- 1Institute of Biomedical Sciences, University of São Paulo, São Paulo, Brazil
- 2Virology Laboratory, Butantan Institute, São Paulo, Brazil
- 3Albert Einstein Institute for Teaching and Research (IIEP), Hospital Israelita Albert Einstein, São Paulo, Brazil
- 4Hospital das Clínicas da Faculdade de Medicina, University of São Paulo, São Paulo, Brazil
- 5Faculty of Animal Science and Food Engineering, University of São Paulo, São Paulo, Brazil
- 6Scientific Platform Pasteur-USP, São Paulo, Brazil
- 7Faculty of Medicine of Ribeirão Preto, University of São Paulo, São Paulo, Brazil
Hemorrhagic fever viruses (HFVs) pose a threat to global public health owing to the emergence and re-emergence of highly fatal diseases. Viral hemorrhagic fevers (VHFs) caused by these viruses are mostly characterized by an acute febrile syndrome with coagulation abnormalities and generalized hemorrhage that may lead to life-threatening organ dysfunction. Currently, the events underlying the viral pathogenicity associated with multiple organ dysfunction syndrome still underexplored. In this minireview, we address the current knowledge of the mechanisms underlying VHFs pathogenesis and discuss the available development of preventive and therapeutic options to treat these infections. Furthermore, we discuss the potential of HFVs to cause worldwide emergencies along with factors that favor their spread beyond their original niches.
Introduction
Hemorrhagic fever viruses (HFVs) are highly infectious RNA viruses that can lead to viral hemorrhagic fever (VHF) in humans. VHFs are mostly characterized by mild to acute febrile syndrome with coagulation abnormalities and generalized hemorrhage that can lead to multiorgan failure, and death (Schnittler and Feldmann, 2003; Basler, 2017). The frequency of hemorrhagic manifestations driven by HFVs can vary; however, they represent the acute form of the disease and one of the most common signs of the infection (Peters and Zaki, 2002; Paessler and Walker, 2013).
These viruses are a major concern to global public health because of their potential as bioweapons (Abir et al., 2022; Hickman et al., 2022) and the possibility to cause outbreaks with high fatality rates. In this minireview, we present the current knowledge regarding HFVs, including their common features and recently described pathogenic mechanisms underlying virulence leading to life-threatening infections. In addition, we present therapeutics and vaccines that are being used or under development to treat these diseases (Table 1). Finally, we highlight the potential of HFVs to transpose national boundaries and emerge as global threats.
Clinical presentation and pathogenesis
VHFs are characterized by abnormal vascular regulation and damage (Schnittler and Feldmann, 2003). Despite sharing some clinical manifestations, the cell and organ tropism, as well as the molecular mechanisms underlying their pathogenesis vary according to the causative agent (Peters and Zaki, 2002; Paessler and Walker, 2013; Table 1). However, all of them, target the cells responsible for initiating the antiviral response, causing a delay in the immune response. This delay, leads VHF patients to present high viremias and immunosuppression that can lead to a fulminant shock-like syndrome where inflammatory mediators play a major role (Marty et al., 2006; Reynard et al., 2014; Russier et al., 2014; Woolsey et al., 2022; Figure 1).
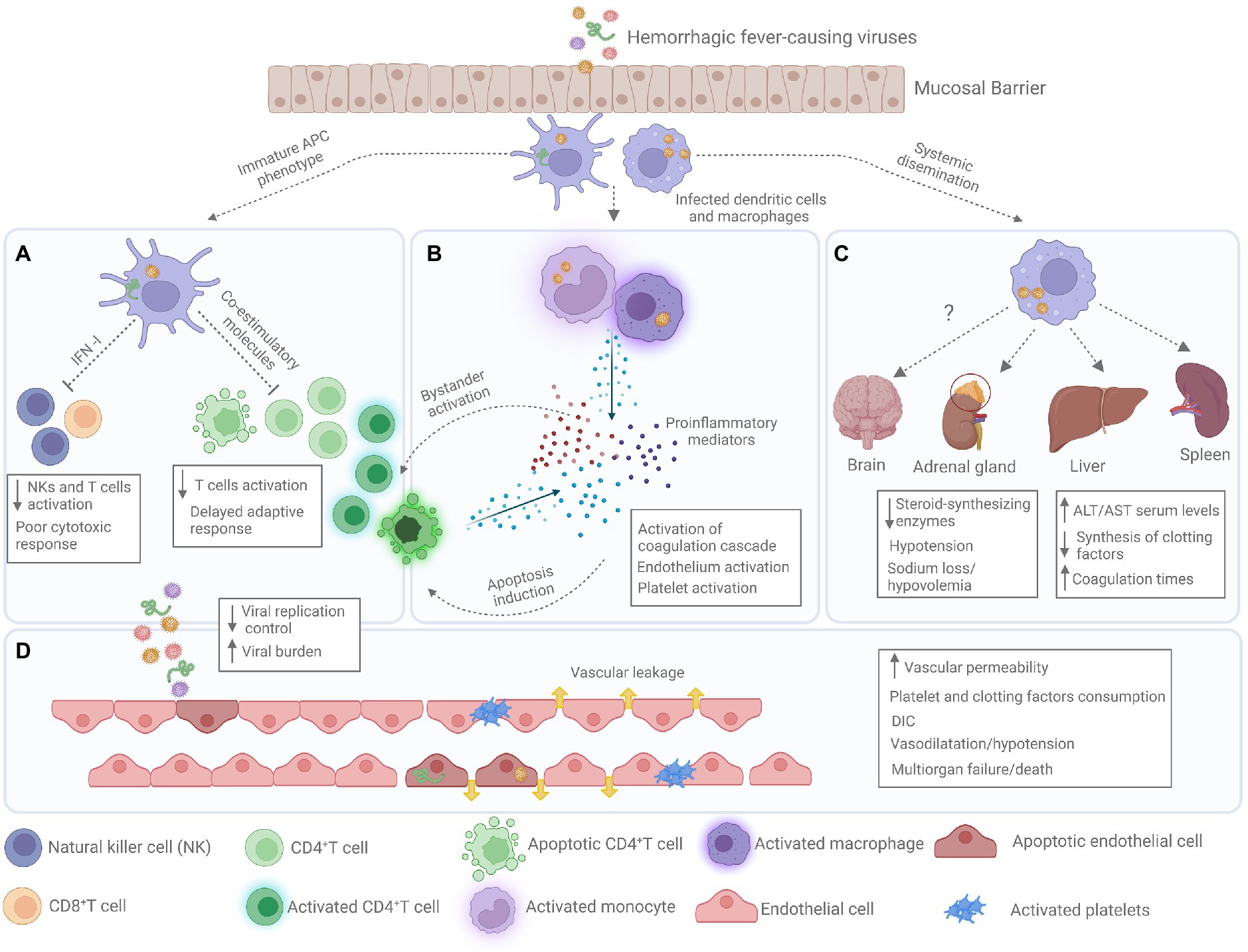
Figure 1. Pathogenesis of severe viral hemorrhagic fever. (A) Antigen presenting cells (APCs) present an immature phenotype with low expression of co-stimulatory molecules and low type I interferon (IFN-I) production, leading to poor NK and T cells activation causing a generalized immunosuppression, which lead to uncontrolled viral replication and high viral burdens. (B) During the infection monocytes and macrophages produced large quantities of proinflammatory mediators causing the phenomenon known as cytokine storm. These proinflammatory mediators cause endothelial and platelet activation and induce bystander activation and apoptosis of T cells. (C) Infected APC transport Hemorrhagic fever viruses (HFVs) to lymph nodes and other organs causing a systemic dissemination. Infection of some organs such as liver and adrenal gland results in high serum levels of hepatic enzymes (aspartate amino transferase-AST, and alanine amino transferase-ALT), low production of clotting factors and hypovolemia. (D) Sustained proinflammatory state and viral infection of endothelial cells induce abnormal vascular regulation and organ effusion, hemorrhages, and disseminated intravascular coagulation (DIC).
HFVs are transmitted through contact with or inhalation of contaminated materials from animal reservoirs or arthropod vectors; however, human-to-human spread through contact with infected blood and other body fluids is possible for most HFVs (Marty et al., 2006; Koehler et al., 2022). The incubation period varies from 2 to 35 days and begins with a prodromal period that typically last less than 1 week. This phase is followed by an increase in viral replication, which leads to an excessive release of cytokines, causing endothelial activation, increased vascular permeability, vasodilatation with subsequent hypotension, multiorgan failure, and death (Marty et al., 2006; Koehler et al., 2022).
Macrophages and dendritic cells (DCs) are the primary targets of most HFVs (Schmid et al., 2014; Huang et al., 2015; Schönrich and Raftery, 2019). Usually, despite being infected, these cells do not show an increase in activation markers or cytokine production. This immature profile of antigen-presenting cells (APCs) leads to deficient natural killer (NK) and T cell activation, impairing the induction of the subsequent immune response (Baize et al., 2004; Jin et al., 2010). Besides they can act as a viral reservoir and transport the virus into draining lymph nodes and other organs during immune patrol (Baize et al., 2004; Jin et al., 2010), explaining the high viral loads and systemic infection observed during VHFs (Reynard et al., 2014; Russier et al., 2014).
