, alginate, or cellulose; and they do not grow in medium with Tween-80. Among the carbon sources tested on simplified Basal Medium Agar only grows weakly on lactose. In API ZYM, it is positive for alkaline phosphatase, leucine and valine arylamidases, acid phosphatase, and naphtol AS-BI phosphohydrolase. In API 20NE, it is positive for hydrolysis of aesculin and assimilation of glucose, arabinose, mannose, mannitol, N-acetylglucosamine, maltose, caprate, adipate, malate, and phenylacetate.
The type strain is F297T (= CECT 30794T = CCM 9342T), which was isolated from microcosm experiments made with Blanes Bay seawater. The DNA G + C content of the strain is 38.9 mol%, and its genome size is 3.83 Mbp. The GenBank accession numbers for the whole genome and 16S rRNA gene sequences are GCA_031846505 and OP343113, respectively.
4.32 Description of Autumnicola lenta sp. nov.
Autumnicola lenta (len.ta, L. fem. adj. lenta, referring to its slow growth).
The description is as for the genus, with the following additions. Cells are short, thick rods, sometimes thicker on the margins, often forming pairs or chains. Motility is not observed. Colonies on Marine Agar are small, circular, convex, translucent, non-mucous, and yellowish in color; they grow between 15°C and 28°C, with weak growth at 4°C, and between 1.5 and 11% (w/v) sea salts; they do not grow in 2% (w/v) NaCl as the only salt; they are Gram-negative, oxidase-positive, and catalase-positive; they hydrolyze DNA and weakly hydrolyze starch; they do not hydrolyze casein, alginate, or cellulose and did not grow in medium with Tween-80; and they do not grow on any of the carbon sources tested on simplified Basal Medium Agar. In API 20NE, it is positive for hydrolysis of aesculin and assimilation of glucose, arabinose, mannose, mannitol, N-acetylglucosamine, maltose, gluconate, adipate, malate, citrate, and phenylacetate.
The type strain is F260T (= CECT 30806T = CCM 9346T), which was isolated from microcosm experiments made with Blanes Bay seawater. The DNA G + C content of the strain is 38.6 mol%, and its genome size is 3.93 Mbp. The GenBank accession numbers for the whole genome and 16S rRNA gene sequences are GCA_031846515 and OP343076, respectively.
4.33 Description of Autumnicola psychrophila sp. nov.
Autumnicola psychrophila (psy.chro’phi.la. Gr. masc. adj. psychros, cold; Gr. masc. adj. philos, liking, loving; N.L. fem. adj. psychrophila, cold-liking, as it grows in low temperatures).
The description is as for the genus, with the following additions. Cells are long rods, often found in pairs. Motility is not observed. Colonies on Marine Agar are small, circular, convex, translucent, non-mucous, and yellow in color; they grow between 4°C and 37°C and between 0.5 and 11% (w/v) sea salts; they do not grow in 2% (w/v) NaCl as the only salt; they are Gram-negative, oxidase-positive, and catalase positive; they hydrolyze DNA and weakly hydrolyze starch; they do not hydrolyze casein, alginate, or cellulose; and they do not grow in medium with Tween-80. Among the carbon sources tested on simplified Basal Medium Agar grows on D-trehalose, maltose, and lactose. In API 20NE, it is positive for hydrolysis of aesculin, β-galactosidase activity, and assimilation of glucose, arabinose, mannose, mannitol, N-acetylglucosamine, maltose, gluconate, adipate, malate, and phenylacetate.
The type strain is F225T (= CECT 30793T = CCM 9347T), which was isolated from microcosm experiments made with Blanes Bay seawater. The DNA G + C content of the strain is 38.4 mol%, and its genome size is 3.96 Mbp. The GenBank accession numbers for the whole genome and 16S rRNA gene sequences are GCA_031846645 and OP343046, respectively.
4.34 Description of Croceitalea rosinachiae sp. nov.
Croceitalea rosinachiae (ro.si.nach’i.ae, N.L. fem. n. rosinachiae, in honor of Zoe Rosinach Pedrol (1894–1973), the first Spanish woman to get a Ph.D. in pharmacy and a pioneering feminist activist).
Cells are very thin rods that often form short chains. Motility is not observed. Colonies on Marine Agar are small, circular, convex, translucent, non-mucous, and intense orange in color; they do not produce flexirubin-type pigments; they grow at 28°C only and between 2 and 5% (w/v) sea salts; they do not grow in 2% (w/v) NaCl as the only salt; they are Gram-negative, oxidase-positive, and catalase-positive; they hydrolyze alginate, but not cellulose; and they do not grow in mediums with casein, starch, Tween-80, or DNA. Among the carbon sources tested on simplified Basal Medium Agar grows only on lactose. In API ZYM, it is positive for alkaline phosphatase, leucine and valine arylamidases, trypsin, acid phosphatase, naphtol AS-BI phosphohydrolase, α-glucosidase, and N-acetyl-β-glucosaminidase. In API 20NE, it is positive for hydrolysis of aesculin, β-galactosidase activity, and assimilation of mannose, mannitol, N-acetylglucosamine, maltose, gluconate, caprate, adipate, malate, and phenylacetate. The predicted major polar lipid is PE. The predicted main respiratory quinone is menaquinone 6.
The type strain is F388T (= CECT 30807T = CCM 9338T), which was isolated from microcosm experiments made with Blanes Bay seawater. The DNA G + C content of the strain is 36.4 mol%, and its genome size is 3.6 Mbp. The GenBank accession numbers for the whole genome and 16S rRNA gene sequences are GCA_031846445 and OP343188, respectively.
4.35 Description of Croceitalea vernalis sp. nov.
Croceitalea vernalis (ver.na’lis. L. fem. adj. vernalis, belonging to the spring, the season when the type strain was isolated).
