- 1State Key Laboratory of Vegetable Biobreeding, Institute of Vegetables and Flowers, Chinese Academy of Agricultural Sciences, Beijing, China
- 2Department of Plant Pathology, College of Plant Protection, China Agricultural University, Beijing, China
- 3Biocontrol Engineering Laboratory of Crop Diseases and Pests of Gansu Province, College of Plant Protection, Gansu Agricultural University, Lanzhou, China
Chemical study of the nematicidal biocontrol fungus Pochonia chlamydosporia PC-170 led to discovery of six resorcylic acid lactones (RALs), including three nematicidal glycosylated RALs, monocillin VI glycoside (1), colletogloeolactone A (2) and monocillin II glycoside (3), and three antibacterial non-glycosylated RALs, monocillin VI (4), monocillin IV (5) and monocillin II (6). The planar structure of the new compound monocillin VI glycoside (1) was elucidated using HRESIMS and NMR data, and its monosaccharide configuration was further determined through sugar hydrolysis experiment and GC–MS analysis method. Furthermore, their two biosynthetic-related PKS genes, pchE and pchI, were identified through the gene knockout experiment. The glycosylated RALs 1–3 exhibited nematicidal activity against Meloidogyne incognita, with LC50 values of 94, 152 and 64 μg/mL, respectively, and thus had great potential in the development of new nematicidal natural products to control M. incognita in the future.
1 Introduction
Meloidogyne incognita, a phytopathogenic nematode, adversely influences crop growth via its parasitic activity predominantly within the root system of plants (Li et al., 2015). The manifestations of this infestation may include plant stunting, leaf yellowing, and other associated symptoms, with severe instances culminating in plant mortality (Jones et al., 2013; Goverse and Smant, 2014). Simultaneously, the injury caused by the nematode is thought to create a favorable environment for the proliferation of other phytopathogens. It is crucial to implement preventive or restrictive measures to control the spread of this pest. Currently, chemical control methods are predominantly utilized to manage M. incognita, emphasizing the urgent need to explore natural products exhibiting nematicidal properties.
Pochonia chlamydosporia, a facultative parasitic fungus, manifests a preference for parasitizing root-knot nematode egg masses and females while also displaying significant potential as a biocontrol agent against M. incognita (Khan et al., 2021; Bo et al., 2022), attracting considerable research attention (Manzanilla-López et al., 2013; Zhuang et al., 2021). It was reported that the main secondary metabolites produced by P. chlamydosporia mainly belong to resorcylic acid lactones (RALs), which exhibited various biological activities, including antiviral (Hellwig et al., 2003), antiparasitic (Hellwig et al., 2003), cytotoxic (Sugawara et al., 1992), antimalarial (Isaka et al., 2002), antifungal (Xu et al., 2012) and antibacterial (Qin et al., 2019). We initially investigated a strain of P. chlamydosporia PC-170, and identified seven RALs with modest antibacterial activities against the plant pathogen Xanthomonas campestris (Qin et al., 2019). However, after nematicidal activity evaluation, none of these RALs showed efficacy against M. incognita. Since the crude extract of the fermentation cultures of P. chlamydosporia PC-170 still showed strong inhibitory activity against M. incognita, we continued screening for the potent nematicidal natural products derived from the crude extract of PC-170. Bioassay-guided fractionation of this extract led to the identification of six RALs compounds, including a new RAL, monocillin VI glycoside (1), together with five known analogs, colletogloeolactone A (2), monocillin II glycoside (3), monocillin VI (4), monocillin IV (5) and monocillin II (6). Three glycosides (1–3) exhibited noteworthy nematicidal activity with inhibitory activity against M. incognita with LC50 values of 94, 152 and 64 μg/mL, respectively. In this paper, we report the isolation and structure elucidation of these RALs and their nematicidal activities. Moreover, we also confirmed the functions of two key radicicol biosynthetic genes (pchE and pchI) in the P. chlamydosporia strain PC-170. This study contributes valuable insights into the management of M. incognita and serves as a fundamental basis for developing nematicidal natural products.
2 Materials and methods
2.1 Materials
2.1.1 Experimental procedures
Semi-preparative high-performance liquid chromatography (HPLC) was performed on an Agilent 1,260 G7111A Quaternary Pump equipped with a G7117C diode array detector (DAD). Agilent Technologies 5977A GC/MSD was used for monosaccharide configuration determination. 1H and 13C nuclear magnetic resonance (NMR) data were acquired with a Bruker AVANCE 500 MHz spectrometer with a 5 mm triple resonance cryoprobe at 298 K. The referenced NMR solvent signals were used as acetone-d6, δH 2.05/δC 29.8, 206.2, and methanol-d4, δH 3.31, 4.87/δC 49.0, respectively. Heteronuclear multiple quantum correlation (HMQC) and heteronuclear multiple bond correlation (HMBC) experiments were optimized for 145 and 8 Hz, respectively. High-resolution electrospray ionization mass spectrometry (HRESIMS) data were recorded using an Agilent Accurate-Mass-Q-TOF LC/MS 6520 instrument equipped with an electrospray ionization (ESI) source. Fragmentor and capillary voltages were maintained at 125 and 3,500 V, respectively. Nitrogen was used as the nebulizing and drying gas (300°C) with a flow rate of 10 L/min, and the nebulizer pressure was set at 10 psi. Full-scan spectra were acquired across a scan range of m/z 100–1,000 at a rate of 1.03 spectra per second. Optical rotations were measured on an Anton-Paar MCP 200 polarometer. Circular dichroism (CD) spectra were recorded using a Chirascan spectropolarimeter.
2.1.2 Chemicals, strain, and fermentation
The deuterium reagents were used from Cambridge Isotope Laboratories, Inc. (USA). The agar powder and PDA medium were used from Becton, Dickinson Co., Ltd. (USA). Yeast extracts and peptones were used from Oxoid, Inc. (UK). Driselase and PEG4000 were used from Sigma-Aldrich Trading Co., Ltd. (China). Miracloth was used from Merck-Calbiochem (Germany). The silica gel for column chromatography was used from Qingdao Haiyang Chemical Co., Ltd. (China). The monosaccharides and silanization reagents (L-cysteine methyl ester hydrochloride and N-trimethylsilylimidazole) were used from Shanghai Macklin Biochemical Technology Co., Ltd. (China). All molecular kits were purchased from Vazyme Biotech Co., Ltd. (China). All organic reagents were purchased from Thermo Fisher Scientific, Inc. (USA). The fungal strain P. chlamydosporia PC-170 was used for the study, and its solid (rice medium) fermentation method was the same as we described before (Qin et al., 2019).
