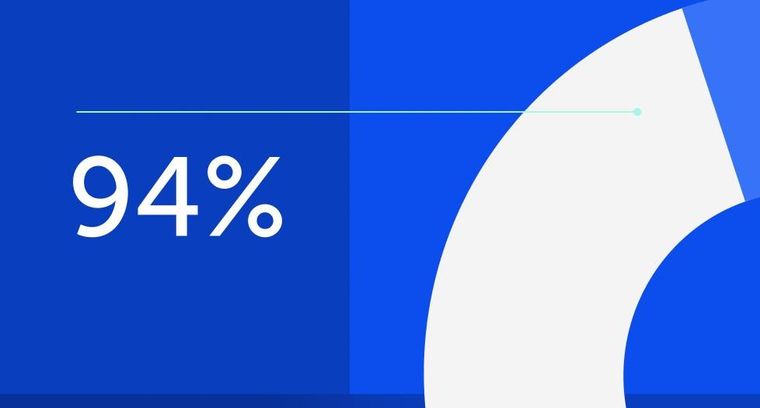
94% of researchers rate our articles as excellent or good
Learn more about the work of our research integrity team to safeguard the quality of each article we publish.
Find out more
PERSPECTIVE article
Front. Microbiol., 18 June 2021
Sec. Microbial Physiology and Metabolism
Volume 12 - 2021 | https://doi.org/10.3389/fmicb.2021.679665
This article is part of the Research TopicFungal BiotechnologyView all 13 articles
pRS episomal plasmids are widely used in Saccharomyces cerevisiae, owing to their easy genetic manipulations and high plasmid copy numbers (PCNs). Nevertheless, their broader application is hampered by the instability of the pRS plasmids. In this study, we designed an episomal plasmid based on the endogenous 2μ plasmid with both improved stability and increased PCN, naming it p2μM, a 2μ-modified plasmid. In the p2μM plasmid, an insertion site between the REP1 promoter and RAF1 promoter was identified, where the replication (ori) of Escherichia coli and a selection marker gene of S. cerevisiae were inserted. As a proof of concept, the tyrosol biosynthetic pathway was constructed in the p2μM plasmid and in a pRS plasmid (pRS423). As a result, the p2μM plasmid presented lower plasmid loss rate than that of pRS423. Furthermore, higher tyrosol titers were achieved in S. cerevisiae harboring p2μM plasmid carrying the tyrosol pathway-related genes. Our study provided an improved genetic manipulation tool in S. cerevisiae for metabolic engineering applications, which may be widely applied for valuable product biosynthesis in yeast.
Yeast, especially Saccharomyces cerevisiae (S. cerevisiae), has been developed as a host organism for the heterologous production of high-value compounds (Luo et al., 2015; Suastegui and Shao, 2016; Gao et al., 2017; Cao M. et al., 2020; Cao X. et al., 2020; Liu H. et al., 2020; Liu Q. et al., 2020; Ren et al., 2020), free fatty acid (Zhang et al., 2019), soluble cytosolic proteins (Boulet et al., 2017; González et al., 2018; Huang et al., 2018; Zhang et al., 2019), and biofuels (Zhang et al., 2021). Many genetic manipulations of S. cerevisiae rely on the utilization of plasmids (Romanos et al., 1992). There are three commonly used plasmids: (1) yeast-integrating plasmid (YIp) lacks the yeast replication initiation site and can only be stabilized when integrated into the yeast chromosome (Jensen et al., 2014). However, YIp brings only one copy of target sequences to the chromosome. (2) Yeast centromere plasmid (YCp) contains an autonomously replicating sequence (ARS) and a yeast centromere (CEN) (Chlebowicz-Śledziewska and Śledziewski, 1985; Lee et al., 2016), which has high mitotic stability but low copy number. (3) Yeast episomal plasmid (YEp) harbors a 2μ plasmid replication origin and a partitioning locus (STB or REP3) (Murray and Cesareni, 1986), which has high copy numbers but low stability (Hohnholz et al., 2017). In summary, plasmids with stable expression usually cannot provide high copy number, while plasmids with high copy number will be easily lost after long-term fermentation in the nutrient medium. Therefore, a stable plasmid system with high copy number is urgently needed.
Yeast endogenous 2μ plasmid is a cryptic nuclear plasmid (Stevens and Moustacchi, 1971; Petes and Williamson, 1975), which confers no phenotype beyond the ability to maintain itself a high copy number at 60–330 copies per cell with the help of FLP-mediated recombination (Gerbaud et al., 1979; Murray and Cesareni, 1986; Reider Apel et al., 2017). The 2μ plasmid is a circular DNA plasmid with a size of 6,318 bp and a circumference of about 2 μm (Hartley and Donelson, 1980).