Cells are very thin rods, often forming short to long chains. Motility is not observed. Colonies on Marine Agar are small, circular, convex, translucent, non-mucous, and intense orange in color; they do not produce flexirubin-type pigments; they grow between 15°C and 28°C and between 3 and 5% (w/v) sea salts. One of the strains has weak growth in 2 and 5% (w/v) sea salts. They do not grow in 2% (w/v) NaCl as the only salt; they are Gram-negative, oxidase-positive, and catalase-positive; they hydrolyze alginate but not starch, casein, or cellulose; and they do not grow in medium containing Tween-80 or DNA. Among the carbon sources tested on simplified Basal Medium Agar grows weakly on 2-ketoglutarate. In API ZYM, it is positive for alkaline phosphatase, esterase C4 (strain P007T, weakly), leucine and valine arylamidases, trypsin, acid phosphatase, naphtol AS-BI phosphohydrolase, α-glucosidase (strain P007T), and N-acetyl-β-glucosaminidase. In API 20NE, it is positive for hydrolysis of aesculin and assimilation of glucose, arabinose, mannose, mannitol, N-acetylglucosamine, maltose, gluconate, adipate, malate, and phenylacetate. The predicted major polar lipid is PE. The predicted main respiratory quinone is menaquinone 6.
The type strain is P007T (= CECT 30801T = CCM 9330T), which was isolated from microcosm experiments made with Blanes Bay seawater. The DNA G + C content of the strain is 34.7 mol%, and its genome size is 3.38 Mbp. The GenBank accession numbers for the whole genome and 16S rRNA gene sequences are GCA_031846405 and OP343207, respectively. Strain P059 also belongs to this species.
4.36 Description of Pricia mediterranea sp. nov.
Pricia mediterranea (me.di.ter.ra’ne.a. L. fem. adj. mediterranea, belonging to the Mediterranean Sea).
Cells are thin rods. Motility is not observed. Colonies on Marine Agar are circular, convex, with undulate margin, translucent, mucous, and intense orange in color; they do not produce flexirubin-type pigments; they grow between 15°C and 28°C and between 0.5 and 5% (w/v) sea salts; they do not grow in 2% (w/v) NaCl as the only salt; they are Gram-negative, oxidase-positive, and catalase weakly positive; they hydrolyze casein and Tween-80, but not starch or cellulose. Among the carbon sources tested on simplified Basal Medium Agar grows weakly on D-mannose, D-trehalose, L-rhamnose, maltose, lactose, mannitol, D-gluconate, salicilin, amigdalin, fumarate, 3-hydroxybutyrate, L-arginine, and L-ornithine. In API ZYM, it is positive for alkaline phosphatase, leucine, valine and cystine arylamidases, trypsin, acid phosphatase, naphtol AS-BI phosphohydrolase, β-galactosidase, α-glucosidase, β-glucosidase, N-acetyl-β-glucosaminidase, and α-mannosidase. In API 20NE, it is positive for hydrolysis of aesculin and β-galactosidase activity. The predicted major polar lipids are PE and DPG. The predicted main respiratory quinone is menaquinone 6.
The type strain is S334T (= CECT 30471T = CCM 9387T), which was isolated from microcosm experiments made with Blanes Bay seawater. The DNA G + C content of the strain is 47 mol%, and its genome size is 4.57 Mbp. The GenBank accession numbers for the whole genome and 16S rRNA gene sequences are GCA_032248455 and OP343883, respectively.
4.37 Description of Rubrivirga litoralis sp. nov.
Rubrivirga litoralis (li.to.ra’lis. L. masc./fem. adj. litoralis, coastal).
Cells are peanut-shaped rods with an important extracellular matrix. Big spheres are also observed on scanning electron microscopy. Motility is not observed. Colonies on Marine Agar are small, circular, convex, opaque, aqueous, and pale red in color that turns more intense with time; they grow between 15°C and 37°C. One of the strains grows between 3 and 6% (w/v) sea salts and the other does between 1.5 and 3% (w/v) sea salts, with weak growth at 4%; they do not grow in 2% (w/v) NaCl as the only salt; they are Gram-negative, oxidase-positive, and catalase-positive; they hydrolyze starch, but not alginate or cellulose; they do not grow in medium containing casein, Tween-80, or DNA; and they do not grow on any of the carbon sources tested on simplified Basal Medium Agar. In API ZYM, it is positive for alkaline phosphatase, leucine and valine arylamidases, α-chymotrypsin, acid phosphatase, naphtol AS-BI phosphohydrolase, and α-glucosidase. In API 20NE, it is positive for reduction of nitrate to nitrite and aesculin hydrolysis, β-galactosidase activity (strain S365), glucose, arabinose, mannose, mannitol, N-acetylglucosamine, maltose, gluconate, adipate, and phenylacetate (all the assimilations were positive in strain F394T). The predicted major polar lipids are PE and PG. The predicted main respiratory quinone is menaquinone 7.
The type strain is F394T (= CECT 30808T = CCM 9352T), which was isolated from microcosm experiments made with Blanes Bay seawater. The DNA G + C content of the strain is 73.7 mol%, and its genome size is 3.72 Mbp. The GenBank accession numbers for the whole genome and 16S rRNA gene sequences are GCA_031846415 and OP343191, respectively. Strain S365 also belongs to this species.
Data availability statement
The 16S rRNA gene Sanger sequences can be found in GenBank under accessions OP342948, OP342988, OP343012, OP343046, OP343076, OP343113, OP343172, OP343188, OP343189, OP343191, OP343207, OP343250, OP343258, OP343310, OP343522, OP343883, OP343904, OP343913, OP344205, OP344261, OP344277, OP344279, OP344286, OP344303, OP344346, and OP344367. The genome sequences used in this study can be found in GenBank under BioProject PRJNA1016293. The Tara Oceans metagenomic datasets can be found in ENA under BioProject PRJEB1787 and PJREB9740.
Author contributions
XR-V: Conceptualization, Data curation, Formal analysis, Investigation, Methodology, Writing – original draft, Writing – review & editing. TL: Data curation, Validation, Writing – review & editing. AB: Data curation, Investigation, Validation, Writing – review & editing. JG: Funding acquisition, Resources, Supervision, Validation, Writing – review & editing. OS: Funding acquisition, Resources, Supervision, Validation, Writing – review & editing. DA: Conceptualization, Data curation, Funding acquisition, Investigation, Methodology, Resources, Supervision, Validation, Writing – review & editing. MP: Conceptualization, Funding acquisition, Investigation, Methodology, Resources, Supervision, Validation, Writing – review & editing.
