- 1School of Environmental Science and Engineering, Tongji University, Shanghai, China
- 2School of Environment and Architecture, University of Shanghai for Science and Technology, Shanghai, China
- 3YANGTZE Eco-Environment Engineering Research Center, China Three Gorges Corporation, Beijing, China
The thermophilic spore-forming strain Geobacillus sp. CX412 was isolated from hot spring soil in Tengchong City, Yunnan Province, China. We sequenced the complete genome of Geobacillus sp. CX412 using PacBio SMRT Sequencing. Genome-scale phylogenetic analysis and average nucleotide identity (ANI) results indicated that Geobacillus sp. CX412 is a novel species in the genus Geobacillus. The metabolic potential of Geobacillus sp. CX412 based on COG, KEGG, and CAZymes analysis demonstrated that Geobacillus sp. CX412 was a highly adaptable strain with an unusually high number of 73 annotated transposons in the genome, which is relatively rare in Geobacillus. Compared with the near-derived strains, it was found that Geobacillus sp. CX412 has the unique β-lactam resistance and more active metabolism (more than 50.5–100.1%). Additionally, its genome encodes glycoside hydrolases and other genes related to lignocellulose breakdown, suggesting that Geobacillus sp. CX412 has a considerable biomass degradation potential. Thus, Geobacillus sp. CX412 is a new thermophilic bacterial species that add to the increasing repertoire of known lignocellulose degraders.
Introduction
Geobacillus were categorized initially as “Group 5” in the genus Bacillus. They were subsequently split into the new genus based on 16S rRNA gene sequence analysis, phenotypic characterization, and DNA-DNA hybridization experiments, including thermophilic gram-positive spore-forming bacteria that form phylogenetically consistent clades within the Bacillus family (Nazina et al., 2001; Chen et al., 2015; Brumm et al., 2016). In 2016, the genus Geobacillus was subdivided into two genera based on whole-genome approaches, with the addition of Parageobacillus (Aliyu et al., 2016, 2018; Najar et al., 2020). In 2020, Reclassification of Geobacillus galactosidasius and Geobacillus yumthangensis as Parageobacillus galactosidasius comb. nov. and Parageobacillus yumthangensis comb. nov., respectively (Najar et al., 2020). Therefore, Geobacillus and Parageobacillus are relatively similar in the phylogenetic tree and often cross over.
Geobacillus species have been found mainly in hot springs in the United States (Brumm et al., 2015a,b), Africa (Hawumba et al., 2002), and Russia (Nazina et al., 2004), the Mariana Trench (Takami et al., 2004), deep-sea vents (Maugeri et al., 2002), high-temperature oilfields (Kuisiene et al., 2004), a corroded pipeline in an extremely deep well (Popova et al., 2002), and composting materials (Bhalla et al., 2013; Li et al., 2014; Brumm et al., 2016). It demonstrates the ability of Geobacillus to thrive in this diverse and often harsh environment and suggests that these species have enzymes suitable for application in challenging industrial environments (such as enzymes that efficiently break down lignocellulose) (Bouzas et al., 2006; Bergquist et al., 2014; Chen et al., 2015). Geobacillus species can grow in high-temperature environments (up to 70°C or more), and the advantages of using thermophilic bacteria as whole-cell biocatalysts include reduced risk of contamination and accelerated biochemical processes in fermentation (Chen et al., 2015). Composting Materials, as the main sources of thermal bacteria, also imply that thermal bacteria would use organic matter to self-reproduce during composting. When antibiotic production residue is used as compost substrate, due to the inhibitory and poisoning effect of antibiotics, thermal bacteria may not be able to reproduce and grow well, which would reduce the composting effect (Yang et al., 2016). Therefore, finding bacteria that may resist antibiotics under high-temperature conditions is necessary. Geobacillus are generally used in complex environments, and the number of genes coding for transposons implies the adaptability of Geobacillus to the environment (Frost et al., 2005). For example, the genome of Geobacillus sp. WCH70 has 125 annotation transpositions, which indicates that Geobacillus sp. WCH70 has a highly variable chromosomal, which can add or delete non-essential genes and gene clusters according to environmental conditions (Brumm et al., 2016).
Furthermore, many glycolytic thermophiles can use polymeric or short oligomeric carbohydrates with low nutritional requirements to produce lactic acid, formic acid, acetic acid, and ethanol as products (Niehaus et al., 1999; Taylor et al., 2009). Strains such as Geobacillus thermoglucosidasius DSM2542 have been developed for industrial bioethanol production from lignocellulosic feedstocks (Cripps et al., 2009; Chen et al., 2015). Geobacillus sp. Strain DUSELR13 has been developed for thermostable xylanase and ethanol production with lignocellulosic biomass (Bibra et al., 2018). Geobacillus sp. strain WSUCF1 is a thermophilic exopolysaccharide-producing bacterium and producing highly thermostable xylanase utilizing lignocellulosic biomas (Bhalla et al., 2014; Wang et al., 2019, 2021). Therefore, the study of Geobacillus as a significant source of thermostable enzymes and a platform host for lignocellulosic biomass natural products is critical (Chen et al., 2015).
Geobacillus sp. CX412 was isolated from Tengchong City, Yunnan Province, China. The genome of Geobacillus sp. CX412 strain was sequenced, and its metabolic potential was analyzed.
Materials and methods
Organism information
Classification and features
Geobacillus sp. CX412 is a novel thermophilic species obtained from hot spring soil in Tengchong City, Yunnan Province, China (24.953861° latitude and 98.443661° longitude). The organism was isolated from hot spring soil by enrichment and plating on a screening medium (screening medium contains (per liter) 8.0 g tryptone, 7.0 g casein, 3.0 g glucose, 5.0 g sodium chloride, 2.0 g disodium hydrogen phosphate, 10.0 g dehydrated calf brain extract, 15.0 g agar, pH 7.0–7.4) at 75°C.
Genome sequencing information
Illumina Hiseq is used for sequencing to obtain the original data of the sequencing. FastQC assesses the quality of the original sequencing data, and then the Illumina sequencing data is cut by Trimmomatic (Bolger et al., 2014) to obtain relatively accurate and practical data. The Pacific Biosciences (PacBio) RS II is used for sequencing, and the original data is quality-cut to obtain high-quality data. Pacbio/single-molecule sequencing data were assembled using Canu (Koren et al., 2017), Illumina Hiseq sequencing data were introduced, and GapFiller (Boetzer and Pirovano, 2012) was used to complement the assembled scaffolds with GAP. Finally, sequence correction was performed using PrInSeS-G (Massouras et al., 2010). The editing errors and indels were fixed in segments during splicing. After obtaining the genome sequence, Prokka (Seemann, 2014) was used to predict the genetic elements: gene, tRNA, rRNA, etc. Sequencing was done at Sangon Biotech (Shanghai) Co., Ltd.