2.2 Methods
2.2.1 Extraction, isolation, and purification
The P. chlamydosporia PC-170 fermentation sample was extracted twice with ethyl acetate (EtOAc) and then recovered using a rotary evaporator to obtain the crude extract of 8.4 g. The crude extract was separated by reduced-pressure silica gel column chromatography using the gradient petroleum ether/EtOAc as the elution solvent, leading to 20 fractions named Fr1-Fr20 (EtOAc/petroleum ether at the following ratios: 0, 1, 3, 5, 8, 10, 15, 20, 25, 30, 35, 40, 45, 50, 55, 60, 70, 80, 90, and 100%, respectively). The 80 and 90% EtOAc eluted fractions (Fr18 and Fr19) were combined (100 mg) and further purified by RP-HPLC (Kromasil C18 column, 10 μm, 10 × 250 mm, 30% MeCN in H2O over 15 min, 45% MeCN in H2O over 20 min, and then 100% MeCN over10 min, 2 mL/min) to afford 1 (4.3 mg, tR 16.6 min), 3 (4 mg, tR 30.1 min) and 2 (5.7 mg, tR 37.9 min), respectively. The 20% EtOAc eluted fraction (200 mg) was purified by RP-HPLC (60% MeCN in H2O over 20 min, and then 100% MeCN over10 min, 2 mL/min) to afford 6 (5 mg, tR 15.5 min) and 5 (4 mg, tR 22.8 min), respectively. The 30% EtOAc eluted fraction (300 mg) was purified by RP-HPLC (45% MeCN in H2O over 20 min, and then 100% MeCN over 10 min, 2 mL/min) to afford 4 (5 mg, tR 14.6 min).
Monocillin VI glycoside (1): white powder; [α]25D + 122.98 (c 0.1, MeOH); UV (MeOH) λmax (log ε), 225 (3.47), 260 (3.53), 313 (3.34) nm; CD (c 2.0 μM, MeOH) λmax (Δε), 207 (+19.18), 221 (−3.48), 230 (−0.23), 300 (+22.60) nm; 1H, 13C, and HMBC NMR data for 1 are listed in Table 1; HRESIMS, m/z 489.1737 [M + Na]+ (calculated for C23H30O10Na+, 489.1731).
Colletogloeolactone A (2): white powder; [α]25D + 123.98 (c 0.1, MeOH); UV (MeOH) λmax (log ε), 216 (3.34), 262 (3.04), 302 (2.70) nm; CD (c 2.1 μM, MeOH) λmax (Δε), 207 (+7.83), 216 (−13.03), 225 (+7.88), 298 (+20.21) nm; HRESIMS, m/z 473.1798 [M + Na]+ (calculated for C23H30O9Na+, 473.1782).
Monocillin II glycoside (3): white powder; [α]25D + 120.98 (c 0.1, MeOH); UV (MeOH) λmax (log ε), 215 (3.43), 258 (3.14), 313 (2.71) nm; CD (c 2.0 μM, MeOH) λmax (Δε), 208 (+12.65), 237 (−27.87), 262 (+20.62), 316 (+8.03) nm; HRESIMS, m/z 471.1630 [M + Na]+ (calculated for C23H28O9Na+, 471.1626).
2.2.2 Hydrolysis and determination of the absolute configuration of sugar moieties
Each compound (3 mg) was hydrolyzed by heating in MeOH (3 mL) and 3 M HCl (3 mL) at 70°C for 6 h. After removing MeOH and HCl by evaporation, the reaction mixture was diluted with H2O (10 mL) and extracted with EtOAc. The aqueous phase was evaporated in a vacuum to obtain the monosaccharide residue. The monosaccharide residue (1 mg) was heated in pyridine (1 mL) and 2 mg of L-cysteine methyl ester hydrochloride at 60°C for 2.5 h. The solvent was evaporated, and N-trimethylsilylimidazole (TSIM; 0.2 mL) was added to trimethylsilylate at 60°C for 2.5 h. The mixture was extracted using hexane and water (1 mL) (Lv et al., 2016). Similarly, the standard D-ribose and L-ribose were treated following the same procedure. The hexane extract obtained was subjected to gas chromatography–mass spectrometry (Agilent DB-1701 gas chromatographic column; 30 m × 250 μm × 0.25 μm; 1.5 mL/min) using the following conditions: injection temperature of 210°C; detector temperature of 250°C; initial column temperature of 200°C raised to 280°C at the rate of 60°C/min and maintained at 280°C for 35 min under N2 carrier gas.
2.2.3 Nematicidal activity assay
The collection of J2 nematodes was carried out according to a previously described procedure (Yin et al., 2021). The concentration of nematodes was adjusted to 1,000 strips/mL using sterile water for observation under a microscope. The abamectin (50 μg/mL) was used as the positive control, while the concentrations tested for fractions Fr1-Fr20 were 2 mg/mL. The lethal concentration 50% (LC50) of the compounds was determined. The compounds were prepared in five concentrations: 10 mg/mL, 7 mg/mL, 5 mg/mL, 2.5 mg/mL and 1.25 mg/mL. Each well contained 100 μL (5 μL sample added to 95 μL nematodes) with three biological and five technical repetitions (Seo et al., 2014). The 96-well plates were incubated at 28°C, and nematode morphology was assessed under a microscope after 24 h. Nematodes were stimulated with 0.5 M NaOH and were considered dead if they remained in a rigid morphology. Regression analysis was conducted using logarithmic values of compound concentrations and their corresponding corrected mortality values. Based on the regression curve equations, the LC50 of the compounds was calculated. Mortality and corrected mortality rates were computed as follows:
2.2.4 DNA isolation, vector construction, and gene knockout
The genomic DNA of wild-type P. chlamydosporia PC-170 was extracted using FastPure Microbiome DNA Isolation Kit (Vazyme, China). The upstream arm (1,500 bp) and downstream arm (1,500 bp) of the knockout gene were amplified using the high-fidelity 2 × Phanta Flash Master Mix (Vazyme, China) with PC-170 genomic DNA as a template to obtain the UP and DOWN fragments. The pUC-Cas9-neo vector (Luo et al., 2023) was used as the template for amplify the NEO-resistant fragment. All amplicons were combined and ligated into pUC19 (Supplementary Figure S7A) using ClonExpress MultiS One Step Cloning Kit (Vazyme, China) to construct the knockout vector (Supplementary Figure S7B).