Several authors have reported that HFVs counteract the innate and adaptive immune responses in several ways. For example, Ebola virus inhibits DC maturation by the polyfunctional viral protein of 35 kDa (VP35; Jin et al., 2010), abrogating the adequate induction of adaptive responses. Other important players in the immune response, such as natural killer (NK) cells and gamma delta (γδ) T cells, decrease in number early during infection with pathogenic and non-pathogenic arenaviruses (Rodas et al., 2009), however, the underlying mechanisms are still unknown. Pathogenic arenaviruses are also able to suppress the type I interferon (IFN-I) response, avoiding interferon regulatory factor 3 (IRF3) activation and translocation to the nucleus through nucleoprotein (NP), they also inhibit RNA sensors activity by Z protein interactions (Pythoud et al., 2012; Rodrigo et al., 2012; West et al., 2014; Kang et al., 2021; Rojas et al., 2021). Inhibition of IFN-related signalling has been reported for other viruses as well; yellow fever virus (YFV) and dengue virus (DENV) inhibit the IFN response through the signal transducer and activator of transcription 2 (STAT2) and non-structural protein 5 (NS5) interaction (Fernandez-Garcia et al., 2016). This JAK/STAT pathway is also abroad by hantaviruses, which are also capable of inhibiting RIG-I-dependent IFN production, thus impairing the induction of adequate antiviral response (Levine et al., 2010; Gallo et al., 2021).
Not only the magnitude of IFN-I but also the T cell response have been associated with the disease outcome, where non-survivor patients may not generate an efficient immune response compared with the survivors, in whom the response is early and robust. Regarding T cells, both CD4+ and CD8+ T cells are important for protection against HFV. During infection, these cells show a predominant HLA-DR+ CD38+ phenotype, which is characteristic of an effector profile and differentiate towards a type I effector response characterized by IFN-γ production and cytotoxicity. Besides these effector mechanisms, CD4+ T cells are also involved in B-cell activation for antibody production (Farooq et al., 2016; Meyer and Ly, 2016; Ruibal et al., 2016; Speranza et al., 2018). However, during the infection the exacerbated response of these effector cells contributes to endothelial and hepatic damage, two hallmarks of the disease (Sung et al., 2012; Beier et al., 2015; Perdomo-Celis et al., 2019). On the other hand, regulatory CD4+ T cells, despite a conserved phenotype, showed a reduced number in peripheral blood, contributing to loss of activation control and leading to chronic inflammation (Zhu et al., 2009). This scenario leads to T-cell apoptosis, explaining the pronounced lymphopenia that characterizes most VHFs with the exception of those caused by hantaviruses, were lymphocytosis is normally observed (Marty et al., 2006; Rodrigues et al., 2012; Brisse and Ly, 2019). The lymphocyte apoptosis mechanism is not completely understood, but the involvement of TNF-related apoptosis ligand (TRAIL) and Fas/FasL (Fas Ligand) pathways have been proposed (Hensley, 2002). Aberrant responses with high expression of inhibitory receptors such as CTLA-4 and PD-1 have also been reported (Wauquier et al., 2010).
An increase in soluble proinflammatory mediators is another characteristic of VHFs. This proinflammatory state has been called “cytokine storm” and is characterized by the presence of high levels of IFNs, interleukin (IL)-6, IL-8, IL-10, IL-12, tumor necrosis factor-alfa (TNF-α), and reactive oxygen (ROS) and nitrogen (NO) species in serum (Messaoudi and Basler, 2015). As this phenomenon occurs despite the capacity of HFVs to suppress the immune response. It is unclear whether infected cells are the primary source of cytokines or are triggered via a bystander effect (Basler, 2017; Figure 1). However, some evidence suggests that infected monocytes and macrophages can be involved in the abnormal proinflammatory cytokine, chemokine, and ROS production in DENV and Ebola virus infections (Olejnik et al., 2017; Naranjo-Gómez et al., 2019). Direct interaction between the virus and T cells causing non-antigen specific activation and cytokine production has been reported for Ebola virus as well (Younan et al., 2017). Although these proinflammatory mediators are produced to control infection, at high and maintained levels, they exert cytotoxic effects by contributing to the death of bystander lymphocytes (Whiteside et al., 2018), tissue damage, loss of vascular integrity (Kang and Kishimoto, 2021), and hypotension mediated by NO (Li and Förstermann, 2000). Together, the information gathered to date suggests that an impaired and ineffective immune response leads to high viral loads and production of high levels of proinflammatory mediators, which are the main players in hemorrhages followed by shock with multiorgan failure that characterizes VHF clinical presentation.
Hepatic damage with high serum levels of alanine aminotransferase (ALT) and aspartate aminotransferase (AST), renal impairment evidenced by oliguria, coagulopathy, thrombocytopenia with prolonged coagulation times, and disseminated intravascular coagulation (DIC) also occur depending on the severity of VHFs (Figure 1; Mariappan et al., 2021). Some of these clinical signs can be explained as effects of “cytokine storm,” whereas others are related to direct viral infections facilitated by extravasation of infected monocytes/macrophages to tissues (Schnittler and Feldmann, 2003). During YFV infection, apoptosis and necrosis occur in up to 70% of hepatocytes and Kupffer cells, and viral nucleic acids and cellular infiltrates are observed in these tissues (Quaresma et al., 2007). Necrotic lesions have been found in the spleen, liver, bone marrow, heart, and kidneys during filovirus and arenavirus infection. Despite brain infection being observation only in animal models, survivors of Junín virus infection often develop neurological sequelae, suggesting an infection of the central nervous system (CNS; Paessler and Walker, 2013). Impaired endothelial function causes a wide spectrum of vascular effects observed during VHF, although the molecular mechanisms are largely unknown. According to experimental data, changes in the protein organization of tight junctions, particularly vascular endothelial cadherin (VE-cadherin)/catenin, are implicated (Mariko et al., 2019). Increased liberation of bradykinin, a potent inducer of vascular permeability, has also been reported in hantaviruses infections (Taylor et al., 2013). In addition, the virion glycoprotein (GP) of the Ebola virus has been shown to mediate abnormal activation and disruption of endothelial cells even in the absence of viral replication (Geisbert et al., 2003; Yang and Yang, 2021; Moni et al., 2022). Humans and nonhuman primates with VHF also present adrenocortical infection and necrosis, leading to impaired secretion of steroid-synthesizing enzymes that cause hypotension and sodium loss due to hypovolemia; these two important events have been reported in nearly all cases of VHF (Mariappan et al., 2021).
Diagnosis and treatment
VHFs present with nonspecific symptoms making it difficult to clinically discriminate from other diseases, such as malaria, typhoid fever and even between the different causative agents of VHFs. This, together with the fact that VHFs also share laboratory parameters and the poor diagnostic value of serology given the impairment of APCs and lymphocyte functions during the acute phase of the disease (Feldmann and Geisbert, 2011); causes a delay in the diagnosis even after the fulminant disease process ensues (Racsa et al., 2016). Considering that, the viral genome detection is the better tool for diagnosis, however, sampling of blood requires medically trained personal and comprises important risks for the patient as well as for the health care personal (Racsa et al., 2016). Because of this situation traditional diagnosis has been restricted to large reference laboratories centered in Europe and the United States limiting the availability of the diagnosis in endemic areas (Racsa et al., 2016). Therefore, the sampling by non-invasive methods (e.g., saliva and/ or urine) might be a very valuable alternative. Currently, some laboratory diagnostic improvements are under development, including multiplex PCR, lateral flow assays and non-invasive sampling, including saliva and urine (Das et al., 2015; Niedrig et al., 2018; Barnes et al., 2020; Humaidi et al., 2021; Valinetz and Cangelosi, 2021).