Taxonomic assignment and phylogenetic analysis
The predicted 16S rRNA sequence was compared with the NCBI 16S database using NCBI Blast+ (Altschul et al., 1997) to obtain information on its homologous strains, and a phylogenetic tree was constructed. Download genome sequences of approximate strains, and perform average nucleotide identity (ANI) and digital DNA-DNA hybridization (DDH) were analyzed by JSpeciesWS and GGDC 3.0, respectively (Richter et al., 2016; Meier-Kolthoff et al., 2022).
Functional annotation
NCBI Blast+ (Altschul et al., 1997) was used to compare the gene protein sequence with the COG database (Tatusov et al., 2000) to obtain its functional annotation information, KAAS (Kanehisa and Goto, 2000; Moriya et al., 2007) was used to obtain the gene KEGG annotation information, and HMMER3 (Eddy, 2009) was used to compare the gene protein sequence with the Carbohydrate active enzymes (CAZymes) database (Lombard et al., 2014) to obtain its functional annotation information.
Accession numbers
The complete genome information of Geobacillus sp. CX412 was deposited in GenBank under the accession number CP103461-CP103464.
Results and discussion
Complete genome sequence of Geobacillus sp. CX412
Geobacillus sp. CX412 is a Gram-positive, rod-shaped bacterium with an optimum growth temperature of 75°C and a maximum growth temperature of 85°C (Table 1). The total genome length of Geobacillus sp. CX412 was 3,560,825 bp, the average G + C content was 42.5%, and there were 91 tRNA genes and 26 rRNA genes (Table 2 and Figure 1). There are 3,763 predicted protein-coding regions in the genome (Table 2). A total of 2,678 genes (71.17%) were annotated in the COG database, and about 30% of the annotated genes were not assigned to COG or had unknown functions (Table 3).
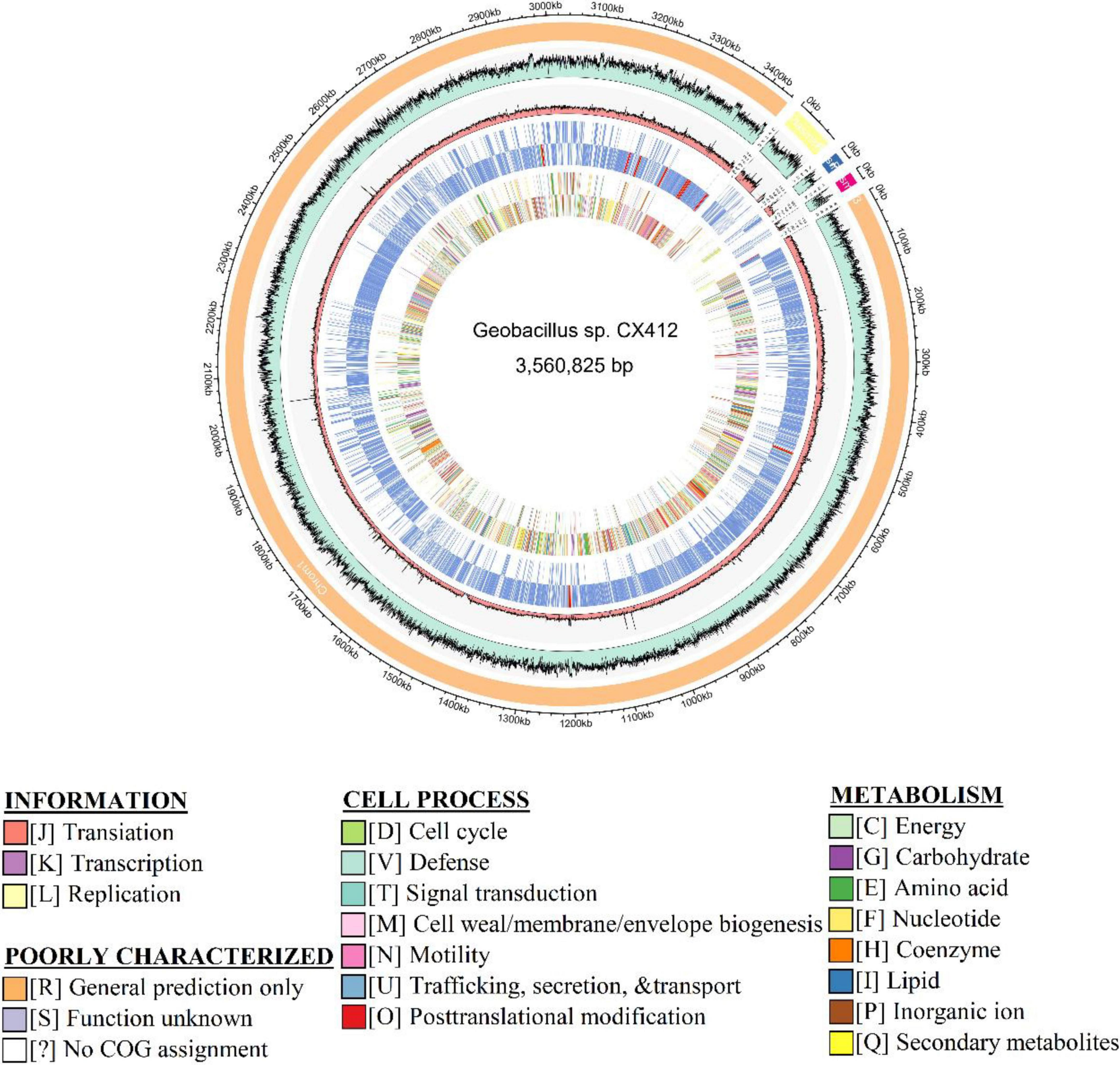
Figure 1. Genome map of Geobacillus sp. CX412. From outer to inner: Scale marks in kb, GC%, Coverage, Gene category, and COG category, respectively.
Taxonomic assignment and phylogenetic analysis
After the 16S rRNA sequences were compared in the NCBI database, 16S rRNA sequences of the strains were selected according to the similarity to construct a phylogenetic tree. As shown in Figure 2, Geobacillus sp. CX412 is closely related to other Geobacillus and is an independent branch in the phylogenetic tree, confirming that it is Geobacillus. The four closest strains with complete genome sequences (Table 2) were selected for comparative analysis, and the results showed that these genomes shared 1,315 homologous gene clusters.
Average Nucleotide Identity (ANI) is an indicator for comparing the relatedness of two genomes at the nucleotide level. ANI is the average base similarity between homologous segments of two microbial genomes, characterized by a high degree of discrimination between closely related species. Compared with the traditional DDH, the calculation of the ANI index is simple and time-saving, and it is helpful to build a structured database, which is convenient for the follow-up research of bioinformatics scholars (Brumm et al., 2016). The ANI of Geobacillus sp. CX412 and the closely related strain Geobacillus sp. WCH70 was 92.1%, and the ANI of the strain Parageobacillus toebii NBRC 107807 was 91.4%, lower than the new species’ critical value of 95% (Figure 3). At the same time, the DDH of Geobacillus sp. CX412 and Geobacillus sp. WCH70 was 36.8%, and the DDH of Parageobacillus toebii 107,807 was 35.7%, lower than the new species’ critical value of 70% (Supplementary Table 1). This suggests that Geobacillus sp. CX412 should be a new Geobacillus sp.