Funding
The author(s) declare that financial support was received for the research, authorship, and/or publication of this article. This research was supported by grants RTI2018-101025-B-I00, PID2021-125469NB-C31, and PID2021-125469NB-C32 funded by the Spanish Ministry of Science and Innovation and AICO2020-181 funded by Generalitat Valenciana. This study acknowledges the Severo Ochoa Center of Excellence accreditation (CEX2019-000928-S). XR-V was supported by a Spanish FPU grant.
Acknowledgments
We thank the University of Valencia for hosting XR-V during his stay in València, where a great part of the study was done. Computational power was provided by CESGA (Centro de Supercomputación de Galicia).
Conflict of interest
The authors declare that the research was conducted in the absence of any commercial or financial relationships that could be considered as a potential conflict of interest.
Publisher’s note
All claims expressed in this article are solely those of the authors and do not necessarily represent those of their affiliated organizations, or those of the publisher, the editors and the reviewers. Any product that may be evaluated in this article, or claim that may be made by its manufacturer, is not guaranteed or endorsed by the publisher.
Supplementary material
The Supplementary material for this article can be found online at: https://www.frontiersin.org/articles/10.3389/fmicb.2024.1407904/full#supplementary-material
SUPPLEMENTARY TABLE S1 | Biogeochemically relevant genes screened in this study and their references.
SUPPLEMENTARY TABLE S2 | Extended genomic characteristics of the strains, including information of the 16S rRNA gene sequences and the closest neighbors according to ANI (Average Nucleotide Identity), AAI (Average Aminoacid Identity) and dDDH (digital DNA-DNA hybridization) values.
SUPPLEMENTARY TABLE S3 | Taxonomic classification of the novel strains from their 16S rRNA gene sequences and their genomes.
SUPPLEMENTARY TABLE S4 | ANI (Average Nucleotide Identity) and AAI (Average Aminoacid Identity) values between the novel strains.
SUPPLEMENTARY TABLE S5 | Full list of and digital DNA-DNA hybridization (dDDH), AAI (Average Aminoacid Identity) and ANI (Average Nucleotide Identity) values between the novel strains and their close relatives. The TYGS (Type Strain Genome Server) section includes all the genomes that were used to compute the phylogenomic trees of each group and their accession numbers.
SUPPLEMENTARY TABLE S6 | Results for sole carbon source utilization in simplified Basal Medium Agar. w, weak growth; NT, not tested.
SUPPLEMENTARY TABLE S7 | Taxogenomic characteristics (major polar lipids, respiratory quinones and gliding motility genes) of the strains as predicted from their genomes. MK, menaquinone; Q, ubiquinone; PE, phosphatidilethanolamine; PG, phosphatidilglycerol; DPG, diphosphatidilglyerol; PI, phosphatidil m-inositol; PC, phosphatidilcholine.
SUPPLEMENTARY TABLE S8 | Comparison of phenotypic and genomic characteristics of the strains and their closest relatives. Positive results are colored in red, weakly positive results are colored in pale red and negative results are colored in blue. NT, not tested (or not reported in the literature); NA, non-applicable (mostly if the given test was not performed in the strain of this study); NG, no growth (for hydrolysis tests); w, weak results. MK, menaquinone; Q, ubiquinone; PE, phosphatidilethanolamine; PG, phosphatidilglycerol; DPG, diphosphatidilglyerol; PI, phosphatidil m-inositol; PC, phosphatidilcholine.
SUPPLEMENTARY FIGURE S1 | AAI (Average Aminoacid Identity) matrix of strains P117T, W364, W409T and their closest neighbors obtained with the Kostas Lab AAI/ANI matrix tool (Rodriguez-R and Konstantinidis, 2016).
SUPPLEMENTARY FIGURE S2 | AAI (Average Aminoacid Identity) matrix of strain S365T and its closest neighbors obtained with the Kostas Lab AAI/ANI matrix tool (Rodriguez-R and Konstantinidis, 2016).
SUPPLEMENTARY FIGURE S3 | Graphical representation of the relevance of the novel genus Autumnicola in the cycles of carbon, nitrogen and phosphorus. The genes that appear in the drawing are contained by at least one of the members of this genus.
Footnotes
1. ^ http://www.bioinformatics.babraham.ac.uk/projects/fastqc
2. ^ https://github.com/erikrikarddaniel/biomakefiles
References
Alonso-Sáez, L., Morán, X. A. G., and González, J. M. (2020). Transcriptional patterns of biogeochemically relevant marker genes by temperate marine Bacteria. Front. Microbiol. 11, 1–10. doi: 10.3389/fmicb.2020.00465
Altschul, S. F., Gish, W., Miller, W., Myers, E. W., and Lipman, D. J. (1990). Basic local alignment search tool. J. Mol. Biol. 215, 403–410. doi: 10.1016/S0022-2836(05)80360-2
Auladell, A., Ferrera, I., Montiel Fontanet, L., Júnior, C. D. S., Sebastián, M., Logares, R., et al. (2023). Seasonality of biogeochemically relevant microbial genes in a coastal ocean microbiome. Environ. Microbiol. 25, 1465–1483. doi: 10.1111/1462-2920.16367
Azam, F., Fenchel, T., Field, J., Gray, J., Meyer-Reil, L., and Thingstad, F. (1983). The ecological role of water-column microbes in the sea. Mar. Ecol. Prog. Ser. 10, 257–263. doi: 10.3354/meps010257
Aziz, R. K., Bartels, D., Best, A., DeJongh, M., Disz, T., Edwards, R. A., et al. (2008). The RAST server: rapid annotations using subsystems technology. BMC Genomics 9, 1–15. doi: 10.1186/1471-2164-9-75
Bache, S. M., and Wickham, H. (2022). magrittr: A Forward-Pipe Operator for R. R package version 2.0.3. Available at: https://CRAN.R-project.org/package=magrittr.
Baik, K. S., Park, Y. D., Seong, C. N., Kim, E. M., Bae, K. S., and Chun, J. (2006). Glaciecola nitratireducens sp. nov., isolated from seawater. Int. J. Syst. Evol. Microbiol. 56, 2185–2188. doi: 10.1099/ijs.0.64330-0
Bar-On, Y. M., and Milo, R. (2019). The biomass composition of the oceans: a blueprint of our blue planet. Cell 179, 1451–1454. doi: 10.1016/j.cell.2019.11.018
Baumann, P., and Baumann, L. (1981). “The marine gram-negative eubacteria: genera Photobacterium, Beneckea, Alteromonas, Pseudomonas and Alcaligenes” in The prokaryotes. eds. M. P. Starr, H. Stolp, H. G. Trueper, A. Balows, and H. Schleger (Berlin, Heidelberg: Springer), 1302–1331.