For VHF treatment, two main components should be considered: (i) specific antiviral treatment and (ii) life support to prevent multiorgan failure (Ippolito et al., 2012). The appropriate treatment needs to be administered according to the phase of VHF: incubation, precoagulopathy, and coagulopathy (Ergonul et al., 2007). During incubation, post-exposure active/passive immunization and the administration of molecules with antiviral activity are the most effective approaches (Ippolito et al., 2012). Regarding active immunization, there are some approved vaccines against HFVs (Table 1). The YFV 17D, a live attenuated vaccine, has been administered around the world since 1932 with few side effects and providing life-long protection (de Menezes et al., 2015). The only vaccine successfully administered for an arenavirus is Candid #1, manufactured by the Argentinian government to prevent disease by the Junín virus (McLay et al., 2014). This vaccinal strain carries a mutation that attenuates the Junín virus as well as the Machupo virus infections, suggesting that it can provide cross-protection against arenaviruses (Patterson et al., 2014). There are no currently available Food and Drug Administration (FDA)-approved prophylaxis or vaccines for Crimean-Congo hemorrhagic fever (CCHF). However, an inactivated vaccine, developed in Bulgaria that induces strong humoral and T-cell responses has been used since 70s in the country (Mousavi-Jazi et al., 2012). There are other vaccines like Hantavax, that has been tested in patients with hemorrhagic fever and renal syndrome and has shown high seroconversion rates and a decrease in patient hospitalization (Cho et al., 2002; Song et al., 2016; Jung et al., 2018) and tetravalent DENV vaccine that has been shown to protect against severe forms of the disease for at least 5 years (Villar et al., 2015; Sridhar et al., 2018). Reassortant of Mopeia virus (MOPV) and Lassa fever virus (LASV) vaccine candidate, has been shown to protect against lethal LASV challenge in rodents. Viral particles produced by ML29-infected cells also interfere with the lymphocytic choriomeningitis virus (LCMV) carrier status, suggesting the potential of this formulation as a pan-arenaviral vaccine (Carnec et al., 2018; Johnson et al., 2019). Vesicular stomatitis virus (VSV) and adenoviral vectors have been evaluated in phase 1 studies and have been proposed as post-exposure vaccinations after accidental exposure of healthcare workers and laboratory workers to filoviruses (Tuffs, 2009; Ippolito et al., 2012; Ledgerwood et al., 2017; Regules et al., 2017). Recently, ERVEBO was licensed by the European Medicines Agency (EMA) and prequalified by the WHO as protective vaccination against Zaire ebolavirus. Additionally, in 2020, the EMA recommended granting marketing authorization to a second new vaccine called Zabdeno (Ad26.ZEBOV) and Mvabea (MVA-BN-Filo) delivered in two doses for individuals a year old and upwards (WHO, 2022a; Table 1).
Convalescent plasma therapy has been used as passive immunization and has proven to be effective in improving the clinical course and reducing mortality during hantaviral infection (Enria et al., 2008; Vial et al., 2015; Winkler and Koepsell, 2015). Additionally, Inmazeb, a mixture of three monoclonal antibodies against Zaire Ebola proteins received an orphan drug designation for the treatment of Ebola virus infection by the FDA (FDA, 2022). Finally, regarding molecules with antiviral activity. Nucleoside analogs have been tested against HFVs with promising results. Ribavirin has been effective against arenavirus and bunyavirus infection, reducing the mortality by 44% (Bossi et al., 2004; Soares-Weiser et al., 2010; Table 1), but other studies have shown insufficient efficacy of these compounds against hemorrhagic fever with renal syndrome (Malinin and Platonov, 2017). Favipiravir, one of the widely tested compounds in this group, has shown activity against several HFVs in animal models (Furuta et al., 2009; Safronetz et al., 2013; Madelain et al., 2017). These compounds can also be administered during the second phase of VHF, the precoagulophaty, when viral replication and high viremia occurs.
The third and last disease phase, the coagulophaty, is characterized by the onset of coagulation abnormalities driven mainly by the cytokine storm; immune-modulating drugs are the most effective pharmacological options during this phase. Factors that modulate coagulation, including recombinant inhibitors of factor VIIa and activated protein C, are associated with increased survival rates (Geisbert et al., 2003; Hensley et al., 2007). Inhibitors of platelet activation and macrophage migration have also been evaluated with promising results (Souza et al., 2009; Assunção-Miranda et al., 2010). Life support for patients with VHF and aggressive therapy to address multiorgan failure have proven to be lifesaving (Feldmann and Geisbert, 2011). Combined treatment with steroids, vasoactive drugs, hemodialysis, and mechanical ventilation resulted in favorable outcomes in patients infected with the Puumala virus battling multiorgan failure (Seitsonen et al., 2006). It has been reported that extracorporeal membrane oxygenation can also improve survival in patients with cardiopulmonary syndrome due to hantavirus infection (Dietl et al., 2008; Llah et al., 2018).
Emergence and re-emergence potential
The ongoing recent trend of emerging and re-emerging diseases occurs almost every year. Influencing factors such as increased global population, aging, travel, and climate change, among other factors, favor the evolution and spread of new pathogens and the re-emergence of the older ones (Bloom et al., 2017). The distribution of HFVs, is closely linked to the ecology of their vectors and reservoirs such as rodents or arthropods. The changes in the distribution of these animal populations, recently well documented due to agricultural intensification and deforestation, represent a latent risk of re-emergence due to changes in the sites of circulation and spread of these viruses (Marty et al., 2006; Jones et al., 2013; Ellwanger et al., 2020).
For DENV and YFV, changes in the ecology of already established vectors and the appearance of other species with a better vectorial capacity can mark a greater expansion of these diseases and the occurrence of outbreaks in areas where they were already controlled (Brady and Hay, 2020; Pereira-dos-Santos et al., 2020). This was observed in Brazil, where major YFV outbreaks were observed in cities that were free of disease for almost 70 years due to viral adaptation to Ae. Albopictus (Amraoui et al., 2018). This vector has been identified as a potential player in emerging and re-emerging events because of its capacity to colonize natural breeding sites and ability to transmit more than a dozen arboviruses (Pereira-dos-Santos et al., 2020). The expansion of tick species into new geographic areas also represents a threat to the introduction of infections (Wikel, 2018). CCHF, the most widespread tick-borne viral disease affecting humans, has been restricted for many years to some regions of Africa, Asia, and eastern and southern Europe; however, its distribution is increasing rapidly in the eastern Mediterranean region due to the transport of tick vectors into these regions by birds (Al-Abri et al., 2017). The same phenomenon has been observed for other viruses, such as Alkhurma, a zoonosis associated with livestock in Saudi Arabia; it is now thought to be more widely disseminated than the initial focus in the Karnataka state in southwestern India because of abnormal vector migration (Mansfield et al., 2017).
In the case of rodents, their behavioral and demographic characteristics may contribute to their capacity to harbor, maintain, and spread the virus (Wikel, 2018). Rodents generally exhibit an r-selected life history, characterized by early sexual maturity and large litter sizes. This makes them vulnerable to resource depletion and climatic variation, impacting the prevalence of some RNA viruses in the human population (Easterbrook and Klein, 2008; Andreassen et al., 2021). The warming of the European climate has been predicted to increase the risk of hantavirus infection in the region and in the United States, where El Niño-related weather events have been linked to an increase in hantavirus pulmonary syndrome (HPS) incidence (Hjelle and Glass, 2000; Tersago et al., 2009). These data show how various external drivers create suitable conditions that allow zoonotic pathogens to expand and adapt to new niches. Often, these drivers are not just ecological but also economic, social, and political, functioning in various geographical and administrative territories (Wormser et al., 2010). Hence, the latent risk of re-emergence, the particular characteristics of this group of viruses, including their ability to cause disease with high mortality rates, the possibility of person-to-person transmission, and the easy dissemination through the air, have caused these viruses to be classified as bioweapon agents category A by the Center for disease Control and Prevention (CDC; CDC, 2022a).
This scenario, together with recent outbreaks affecting the lives and health of millions of people, highlights the need for improvement in global outbreak surveillance. A survey on laboratory preparedness for the response and diagnosis of CCHF conducted among the European Network for Diagnostics of ‘Imported’ Viral Diseases (ENIVD) members, revealed that despite more than 70% of laboratories having conditions to handle infectious samples, more than 60% emphasized the need for further training for laboratory workers, medical staff, and nursing staff (Fernandez-García et al., 2014). Detection of viral RNA combined with serology is performed routinely to ensure the early diagnosis of these diseases and is essential for effective surveillance, management of individual patients, and outbreak prevention. Nevertheless, progress in viral isolation, an essential part of virology studies is limited and consequently several aspects of the biology, ecology, and pathogenesis of HFVs remain unknown. In addition, treatment and vaccine development are rarely applied because high biocontainment laboratory (Biosafety level 4 - BSL4) facilities are deficient in endemic areas (Fernandez-García et al., 2014).
Conclusion and future directions
Most HFVs cause high fatality rate due to their elevated pathogenicity, moreover, there are not many preventive vaccines and therapeutic options to treat VHF patients. This, together with the high risk of emergence and re-emergence of these pathogens, highlights the need of implementing several actions to suppress the latent risks that VHFs represent. These actions include the establishment of rapid and reliable protocols for laboratory diagnosis; guidelines for storage, processing, and transportation of samples; besides, conducting a comprehensive review of the BSL4 facilities suited to this work in the endemic areas, in addition to their capacities and capabilities, that will allow a safe management of these viruses (Fernandez-García et al., 2014). Is also necessary to study the spatial and temporal distribution of infection in reservoir populations, to predict interactions that favor spillovers (Plowright et al., 2019). These actions will allow to be prepared for future outbreaks reducing the impact of these diseases in human populations.
Author contributions
ES, LF-A, CW, and ED conceived the manuscript. LF-A and ES wrote the manuscript. PH, JP, MC, VB, EA, HF, CT, GP, DO, LS, and PM contributed to manuscript revision and text editing. All authors contributed to the article and approved the submitted version.