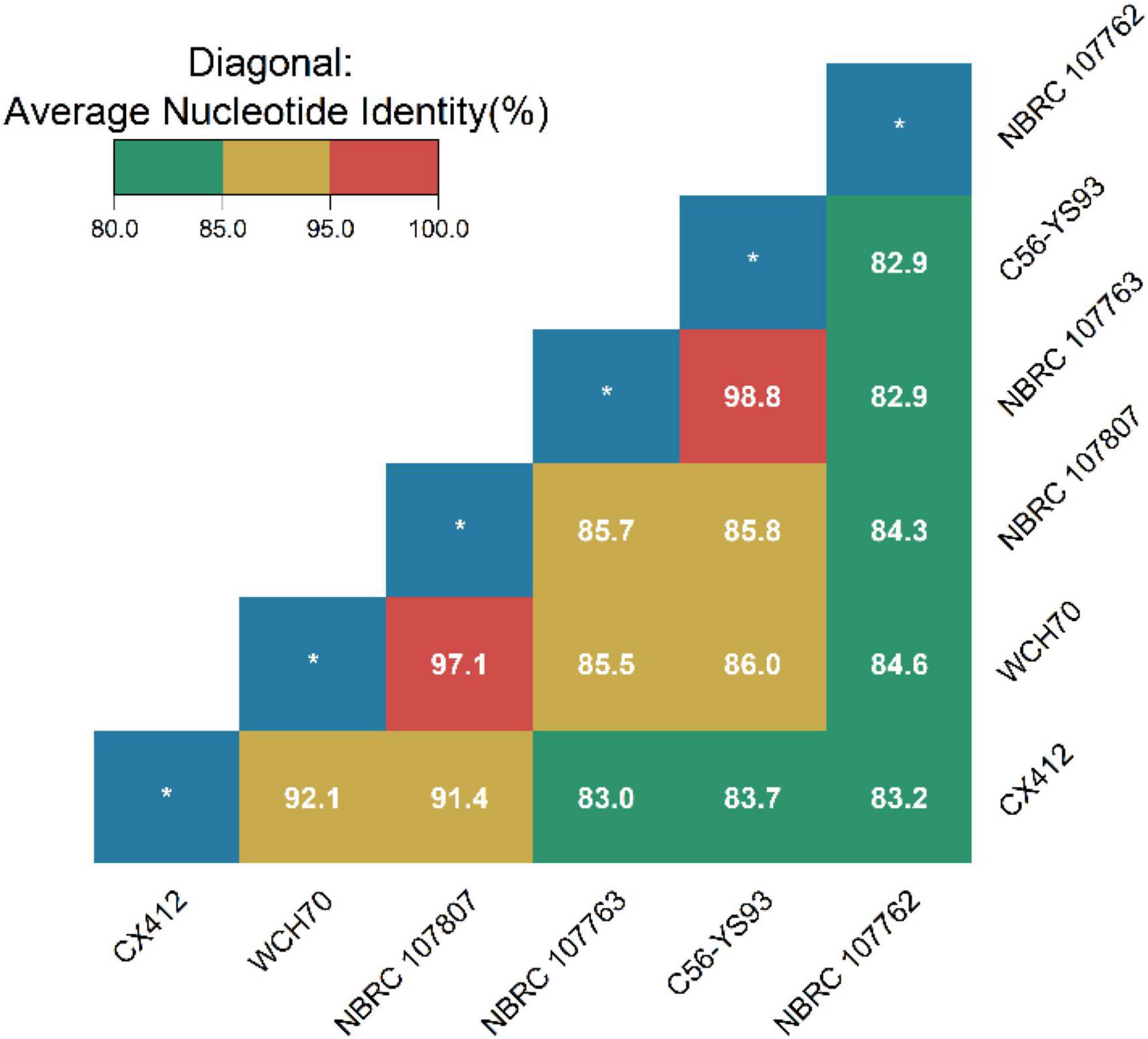
Figure 3. The average nucleotide identity (ANI) values (%). CX412, Geobacillus sp. CX412; WCH70, Geobacillus sp. WCH70; NBRC 107807, Parageobacillus toebii NBRC 107807; NBRC 107763, Parageobacillus thermoglucosidasius NBRC 107763; C56-YS93, Parageobacillus thermoglucosidasius C56-YS93; NBRC 107762, Parageobacillus caldoxylosilyticus NBRC 107762. *Represents self-ANI analysis with a theory of 100.
Comparison with other Geobacillus
In order to better understand the characteristics of Geobacillus sp. CX412, the number and metabolic potential of Geobacillus sp. CX412 and the other four species with complete genome sequence similarity were analyzed based on COG and CAZymes.
It shows that superoxide dismutase (SOD) is an essential protein for cells to resist high temperature, and Cu/Zn superoxide dismutase (SOD1) enzymes under Cu2+/Zn2+ presence can also make cells have high-temperature resistance (Askwith et al., 1994). At the same time, ClpP protein is found to affect the temperature resistance of the strain (Gerth et al., 2008). As a thermophilic bacterium, Geobacillus sp. strain CX412 should have genes related to high-temperature tolerance. The results showed that Geobacillus sp. CX412 had related genes for SOD, SOD1, and ClpP proteins; the related genes generally existed in the near-derived strains (Supplementary Table 2). The existence of related genes suggests the reasons for the high-temperature resistance of Geobacillus sp. CX412.
The ability of Geobacillus to thrive in this diverse and often harsh environment may be due to the predicted encoding transposons of many Geobacillus species (Bouzas et al., 2006; Bergquist et al., 2014). To some extent, the number of predicted coding transposons indicates the variability of the organism’s chromosomes, which can add or delete non-essential genes and gene clusters according to environmental conditions, representing the ability of the organism to adapt to the environment (Brumm et al., 2016). As shown in Table 4, Geobacillus sp. CX412 contained 73 predicted coding transposons. After comparing with the near-derived strains and reviewing the literature (Supplementary Table 3; Brumm et al., 2016), it was found that the predicted number of transposons encoded by Geobacillus sp. CX412 in Geobacillus was more than three times that of Parageobacillus toebii NBRC 107807. At the same time, the predicted number of transposons encoded by Geobacillus sp. CX412 in Geobacillus was significantly more than that of Parageobacillus thermoglucosidasius NBRC 107763 and Parageobacillus thermoglucosidasius C56-YS93. It shows that Geobacillus sp. CX412 also has a strong ability to adapt to the environment.
KEGG (Kyoto Encyclopedia of Genes and Genomes) is a comprehensive database of biological systems that integrates genomic, chemical, and system functional information. KEGG GENES collects all known complete genome gene protein sequences, including the minimum information for each gene. The KO (KEGG ORTHOLOG) system links the various KEGG annotation systems together. After the KO annotation of the gene, the KEGG metabolic pathway classification is carried out according to the connection between the KO and pathway. There are seven categories: cellular processes, environmental information processing, genetic information processing, human diseases, metabolism, organismal systems, and drug development.