Becker, R. A., and Wilks, A. R. (2022). Package “mapdata” - Extra Map Databases. Available at: https://cran.r-project.org/web/packages/maps/index.html
Becker, R. A., and Wilks, A. R. (2023). Package “maps”: Draw geographical maps. Available at: https://cran.r-project.org/web/packages/mapdata/index.html
Beja, O., Aravind, L., Koonin, E. V., Suzuki, M. T., Hadd, A., Nguyen, L. P., et al. (2000). Bacterial rhodopsin: evidence for a new type of phototrophy in the sea. Science 289, 1902–1906. doi: 10.1126/science.289.5486.1902
Bernadet, J.-F., Nakagawa, Y., and Holmes, B. (2002). Proposed minimal standards for describing new taxa of the family Flavobacteriaceae and Jean-franc by the members of the subcommittee on the taxonomy of Flavobacterium and Cytophaga -like bacteria of the international committee on systematics of. Int. J. Syst. Evol. Microbiol. 52, 1049–1070. doi: 10.1099/ijs.0.02136-0.02136
Bowman, J. P., and Mccammon, S. a, Brown, J. L., and Mcmeekin, T. A (1998). Glaciecola punicea gen. Nov., sp. nov. and Glaciecola pallidula gen. Nov., sp. nov.: psychrophilic bacteria from Antarctic Sea-ice habitats. Int. J. Syst. Bacteriol. 48, 1213–1222.
Buchfink, B., Reuter, K., and Drost, H. G. (2021). Sensitive protein alignments at tree-of-life scale using DIAMOND. Nat. Methods 18, 366–368. doi: 10.1038/s41592-021-01101-x
Bushnell, B. (2012). BBMap: a fast, accurate, splice-aware aligner. BMC Bioinformatics 13, 1–2. doi: 10.1186/1471-2105-13-238
Cantalapiedra, C. P., Hernandez-Plaza, A., Letunic, I., Bork, P., and Huerta-Cepas, J. (2021). eggNOG-mapper v2: functional annotation, Orthology assignments, and domain prediction at the metagenomic scale. Mol. Biol. Evol. 38, 5825–5829. doi: 10.1093/molbev/msab293
Chaumeil, P. A., Mussig, A. J., Hugenholtz, P., and Parks, D. H. (2020). GTDB-Tk: a toolkit to classify genomes with the genome taxonomy database. Bioinformatics 36, 1925–1927. doi: 10.1093/bioinformatics/btz848
Chaumeil, P. A., Mussig, A. J., Hugenholtz, P., and Parks, D. H. (2022). GTDB-Tk v2: memory friendly classification with the genome taxonomy database. Bioinformatics 38, 5315–5316. doi: 10.1093/bioinformatics/btac672
Chen, B., Ye, Y., Lin, D., Zhang, M., Sun, J., and Tang, K. (2022). Croceicoccus hydrothermalis sp. nov., isolated from shallow-sea hydrothermal system off Kueishantao Island. Int. J. Syst. Evol. Microbiol. 72, 1–7. doi: 10.1099/ijsem.0.005472
Chen, C., Zheng, Q., Wang, Y. N., Yan, X. J., Hao, L. K., Du, X., et al. (2010). Stakelama pacifica gen. Nov., sp. nov., a new member of the family Sphingomonadaceae isolated from the Pacific Ocean. Int. J. Syst. Evol. Microbiol. 60, 2857–2861. doi: 10.1099/ijs.0.018945-0
Cole, J. R., Wang, Q., Fish, J. A., Chai, B., McGarrell, D. M., Sun, Y., et al. (2014). Ribosomal database project: data and tools for high throughput rRNA analysis. Nucleic Acids Res. 42, D633–D642. doi: 10.1093/nar/gkt1244
Cordero, P. R. F., Bayly, K., Man Leung, P., Huang, C., Islam, Z. F., Schittenhelm, R. B., et al. (2019). Atmospheric carbon monoxide oxidation is a widespread mechanism supporting microbial survival. ISME J. 13, 2868–2881. doi: 10.1038/s41396-019-0479-8
Del Giorgio, P. A., and Duarte, C. M. (2002). Respiration in the open ocean. Nature 420, 379–384. doi: 10.1038/nature01165
Duarte, C. M. (2015). Seafaring in the 21st century: the Malaspina 2010 circumnavigation expedition. Limnol. Oceanogr. Bull. 24, 11–14. doi: 10.1002/lob.10008
Ewels, P. A., Peltzer, A., Fillinger, S., Patel, H., Alneberg, J., Wilm, A., et al. (2020). The nf-core framework for community-curated bioinformatics pipelines. Nat. Biotechnol. 38, 276–278. doi: 10.1038/s41587-020-0439-x
Falkowski, P. G., Fenchel, T., and Delong, E. F. (2008). The microbial engines that drive earth’s biogeochemical cycles. Science 320, 1034–1039. doi: 10.1126/science.1153213
Farooq, A. (1998). Microbial control of oceanic carbon flux: the plot thickens. Science 280, 694–696. doi: 10.1126/science.280.5364.694
Fenchel, T. (2008). The microbial loop - 25 years later. J. Exp. Mar. Biol. Ecol. 366, 99–103. doi: 10.1016/j.jembe.2008.07.013
Ferrera, I., Sebastian, M., Acinas, S. G., and Gasol, J. M. (2015). Prokaryotic functional gene diversity in the sunlit ocean: stumbling in the dark. Curr. Opin. Microbiol. 25, 33–39. doi: 10.1016/j.mib.2015.03.007
Fotedar, R., Caldwell, M. E., Sankaranarayanan, K., Al-Zeyara, A., Al-Malki, A., Kaul, R., et al. (2020). Ningiella ruwaisensis gen. Nov., sp. nov., a member of the family Alteromonadaceae isolated from marine water of the Arabian gulf. Int. J. Syst. Evol. Microbiol. 70, 4130–4138. doi: 10.1099/ijsem.0.004256
Gasol, J. M., and Kirchman, D. L. (2018). Microbial ecology of the oceans. 3rd Edn. Hoboken, NJ, USA: Wiley-Blackwell.