Funding
The authors would like to thank the Fundação de Amparo à Pesquisa do Estado de São Paulo (FAPESP) for financial support within the projects 2022/01812–8 (LF-A), 2015/26722–8, 2017/03966–4 (CW), 2020/12277–0 (ES), 2018/18257–1, 2018/15549–1, 2020/04923–0 (GP), 2020/06409–1 (ED), 2019/12303–4 (VB), 2017/27131–9 (PM), 2016/20045–7 (LS), 2016/08730–6 (CT); the Conselho Nacional de Ciência e Tecnologia (CNPq) for support within the projects 305,430/2019–0 (PH); 404,176/2019–4 (NIH project number 1 R01 AI149608-01; MC); 307,854/2018–3 (GP); 301,524/2019–0 (CW) and the Fundação Butantan for support (PH and VB).
Acknowledgments
Figures were created with BioRender.com, (License #2364–1,511, Toronto, ON, Canada).
Conflict of interest
The authors declare that the research was conducted in the absence of any commercial or financial relationships that could be construed as a potential conflict of interest.
Publisher’s note
All claims expressed in this article are solely those of the authors and do not necessarily represent those of their affiliated organizations, or those of the publisher, the editors and the reviewers. Any product that may be evaluated in this article, or claim that may be made by its manufacturer, is not guaranteed or endorsed by the publisher.
Abbreviations
NP, Nucleoprotein; Z protein, Small RING finger protein; L protein, Large multifunctional protein; Gn, Viral envelope glycoprotein; N, Nucleocapsid; GPC, Glycoprotein precursor; NS, Non-structural protein; VP35, Viral protein of 35 kDa; VP24, Viral protein of 24 kDa; VP40, Viral protein of 40 kDa; E, Envelope protein.
References
Abir, M. H., Rahman, T., Das, A., Etu, S. N., Nafiz, I. H., Rakib, A., et al. (2022). Pathogenicity and virulence of Marburg virus. Virulence 13, 609–633. doi: 10.1080/21505594.2022.2054760
Aguirre, S., Maestre, A. M., Pagni, S., Patel, J. R., Savage, T., Gutman, D., et al. (2012). DENV inhibits type I IFN production in infected cells by cleaving human STING. PLoS Pathog. 8:e1002934. doi: 10.1371/journal.ppat.1002934
Al-Abri, S. S., Al, A. I., Fazlalipour, M., Mostafavi, E., Leblebicioglu, H., Pshenichnaya, N., et al. (2017). Current status of Crimean-Congo haemorrhagic fever in the World Health Organization eastern Mediterranean region: issues, challenges, and future directions. Int. J. Infect. Dis. 58, 82–89. doi: 10.1016/j.ijid.2017.02.018
Al-Tawfiq, J. A., and Memish, Z. A. (2017). Alkhurma hemorrhagic fever virus. Microbes Infect. 19, 305–310. doi: 10.1016/j.micinf.2017.04.004
Amraoui, F., Pain, A., Piorkowski, G., Vazeille, M., Couto-Lima, D., de Lamballerie, X., et al. (2018). Experimental adaptation of the yellow fever Virus to the mosquito Aedes albopictus and potential risk of urban epidemics in Brazil, South America. Sci. Rep. 8:14337. doi: 10.1038/s41598-018-32198-4
Andreassen, H. P., Sundell, J., Ecke, F., Halle, S., Haapakoski, M., Henttonen, H., et al. (2021). Population cycles and outbreaks of small rodents: ten essential questions we still need to solve. Oecologia 195, 601–622. doi: 10.1007/s00442-020-04810-w
Assunção-Miranda, I., Amaral, F. A., Bozza, F. A., Fagundes, C. T., Sousa, L. P., Souza, D. G., et al. (2010). Contribution of macrophage migration inhibitory factor to the pathogenesis of dengue virus infection. FASEB J. 24, 218–228. doi: 10.1096/fj.09-139469
Baize, S., Kaplon, J., Faure, C., Pannetier, D., Georges-Courbot, M.-C., and Deubel, V. (2004). Lassa Virus infection of human dendritic cells and macrophages is productive but fails to activate cells. J. Immunol. 172, 2861–2869. doi: 10.4049/jimmunol.172.5.2861
Barnes, K. G., Lachenauer, A. E., Nitido, A., Siddiqui, S., Gross, R., Beitzel, B., et al. (2020). Deployable CRISPR-Cas13a diagnostic tools to detect and report Ebola and Lassa virus cases in real-time. Nat. Commun. 11:4131. doi: 10.1038/s41467-020-17994-9
Basler, C. F. (2017). Molecular pathogenesis of viral hemorrhagic fever. Semin. Immunopathol. 39, 551–561. doi: 10.1007/s00281-017-0637-x
Basler, C. F., Mikulasova, A., Martinez-Sobrido, L., Paragas, J., Mühlberger, E., Bray, M., et al. (2003). The Ebola Virus VP35 protein inhibits activation of interferon regulatory factor 3. J. Virol. 77, 7945–7956. doi: 10.1128/JVI.77.14.7945-7956.2003
Beier, J. I., Jokinen, J. D., Holz, G. E., Whang, P. S., Martin, A. M., Warner, N. L., et al. (2015). Novel mechanism of Arenavirus-induced liver pathology. PLoS One 10:e0122839. doi: 10.1371/journal.pone.0122839
Bloom, D. E., Black, S., and Rappuoli, R. (2017). Emerging infectious diseases: A proactive approach. Proc. Natl. Acad. Sci. 114, 4055–4059. doi: 10.1073/pnas.1701410114
Bossi, P., Tegnell, A., Baka, A., van Loock, F., Werner, A., Hendriks, J., et al. (2004). Bichat guidelines for the clinical management of viral encephalitis and bioterrorism-related viral encephalitis. Eur. Secur. 9, 39–40. doi: 10.2807/esm.09.12.00509-en
Brady, O. J., and Hay, S. I. (2020). The global expansion of dengue: how Aedes aegypti mosquitoes enabled the first pandemic Arbovirus. Annu. Rev. Entomol. 65, 191–208. doi: 10.1146/annurev-ento-011019-024918
Brauburger, K., Hume, A. J., Mühlberger, E., and Olejnik, J. (2012). Forty-five years of Marburg Virus research. Viruses 4, 1878–1927. doi: 10.3390/v4101878
Brisse, M. E., and Ly, H. (2019). Hemorrhagic fever-causing arenaviruses: lethal pathogens and potent immune suppressors. Front. Immunol. 10:372. doi: 10.3389/fimmu.2019.00372
Carnec, X., Mateo, M., Page, A., Reynard, S., Hortion, J., Picard, C., et al. (2018). A vaccine platform against Arenaviruses based on a recombinant Hyperattenuated Mopeia Virus expressing heterologous glycoproteins. J. Virol. 92:e02230-17. doi: 10.1128/JVI.02230-17
CDC (2022a). Bioterrorism Agents/Diseases 2022. Available at: https://emergency.cdc.gov/agent/agentlist-category.asp [Accessed July 15, 2022].
CDC (2022b). Signs and Symptoms | Lymphocytic Choriomeningitis (LCM) | CDC. Available at: https://www.cdc.gov/vhf/lcm/symptoms/index.html [Accessed July 15, 2022].
CDC (2022c). Yellow Fever. Available at: https://www.cdc.gov/yellowfever/index.html [Accessed July 15, 2022].
CDC (2022d). Areas with Risk of Dengue | Dengue | CDC. Available at: https://www.cdc.gov/dengue/areaswithrisk/index.html [Accessed July 15, 2022].
CDC (2022e). Biosafety in Microbiological and Biomedical Laboratories (BMBL) 6th Edition | CDC Laboratory Portal | CDC. Available at: https://www.cdc.gov/labs/BMBL.html [Accessed August 2, 2022].
Centers for Disease Control and Prevention C (2022). Hemorrhagic Fever with Renal Syndrome (HFRS) 2022. Available at: https://www.cdc.gov/vhf/lcm/symptoms/index.html.