The knockout of targeted genes was performed through the PEG-mediated transformation of fungal protoplasts (Supplementary Figure S7C; Bok and Keller, 2004; Li et al., 2006). Freshly germinated conidia were collected on Miracloth, rinsed with 0.7 M NaCl, and digested with 20 mg/mL driselase for 3.5 h. Enzyme lysis products were filtered by Miracloth and rinsed with STC buffer (145.6 g/L Sorbitol, 7.4 g/L CaCl2·2H2O, 1.6 g/L Tris–HCl). The protoplast concentration was adjusted to 1 × 107 with STC buffer. About 120 μL of protoplast was incubated with 5 μg of linearized DNA which was supplemented with STC buffer to 60 μL on ice for 20 min. Then, 160 μL of PEG4000 was added and incubated at room temperature for 15 min. Total 20 μL of the protoplasts treated as above was transferred into 5 mL of T-TOP medium (0.5 g/L KCl, 0.5 g/L MgSO4·7H2O, 1.0 g/L KH2PO4, 2.0 g/L NaNO3, 200.0 g/L sucrose, 20.0 g/L glucose, 10 g/L agar powder) and then spread evenly on PDA dishes. After cultured at 28°C for 24 h, the plates were covered with 10 mL T-TOP containing 400 μg/mL G418. After incubation at 28°C for 3–5 days, the transformants were transferred to new PDA plates with G418. All transformants were sub-cultured for 3 generations to obtain stable transformants. All mutants were identified by diagnostic PCR. All primers and plasmids used in this study are described in Supplementary Table S1.
3 Results
3.1 In vitro nematicidal activity of the crude extract from Pochonia chlamydosporia PC-170
The in vitro nematicidal activity of the crude extract from P. chlamydosporia PC-170 was evaluated on M. incognita J2s mortality. The EtOAc crude extract (8.4 g) was separated by reduced-pressure silica gel column chromatography to get 20 fractions (Fr1-Fr20). After in vitro nematicidal activity test, fractions Fr18 and Fr19 showed the highest nematicidal activity (mortality rates at 92.4 and 92.6%, respectively), in line with that of the positive control abamectin (50 μg/mL) against J2s of M. incognita (mortality rates at 93.3%), while other fractions showed a relative lower nematicidal activity, and the crude extract from the pure medium showed no nematicidal activity (Figure 1). This result indicated that some natural products in the crude extract of P. chlamydosporia PC-170 had a nematicidal activity that required further isolation and identification.
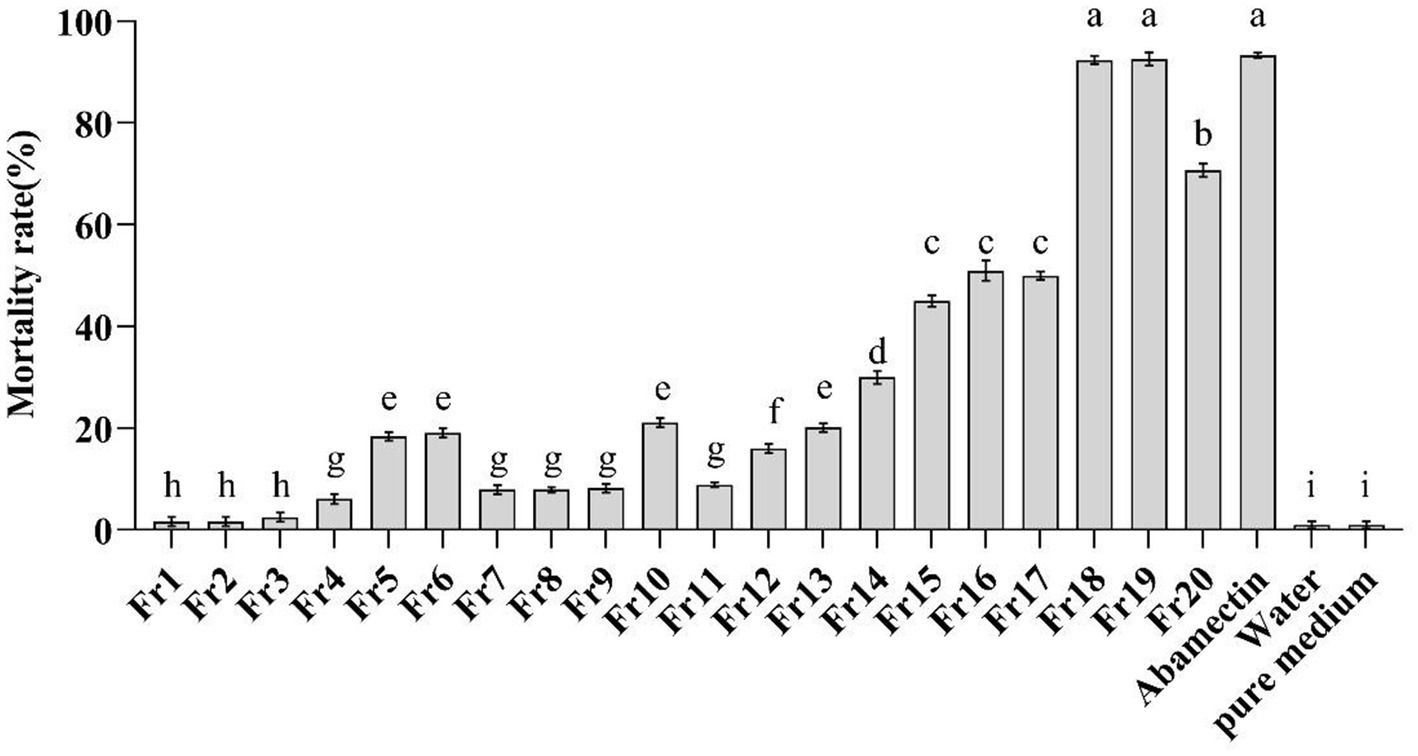
Figure 1. In-vitro test of the mortality of the J2s of M. incognita. Fr1-Fr20 (2 mg/ml) were as the treatment group, abamectin (50 μg/ml) was as the positive control, and water and the pure medium extract were as the negative control. The error bars represent SEs (n = 15) for three biological and five technical repetitions and different lowercase letters indicate significant differences between treatments (p < 0.05).