Giovannoni, S., and Stingl, U. (2007). The importance of culturing bacterioplankton in the “omics” age. Nat. Rev. Microbiol. 5, 820–826. doi: 10.1038/nrmicro1752
Gómez-Consarnau, L., Akram, N., Lindell, K., Pedersen, A., Neutze, R., Milton, D. L., et al. (2010). Proteorhodopsin phototrophy promotes survival of marine bacteria during starvation. PLoS Biol. 8, e1000358–e1000311. doi: 10.1371/journal.pbio.1000358
Gurevich, A., Saveliev, V., Vyahhi, N., and Tesler, G. (2013). QUAST: quality assessment tool for genome assemblies. Bioinformatics 29, 1072–1075. doi: 10.1093/bioinformatics/btt086
Gutierrez, T., Green, D. H., Whitman, W. B., Nichols, P. D., Semple, K. T., and Aitken, M. D. (2012). Algiphilus aromaticivorans gen. Nov., sp. nov., an aromatic hydrocarbon-degrading bacterium isolated from a culture of the marine dinoflagellate Lingulodinium polyedrum, and proposal of Algiphilaceae fam. Nov. Int. J. Syst. Evol. Microbiol. 62, 2743–2749. doi: 10.1099/ijs.0.033324-0
Harwati, T. U., Kasai, Y., Kodama, Y., Susilaningsih, D., and Watanabe, K. (2009). Tropicimonas isoalkanivorans gen. Nov., sp. nov., a branched-alkane-degrading bacterium isolated from Semarang port in Indonesia. Int. J. Syst. Evol. Microbiol. 59, 388–391. doi: 10.1099/ijs.0.65822-0
Hoch, M. P., Snyder, R. A., Jeffrey, W. H., Dillon, K. S., and Coffin, R. B. (2006). Expression of glutamine synthetase and glutamate dehydrogenase by marine bacterioplankton: assay optimizations and efficacy for assessing nitrogen to carbon metabolic balance in situ. Limnol. Oceanogr. Methods 4, 308–328. doi: 10.4319/lom.2006.4.308
Huerta-Cepas, J., Szklarczyk, D., Heller, D., Hernández-Plaza, A., Forslund, S. K., Cook, H., et al. (2019). EggNOG 5.0: a hierarchical, functionally and phylogenetically annotated orthology resource based on 5090 organisms and 2502 viruses. Nucleic Acids Res. 47, D309–D314. doi: 10.1093/nar/gky1085
Imachi, H., Nobu, M. K., Nakahara, N., Morono, Y., Ogawara, M., Takaki, Y., et al. (2020). Isolation of an archaeon at the prokaryote–eukaryote interface. Nature 577, 519–525. doi: 10.1038/s41586-019-1916-6
Jain, C., Rodriguez-R, L. M., Phillippy, A. M., Konstantinidis, K. T., and Aluru, S. (2018). High throughput ANI analysis of 90K prokaryotic genomes reveals clear species boundaries. Nat. Commun. 9, 5114–5118. doi: 10.1038/s41467-018-07641-9
Jiang, H., Lei, R., Ding, S. W., and Zhu, S. (2014). Skewer: a fast and accurate adapter trimmer for next-generation sequencing paired-end reads. BMC Bioinformatics 15, 1–12. doi: 10.1186/1471-2105-15-182
Jin, Z., Sato, Y., Kawashima, M., and Kanehisa, M. (2023). KEGG tools for classification and analysis of viral proteins. Protein Sci. 32, e4820–e4828. doi: 10.1002/pro.4820
Kawano, K., Ushijima, N., Kihara, M., and Itoh, H. (2020). Patiriisocius marinistellae gen. Nov., sp. nov., isolated from the starfish Patiria pectinifera, and reclassification of Ulvibacter marinus as a member of the genus Patiriisocius comb. nov. Int. J. Syst. Evol. Microbiol. 70, 4119–4129. doi: 10.1099/ijsem.0.004254
Kearse, M., Moir, R., Wilson, A., Stones-Havas, S., Cheung, M., Sturrock, S., et al. (2012). Geneious basic: an integrated and extendable desktop software platform for the organization and analysis of sequence data. Bioinformatics 28, 1647–1649. doi: 10.1093/bioinformatics/bts199
Kim, J., Na, S. I., Kim, D., and Chun, J. (2021). UBCG2: up-to-date bacterial core genes and pipeline for phylogenomic analysis. J. Microbiol. 59, 609–615. doi: 10.1007/s12275-021-1231-4
Kim, D., Park, S., and Chun, J. (2021). Introducing EzAAI: a pipeline for high throughput calculations of prokaryotic average amino acid identity. J. Microbiol. 59, 476–480. doi: 10.1007/s12275-021-1154-0
Lappan, R., Shelley, G., Islam, Z. F., Leung, P. M., Lockwood, S., Nauer, P. A., et al. (2023). Molecular hydrogen in seawater supports growth of diverse marine bacteria. Nat. Microbiol. 8, 581–595. doi: 10.1038/s41564-023-01322-0
Lee, H. S., Kwon, K. K., Yang, S. H., Bae, S. S., Park, C. H., Kim, S. J., et al. (2008). Description of Croceitalea gen. Nov. in the family Flavobacteriaceae with two species, Croceitalea eckloniae sp. nov. and Croceitalea dokdonensis sp. nov., isolated from the rhizosphere of the marine alga Ecklonia kurome. Int. J. Syst. Evol. Microbiol. 58, 2505–2510. doi: 10.1099/ijs.0.65697-0
Lei, S., Pulakat, L., and Gavini, N. (1999). Genetic analysis of nif regulatory genes by utilizing the yeast two- hybrid system detected formation of a NifL-NifA complex that is implicated in regulated expression of nif genes. J. Bacteriol. 181, 6535–6539. doi: 10.1128/jb.181.20.6535-6539.1999
Letunic, I., and Bork, P. (2021). Interactive tree of life (iTOL) v5: an online tool for phylogenetic tree display and annotation. Nucleic Acids Res. 49, W293–W296. doi: 10.1093/nar/gkab301
Li, H. (2013). Aligning sequence reads, clone sequences and assembly contigs with BWA-MEM. arXiv, 1–3. Available at: http://arxiv.org/abs/1303.3997.