In order to analyze the metabolic pathway of Geobacillus sp. CX412, the genes of Geobacillus sp. CX412 and other four near-derived strains were compared with the KEGG functional pathway database for functional annotation (Figure 4). The proportion of six functional genes of Geobacillus sp. CX412 was 2.8% respectively (cellular processes), 11.6% (environmental information processing), 9.3% (genetic information processing), 1.8% (human diseases), 73.2% (metabolism), 1.2% (organismal systems). It was indicated that there are six categories of functional genes of Geobacillus sp. CX412 (excluding drug development). At the same time, it can be seen from Figure 4 that the metabolic function genes of Geobacillus sp. CX412 are mainly carbohydrate metabolism and amino acid metabolism, and the metabolic function genes of Geobacillus sp. CX412 are significantly more than those of other near-derived strains (more than 50.5–100.1%). It was revealed that Geobacillus sp. CX412 is the strain with more robust metabolism in the genus Geobacillus. In addition, Geobacillus sp. CX412 has the human disease group that other near-derived strains do not have, in which the number of genes annotated to the ko00312 pathway (β-lactam resistance) accounts for 34.6% of the total genes associated with human disease. The near-derived strain Geobacillus sp. WCH70 of Geobacillus sp. CX412 was isolated from the aerobic fermenter (Brumm et al., 2016). Combined with the results of the KEGG analysis, it could be seen that Geobacillus sp. CX412 could also be used in composting, which had related genes of β-lactam resistance and more active metabolism (Figure 4). It also implies that the Geobacillus sp. CX412 has a broader range of applications.
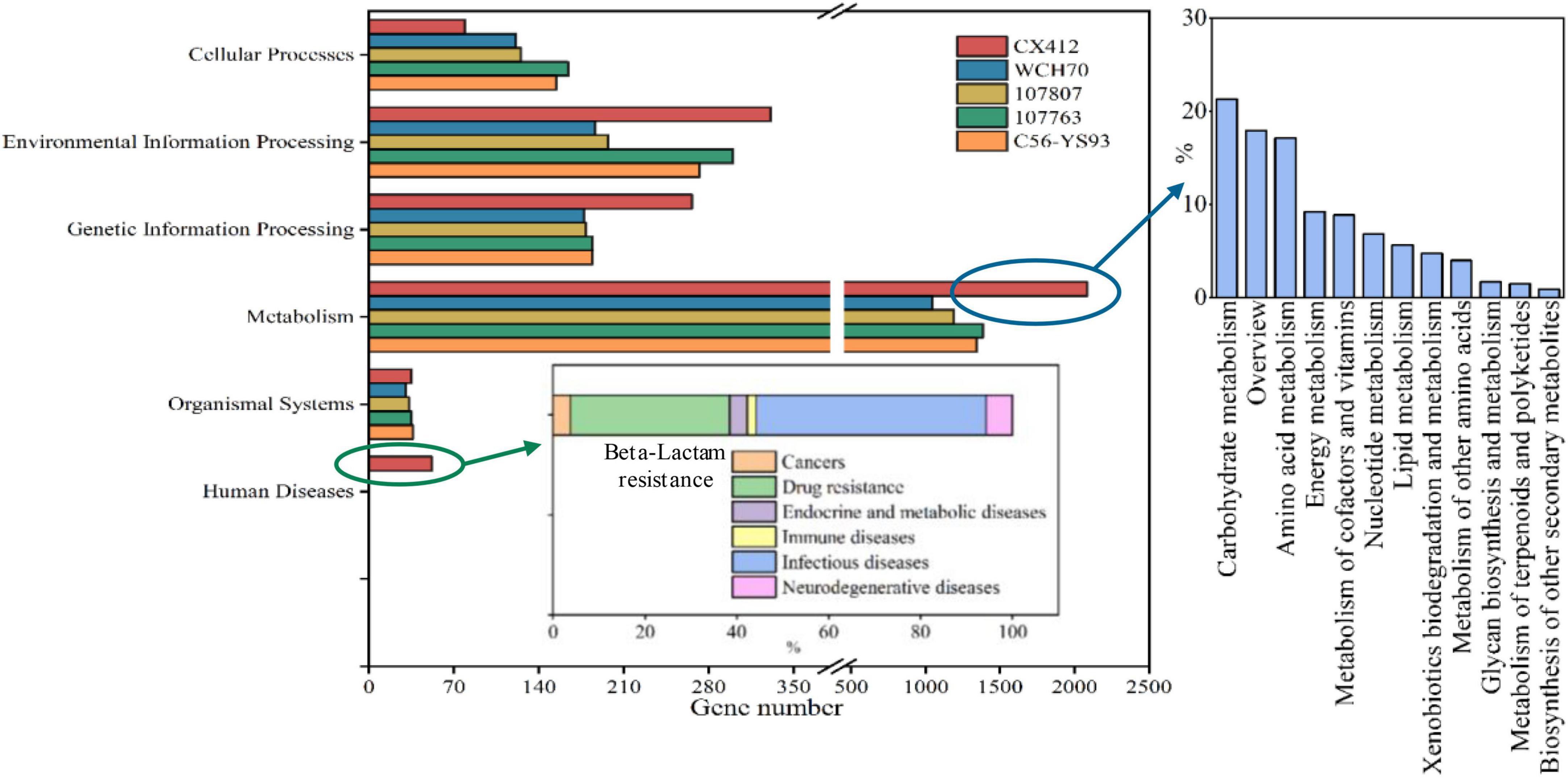
Figure 4. KEGG Pathway categories histogram. CX412, Geobacillus sp. CX412; WCH70, Geobacillus sp. WCH70; 107807, Parageobacillus toebii NBRC 107807; 107763, Parageobacillus thermoglucosidasius NBRC 107763; C56-YS93, Parageobacillus thermoglucosidasius C56-YS93.
Studies have also shown that Geobacillus species can grow in high-temperature environments (up to 70°C or more), and the advantages of using thermophilic bacteria as whole-cell biocatalysts include reducing the risk of contamination and accelerating biochemical processes in fermentation (Chen et al., 2015). Unexpectedly, Geobacillus sp. WCH70 lacks the predicted polysaccharide degradation clusters in many Geobacillus species, including metabolic clusters for hemicellulose degradation (Markowitz et al., 2014; Brumm et al., 2016). Nearly related strains of Geobacillus sp. CX412 include Geobacillus sp. WCH70. Therefore, to determine the metabolic potential of Geobacillus sp. CX412, CAZymes analysis was performed (Supplementary Table 4; Figure 5). CAZymes are divided into different families such as glycoside hydrolases (GH), glycosyltransferases (GT), carbohydrate-binding modules (CBM), carbohydrate esterases (CE), accessory activity (AA), and polysaccharide lyase (PL) (Lemos et al., 2017). Geobacillus sp. CX412 encompassing all six CAZymes families, as follows: 24.5% GHs, 30.8% GTs, 17.6% CEs, 15.7% AAs, 10.1% CBMs, and 1.3% PLs.