3.2 Purification and structural identification of RALs
Six RALs compounds were isolated by RP-HPLC from the nematicidal activity fractions. The known compounds 2–6 (Figure 2) were identified as colletogloeolactone A (2) (Kim et al., 2023), monocillin II glycoside (3) (Hellwig et al., 2003), monocillin VI (4) (Qin et al., 2019), monocillin IV (5) (Ayer et al., 1980) and monocillin II (6) (Ayer et al., 1980) respectively, by comparison of their NMR (Supplementary Figures S2–S6) and MS data (Supplementary Figures S8B–S8F) to those previously reported.
The new compound monocillin VI glycoside (1) was assigned the molecular formula C23H30O10 (nine degrees of unsaturation) based on HRESIMS (Supplementary Figure S8A, m/z 489.1737 [M + Na]+). Analysis of its 1H, 13C, and HMBC NMR spectroscopic data (Table 1; Supplementary Figure S1) revealed the presence of one methyl group, five methylenes, two oxymethines, eight aromatic/olefinic carbons (four of which were protonated and two of which were attached to oxygen), one carboxyl carbon (δC 171.8), one ketone carbon (δC 210.5), and one pentose sugar unit. Its NMR spectrum feature revealed that 1 possessed similar fragments to those of other RAL analogs (Figure 2). Interpretation of the 1H–1H correlation spectroscopy (COSY) and HMBC NMR data (Figure 3) for 1 established its main structure as a 14-membered resorcylic acid lactone ring, which was an analog of the known compound monocillin VI (4) (Qin et al., 2019), a metabolite that was co-isolated from this extract. Comparison of the NMR spectroscopic data of 1 (Table 1) with those of 4 suggested that they possess the same monocillin VI moiety, but 1 contains one more pentose sugar substituted unit. 1H–1H COSY and HSQC data of 1 identified an isolated spin-system of C-1’–C-5′, and HMBC correlations from H2-5′ to C-3′ and C-4′, and from H-1′ to C-4′, confirmed the pentose sugar moiety (C-1’–C-5′), whereas a key HMBC correlation from H-1′ to C-5 indicated that the pentose sugar moiety must be attached to C-5 of the 2,4-dihydroxybenzoate unit. On the basis of these data, the gross structure of monocillin VI glycoside was established, as shown in 1. The geometry of the disubstituted double bond (C-14/C-15) in the side chain was deduced to be trans from the large coupling constant (J14,15 = 15.7 Hz) of the olefinic protons. The absolute configurations of the C-16 secondary alcohol and C-17 in 1 were assigned to be the same as that of compound 4 because of the identical CD curves between compounds 1 and 4 (Figure 4) and the positive specific rotation values for both compounds 1 and 4. Sugar hydrolysis experiment (Lv et al., 2016) was applied to assign the absolute configuration of the pentose sugar residue resulting from acid hydrolysis of monocillin VI glycoside (1). The pentose sugar residue present in the acid hydrolyzate of 1 was analyzed by GC–MS along with those of authentic D- and L-pentose sugars (Figure 5). After analysis, the pentose sugar residue in 1 was determined to be D-ribose.
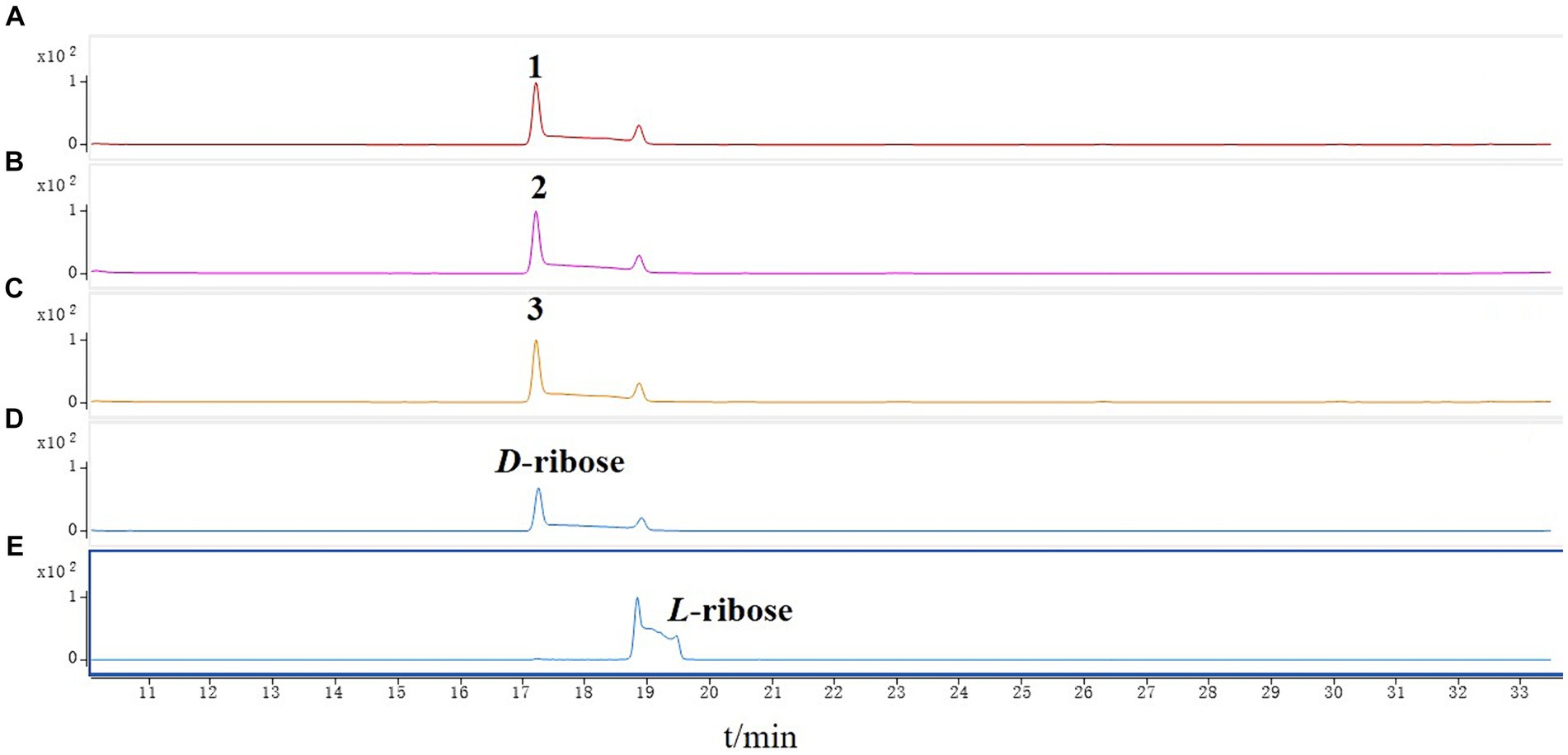
Figure 5. GC–MS analysis of the silylated monosaccharide of (A) monocillin VI glycoside (1), (B) colletogloeolactone A (2), (C) monocillin II glycoside (3), (D) D-ribose, (E) L-ribose.