Cho, H.-W., Howard, C. R., and Lee, H.-W. (2002). Review of an inactivated vaccine against hantaviruses. Intervirology 45, 328–333. doi: 10.1159/000067925
Dalrymple, N. A., Cimica, V., and Mackow, E. R. (2015). Dengue Virus, NS Proteins inhibit RIG-I/MAVS signaling by blocking TBK1/IRF3 phosphorylation: dengue Virus serotype 1 NS4A is a unique interferon-regulating virulence determinant. MBio 6:e00553-15. doi: 10.1128/mBio.00553-15
Das, S., Rundell, M. S., Mirza, A. H., Pingle, M. R., Shigyo, K., Garrison, A. R., et al. (2015). A multiplex PCR/LDR assay for the simultaneous identification of category A infectious pathogens: agents of viral hemorrhagic fever and Variola Virus. PLoS One 10:e0138484. doi: 10.1371/journal.pone.0138484
de Menezes, M. R., da Luz Fernandes Leal, M., and Homma, A. (2015). Serious adverse events associated with yellow fever vaccine. Hum. Vaccin. Immunother. 11, 2183–2187. doi: 10.1080/21645515.2015.1022700
Dietl, C. A., Wernly, J. A., Pett, S. B., Yassin, S. F., Sterling, J. P., Dragan, R., et al. (2008). Extracorporeal membrane oxygenation support improves survival of patients with severe hantavirus cardiopulmonary syndrome. J. Thorac. Cardiovasc. Surg. 135, 579–584. doi: 10.1016/j.jtcvs.2007.11.020
Easterbrook, J. D., and Klein, S. L. (2008). Immunological mechanisms mediating hantavirus persistence in rodent reservoirs. PLoS Pathog. 4:e1000172. doi: 10.1371/journal.ppat.1000172
Ellwanger, J. H., Kulmann-Leal, B., Kaminski, V. L., Valverde-Villegas, J. M., Veiga Abg, D. A., Spilki, F. R., et al. (2020). Beyond diversity loss and climate change: impacts of Amazon deforestation on infectious diseases and public health. An. Acad. Bras. Cienc. 92:e20191375. doi: 10.1590/0001-3765202020191375
EMA (2022). EU Clinical Trials Register. Available at: https://www.clinicaltrialsregister.eu/ctr-search/search (Accessed September 7, 2022).
Enria, D. A., Briggiler, A. M., and Sánchez, Z. (2008). Treatment of argentine hemorrhagic fever. Antivir. Res. 78, 132–139. doi: 10.1016/j.antiviral.2007.10.010
Ergonul, O., Mirazimi, A., and Dimitrov, D. S. (2007). Treatment of Crimean-Congo hemorrhagic fever. Crimean-Congo Hemorrhagic Fever, Dordrecht: Springer Netherlands, 245–269.
Fan, L., Briese, T., and Lipkin, W. I. (2010). Z proteins of New World Arenaviruses bind RIG-I and interfere with type I interferon induction. J. Virol. 84, 1785–1791. doi: 10.1128/JVI.01362-09
Farooq, F., Beck, K., Paolino, K. M., Phillips, R., Waters, N. C., Regules, J. A., et al. (2016). Circulating follicular T helper cells and cytokine profile in humans following vaccination with the rVSV-ZEBOV Ebola vaccine. Sci. Rep. 6:27944. doi: 10.1038/srep27944
FDA (2022). FDA Approves First Treatment for Ebola Virus | FDA. Available at: https://www.fda.gov/news-events/press-announcements/fda-approves-first-treatment-ebola-virus [Accessed August 2, 2022].
Feldmann, H., and Geisbert, T. W. (2011). Ebola haemorrhagic fever. Lancet 377, 849–862. doi: 10.1016/S0140-6736(10)60667-8
Fernandez-Garcia, M. D., Meertens, L., Chazal, M., Hafirassou, M. L., Dejarnac, O., Zamborlini, A., et al. (2016). Vaccine and wild-type strains of yellow fever Virus engage distinct entry mechanisms and differentially stimulate antiviral immune responses. MBio 7, e01956–e01915. doi: 10.1128/mBio.01956-15
Fernandez-García, M. D., Negredo, A., Papa, A., Donoso-Mantke, O., Niedrig, M., Zeller, H., et al. (2014). European survey on laboratory preparedness, response and diagnostic capacity for Crimean-Congo haemorrhagic fever, 2012. Eur. Secur. 19:pii=20844. doi: 10.2807/1560-7917.ES2014.19.26.20844
Fillâtre, P., Revest, M., and Tattevin, P. (2019). Crimean-Congo hemorrhagic fever: an update. Méd. Mal. Infect. 49, 574–585. doi: 10.1016/j.medmal.2019.09.005
Furuta, Y., Takahashi, K., Shiraki, K., Sakamoto, K., Smee, D. F., Barnard, D. L., et al. (2009). T-705 (favipiravir) and related compounds: novel broad-spectrum inhibitors of RNA viral infections. Antivir. Res. 82, 95–102. doi: 10.1016/j.antiviral.2009.02.198
Gallo, G., Caignard, G., Badonnel, K., Chevreux, G., Terrier, S., Szemiel, A., et al. (2021). Interactions of viral proteins from pathogenic and low or non-pathogenic Orthohantaviruses with human type I interferon signaling. Viruses 13:140. doi: 10.3390/v13010140
Geisbert, T. W., Hensley, L. E., Jahrling, P. B., Larsen, T., Geisbert, J. B., Paragas, J., et al. (2003). Treatment of Ebola virus infection with a recombinant inhibitor of factor VIIa/tissue factor: a study in rhesus monkeys. Lancet 362, 1953–1958. doi: 10.1016/S0140-6736(03)15012-X
Geisbert, T. W., Young, H. A., Jahrling, P. B., Davis, K. J., Larsen, T., Kagan, E., et al. (2003). Pathogenesis of Ebola hemorrhagic fever in primate models. Am. J. Pathol. 163, 2371–2382. doi: 10.1016/S0002-9440(10)63592-4
He, F. B., Khan, H., Huttunen, M., Kolehmainen, P., Melén, K., Maljanen, S., et al. (2022). Filovirus VP24 proteins differentially regulate RIG-I and MDA5-dependent type I and III interferon promoter activation. Front. Immunol. 12:694105. doi: 10.3389/fimmu.2021.694105
Hensley, L. (2002). Proinflammatory response during Ebola virus infection of primate models: possible involvement of the tumor necrosis factor receptor superfamily. Immunol. Lett. 80, 169–179. doi: 10.1016/S0165-2478(01)00327-3
Hensley, L. E., Stevens, E. L., Yan, S. B., Geisbert, J. B., Macias, W. L., Larsen, T., et al. (2007). Recombinant human activated protein C for the Postexposure treatment of Ebola hemorrhagic fever. J. Infect. Dis. 196, S390–S399. doi: 10.1086/520598
Hickman, M. R., Saunders, D. L., Bigger, C. A., Kane, C. D., and Iversen, P. L. (2022). The development of broad-spectrum antiviral medical countermeasures to treat viral hemorrhagic fevers caused by natural or weaponized virus infections. PLoS Negl. Trop. Dis. 16:e0010220. doi: 10.1371/journal.pntd.0010220
Hjelle, B., and Glass, G. E. (2000). Outbreak of Hantavirus Infection in the Four Corners Region of the United States in the Wake of the 1997–1998 El Niño–Southern Oscillation. J. Infect. Dis. 181, 1569–1573. doi: 10.1086/315467
Holbrook, M. R. (2012). Kyasanur forest disease. Antivir. Res. 96, 353–362. doi: 10.1016/j.antiviral.2012.10.005
Huang, C., Kolokoltsova, O. A., Yun, N. E., Seregin, A. V., Ronca, S., Koma, T., et al. (2015). Highly pathogenic New World and Old World human Arenaviruses induce distinct interferon responses in human cells. J. Virol. 89, 7079–7088. doi: 10.1128/JVI.00526-15
Humaidi, M., Tien, W. P., Yap, G., Chua, C. R., and Ng, L. C. (2021). Non-invasive dengue diagnostics—the use of saliva and urine for different stages of the illness. Diagnostics 11:1345. doi: 10.3390/diagnostics11081345
Ikegami, T., and Makino, S. (2011). The pathogenesis of Rift Valley fever. Viruses 3, 493–519. doi: 10.3390/v3050493
Ikegami, T., Narayanan, K., Won, S., Kamitani, W., Peters, C. J., and Makino, S. (2009). Rift Valley fever Virus NSs protein promotes post-transcriptional Downregulation of protein kinase PKR and inhibits eIF2α phosphorylation. PLoS Pathog. 5:e1000287. doi: 10.1371/journal.ppat.1000287