Locey, K. J., and Lennon, J. T. (2016). Scaling laws predict global microbial diversity. Proc. Natl. Acad. Sci. USA 113, 5970–5975. doi: 10.1073/pnas.1521291113
Lockwood, S., Greening, C., Baltar, F., and Morales, S. E. (2022). Global and seasonal variation of marine phosphonate metabolism. ISME J. 16, 2198–2212. doi: 10.1038/s41396-022-01266-z
Luo, H., Benner, R., Long, R. A., and Hu, J. (2009). Subcellular localization of marine bacterial alkaline phosphatases. Proc. Natl. Acad. Sci. USA 106, 21219–21223. doi: 10.1073/pnas.0907586106
Mabeau, S., and Kloareg, B. (1987). Isolation and analysis of the cell walls of brown algae: Fucus spiralis, F. ceranoides, F. vesiculosus, F. serratus, Bifurcaria bifurcata and Laminaria digitata. J. Exp. Bot. 38, 1573–1580. doi: 10.1093/jxb/38.9.1573
Macián, M. C., Lucena, T., Arahal, D. R., Ruvira, M. A., Aznar, R., and Pujalte, M. J. (2021). Pseudidiomarina piscicola sp. nov., isolated from cultured European seabass, Dicenthrarchus labrax. Arch. Microbiol. 203, 1293–1298. doi: 10.1007/s00203-020-02131-3
Martínez, A., Osburne, M. S., Sharma, A. K., Delong, E. F., and Chisholm, S. W. (2012). Phosphite utilization by the marine picocyanobacterium Prochlorococcus MIT9301. Environ. Microbiol. 14, 1363–1377. doi: 10.1111/j.1462-2920.2011.02612.x
Martiny, A. C. (2019). High proportions of bacteria are culturable across major biomes. ISME J. 13, 2125–2128. doi: 10.1038/s41396-019-0410-3
McCammon, S., and Bowman, J. (2000). Taxonomy of Antarctic Flavobacterium species: description of Flavobacterium gillisiae sp. nov., Flavobacterium tegetincola sp. nov., and Flavobacterium xanthum sp. nov., nom. Rev. Int. J. Syst. Evol. Microbiol. 50, 1055–1063. doi: 10.1099/00207713-50-3-1055
Meier-Kolthoff, J. P., Auch, A. F., Klenk, H. P., and Göker, M. (2013). Genome sequence-based species delimitation with confidence intervals and improved distance functions. BMC Bioinformatics 14:60. doi: 10.1186/1471-2105-14-60
Meier-Kolthoff, J. P., and Göker, M. (2019). TYGS is an automated high-throughput platform for state-of-the-art genome-based taxonomy. Nat. Commun. 10:2182. doi: 10.1038/s41467-019-10210-3
Meyer, B., Imhoff, J. F., and Kuever, J. (2007). Molecular analysis of the distribution and phylogeny of the soxB gene among sulfur-oxidizing bacteria - evolution of the sox sulfur oxidation enzyme system. Environ. Microbiol. 9, 2957–2977. doi: 10.1111/j.1462-2920.2007.01407.x
Mistry, J., Chuguransky, S., Williams, L., Qureshi, M., Salazar, G. A., Sonnhammer, E. L. L., et al. (2021). Pfam: the protein families database in 2021. Nucleic Acids Res. 49, D412–D419. doi: 10.1093/nar/gkaa913
Moran, M. A., Reisch, C. R., Kiene, R. P., and Whitman, W. B. (2012). Genomic insights into bacterial DMSP transformations. Annu. Rev. Mar. Sci. 4, 523–542. doi: 10.1146/annurev-marine-120710-100827
Nedashkovskaya, O. I., Kim, S. B., Han, S. K., Snauwaert, C., Vancanneyt, M., Swings, J., et al. (2005). Winogradskyella thalassocola gen. Nov., sp. nov., Winogradskyella epiphytica sp. nov. and Winogradskyella eximia sp. nov., marine bacteria of the family Flavobacteriaceae. Int. J. Syst. Evol. Microbiol. 55, 49–55. doi: 10.1099/ijs.0.63307-0
Nedashkovskaya, O. I., Vancanneyt, M., Kim, S. B., and Zhukova, N. V. (2009). Winogradskyella echinorum sp. nov., a marine bacterium of the family Flavobacteriaceae isolated from the sea urchin Strongylocentrotus intermedius. Int. J. Syst. Evol. Microbiol. 59, 1465–1468. doi: 10.1099/ijs.0.005421-0
Newton, R. J., Griffin, L. E., Bowles, K. M., Meile, C., Gifford, S., Givens, C. E., et al. (2010). Genome characteristics of a generalist marine bacterial lineage. ISME J. 4, 784–798. doi: 10.1038/ismej.2009.150
Nicholson, A. C., Gulvik, C. A., Whitney, A. M., Humrighouse, B. W., Bell, M. E., Holmes, B., et al. (2020). Division of the genus Chryseobacterium: observation of discontinuities in amino acid identity values, a possible consequence of major extinction events, guides transfer of nine species to the genus Epilithonimonas, eleven species to the genus Kaistella, and three species to the genus Halpernia gen. Nov., with description of Kaistella daneshvariae sp. nov. and Epilithonimonas vandammei sp. nov. derived from clinical speciments. Int. J. Syst. Evol. Microbiol. 70, 4432–4450. doi: 10.1099/ijsem.0.003935
Orchard, E. D., Webb, E. A., and Dyhrman, S. T. (2009). Molecular analysis of the phosphorus starvation response in Trichodesmium spp. Environ. Microbiol. 11, 2400–2411. doi: 10.1111/j.1462-2920.2009.01968.x
Page, K. A., Connon, S. A., and Giovannoni, S. J. (2005). Representative freshwater Bacterioplankton isolated from crater Lake, Oregon. Appl. Environ. Microbiol. 70:22. doi: 10.1128/AEM.70.11.6542
Park, S. J., Cha, I. T., Kim, S. J., Shin, K. S., Hong, Y. S., Roh, D. H., et al. (2012). Salinisphaera orenii sp. nov., isolated from a solar saltern. Int. J. Syst. Evol. Microbiol. 62, 1877–1883. doi: 10.1099/ijs.0.028647-0
Park, S., Choi, J., Won, S. M., and Yoon, J. H. (2018). Thalassotalea insulae sp. nov., isolated from tidal flat sediment. Int. J. Syst. Evol. Microbiol. 68, 1321–1326. doi: 10.1099/ijsem.0.002671