Structurally, the known compounds colletogloeolactone A (2) and monocillin II glycoside (3) were the glycosylation derivatives of compounds monocillin IV (5) and monocillin II (6), respectively. However, the absolute configurations of the pentose sugar residues in 2 and 3 were still unknown. We applied the sugar hydrolysis experiment and GC–MS analysis method to determine both of the pentose sugar residues in 2 and 3 were also D-ribose (Figure 5). Therefore, the structures of 2 and 3 were determined to be 5-D-ribose monocillin IV and 5-D-ribose monocillin II, respectively.
3.3 Nematicidal activity of RALs
All of these compounds 1–6 were tested for nematicidal activity against M. incognita. The glycosylated compounds 1–3 showed a modest inhibitory effect, with LC50 values of 94, 152, and 64 μg/mL, respectively. The non-glycosylated compounds 4–6 showed no nematicidal activity above 1,000 μg/mL (Supplementary Table S2).
3.4 Identification of the key biosynthetic genes and validation of mutant strains
In a previously published paper, we predicted the gene cluster for the synthesis of RALs compounds in P. chlamydosporia PC-170 (Qin et al., 2019). Among them, the pchE (nrPKS) and pchI (hrPKS) genes were proposed to be the two key biosynthetic genes (Figure 6A). Herein, we successfully constructed the knockout vectors pUC19-PcSM08964 and pUC19-PcSM08959 and obtained mutant strains ΔpchE and ΔpchI through homologous recombination (Supplementary Figure S9). The crude extracts of the wild-type and mutant strains were analyzed by HPLC under the same conditions. The results showed that all six compounds, including the nematicidal glycosylated compounds, disappeared in the mutant strains compared to the wild-type (Figure 6B). Additionally, the nematicidal activity of the mutant strains also disappeared (Figure 6C). Based on the above results, the RALs biosynthesis gene cluster was validated, and the two PKS genes, pchE and pchI, were confirmed to be the RALs biosynthetic core genes (Figure 6).
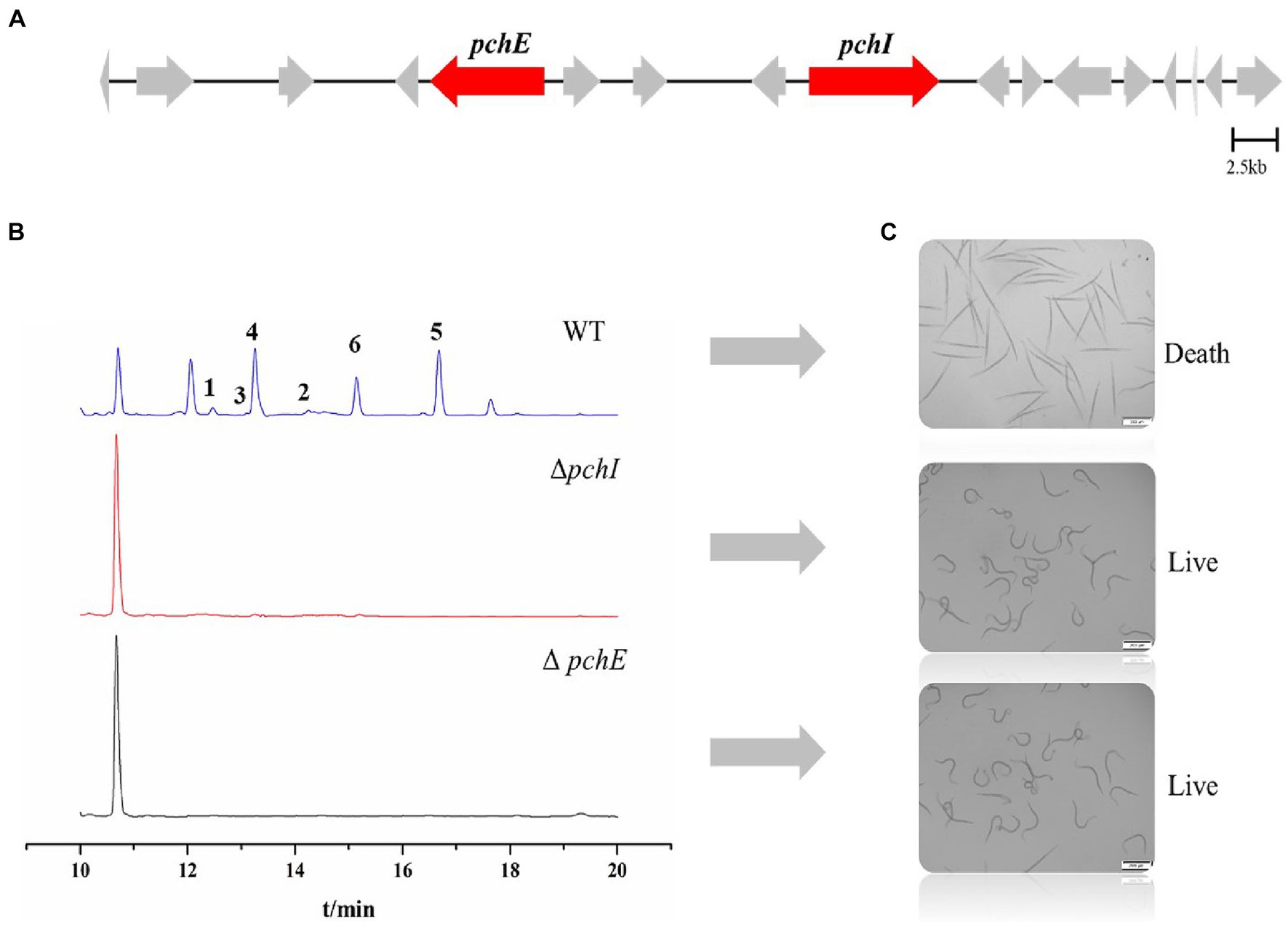
Figure 6. Identification of core genes of P. chlamydosporia strain PC-170 and nematicidal activity of ΔpchI and ΔpchE. (A) The biosynthetic gene cluster for RALs compounds in P. chlamydosporia strain PC-170, two key genes. (B) HPLC analysis of rice fermentation crude extract from the strains wild-type (WT), ΔpchI, and ΔpchE of P. chlamydosporia PC-170. (C) Nematicidal activity of rice fermentation crude extract from the strains wild-type (WT), ΔpchI, and ΔpchE of P. chlamydosporia PC-170.