Ippolito, G., Feldmann, H., Lanini, S., Vairo, F., Di Caro, A., Capobianchi, M. R., et al. (2012). Viral hemorrhagic fevers: advancing the level of treatment. BMC Med. 10:31. doi: 10.1186/1741-7015-10-31
Jin, H., Yan, Z., Prabhakar, B. S., Feng, Z., Ma, Y., Verpooten, D., et al. (2010). The VP35 protein of Ebola virus impairs dendritic cell maturation induced by virus and lipopolysaccharide. J. Gen. Virol. 91, 352–361. doi: 10.1099/vir.0.017343-0
Johnson, D., Jokinen, J., and Lukashevich, I. (2019). Attenuated replication of Lassa Virus vaccine candidate ML29 in STAT-1−/− mice. Pathogens 8:9. doi: 10.3390/pathogens8010009
Jones, B. A., Grace, D., Kock, R., Alonso, S., Rushton, J., Said, M. Y., et al. (2013). Zoonosis emergence linked to agricultural intensification and environmental change. Proc. Natl. Acad. Sci. 110, 8399–8404. doi: 10.1073/pnas.1208059110
Jung, J., Ko, S.-J., Oh, H. S., Moon, S. M., Song, J.-W., and Huh, K. (2018). Protective effectiveness of inactivated hantavirus vaccine against hemorrhagic fever with renal syndrome. J. Infect. Dis. 217, 1417–1420. doi: 10.1093/infdis/jiy037
Kang, H., Cong, J., Wang, C., Ji, W., Xin, Y., Qian, Y., et al. (2021). Structural basis for recognition and regulation of arenavirus polymerase L by Z protein. Nat. Commun. 12:4134. doi: 10.1038/s41467-021-24458-1
Kang, S., and Kishimoto, T. (2021). Interplay between interleukin-6 signaling and the vascular endothelium in cytokine storms. Exp. Mol. Med. 53, 1116–1123. doi: 10.1038/s12276-021-00649-0
Koehler, F. C., Di Cristanziano, V., Späth, M. R., Hoyer-Allo, K. J. R., Wanken, M., Müller, R.-U., et al. (2022). The kidney in hantavirus infection—epidemiology, virology, pathophysiology, clinical presentation, diagnosis and management. Clin. Kidney J. 15, 1231–1252. doi: 10.1093/ckj/sfac008
Ledgerwood, J. E., DeZure, A. D., Stanley, D. A., Coates, E. E., Novik, L., Enama, M. E., et al. (2017). Chimpanzee Adenovirus Vector Ebola Vaccine. N. Engl. J. Med. 376, 928–938. doi: 10.1056/NEJMoa1410863
Levine, J. R., Prescott, J., Brown, K. S., Best, S. M., Ebihara, H., and Feldmann, H. (2010). Antagonism of type I interferon responses by New World hantaviruses. J. Virol. 84, 11790–11801. doi: 10.1128/JVI.00916-10
Li, H., and Förstermann, U. (2000). Nitric oxide in the pathogenesis of vascular disease. J. Pathol. 190, 244–254. doi: 10.1002/(SICI)1096-9896(200002)190:3<244::AID-PATH575>3.0.CO;2-8
Litvoc, M. N., Novaes, C. T. G., and Lopes, M. I. B. F. (2018). Yellow fever. Rev. Assoc. Med. Bras. 64, 106–113. doi: 10.1590/1806-9282.64.02.106
Llah, S. T., Mir, S., Sharif, S., Khan, S., and Mir, M. A. (2018). Hantavirus induced cardiopulmonary syndrome: A public health concern. J. Med. Virol. 90, 1003–1009. doi: 10.1002/jmv.25054
Luthra, P., Ramanan, P., Mire, C. E., Weisend, C., Tsuda, Y., Yen, B., et al. (2013). Mutual antagonism between the Ebola Virus VP35 protein and the RIG-I activator PACT determines infection outcome. Cell Host Microbe 14, 74–84. doi: 10.1016/j.chom.2013.06.010
Madelain, V., Guedj, J., Mentré, F., Nguyen, T. H. T., Jacquot, F., Oestereich, L., et al. (2017). Favipiravir pharmacokinetics in nonhuman primates and insights for future efficacy studies of hemorrhagic fever viruses. Antimicrob. Agents Chemother. 61:e01305-16. doi: 10.1128/AAC.01305-16
Malinin, O. V., and Platonov, A. E. (2017). Insufficient efficacy and safety of intravenous ribavirin in treatment of haemorrhagic fever with renal syndrome caused by Puumala virus. Infect. Dis. 49, 514–520. doi: 10.1080/23744235.2017.1293841
Malvy, D., McElroy, A. K., de Clerck, H., Günther, S., and van Griensven, J. (2019). Ebola virus disease. Lancet 393, 936–948. doi: 10.1016/S0140-6736(18)33132-5
Mansfield, K. L., Jizhou, L., Phipps, L. P., and Johnson, N. (2017). Emerging tick-borne viruses in the twenty-first century. Front. Cell. Infect. Microbiol. 7:298. doi: 10.3389/fcimb.2017.00298
Mariappan, V., Pratheesh, P., Shanmugam, L., Rao, S. R., and Pillai, A. B. (2021). Viral hemorrhagic fever: molecular pathogenesis and current trends of disease management-an update. Curr. Res. Virol. Sci. 2:100009. doi: 10.1016/j.crviro.2021.100009
Mariko, R., Darwin, E., Yanwirasti, Y., and Hadinegoro, S. R. (2019). The difference of sVE-cadherin levels between dengue hemorrhagic fever patients with shock and without shock. Open Access Maced. J. Med. Sci. 7, 2277–2281. doi: 10.3889/oamjms.2019.602
Marty, A. M., Jahrling, P. B., and Geisbert, T. W. (2006). Viral hemorrhagic fevers. Clin. Lab. Med. 26, 345–386. doi: 10.1016/j.cll.2006.05.001
Matthys, V. S., Cimica, V., Dalrymple, N. A., Glennon, N. B., Bianco, C., and Mackow, E. R. (2014). Hantavirus GnT elements mediate TRAF3 binding and inhibit RIG-I/TBK1-directed Beta interferon transcription by blocking IRF3 phosphorylation. J. Virol. 88, 2246–2259. doi: 10.1128/JVI.02647-13
Mazzon, M., Jones, M., Davidson, A., Chain, B., and Jacobs, M. (2009). Dengue Virus NS5 inhibits interferon-α signaling by blocking signal transducer and activator of transcription 2 phosphorylation. J. Infect. Dis. 200, 1261–1270. doi: 10.1086/605847
McLay, L., Liang, Y., and Ly, H. (2014). Comparative analysis of disease pathogenesis and molecular mechanisms of New World and Old World arenavirus infections. J. Gen. Virol. 95, 1–15. doi: 10.1099/vir.0.057000-0
Messaoudi, I., and Basler, C. F. (2015). Immunological features underlying viral hemorrhagic fevers. Curr. Opin. Immunol. 36, 38–46. doi: 10.1016/j.coi.2015.06.003
Meyer, B., and Ly, H. (2016). Inhibition of innate immune responses is key to pathogenesis by Arenaviruses. J. Virol. 90, 3810–3818. doi: 10.1128/JVI.03049-15
Monath, T. P., and Barrett, A. D. (2003). Pathogenesis and pathophysiology of yellow fever. Adv. Virus Res. 60, 343–395. doi: 10.1016/s0065-3527(03)60009-6
Moni, B. M., Sakurai, Y., and Yasuda, J. (2022). Ebola Virus GP activates endothelial cells via host cytoskeletal signaling factors. Viruses 14:142. doi: 10.3390/v14010142
Mousavi-Jazi, M., Karlberg, H., Papa, A., Christova, I., and Mirazimi, A. (2012). Healthy individuals’ immune response to the Bulgarian Crimean-Congo hemorrhagic fever virus vaccine. Vaccine 30, 6225–6229. doi: 10.1016/j.vaccine.2012.08.003
Naranjo-Gómez, J. S., Castillo, J. A., Rojas, M., Restrepo, B. N., Diaz, F. J., Velilla, P. A., et al. (2019). Different phenotypes of non-classical monocytes associated with systemic inflammation, endothelial alteration and hepatic compromise in patients with dengue. Immunology 156, 147–163. doi: 10.1111/imm.13011
Niedrig, M., Patel, P., El Wahed, A. A., Schädler, R., and Yactayo, S. (2018). Find the right sample: A study on the versatility of saliva and urine samples for the diagnosis of emerging viruses. BMC Infect. Dis. 18:707. doi: 10.1186/s12879-018-3611-x
Nielsen, S. S., Alvarez, J., Bicout, D. J., Calistri, P., Depner, K., Drewe, J. A., et al. (2020). Rift Valley fever – epidemiological update and risk of introduction into Europe. EFSA J. 18:6041. doi: 10.2903/j.efsa.2020.6041
NIH (2022). ClinicalTrials.gov https://clinicaltrials.gov/ct2/home (Accessed September 7, 2022).