In the 2μ plasmid, there is an ∼600-bp DNA sequence essential for the faithful partitioning of the 2μ plasmid along with the trans-acting ORFs REP1 and REP2 (Kikuchi, 1983), named STB (Murray and Cesareni, 1986). In the absence of STB, the 2μ-based plasmids are rapidly lost due to extreme mother bias during mitosis. In addition, the 2μ plasmid codes for four proteins (REP1, REP2, RAF1, and FLP) that are vital for its own survival. REP1 and REP2 are the primary factors responsible for the 2μ plasmid stability (Jayaram et al., 1983). RAF1 interacts with both REP1 and REP2 independently and blocks their interaction, thus reducing the cellular concentration of the REP1–REP2 complex that acts as a repressor of REP1, FLP, and RAF1 genes. This blockage resulted in reduced plasmid stability and increased plasmid copy number (PCN). Both the deletion and overexpression of RAF1 have a similar effect on the plasmid stability and copy number, resulting in an increased PCN and decreased plasmid stability (Rizvi et al., 2018). FLP is a conservative site-specific recombinase (Sadowski, 1995). The flip of one half of the 2μ plasmid with respect to the other is predominantly FLP dependent (Gerbaud et al., 1979; Broach and Hicks, 1980). The FLP-mediated recombination is also believed to be responsible for the interconversion of the plasmid replication between the theta and the rolling circle modes of replication.
Many researchers took advantage of the high PCN and stable inheritance of the 2μ plasmid to directly transform 2μ plasmid as an expression tool. Ludwig et al. selected the HPAI restriction site of STB as the insertion site (Ludwig and Bruschi, 1991), but the loss of STB led to a high loss rate of the plasmid (Murray and Szostak, 1983; McQuaid et al., 2019). Misumi et al. (2018) inserted the yeast promoter, terminator, and nutritional deficiency marker gene leu2 between RAF1 and STB and called this plasmid YHp. The application of YHp was restricted in [cir0] strains (Misumi et al., 2018). Zeng et al. (2021) chose two sites as the targets for insertion of heterogeneous DNA fragment: one is at the downstream of the RAF1, while the other is at the end of REP2. The derivative plasmids generated by inserting the same target gene at these two sites have lower plasmid loss rates and better expression level than the conventional 2μ-based plasmid pRS425 (Zeng et al., 2021). To our knowledge, no commonly used methods have been developed in laboratory strains with the wild-type (WT) 2μ plasmid (Supplementary Figure 1A).
Based on these previous studies described above (Hartley and Donelson, 1980; Jayaram et al., 1983; Rizvi et al., 2018; McQuaid et al., 2019), we identified a new insertion site between the REP1 promoter and RAF1 promoter (Supplementary Figure 1B). The pBR322ori, KanMX selection marker gene, and three endonuclease sites XhoI/PmeI/NotI were inserted in this site. The 2μ-modified plasmid was named p2μM. In plasmid stability measurement, the p2μM plasmid system was more stable than the pRS423 plasmid system. To test the application of p2μM in the biosynthesis of natural products, the tyrosol [a phenethyl alcohol derivative that has antioxidant and anti-inflammatory effects (Choe et al., 2012)] pathway-related genes were introduced into p2μM. The results confirmed that the stability and property of the p2μM were better than those of the pRS423M plasmid. Our study provided an improved genetic manipulation tool in S. cerevisiae for metabolic engineering applications, and it may be widely applied in valuable natural product biosynthesis in yeast.
In order to construct a stable endogenous 2μ-based plasmid and apply it for DNA expression and pathway construction, the proper insertion site should be selected to insert essential elements and heterogeneous DNA fragments. Besides the known genes and sequences, there are still uncharacterized transcripts transcribed from the 2μ plasmid (Rizvi et al., 2017). It was found that the promoters of RAF1 and REP1 on the endogenous 2μ plasmid were adjacent and there was no other element between them by analyzing the elements related to stability. Thus, this site was selected as the insertion site (Supplementary Figure 1A). To edit the endogenous 2μ plasmid for a better genetic manipulation tool, the origin replication of Escherichia coli, combined with G418 resistance marker, was chosen to be inserted to construct p2μM (Supplementary Figure 1B).
To characterize the property of the p2μM plasmid, plasmid pRS423 with G418 resistance was chosen as a control to generate plasmid pRS423M (Supplementary Figure 1C). Plasmid pRS423 is also commonly used in yeast among the YEp pRS42 series plasmids due to its relatively high stability and copy number (Christianson et al., 1992).
Tyrosol is mainly extracted from olive oil, wine, and plant tissues. It has proven to be an effective cellular antioxidant and is widely used in food and medicine industries (Benedetto et al., 2007; Karković Marković et al., 2019). Taking into account the impact of the size of inserted fragment on the p2μM plasmid, we constructed three modules of different sizes using genes of the tyrosol biosynthetic pathway (Supplementary Figure 1D). The small module (mutation module, 3.8 kb) of ARO4K229L and ARO7G141S could efficiently relieve feedback inhibition and increase the production of tyrosol in S. cerevisiae (Liu H. et al., 2020), which was introduced to generate plasmid p2μM-ARO4K229L-ARO7G141S (p2μM-small-module). The rewiring module containing pentose phosphate pathway genes TKL1 and RKI1 could tune the flux of the precursor pathway (Walfridsson et al., 1996; Kondo et al., 2004; Bera et al., 2011). The adjustment module that contains ARO2 and ARO10 could adjust the shikimate pathway and L-tyrosine branch by catalyzing the conversion of chorismate from EPSP and the decarboxylation of 4-HPP to 4-HPPA (Liu H. et al., 2020), respectively. The medium module (9.8 kb) composed of the rewiring module and the adjustment module was overexpressed by p2μM plasmid, resulting in plasmid p2μM-TKL1-RKI1-ARO10-ARO2 (p2μM-medium-module). Finally, the medium module was introduced into plasmid p2μ-small-module, resulting in plasmid p2μM-TKL1-RKI1-ARO10-ARO2-ARO4K229L-ARO7G141S (p2μM-large-module, the size of the large module was 13.6 kb). Then, these three modules were also inserted into the multiple cloning sites of plasmid pRS423M to generate pRS423M-small-module, pRS423M-medium-module, and pRS423M-large-module, collectively called pRS423M-based plasmids (Supplementary Figure 1F). The structures of the three modules are shown in Supplementary Figure 2.