4 Discussion
The nematicidal activity of six RALs compounds was tested using the same method. It was found that only the glycosylated compounds were nematicidal, while those non-glycosylated compounds were not. We speculate that glycosylation plays a key role in nematicidal activity. The gene cluster for the synthesis of RALs compounds in P. chlamydosporia PC-170 has been identified. A total of 30 RALs compounds have been discovered in P. chlamydosporia (Khambay et al., 2000; Hellwig et al., 2003; Shinonaga et al., 2009a,b; Qin et al., 2019). RALs compounds have a range of biological activities, but less nematicidal activity has been reported. Studies have shown that 10, 11-Dehydrocurvularin exhibits nematicidal activity (Zhang et al., 2017), which may be related to its heat shock response and immune-modulatory activities (Xu et al., 2013). Glycosylation of natural products is an important resource for developing lead compounds in the field of pesticides (Kohara et al., 2007). Glycosylation has the potential to enhance the polarization and water-solubility of compounds, thereby influencing their intracellular and intercellular transportation (Moradi et al., 2016). For example, the anticancer properties of paclitaxel face a significant obstacle in development due to its limited solubility. The monoglycosylated and double-glycosylated derivatives exhibit 49.8-fold and 97.4-fold, respectively, greater aqueous solubility than paclitaxel, and preserve their higher efficacy (Mao et al., 2018). Glycosylation not only changes the physicochemical properties of a compound but also influences its biological activity (Chen, 2011; Hofer, 2016). For another example, megalomycin (glycosylated erythrocin D) exhibits notable antiviral and antiparasitic activities, but erythrocin D itself does not possess similar biological activities (Useglio et al., 2010). These findings are consistent with the results of the current research.
It is crucial to explore the genes related to glycosylation in P. chlamydosporia PC-170. While glycosyltransferase genes in plants and bacteria have been extensively researched, little is known about glycosyltransferase genes in fungi, most of which are glucosyltransferases (Tiwari et al., 2016; Xie et al., 2018). There are no genes encoding glycosyltransferase functions on the RAL synthesis gene cluster of P. chlamydosporia PC-170, but analysis of the P. chlamydosporia 170 genome data revealed 118 glycosyltransferases, and the glycosylation products are formed by catalysis of glycosyltransferases outside the gene cluster (Lin et al., 2018). For example, the glycosylation of phenolic compounds is catalyzed by the glycosyltransferase MhGT1, identified from Mucor hiemalis (Feng et al., 2017). Furthermore, a novel glycosyltransferase (BbGT86) was identified from Beauveria bassiana ARSEF 2860 using bioinformatics and genome mining, and the glycosylation of desmethyl-lasiodiplodin (DLD) was achieved by heterologous expression (Xie et al., 2018). There is still no discovery of D-ribosyltransferases in fungi. Therefore, the subsequent plan is to identify genes with glycosyl transfer function in P. chlamydosporia PC-170 and assess the impact of glycosylation on various RALs products, aiming to discover new natural products with potent nematicidal activity against M. incognita.
5 Conclusion
In conclusion, we isolated and characterized six resorcylic acid lactones (RALs) from the nematicidal biocontrol fungus P. chlamydosporia PC-170, identifying three glycosylated RALs with potent nematicidal activity and three non-glycosylated RALs with antibacterial properties. The novel compound, monocillin VI glycoside (1), was elucidated using high-resolution electrospray ionization mass spectrometry (HRESIMS) and nuclear magnetic resonance (NMR) data, with its monosaccharide configuration confirmed through sugar hydrolysis and gas chromatography–mass spectrometry (GC–MS) analysis. Further, the identification of two biosynthetic polyketide synthase (PKS) genes, pchE and pchI, was achieved via gene knockout experiments. Notably, the glycosylated RALs 1–3 demonstrated significant nematicidal activity against M. incognita, with LC50 values of 94, 152, and 64 μg/mL, respectively, underscoring their potential for developing new nematicidal agents against M. incognita.
Data availability statement
The datasets presented in this study can be found in online repositories. The names of the repository/repositories and accession number(s) can be found at: https://www.ncbi.nlm.nih.gov/, ASM165323v2.
Author contributions
ZL: Investigation, Writing – original draft. NL: Data curation, Investigation, Writing – original draft. WZ: Formal analysis, Investigation, Writing – review & editing. RAAK: Data curation, Writing – review & editing. JL: Conceptualization, Writing – original draft. JZ: Formal analysis, Methodology, Writing – original draft. YY: Writing – original draft. ZM: Conceptualization, Writing – review & editing. BX: Conceptualization, Writing – review & editing. LZ: Conceptualization, Writing – review & editing. YL: Conceptualization, Supervision, Writing – review & editing.
Funding
The author(s) declare that financial support was received for the research, authorship, and/or publication of this article. This study was financially supported by the grants from the National Key R&D Program of China (2022YFD1400700 and 2023YFD1400400) and the Agricultural Science and Technology Innovation Program of CAAS.