Olejnik, J., Forero, A., Deflubé, L. R., Hume, A. J., Manhart, W. A., Nishida, A., et al. (2017). Ebolaviruses associated with differential pathogenicity induce distinct host responses in human macrophages. J. Virol. 91:e00179-17. doi: 10.1128/JVI.00179-17
Paessler, S., and Walker, D. H. (2013). Pathogenesis of the viral hemorrhagic fevers. Annu. Rev. Pathol. Mech. Dis. 8, 411–440. doi: 10.1146/annurev-pathol-020712-164041
Patterson, M., Koma, T., Seregin, A., Huang, C., Miller, M., Smith, J., et al. (2014). A substitution in the Transmembrane region of the glycoprotein leads to an unstable attenuation of Machupo Virus. J. Virol. 88, 10995–10999. doi: 10.1128/JVI.01007-14
Perdomo-Celis, F., Salvato, M. S., Medina-Moreno, S., and Zapata, J. C. (2019). T-cell response to viral hemorrhagic fevers. Vaccine 7:11. doi: 10.3390/vaccines7010011
Pereira-dos-Santos, T., Roiz, D., Lourenço-de-Oliveira, R., and Paupy, C. (2020). A systematic review: is Aedes albopictus an efficient bridge vector for zoonotic Arboviruses? Pathogens 9:266. doi: 10.3390/pathogens9040266
Peters, C. J., and Zaki, S. R. (2002). Role of the endothelium in viral hemorrhagic fevers. Crit. Care Med. 30, S268–S273. doi: 10.1097/00003246-200205001-00016
Plowright, R. K., Becker, D. J., McCallum, H., and Manlove, K. R. (2019). Sampling to elucidate the dynamics of infections in reservoir hosts. Philos. Trans. R Soc. B Biol Sci. 374:20180336. doi: 10.1098/rstb.2018.0336
Pythoud, C., Rodrigo, W. W. S. I., Pasqual, G., Rothenberger, S., Martínez-Sobrido, L., de la Torre, J. C., et al. (2012). Arenavirus nucleoprotein targets interferon regulatory factor-activating kinase IKKε. J. Virol. 86, 7728–7738. doi: 10.1128/JVI.00187-12
Quaresma, J. A. S., Barros, V. L. R. S., Pagliari, C., Fernandes, E. R., Andrade, H. F., Vasconcelos, P. F. C., et al. (2007). Hepatocyte lesions and cellular immune response in yellow fever infection. Trans. R. Soc. Trop. Med. Hyg. 101, 161–168. doi: 10.1016/j.trstmh.2006.02.019
Racsa, L. D., Kraft, C. S., Olinger, G. G., and Hensley, L. E. (2016). Viral hemorrhagic fever diagnostics. Clin. Infect. Dis. 62, 214–219. doi: 10.1093/cid/civ792
Raftery, M. J., Abdelaziz, M. O., Hofmann, J., and Schönrich, G. (2018). Hantavirus-driven PD-L1/PD-L2 Upregulation: an imperfect viral immune evasion mechanism. Front. Immunol. 9:2560. doi: 10.3389/fimmu.2018.02560
Regules, J. A., Beigel, J. H., Paolino, K. M., Voell, J., Castellano, A. R., Hu, Z., et al. (2017). A recombinant vesicular stomatitis Virus Ebola vaccine. N. Engl. J. Med. 376, 330–341. doi: 10.1056/NEJMoa1414216
Reynard, S., Russier, M., Fizet, A., Carnec, X., and Baize, S. (2014). Exonuclease domain of the Lassa Virus nucleoprotein is critical to avoid RIG-I signaling and to inhibit the innate immune response. J. Virol. 88, 13923–13927. doi: 10.1128/JVI.01923-14
Rodas, J. D., Cairo, C., Djavani, M., Zapata, J. C., Ruckwardt, T., Bryant, J., et al. (2009). Circulating natural killer and γδ T cells decrease soon after infection of rhesus macaques with lymphocytic choriomeningitis virus. Mem. Inst. Oswaldo Cruz 104, 583–591. doi: 10.1590/S0074-02762009000400009
Rodrigo, W. W. S. I., Ortiz-Riaño, E., Pythoud, C., Kunz, S., de la Torre, J. C., and Martínez-Sobrido, L. (2012). Arenavirus nucleoproteins prevent activation of nuclear factor kappa B. J. Virol. 86, 8185–8197. doi: 10.1128/JVI.07240-11
Rodrigues, R., Paranhos-Baccalà, G., Vernet, G., and Peyrefitte, C. N. (2012). Crimean-Congo hemorrhagic fever Virus-infected hepatocytes induce ER-stress and apoptosis crosstalk. PLoS One 7:e29712. doi: 10.1371/journal.pone.0029712
Rojas, J. M., Alejo, A., Martín, V., and Sevilla, N. (2021). Viral pathogen-induced mechanisms to antagonize mammalian interferon (IFN) signaling pathway. Cell. Mol. Life Sci. 78, 1423–1444. doi: 10.1007/s00018-020-03671-z
Ruibal, P., Oestereich, L., Lüdtke, A., Becker-Ziaja, B., Wozniak, D. M., Kerber, R., et al. (2016). Unique human immune signature of Ebola virus disease in Guinea. Nature 533, 100–104. doi: 10.1038/nature17949
Russier, M., Reynard, S., Carnec, X., and Baize, S. (2014). The exonuclease domain of Lassa Virus nucleoprotein is involved in antigen-presenting-cell-mediated NK cell responses. J. Virol. 88, 13811–13820. doi: 10.1128/JVI.01908-14
Safronetz, D., Falzarano, D., Scott, D. P., Furuta, Y., Feldmann, H., and Gowen, B. B. (2013). Antiviral efficacy of Favipiravir against two prominent etiological agents of hantavirus pulmonary syndrome. Antimicrob. Agents Chemother. 57, 4673–4680. doi: 10.1128/AAC.00886-13
Schmid, M. A., Diamond, M. S., and Harris, E. (2014). Dendritic cells in dengue Virus infection: targets of Virus replication and mediators of immunity. Front. Immunol. 5:647. doi: 10.3389/fimmu.2014.00647
Schnittler, H.-J., and Feldmann, H. (2003). Viral hemorrhagic fever--a vascular disease? Thromb. Haemost. 89, 967–972.
Scholte, F. E. M., Zivcec, M., Dzimianski, J. V., Deaton, M. K., Spengler, J. R., Welch, S. R., et al. (2017). Crimean-Congo hemorrhagic fever Virus suppresses innate immune responses via a ubiquitin and ISG15 specific protease. Cell Rep. 20, 2396–2407. doi: 10.1016/j.celrep.2017.08.040
Schönrich, G., and Raftery, M. J. (2019). Dendritic cells (DCs) as “fire accelerants” of Hantaviral pathogenesis. Viruses 11:849. doi: 10.3390/v11090849
Seitsonen, E., Hynninen, M., Kolho, E., Kallio-Kokko, H., and Pettilä, V. (2006). Corticosteroids combined with continuous veno-venous hemodiafiltration for treatment of hantavirus pulmonary syndrome caused by Puumala virus infection. Eur. J. Clin. Microbiol. Infect. Dis. 25, 261–266. doi: 10.1007/s10096-006-0117-z
Shah, S. Z., Jabbar, B., Ahmed, N., Rehman, A., Nasir, H., Nadeem, S., et al. (2018). Epidemiology, pathogenesis, and control of a tick-borne disease- Kyasanur Forest disease: current status and future directions. Front. Cell. Infect. Microbiol. 8:149. doi: 10.3389/fcimb.2018.00149
Singh, S., Numan, A., Sharma, D., Shukla, R., Alexander, A., Jain, G. K., et al. (2022). Epidemiology, virology and clinical aspects of hantavirus infections: an overview. Int. J. Environ. Health Res. 32, 1815–1826. doi: 10.1080/09603123.2021.1917527
Soares-Weiser, K., Thomas, S., and G GT, Garner P., (2010). Ribavirin for Crimean-Congo hemorrhagic fever: systematic review and meta-analysis. BMC Infect. Dis. 10:207. doi: 10.1186/1471-2334-10-207
Solà-Riera, C., Gupta, S., Ljunggren, H.-G., and Klingström, J. (2019). Orthohantaviruses belonging to three phylogroups all inhibit apoptosis in infected target cells. Sci. Rep. 9:834. doi: 10.1038/s41598-018-37446-1
Song, J. Y., Woo, H. J., Cheong, H. J., Noh, J. Y., Baek, L. J., and Kim, W. J. (2016). Long-term immunogenicity and safety of inactivated Hantaan virus vaccine (Hantavax™) in healthy adults. Vaccine 34, 1289–1295. doi: 10.1016/j.vaccine.2016.01.031
Souza, D. G., Fagundes, C. T., Sousa, L. P., Amaral, F. A., Souza, R. S., Souza, A. L., et al. (2009). Essential role of platelet-activating factor receptor in the pathogenesis of dengue virus infection. Proc. Natl. Acad. Sci. 106, 14138–14143. doi: 10.1073/pnas.0906467106
Speranza, E., Ruibal, P., Port, J. R., Feng, F., Burkhardt, L., Grundhoff, A., et al. (2018). T-cell receptor diversity and the control of T-cell homeostasis mark Ebola Virus disease survival in humans. J. Infect. Dis. 218, S508–S518. doi: 10.1093/infdis/jiy352
Sridhar, S., Luedtke, A., Langevin, E., Zhu, M., Bonaparte, M., Machabert, T., et al. (2018). Effect of dengue Serostatus on dengue vaccine safety and efficacy. N. Engl. J. Med. 379, 327–340. doi: 10.1056/NEJMoa1800820