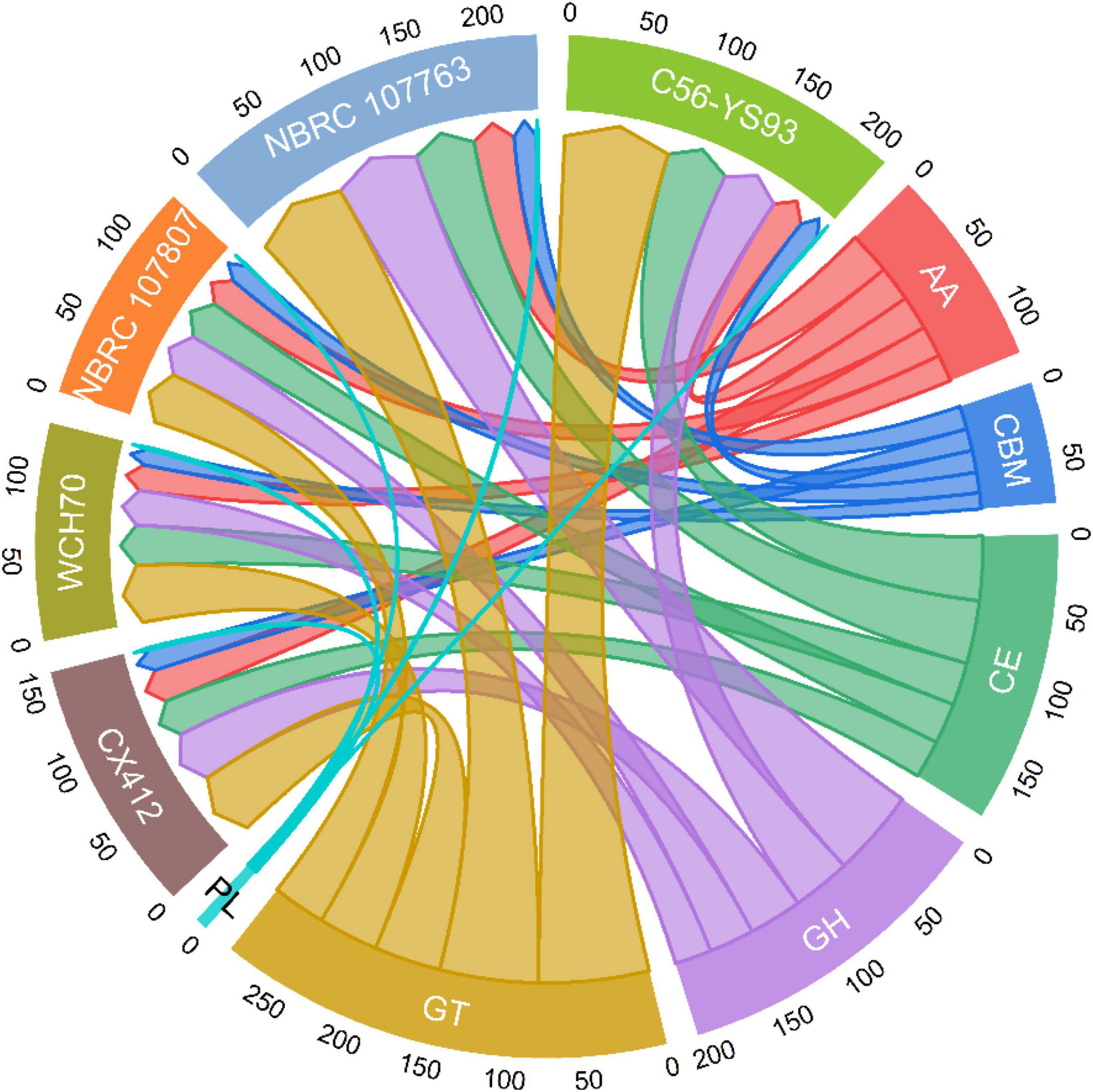
Figure 5. Carbohydrate-Active Enzymes (CAZymes). CAZymes classification result: AA, Auxiliary Activities; CBM, Carbohydrate-Binding Modules; CE, Carbohydrate Esterases; GH, Glycoside Hydrolases; GT, Glycosyl Transferases; PL, Polysaccharide Lyase; CX412, Geobacillus sp. CX412; WCH70, Geobacillus sp. WCH70; 107807, Parageobacillus toebii NBRC 107807; 107763, Parageobacillus thermoglucosidasius NBRC 107763; C56-YS93, Parageobacillus thermoglucosidasius C56-YS93.
CAZymes are involved in constructing and breaking down complex carbohydrates and glycoconjugates in various biological processes (Lemos et al., 2017; Gavande et al., 2021). CBMs are the necessary modules for cellulolytic enzymes to bind to their substrates. AAs are involved in the degradation of lignin polymers, and CEs are the key to efficient hemicellulase activity. Cellulases and hemicellulases in GHs play an essential role in cellulose depolymerization (Gavande et al., 2021). Therefore, the genes encoding lignocellulose-degrading enzymes were screened, and 45 related genes were found in Geobacillus sp. CX412. Among them, there are 12 kinds of enzymes related to cellulolysis (GH1, GH4, GH5, GH9, GH74, and AA7) and 16 kinds of enzymes related to hemicellulose (GH2, GH4, GH36, GH43, GH130, CE1, and CE4), and 17 lignin oxidases (AA1, AA3, AA4, and AA6). The GH36 family is found only in the Geobacillus sp. CX412 genome (Figure 6). The GH36 family includes a thermostable hemicellulose (Lemos et al., 2017). Parageobacillus thermoglucosidasius C56-YS93, isolated from Yellowstone National Park in the United States, is a biomass degrader which can effectively degrade lignocellulose (Brumm et al., 2015c). Geobacillus sp. CX412 contains 18 lignocellulose-degrading enzymes, and Parageobacillus thermoglucosidasius C56-YS93 contains 22 kinds (Figure 6). Compared with Parageobacillus thermoglucosidasius C56-YS93, it can be found that Geobacillus sp. CX412 can also effectively degrade lignocellulose.