Conflict of interest
The authors declare that the research was conducted in the absence of any commercial or financial relationships that could be construed as a potential conflict of interest.
Publisher’s note
All claims expressed in this article are solely those of the authors and do not necessarily represent those of their affiliated organizations, or those of the publisher, the editors and the reviewers. Any product that may be evaluated in this article, or claim that may be made by its manufacturer, is not guaranteed or endorsed by the publisher.
Supplementary material
The Supplementary material for this article can be found online at: https://www.frontiersin.org/articles/10.3389/fmicb.2024.1385255/full#supplementary-material
References
Ayer, W. A., Lee, S. P., Tsuneda, A., and Tiratsuka, Y. (1980). The isolation, identification, and bioassay of the antifungal metabolites produced by Monocillium nordinii. Can. J. Microbiol. 26, 766–773. doi: 10.1139/m80-133
Bo, T. T., Kong, C. X., Zou, S. X., Mo, M. H., and Liu, Y. J. (2022). Bacillus nematocida B16 enhanced the rhizosphere colonization of Pochonia chlamydosporia ZK7 and controlled the efficacy of the root-knot nematode Meloidogyne incognita. Microorganisms 10:218. doi: 10.3390/microorganisms10020218
Bok, J. W., and Keller, N. P. (2004). Lae a, a regulator of secondary metabolism in aspergillus spp. Eukaryot. Cell 3, 527–535. doi: 10.1128/ec.3.2.527-535.2004
Chen, X. (2011). Fermenting next generation glycosylated therapeutics. ACS Chem. Biol. 6, 14–17. doi: 10.1021/cb100375y
Feng, J., Zhang, P., Cui, Y., Li, K., Qiao, X., Zhang, Y. T., et al. (2017). Regio- and stereospecific O-glycosylation of phenolic compounds catalyzed by a fungal glycosyltransferase from Mucor hiemalis. Adv. Synth. Catal. 359, 995–1006. doi: 10.1002/adsc.201601317
Goverse, A., and Smant, G. (2014). The activation and suppression of plant innate immunity by parasitic nematodes. Annu. Rev. Phytopathol. 52, 243–265. doi: 10.1146/annurev-phyto-102313-050118
Hellwig, V. A. M.-B., Müller, H. G. G., Kleymann, G., Zitzmann, W., Tichy, H. V., Stadler, M., et al. (2003). Pochonins a−F, new antiviral and antiparasitic resorcylic acid lactones from Pochonia chlamydosporia var. catenulata. J. Nat. Prod. 66, 829–837. doi: 10.1021/np020556v
Hofer, B. (2016). Recent developments in the enzymatic O-glycosylation of flavonoids. Appl. Microbiol. Biotechnol. 100, 4269–4281. doi: 10.1007/s00253-016-7465-0
Isaka, M., Suyarnsestakorn, C., Tanticharoen, M., Kongsaeree, P., and Thebtaranonth, Y. (2002). Aigialomycins A-E, new resorcylic macrolides from the marine mangrove fungus Aigialus parvus. J. Org. Chem. 67, 1561–1566. doi: 10.1021/jo010930g
Jones, J. T., Haegeman, A., Danchin, E. G. J., Gaur, H. S., Helder, J., Jones, M. G. K., et al. (2013). Top 10 plant-parasitic nematodes in molecular plant pathology. Mol. Plant Pathol. 14, 946–961. doi: 10.1111/mpp.12057
Khambay, B. P. S., Bourne, J. M., Cameron, S., Kerry, B. R., and Zaki, M. J. (2000). A nematicidal metabolite from Verticillium chlamydosporium. Pest Manag. Sci. 56, 1098–1099. doi: 10.1002/1526-4998(200012)56:12<1098::AID-PS244>3.0.CO;2-H
Khan, A., Mfarrej, M. F. B., Tariq, M., Asif, M., Nadeem, H., Siddiqui, M. A., et al. (2021). Supplementing Pochonia chlamydosporia with botanicals for management of Meloidogyne incognita infesting chickpea. Acta. Agr. Scand B-S P. 72, 164–175. doi: 10.1080/09064710.2021.2003853
Kim, J., Baek, J. Y., Bang, S., Kim, J. Y., Jin, Y., Lee, J. W., et al. (2023). New anti-inflammatory β-resorcylic acid lactones derived from an endophytic fungus. Am. Chem. Soc. Omega 8, 3530–3538. doi: 10.1021/acsomega.2c07962
Kohara, A., Nakajima, C., Yoshida, S., and Muranaka, T. (2007). Characterization and engineering of glycosyltransferases responsible for steroid saponin biosynthesis in Solanaceous plants. Phytochemistry 68, 478–486. doi: 10.1016/j.phytochem.2006.11.020
Li, G., Li, R. X., Liu, Q. Y., Wang, Q., Chen, M., and Li, B. J. (2006). A highly efficient polyethylene glycol-mediated transformation method for mushrooms. FEMS Microbiol. Lett. 256, 203–208. doi: 10.1111/j.1574-6968.2006.00110.x
Li, J., Zou, C. G., Xu, J. P., Ji, X. L., Niu, X. M., Yang, J. K., et al. (2015). Molecular mechanisms of nematode-nematophagous microbe interactions: basis for biological control of plant-parasitic nematodes. Annu. Rev. Phytopathol. 53, 67–95. doi: 10.1146/annurev-phyto-080614-120336
Lin, R. M., Qin, F. F., Shen, B. M., Shi, Q. Q., Liu, C. C., Zhang, X., et al. (2018). Genome and secretome analysis of Pochonia chlamydosporia provide new insight into egg-parasitic mechanisms. Sci. Rep. 8:1123. doi: 10.1038/s41598-018-19169-5
Luo, N., Li, Z. Y., Ling, J., Zhao, J. L., Li, Y., Yang, Y. H., et al. (2023). Establishment of a CRISPR/Cas9-mediated efficient knockout system of Trichoderma hamatum T21 and pigment synthesis PKS gene knockout. J. fungi 9:595. doi: 10.3390/jof9050595
Lv, X. J., Li, Y., Ma, S. G., Qu, J., Liu, Y. B., Li, Y. H., et al. (2016). Antiviral triterpenes from the twigs and leaves of Lyonia ovalifolia. J. Nat. Prod. 79, 2824–2837. doi: 10.1021/acs.jnatprod.6b00585
Manzanilla-López, R. H., Esteves, I., Finetti-Sialer, M. M., Hirsch, P. R., Ward, E., Devonshire, J., et al. (2013). Pochonia chlamydosporia: advances and challenges to improve its performance as a biological control agent of sedentary endo-parasitic nematodes. J. Nematol. 45, 1–7.