Park, S., Song, J., Yoshizawa, S., Choi, A., Cho, J. C., and Kogure, K. (2013). Rubrivirga marina gen. Nov., sp. nov., a member of the family Rhodothermaceae isolated from deep seawater. Int. J. Syst. Evol. Microbiol. 63, 2229–2233. doi: 10.1099/ijs.0.046318-0
Parker, C. T., Tindall, B. J., and Garrity, G. M. (2019). International code of nomenclature of prokaryotes. Int. J. Syst. Evol. Microbiol. 69, S1–S111. doi: 10.1099/ijsem.0.000778
Parks, D. H., Chuvochina, M., Rinke, C., Mussig, A. J., Chaumeil, P. A., and Hugenholtz, P. (2022). GTDB: an ongoing census of bacterial and archaeal diversity through a phylogenetically consistent, rank normalized and complete genome-based taxonomy. Nucleic Acids Res. 50, D785–D794. doi: 10.1093/nar/gkab776
Parks, D. H., Imelfort, M., Skennerton, C. T., Hugenholtz, P., and Tyson, G. W. (2015). CheckM: assessing the quality of microbial genomes recovered from isolates, single cells, and metagenomes. Genome Res. 25, 1043–1055. doi: 10.1101/gr.186072.114
Peltzer, A., Straub, D., Bot, N., Garcia, M. U., Taylor, B., Angelov, A., et al. (2021). nf-core/bacass: v2.0.0 nf-core/bacass: “Navy Steel Swordfish.”. Zenodo. doi: 10.5281/zenodo.5289278
Pesant, S., Not, F., Picheral, M., Kandels-Lewis, S., Le Bescot, N., Gorsky, G., et al. (2015). Open science resources for the discovery and analysis of Tara oceans data. Sci. Data 2, 150023–150016. doi: 10.1038/sdata.2015.23
Pruesse, E., Peplies, J., and Glöckner, F. O. (2012). SINA: accurate high-throughput multiple sequence alignment of ribosomal RNA genes. Bioinformatics 28, 1823–1829. doi: 10.1093/bioinformatics/bts252
Pujalte, M. J., Lucena, T., Rodrigo-Torres, L., and Arahal, D. R. (2018). Comparative genomics of Thalassobius including the description of Thalassobius activus sp. nov., and Thalassobius autumnalis sp. nov. Front. Microbiol. 8, 1–15. doi: 10.3389/fmicb.2017.02645
Rey-Velasco, X., Deulofeu-Vapo, O., Sanz-Sáez, I., Cardelús, C., Ferrera, I., Gasol, J. M., et al. (2023). Expanding success in the isolation of abundant marine bacteria after reduction in grazing and viral pressure and increase in nutrient availability. Microbiol. Spectr. 11:e0089023. doi: 10.1128/spectrum.00890-23
Rodriguez-R, L. M., and Konstantinidis, K. T. (2016). The enveomics collection: a toolbox for specialized analyses of microbial genomes and metagenomes. PeerJ Prepr. 4:e1900v1. doi: 10.7287/peerj.preprints.1900v1
Sánchez, O., Ferrera, I., Mabrito, I., Gazulla, C. R., Sebastián, M., Auladell, A., et al. (2020). Seasonal impact of grazing, viral mortality, resource availability and light on the group-specific growth rates of coastal Mediterranean bacterioplankton. Sci. Rep. 10:19773. doi: 10.1038/s41598-020-76590-5
Satinsky, B. M., Smith, C. B., Sharma, S., Ward, N. D., Krusche, A. V., Richey, J. E., et al. (2017). Patterns of bacterial and archaeal gene expression through the lower Amazon River. Front. Mar. Sci. 4, 1–15. doi: 10.3389/fmars.2017.00253
Sebastian, M., and Ammerman, J. W. (2009). The alkaline phosphatase PhoX is more widely distributed in marine bacteria than the classical PhoA. ISME J. 3, 563–572. doi: 10.1038/ismej.2009.10
Seemann, T. (2014). Prokka: rapid prokaryotic genome annotation. Bioinformatics 30, 2068–2069. doi: 10.1093/bioinformatics/btu153
Shen, W., Le, S., Li, Y., and Hu, F. (2016). SeqKit: a cross-platform and ultrafast toolkit for FASTA/Q file manipulation. PLoS One 11, 1–10. doi: 10.1371/journal.pone.0163962
Shin, N. R., Roh, S. W., Kim, M. S., Yun, B., Whon, T. W., Kim, Y. O., et al. (2012). Tropicimonas sediminicola sp. nov., isolated from marine sediment. Int. J. Syst. Evol. Microbiol. 62, 2424–2429. doi: 10.1099/ijs.0.037929-0
Shin, S. K., and Yi, H. (2020). Urechidicola croceus gen. Nov., sp. nov., a member of the family Flavobacteriaceae. Int. J. Syst. Evol. Microbiol. 70, 1751–1757. doi: 10.1099/ijsem.0.003966
Song, J., Joung, Y., Park, S., Cho, J. C., and Kogure, K. (2016). Rubrivirga profundi sp. nov., isolated from deep-sea water, and emended description of the genus Rubrivirga. Int. J. Syst. Evol. Microbiol. 66, 3253–3257. doi: 10.1099/ijsem.0.001182
Steen, A. D., Crits-Christoph, A., Carini, P., DeAngelis, K. M., Fierer, N., Lloyd, K. G., et al. (2019). High proportions of bacteria and archaea across most biomes remain uncultured. ISME J. 13, 3126–3130. doi: 10.1038/s41396-019-0484-y
Straub, D., Tångrot, J., Peltzer, A., Lundin, D., Emnilsson, S. P., et al. (2023). nf-core/ampliseq: Ampliseq Version 2.7.1. Zenodo. doi: 10.5281/zenodo.1493841
Sun, H., Zheng, H., Liao, B., Chen, B., Li, A., and Xiao, B. (2022). Algiphilus acroporae sp. nov. and Coraliihabitans acroporae gen. Nov. sp. nov., isolated from scleractinian coral Acropora digitifera. Int. J. Syst. Evol. Microbiol. 72, 1–13. doi: 10.1099/ijsem.0.005321
Tatusov, R. L., Galperin, M. Y., Natale, D. A., and Koonin, E. V. (2000). The COG database: a tool for genome-scale analysis of protein functions and evolution. Nucleic Acids Res. 28, 33–36. doi: 10.1093/nar/28.1.33
Team, R. C (2022). R: A language and environment for statistical computing. Austria: R. F. for S. Computing Vienna.