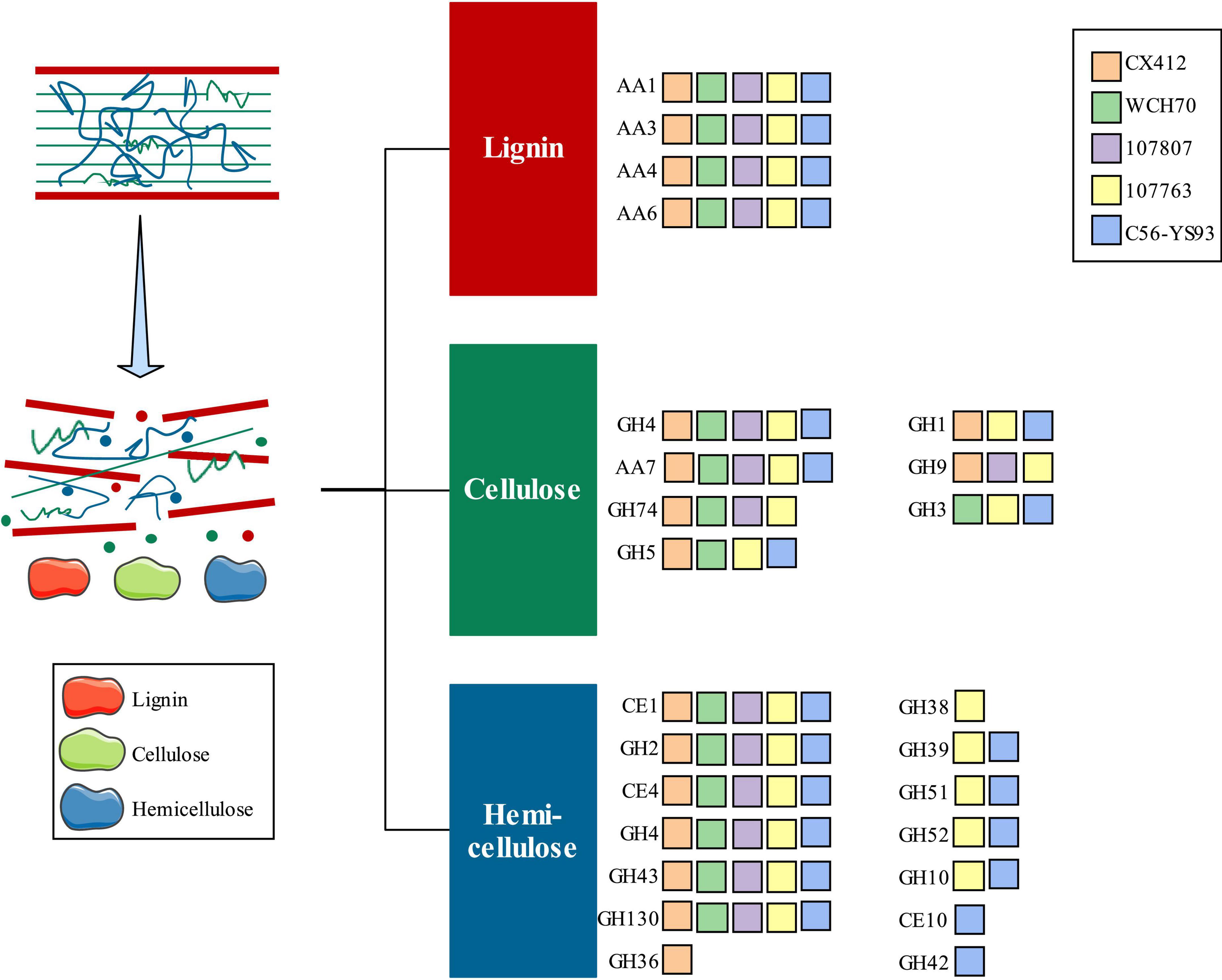
Figure 6. An overview of lignocellulose degradation. AA, Auxiliary Activities; CE, Carbohydrate Esterases; GH, Glycoside Hydrolases. CX412, Geobacillus sp. CX412; WCH70, Geobacillus sp. WCH70; 107807, Parageobacillus toebii NBRC 107807; 107763, Parageobacillus thermoglucosidasius NBRC 107763; C56-YS93, Parageobacillus thermoglucosidasius C56-YS93.
Conclusion
Geobacillus sp. CX412 is a gram-positive, rod-shaped bacterium with an optimum growth temperature of 75°C, a maximum growth temperature of 85°C, and an average G + C content of 42.5%. There are 91 tRNA genes and 26 rRNA genes. Seventy-three predicted coding transposons indicate that Geobacillus sp. CX412 has a highly variable chromosome and that Geobacillus sp. CX412 has a strong ability to adapt to the environment. Compared with the near-derived strains with KEGG analysis, it was found that Geobacillus sp. CX412 has the unique β-lactam resistance and more active metabolism (more than 50.5–100.1%). It was also implied that the Geobacillus sp. CX412 has a broader range of applications. Analysis of the metabolic potential of Geobacillus sp. CX412 showed that Geobacillus sp. CX412 contained 45 genes related to lignocellulose degradation. Among them, there are 12 enzymes related to cellulolysis, 16 kinds of enzymes related to hemicellulose, and 17 lignin oxidases. Geobacillus sp. CX412 has the potential to efficiently degrade lignocellulose. These findings add to the growing library of known lignocellulose degradants and support further research into their biotechnological potential.
Data availability statement
The data presented in this study are deposited in the GenBank repository, accession numbers: CP103461–CP103464.
Author contributions
XL: methodology, software, data curation, writing – original draft, formal analysis, and validation. WZ: conceptualization, methodology, and validation. X-RZ: investigation and data curation. H-XH: investigation and validation. BD: conceptualization, resources, writing – review and editing, and supervision. All authors contributed to the article and approved the submitted version.
Funding
This research was supported by the Project National Natural Science Foundation of China (Nos. 52270136 and 52192684), the National Key Research and Development Project (2020YFC1908700 and 2021YFC3200700), and the Consulting Research Project of Chinese Academy of Engineering (2021-SH-XY-2).
Conflict of interest
Author BD was employed by YANGTZE Eco-Environment Engineering Research Center of China Three Gorges Corporation.
The remaining authors declare that the research was conducted in the absence of any commercial or financial relationships that could be construed as a potential conflict of interest.
Publisher’s note
All claims expressed in this article are solely those of the authors and do not necessarily represent those of their affiliated organizations, or those of the publisher, the editors and the reviewers. Any product that may be evaluated in this article, or claim that may be made by its manufacturer, is not guaranteed or endorsed by the publisher.
Supplementary material
The Supplementary Material for this article can be found online at: https://www.frontiersin.org/articles/10.3389/fmicb.2022.1035311/full#supplementary-material
Abbreviations
ANI, average nucleotide identity; DDH, digital DNA-DNA hybridization; ME, minimum-evolution; COG, cluster of orthologous groups of proteins; KEGG, Kyoto encyclopedia of genes and genomes; CAZymes, carbohydrate active enzymes.
References
Aliyu, H., Lebre, P., Blom, J., Cowan, D., and De Maayer, P. (2016). Phylogenomic re-assessment of the thermophilic genus Geobacillus. Syst. Appl. Microbiol. 39, 527–533.