Mao, Y. K., Zhang, Y. L., Luo, Z., Zhan, R. T., Xu, H., Chen, W. W., et al. (2018). Synthesis, biological evaluation and low-toxic formulation development of glycosylated paclitaxel prodrugs. Molecules 23:3211. doi: 10.3390/molecules23123211
Moradi, S. V., Hussein, W. M., Varamini, P., Simerska, P., and Toth, I. (2016). Glycosylation, an effective synthetic strategy to improve the bioavailability of therapeutic peptides. Chem. Sci. 7, 2492–2500. doi: 10.1039/c5sc04392a
Qin, F. F., Li, Y., Lin, R. M., Zhang, X., Mao, Z. C., Ling, J., et al. (2019). Antibacterial radicicol analogues from Pochonia chlamydosporia and their biosynthetic gene cluster. J. Agric. Food Chem. 67, 7266–7273. doi: 10.1021/acs.jafc.9b01977
Seo, S. M., Kim, J., Koh, S. H., Ahn, Y. J., and Park, I. K. (2014). Nematicidal activity of natural ester compounds and their analogues against pine wood nematode. J. Agric. Food Chem. 62, 9103–9108. doi: 10.1021/jf503631e
Shinonaga, H., Kawamura, Y., Ikeda, A., Aoki, M., Sakai, N., Fujimoto, N., et al. (2009a). Pochonins K–P: new radicicol analogues from Pochonia chlamydosporia var. chlamydosporia and their WNT-5A expression inhibitory activities. Tetrahedron 65, 3446–3453. doi: 10.1016/j.tet.2009.02.027
Shinonaga, H., Kawamura, Y., Ikeda, A., Aoki, M., Sakai, N., Fujimoto, N., et al. (2009b). The search for a hair-growth stimulant: new radicicol analogues as WNT-5A expression inhibitors from Pochonia chlamydosporia var. chlamydosporia. Tetrahedron Lett. 50, 108–110. doi: 10.1016/j.tetlet.2008.10.099
Sugawara, F., Kim, K. W., Kobayashi, K., Uzawa, J., Yoshida, S., Murofushi, N., et al. (1992). Zearalenone derivatives produced by the fungus Drechslera portulacae. Phytochemistry 31, 1987–1990. doi: 10.1016/0031-9422(92)80346-G
Tiwari, P., Sangwan, R. S., and Sangwan, N. S. (2016). Plant secondary metabolism linked glycosyltransferases: an update on expanding knowledge and scopes. Biotechnol. Adv. 34, 714–739. doi: 10.1016/j.biotechadv.2016.03.006
Useglio, M., Peiru, S., Rodriguez, E., Labadie, G. R., Carney, J. R., and Gramajo, H. (2010). TDP-L-megosamine biosynthesis pathway elucidation and megalomicin a production in Escherichia coli. Appl. Environ. Microbiol. 76, 3869–3877. doi: 10.1128/Aem.03083-09
Xie, L. N., Zhang, L. W., Wang, C., Wang, X. J., Xu, Y. M., Yu, H. F., et al. (2018). Methylglucosylation of aromatic amino and phenolic moieties of drug-like biosynthons by combinatorial biosynthesis. Proc. Natl. Acad. Sci. USA 115, E4980–E4989. doi: 10.1073/pnas.1716046115
Xu, Y. Q., Espinosa-Artiles, P., Schubert, V., Xu, Y. M., Zhang, W., Lin, M., et al. (2013). Characterization of the biosynthetic genes for 10,11-Dehydrocurvularin, a heat shock response-modulating anticancer fungal polyketide from aspergillus terreus. ASM J. CD 79, 2038–2047. doi: 10.1128/AEM.03334-12
Xu, L. X., Xue, J. H., Zou, Y., He, S. J., and Wei, X. Y. (2012). Three new β-Resorcylic acid lactones from Paecilomyces sp. SC 0924. Chin. J. Chem. 30, 1273–1277. doi: 10.1002/cjoc.201200406
Yin, N., Zhao, J. L., Liu, R., Li, Y., Ling, J., Yang, Y. H., et al. (2021). Biocontrol efficacy of Bacillus cereus strain Bc-cm103 against Meloidogyne incognita. Plant Dis. 105, 2061–2070. doi: 10.1094/pdis-03-20-0648-re
Zhang, R. R., Zhang, L. W., Zhang, P., Zhang, Y. L., Liu, Z., and Xu, Y. Q. (2017). Studies on the nematocidal activities of benzenediol lactones and their derivatives. Curr. Biotechnol. 7, 632–638+669. doi: 10.19586/j.2095-2341.2017.0026
Keywords: Pochonia chlamydosporia, RALs, nematicidal activity, Meloidogyne incognita, biosynthetic gene
Citation: Li Z, Luo N, Zhang W, Khan RAA, Ling J, Zhao J, Yang Y, Mao Z, Xie B, Zhou L and Li Y (2024) Nematicidal glycosylated resorcylic acid lactones from the fungus Pochonia chlamydosporia PC-170 and their key biosynthetic genes. Front. Microbiol. 15:1385255. doi: 10.3389/fmicb.2024.1385255
Edited by:
Dewu Zhang, Chinese Academy of Medical Sciences, ChinaCopyright © 2024 Li, Luo, Zhang, Khan, Ling, Zhao, Yang, Mao, Xie, Zhou and Li. This is an open-access article distributed under the terms of the Creative Commons Attribution License (CC BY). The use, distribution or reproduction in other forums is permitted, provided the original author(s) and the copyright owner(s) are credited and that the original publication in this journal is cited, in accordance with accepted academic practice. No use, distribution or reproduction is permitted which does not comply with these terms.
*Correspondence: Bingyan Xie, eGllYmluZ3lhbkBjYWFzLmNu; Ligang Zhou, bGd6aG91QGNhdS5lZHUuY24=; Yan Li, bGl5YW4wNUBjYWFzLmNu