Teeling, H., Fuchs, B. M., Becher, D., Klockow, C., Gardebrecht, A., Bennke, C. M., et al. (2012). Substrate-controlled succession of marine bacterioplankton populations induced by a phytoplankton bloom. Science 336, 608–611. doi: 10.1126/science.1218344
Wang, S., Cao, X., Liu, L., Liu, R., Bian, Z., Zhang, X. H., et al. (2023). Stakelama marina sp. nov., isolated from seawater of the Tangyin hydrothermal field in the Okinawa trough. Int. J. Syst. Evol. Microbiol. 73, 1–8. doi: 10.1099/ijsem.0.005902
Whitman, W. B., Coleman, D. C., and Wiebe, W. J. (1998). Prokaryotes: the unseen majority. Proc. Natl. Acad. Sci. USA 95, 6578–6583. doi: 10.1073/pnas.95.12.6578
Wick, R. R., Judd, L. M., Gorrie, C. L., and Holt, K. E. (2017). Unicycler: resolving bacterial genome assemblies from short and long sequencing reads. PLoS Comput. Biol. 13, e1005595–e1005522. doi: 10.1371/journal.pcbi.1005595
Wickham, H., Averick, M., Bryan, J., Chang, W., McGowan, L., François, R., et al. (2019). Welcome to the Tidyverse. J. Open Source Softw. 4:1686. doi: 10.21105/joss.01686
Wong, T. Y., Preston, L. A., and Schiller, N. L. (2000). Alginate lyase: review of major sources and enzyme characteristics, structure-function analysis, biological roles, and applications. Ann. Rev. Microbiol. 54, 289–340. doi: 10.1146/annurev.micro.54.1.289
Wood, D. E., Lu, J., and Langmead, B. (2019). Improved metagenomic analysis with kraken 2. Genome Biol. 20, 257–213. doi: 10.1186/s13059-019-1891-0
Xu, X. W., Wu, Y. H., Wang, C. S., Wang, X. G., Oren, A., and Wu, M. (2009). Croceicoccus marinus gen. Nov., sp. nov., a yellow-pigmented bacterium from deep-sea sediment, and emended description of the family Erythrobacteraceae. Int. J. Syst. Evol. Microbiol. 59, 2247–2253. doi: 10.1099/ijs.0.004267-0
Yoon, S. H., Ha, S. M., Kwon, S., Lim, J., Kim, Y., Seo, H., et al. (2017). Introducing EzBioCloud: a taxonomically united database of 16S rRNA gene sequences and whole-genome assemblies. Int. J. Syst. Evol. Microbiol. 67, 1613–1617. doi: 10.1099/ijsem.0.001755
Yoshizawa, S., Kumagai, Y., Kim, H., Ogura, Y., Hayashi, T., Iwasaki, W., et al. (2014). Functional characterization of flavobacteria rhodopsins reveals a unique class of light-driven chloride pump in bacteria. Proc. Natl. Acad. Sci. USA 111, 6732–6737. doi: 10.1073/pnas.1403051111
Yu, Y., Li, H. R., Zeng, Y. X., Sun, K., and Chen, B. (2012). Pricia Antarctica gen. Nov., sp. nov., a member of the family Flavobacteriaceae, isolated from antarctic intertidal sediment. Int. J. Syst. Evol. Microbiol. 62, 2218–2223. doi: 10.1099/ijs.0.037515-0
Zhang, Y. J., Tang, S. K., Shi, R., Klenk, H. P., Chen, C., Yang, L. L., et al. (2012). Salinisphaera halophila sp. nov., a moderately halophilic bacterium isolated from brine of a salt well. Int. J. Syst. Evol. Microbiol. 62, 2174–2179. doi: 10.1099/ijs.0.035584-0
Zhang, Y., Tang, K., Shi, X., and Zhang, X. H. (2014). Description of Thalassotalea piscium gen. Nov., sp. nov., isolated from flounder (Paralichthys olivaceus), reclassification of four species of the genus Thalassomonas as members of the genus Thalassotalea gen. Nov. and emended description of the genus Tha. Int. J. Syst. Evol. Microbiol. 64, 1223–1228. doi: 10.1099/ijs.0.055913-0
Keywords: taxonomy, genomics, phylogenomics, isolates, marine bacteria, biogeochemistry, biogeography
Citation: Rey-Velasco X, Lucena T, Belda A, Gasol JM, Sánchez O, Arahal DR and Pujalte MJ (2024) Genomic and phenotypic characterization of 26 novel marine bacterial strains with relevant biogeochemical roles and widespread presence across the global ocean. Front. Microbiol. 15:1407904. doi: 10.3389/fmicb.2024.1407904
Edited by:
Bernhard M. Fuchs, Max Planck Society, GermanyReviewed by:
Xue-Wei Xu, National Deep Sea Center (NDSC), ChinaSujata Dabolkar, Government College of Arts, Science and Commerce, India
Tomeu Viver, Spanish National Research Council (CSIC), Spain
Copyright © 2024 Rey-Velasco, Lucena, Belda, Gasol, Sánchez, Arahal and Pujalte. This is an open-access article distributed under the terms of the Creative Commons Attribution License (CC BY). The use, distribution or reproduction in other forums is permitted, provided the original author(s) and the copyright owner(s) are credited and that the original publication in this journal is cited, in accordance with accepted academic practice. No use, distribution or reproduction is permitted which does not comply with these terms.
*Correspondence: David R. Arahal, ZGF2aWQucnVpekB1di5lcw==; Olga Sánchez, b2xnYS5zYW5jaGV6QHVhYi5jYXQ=