Sung, J.-M., Lee, C.-K., and Wu-Hsieh, B. A. (2012). Intrahepatic infiltrating NK and CD8 T cells cause liver cell death in different phases of dengue Virus infection. PLoS One 7:e46292. doi: 10.1371/journal.pone.0046292
Taylor, S. L., Wahl-Jensen, V., Copeland, A. M., Jahrling, P. B., and Schmaljohn, C. S. (2013). Endothelial cell permeability during hantavirus infection involves factor XII-dependent increased activation of the Kallikrein-Kinin system. PLoS Pathog. 9:e1003470. doi: 10.1371/journal.ppat.1003470
Temur, A. I., Kuhn, J. H., Pecor, D. B., Apanaskevich, D. A., and Keshtkar-Jahromi, M. (2021). Epidemiology of Crimean-Congo hemorrhagic fever (CCHF) in Africa—underestimated for decades. Am. J. Trop. Med. Hyg. 104, 1978–1990. doi: 10.4269/ajtmh.20-1413
Tersago, K., Verhagen, R., Servais, A., Heyman, P., Ducoffre, G., and Leirs, H. (2009). Hantavirus disease (nephropathia epidemica) in Belgium: effects of tree seed production and climate. Epidemiol. Infect. 137, 250–256. doi: 10.1017/S0950268808000940
The International Committee on Taxonomy of Viruses (ICTV) (2022). Available at: https://ictv.global/taxonomy
Tuffs, A. (2009). Experimental vaccine may have saved Hamburg scientist from Ebola fever. BMJ 338, b1223–b1223. doi: 10.1136/bmj.b1223
Valinetz, E. D., and Cangelosi, G. A. (2021). A look inside: Oral sampling for detection of non-oral infectious diseases. J. Clin. Microbiol. 59:e0236020. doi: 10.1128/JCM.02360-20
Valmas, C., and Basler, C. F. (2011). Marburg Virus VP40 antagonizes interferon signaling in a species-specific manner. J. Virol. 85, 4309–4317. doi: 10.1128/JVI.02575-10
Vial, P. A., Valdivieso, F., Calvo, M., Rioseco, M. L., Riquelme, R., Araneda, A., et al. (2015). A non-randomized multicentre trial of human immune plasma for treatment of hantavirus cardiopulmonary syndrome caused by Andes Virus. Antivir. Ther. 20, 377–386. doi: 10.3851/IMP2875
Vilibic-Cavlek, T., Savic, V., Ferenc, T., Mrzljak, A., Barbic, L., Bogdanic, M., et al. (2021). Lymphocytic Choriomeningitis—emerging trends of a neglected Virus: A narrative review. Trop. Med. Infect. Dis. 6:88. doi: 10.3390/tropicalmed6020088
Villar, L., Dayan, G. H., Arredondo-García, J. L., Rivera, D. M., Cunha, R., Deseda, C., et al. (2015). Efficacy of a tetravalent dengue vaccine in children in Latin America. N. Engl. J. Med. 372, 113–123. doi: 10.1056/NEJMoa1411037
Wagner, E., Shin, A., Tukhanova, N., Turebekov, N., Nurmakhanov, T., Sutyagin, V., et al. (2022). First indications of Omsk Haemorrhagic fever Virus beyond Russia. Viruses 14:754. doi: 10.3390/v14040754
Wauquier, N., Becquart, P., Padilla, C., Baize, S., and Leroy, E. M. (2010). Human fatal Zaire Ebola Virus infection is associated with an aberrant innate immunity and with massive lymphocyte apoptosis. PLoS Negl. Trop. Dis. 4:e837. doi: 10.1371/journal.pntd.0000837
West, B. R., Hastie, K. M., and Saphire, E. O. (2014). Structure of the LCMV nucleoprotein provides a template for understanding arenavirus replication and immunosuppression. Acta Crystallogr. Sect. D Biol. Crystallogr. 70, 1764–1769. doi: 10.1107/S1399004714007883
Whiteside, S. K., Snook, J. P., Williams, M. A., and Weis, J. J. (2018). Bystander T cells: A balancing act of friends and foes. Trends Immunol. 39, 1021–1035. doi: 10.1016/j.it.2018.10.003
WHO (2022a). Four countries in the African region license vaccine in milestone for Ebola prevention. Available at: https://www.who.int/news/item/14-02-2020-four-countries-in-the-african-region-license-vaccine-in-milestone-for-ebola-prevention [Accessed August 2, 2022].
WHO (2022b). International Clinical Trials Registry Platform (ICTRP). Available at: https://trialsearch.who.int/ [Accessed September 7, 2022].
Wikel, S. (2018). Ticks and tick-borne infections: complex ecology, agents, and host interactions. Vet. Sci. 5:60. doi: 10.3390/vetsci5020060
Wilder-Smith, A., Ooi, E.-E., Horstick, O., and Wills, B. (2019). Dengue. Lancet 393, 350–363. doi: 10.1016/S0140-6736(18)32560-1
Winkler, A. M., and Koepsell, S. A. (2015). The use of convalescent plasma to treat emerging infectious diseases. Curr. Opin. Hematol. 22, 521–526. doi: 10.1097/MOH.0000000000000191
Won, S., Ikegami, T., Peters, C. J., and Makino, S. (2007). NSm protein of Rift Valley fever Virus suppresses Virus-induced apoptosis. J. Virol. 81, 13335–13345. doi: 10.1128/JVI.01238-07
Woolsey, C., Cross, R. W., Agans, K. N., Borisevich, V., Deer, D. J., Geisbert, J. B., et al. (2022). A highly attenuated Vesiculovax vaccine rapidly protects nonhuman primates against lethal Marburg virus challenge. PLoS Negl. Trop. Dis. 16:e0010433. doi: 10.1371/journal.pntd.0010433
Wormser, G. P., Dailey, N. J. M., and Fleischauer, A. T. (2010). Sustaining Global Surveillance and Response to Emerging Zoonotic Diseases Edited by Gerald T. Keusch, Marguerite Pappaioanou, Mila C. Gonzalez, Kimberly A. Scott, and Peggy Tsai Washington, DC: The National Academies Press, 2009. 312 pp. $42.30 paperback). $36 (E-book). Clinical Infectious Diseases. 51, 875–876. doi: 10.1086/656239
Xing, J., Chai, Z., Ly, H., and Liang, Y. (2015). Differential inhibition of macrophage activation by lymphocytic Choriomeningitis Virus and Pichinde Virus is mediated by the Z protein N-terminal domain. J. Virol. 89, 12513–12517. doi: 10.1128/JVI.01674-15
Xing, J., Ly, H., and Liang, Y. (2015). The Z proteins of pathogenic but not nonpathogenic Arenaviruses inhibit RIG-i-like receptor-dependent interferon production. J. Virol. 89, 2944–2955. doi: 10.1128/JVI.03349-14
Yang, B., and Yang, K. D. (2021). Immunopathogenesis of different emerging viral infections: evasion, fatal mechanism, and prevention. Front. Immunol. 12, 1–18. doi: 10.3389/fimmu.2021.690976
Yen, B., Mulder, L. C. F., Martinez, O., and Basler, C. F. (2014). Molecular basis for Ebolavirus VP35 suppression of human dendritic cell maturation. J. Virol. 88, 12500–12510. doi: 10.1128/JVI.02163-14
Younan, P., Iampietro, M., Nishida, A., Ramanathan, P., Santos, R. I., Dutta, M., et al. (2017). Ebola Virus binding to Tim-1 on T lymphocytes induces a cytokine storm. MBio 8:e00845-17. doi: 10.1128/mBio.00845-17
Keywords: hemorrhagic fever viruses, viral hemorrhagic fevers, fatal viral disease, emerging, re-emerging infectious diseases
Citation: Flórez-Álvarez L, de Souza EE, Botosso VF, de Oliveira DBL, Ho PL, Taborda CP, Palmisano G, Capurro ML, Pinho JRR, Ferreira HL, Minoprio P, Arruda E, de Souza Ferreira LC, Wrenger C and Durigon EL (2022) Hemorrhagic fever viruses: Pathogenesis, therapeutics, and emerging and re-emerging potential. Front. Microbiol. 13:1040093. doi: 10.3389/fmicb.2022.1040093
Edited by:
Juan C. De La Torre, The Scripps Research Institute, United StatesReviewed by:
Olivier Escaffre, University of Texas Medical Branch at Galveston, United StatesCopyright © 2022 Flórez-Álvarez, de Souza, Botosso, de Oliveira, Ho, Taborda, Palmisano, Capurro, Pinho, Ferreira, Minoprio, Arruda, de Souza Ferreira, Wrenger and Durigon. This is an open-access article distributed under the terms of the Creative Commons Attribution License (CC BY). The use, distribution or reproduction in other forums is permitted, provided the original author(s) and the copyright owner(s) are credited and that the original publication in this journal is cited, in accordance with accepted academic practice. No use, distribution or reproduction is permitted which does not comply with these terms.
*Correspondence: Carsten Wrenger, Y3dyZW5nZXJAaWNiLnVzcC5icg==; Edison Luiz Durigon, ZWxkdXJpZ29AdXNwLmJy
†These authors have contributed equally to this work