Aliyu, H., Lebre, P., Blom, J., Cowan, D., and De Maayer, P. (2018). Phylogenomic re-assessment of the thermophilic genus Geobacillus (vol 39, pg 527, 2016). Syst. Appl. Microbiol. 41, 529–530. doi: 10.1016/j.syapm.2016.09.004
Altschul, S. F., Madden, T. L., Schaffer, A. A., Zhang, J. H., Zhang, Z., Miller, W., et al. (1997). Gapped BLAST and PSI-BLAST: A new generation of protein database search programs. Nucleic Acids Res. 25, 3389–3402. doi: 10.1093/nar/25.17.3389
Askwith, C., Eide, D., Van Ho, A., Bernard, P. S., Li, L., Davis-Kaplan, S., et al. (1994). The FET3 gene of S. cerevisiae encodes a multicopper oxidase required for ferrous iron uptake. Cell 76, 403–410. doi: 10.1016/0092-8674(94)90346-8
Bergquist, P. L., Morgan, H. W., and Saul, D. (2014). Selected enzymes from extreme thermophiles with applications in biotechnology. Curr. Biotechnol. 3, 45–59. doi: 10.2174/2211550102999131230150918
Bhalla, A., Bischoff, K. M., Uppugundla, N., Balan, V., and Sani, R. K. (2014). Novel thermostable endo-xylanase cloned and expressed from bacterium Geobacillus sp WSUCF1. Bioresour. Technol. 165, 314–318. doi: 10.1016/j.biortech.2014.03.112
Bhalla, A., Kainth, A. S., and Sani, R. K. (2013). Draft Genome Sequence of Lignocellulose-Degrading Thermophilic Bacterium Geobacillus sp. Strain WSUCF1. Genome Announc. 1:e00595–e00613. doi: 10.1128/genomeA.00595-13
Bibra, M., Kunreddy, V. R., and Sani, R. K. (2018). Thermostable Xylanase Production by Geobacillus sp Strain DUSELR13, and Its Application in Ethanol Production with Lignocellulosic Biomass. Microorganisms 6:93. doi: 10.3390/microorganisms6030093
Boetzer, M., and Pirovano, W. (2012). Toward almost closed genomes with GapFiller. Genome Biol. 13:R56. doi: 10.1186/gb-2012-13-6-r56
Bolger, A. M., Lohse, M., and Usadel, B. (2014). Trimmomatic: A flexible trimmer for Illumina sequence data. Bioinformatics 30, 2114–2120. doi: 10.1093/bioinformatics/btu170
Bouzas, T. D. M., Barros-Velazquez, J., and Gonzalez Villa, T. (2006). Industrial applications of hyperthermophilic enzymes: A review. Protein Pept. Lett. 13, 645–651. doi: 10.2174/092986606777790548
Brumm, P., Land, M. L., Hauser, L. J., Jeffries, C. D., Chang, Y. J., and Mead, D. A. (2015a). Complete Genome Sequence of Geobacillus strain Y4.1MC1, a Novel CO-Utilizing Geobacillus thermoglucosidasius Strain Isolated from Bath Hot Spring in Yellowstone National Park. Bioenergy Res. 8, 1039–1045. doi: 10.1007/s12155-015-9585-2
Brumm, P. J., De Maayer, P., Mead, D. A., and Cowan, D. A. (2015b). Genomic analysis of six new Geobacillus strains reveals highly conserved carbohydrate degradation architectures and strategies. Front. Microbiol. 6:430. doi: 10.3389/fmicb.2015.00430
Brumm, P. J., Land, M. L., and Mead, D. A. (2015c). Complete genome sequence of Geobacillus thermoglucosidasius C56-YS93, a novel biomass degrader isolated from obsidian hot spring in Yellowstone National Park. Stand. Genom. Sci. 10:73. doi: 10.1186/s40793-015-0031-z
Brumm, P. J., Land, M. L., and Mead, D. A. (2016). Complete genome sequences of Geobacillus sp WCH70, a thermophilic strain isolated from wood compost. Stand. Genom. Sci. 11:33. doi: 10.1186/s40793-016-0153-y
Chen, J. Y., Zhang, Z. Z., Zhang, C. L., and Yu, B. (2015). Genome sequence of Geobacillus thermoglucosidasius DSM2542, a platform hosts for biotechnological applications with industrial potential. J. Biotechnol. 216, 98–99. doi: 10.1016/j.jbiotec.2015.10.002
Cripps, R. E., Eley, K., Leak, D. J., Rudd, B., Taylor, M., Todd, M., et al. (2009). Metabolic engineering of Geobacillus thermoglucosidasius for high yield ethanol production. Metab. Eng. 11, 398–408. doi: 10.1016/j.ymben.2009.08.005
Eddy, S. R. (2009). A new generation of homology search tools based on probabilistic inference. Genome informatics. Int. Conf. Genome Inform. 23, 205–211. doi: 10.1142/9781848165632_0019
Frost, L. S., Leplae, R., Summers, A. O., and Toussaint, A. (2005). Mobile genetic elements: The agents of open source evolution. Nat. Rev. Microbiol. 3, 722–732. doi: 10.1038/nrmicro1235
Gavande, P. V., Basak, A., Sen, S., Lepcha, K., Murmu, N., Rai, V., et al. (2021). Functional characterization of thermotolerant microbial consortium for lignocellulolytic enzymes with central role of Firmicutes in rice straw depolymerization. Sci. Rep. 11:3032. doi: 10.1038/s41598-021-82163-x
Gerth, U., Kock, H., Kusters, I., Michalik, S., Switzer, R. L., and Hecker, M. (2008). Clp-dependent proteolysis down-regulates central metabolic pathways in glucose-starved Bacillus subtilis. J. Bacteriol. 190, 321–331. doi: 10.1128/JB.01233-07
Hawumba, J. F., Theron, J., and Brozel, V. S. (2002). Thermophilic protease-producing Geobacillus from Buranga hot springs in western Uganda. Curr. Microbiol. 45, 144–150. doi: 10.1007/s00284-001-0116-3
Kanehisa, M., and Goto, S. (2000). KEGG: Kyoto encyclopedia of genes and genomes. Nucleic Acids Res. 28, 27–30. doi: 10.1093/nar/28.1.27
Koren, S., Walenz, B. P., Berlin, K., Miller, J. R., Bergman, N. H., and Phillippy, A. M. (2017). Canu: Scalable and accurate long-read assembly via adaptive k-mer weighting and repeat separation. Genome Res. 27, 722–736. doi: 10.1101/gr.215087.116
Kuisiene, N., Raugalas, J., and Chitavichius, D. (2004). Geobacillus lituanicus sp nov. Int. J. Syst. Evol. Microbiol. 54, 1991–1995. doi: 10.1099/ijs.0.02976-0
Lemos, L. N., Pereira, R. V., Quaggio, R. B., Martins, L. F., Moura, L. M. S., da Silva, A. R., et al. (2017). Genome-Centric Analysis of a Thermophilic and Cellulolytic Bacterial Consortium Derived from Composting. Front. Microbiol. 8:644. doi: 10.3389/fmicb.2017.00644
Li, R., Li, L., Huang, R., Sun, Y., Mei, X., Shen, B., et al. (2014). Variations of culturable thermophilic microbe numbers and bacterial communities during the thermophilic phase of composting. World J. Microbiol. Biotechnol. 30, 1737–1746. doi: 10.1007/s11274-013-1593-9
Lombard, V., Ramulu, H. G., Drula, E., Coutinho, P. M., and Henrissat, B. (2014). The carbohydrate-active enzymes database (CAZy) in 2013. Nucleic Acids Res. 42, D490–D495. doi: 10.1093/nar/gkt1178
Markowitz, V. M., Chen, I. M. A., Palaniappan, K., Chu, K., Szeto, E., Pillay, M., et al. (2014). IMG 4 version of the integrated microbial genomes comparative analysis system. Nucleic Acids Res. 42, D560–D567. doi: 10.1093/nar/gkt963
Massouras, A., Hens, K., Gubelmann, C., Uplekar, S., Decouttere, F., Rougemont, J., et al. (2010). Primer-initiated sequence synthesis to detect and assemble structural variants. Nat. Methods 7, 485–486. doi: 10.1038/nmeth.f.308
Maugeri, T. L., Gugliandolo, C., Caccamo, D., and Stackebrandt, E. (2002). Three novel halotolerant and thermophilic Geobacillus strains from shallow marine vents. Syst. Appl. Microbiol. 25, 450–455. doi: 10.1078/0723-2020-00119
Meier-Kolthoff, J. P., Carbasse, J. S., Peinado-Olarte, R. L., and Goker, M. (2022). TYGS and LPSN: A database tandem for fast and reliable genome-based classification and nomenclature of prokaryotes. Nucleic Acids Res. 50, D801–D807. doi: 10.1093/nar/gkab902
Moriya, Y., Itoh, M., Okuda, S., Yoshizawa, A. C., and Kanehisa, M. (2007). KAAS: An automatic genome annotation and pathway reconstruction server. Nucleic Acids Res. 35, W182–W185.
Najar, I. N., Das, S., and Thakur, N. (2020). Reclassification of Geobacillus galactosidasius and Geobacillus yumthangensis as Parageobacillus galactosidasius comb. nov. and Parageobacillus yumthangensis comb. nov., respectively. Int. J. Syst. Evol. Microbiol. 70, 6518–6523. doi: 10.1099/ijsem.0.004550
Nazina, T. N., Lebedeva, E. V., Poltaraus, A. B., Tourova, T. P., Grigoryan, A. A., Sokolova, D. S., et al. (2004). Geobacillus gargensis sp nov., a novel thermophile from a hot spring, and the reclassification of Bacillus vulcani as Geobacillus vulcani comb. nov. Int. J. Syst. Evol. Microbiol. 54, 2019–2024. doi: 10.1099/ijs.0.02932-0
Nazina, T. N., Tourova, T. P., Poltaraus, A. B., Novikova, E. V., Grigoryan, A. A., Ivanova, A. E., et al. (2001). Taxonomic study of aerobic thermophilic bacilli: Descriptions of Geobacillus subterraneus gen. nov., sp nov and Geobacillus uzenensis sp nov from petroleum reservoirs and transfer of Bacillus stearothermophilus Bacillus thermocatenulatus, Bacillus thermoleovorans, Bacillus kaustophilus, Bacillus thermoglucosidasius and Bacillus thermodenitrificans to Geobacillus as the new combinations G-stearothermophilus, G-thermocatenulatus, G-thermoleovorans, G-kaustophilus, G-thermoglucosidasius and G-thermodenitrificans. Int. J. Syst. Evol. Microbiol. 51, 433–446. doi: 10.1099/00207713-51-2-433
Niehaus, F., Bertoldo, C., Kähler, M., and Antranikian, G. (1999). Extremophiles as a source of novel enzymes for industrial application. Appl. Microbiol. Biotechnol. 51, 711–729.
Popova, N. A., Nikolaev, I. A., Turova, T. P., Lysenko, A. M., Osipov, G. A., Verkhovtseva, N. V., et al. (2002). [Geobacillus uralicus, a new species of thermophilic bacteria]. Mikrobiologiia 71, 391–398.
Richter, M., Rossello-Mora, R., Gloeckner, F. O., and Peplies, J. (2016). JSpeciesWS: a web server for prokaryotic species circumscription based on pairwise genome comparison. Bioinformatics 32, 929–931. doi: 10.1093/bioinformatics/btv681
Takami, H., Nishi, S., Lu, J., Shinamura, S., and Takaki, Y. (2004). Genomic characterization of thermophilic Geobacillus species isolated from the deepest sea mud of the Mariana Trench. Extremophiles 8, 351–356. doi: 10.1007/s00792-004-0394-3
Tatusov, R. L., Galperin, M. Y., Natale, D. A., and Koonin, E. V. (2000). The COG database: A tool for genome-scale analysis of protein functions and evolution. Nucleic Acids Res. 28, 33–36. doi: 10.1093/nar/28.1.33
Taylor, M. P., Eley, K. L., Martin, S., Tuffin, M. I., Burton, S. G., and Cowan, D. A. (2009). Thermophilic ethanologenesis: Future prospects for second-generation bioethanol production. Trends Biotechnol. 27, 398–405. doi: 10.1016/j.tibtech.2009.03.006
Wang, J., Goh, K. M., Salem, D. R., and Sani, R. K. (2019). Genome analysis of a thermophilic exopolysaccharide-producing bacterium - Geobacillus sp. WSUCF1. Sci. Rep. 9:1608. doi: 10.1038/s41598-018-36983-z
Wang, J., Salem, D. R., and Sani, R. K. (2021). Two new exopolysaccharides from a thermophilic bacterium Geobacillus sp. WSUCF1: Characterization and bioactivities. N. Biotechnol. 61, 29–39. doi: 10.1016/j.nbt.2020.11.004
Keywords: Geobacillus sp. CX412, thermophile, transposons, β-lactam resistance, lignocellulose
Citation: Li X, Zhang W, Zhong X-R, Han H-X and Dong B (2022) Genome sequencing analysis of a novel thermophilic strain Geobacillus sp. CX412. Front. Microbiol. 13:1035311. doi: 10.3389/fmicb.2022.1035311
Received: 02 September 2022; Accepted: 10 October 2022;
Published: 11 November 2022.
Edited by:
Laura Zucconi, University of Tuscia, ItalyReviewed by:
Mohit Bibra, Zymergen Inc., United StatesJia Wang, The University of Tennessee, Knoxville, United States
Copyright © 2022 Li, Zhang, Zhong, Han and Dong. This is an open-access article distributed under the terms of the Creative Commons Attribution License (CC BY). The use, distribution or reproduction in other forums is permitted, provided the original author(s) and the copyright owner(s) are credited and that the original publication in this journal is cited, in accordance with accepted academic practice. No use, distribution or reproduction is permitted which does not comply with these terms.
*Correspondence: Bin Dong, ZG9uZ2JpbkB0b25namkuZWR1LmNu