Since the yeast endogenous 2μ plasmid showed high stability and copy number, we assumed that our p2μM plasmid could be more stable than the pRS423M plasmid. To test this hypothesis, the influences of the size of the inserted fragment on the stability of the p2μM plasmid were explored via measuring the plasmid loss rate. As shown in Supplementary Tables 1, 2, the stabilities of the p2μM-based plasmids were significantly higher than those of the pRS423M-based plasmids. First, plasmid p2μM and pRS423M were transformed to S. cerevisiae strain CEN.PK2-1C, respectively. Then, the plasmid loss rates of the 10th, 20th, 40th, and 50th generation strains were tested in YPD without G418 and in YPD + G418 medium (Figures 1A,B). When the size of the inserted fragment was 0, the plasmid loss rates of plasmid p2μM in non-selective medium were 36.3 ± 6.0% for the 10th generation, 62.4 ± 3.3% for the 20th generation, 72.5 ± 7.9% for the 40th generation, and 85.7 ± 1.4% for the 50th generation, lower than those of the pRS423M plasmid (90.4 ± 2.9, 98.8 ± 0.9, 99.3 ± 0.2, and 99.9 ± 0.2%). Plasmid loss rates of p2μM in selective medium were 5.7 ± 1.3, 7.2 ± 0.7, 12.4 ± 0.8, and 27.1 ± 1.4% for each generation, which were much lower than those of pRS423M (17.8 ± 1.1, 31.4 ± 1.8, 74.8 ± 0.9, and 85.1 ± 2.2%).
Figure 1. Plasmid loss rates of the p2μM-based plasmids with different sizes of inserted fragments compared with that of pRS423M-based plasmids. (A) Plasmid loss rates of the p2μM-based plasmids and the pRS423M-based plasmids after cultivation in YPD medium. (B) Plasmid loss rates of the p2μM-based plasmids and the pRS423M-based plasmids after cultivation in YPD + G418 medium. (C) Plasmid loss rates of p2μM-based plasmids and pRS423M-based plasmids in YPD + G418 medium supplemented with G418 every 10 generations. (D) Plasmid loss rates of p2μM-based plasmids and pRS423M-based plasmids in YPD + G418 medium supplemented with G418 at the 38th generation.
Furthermore, three p2μM-based plasmids of the experimental group and three pRS423M-based plasmids of the control group mentioned above were transformed to strain CEN.PK2-1C, respectively. The results showed that the stabilities of p2μM-based plasmids were higher than those of pRS423M-based plasmids both in non-selective medium and selective medium (Figures 1A,B). For non-selective medium, when the sizes of the inserted fragments were 3,842 and 9,821 bp, the plasmid loss rates of p2μM-based plasmids were 54.3 ± 8.5 and 71.4 ± 5.6% (the 10th generation), 87.9 ± 2.4 and 95.8 ± 1.3% (the 20th generation), 91.9 ± 1.0 and 96.9 ± 0.8% (the 40th generation), and 96.4 ± 0.9 and 98.7 ± 0.3% (the 50th generation), while the plasmid loss rates of pRS423M-based plasmids were 98.1 ± 1.3 and 97.2 ± 1.1% for the 10th generation, and the plasmids were all lost at the 20th generation (99.7 ± 0.4 and 100.0%). Until the size of the inserted fragment increased to about 14 kb, the plasmid loss rate of the experimental group was 94.3 ± 1.2% for the 10th generation, but plasmids of the control group were almost all lost. For cultures that were grown in selective medium, when the fragment of 9,821 bp was introduced, 49.3 ± 2.5% strains lost their plasmid p2μM-medium-module, but almost all strains lost the plasmid pRS423M-medium-module after fermentation for 50 generations (94.9 ± 1.3%). All strains lost the plasmid pRS423M-large-module at the 40th generation (98.6 ± 1.2%); however, the plasmid loss rate of the p2μM-large-module was merely 57.0 ± 1.9%. The amounts of plasmid loss in YPD + G418 medium were less than those in YPD medium without G418.
As shown in Figure 1C, supplementing antibiotics to YPD + G418 medium every 10 generations could maintain lower plasmid loss rates. Plasmid loss rates of the 40th generation were greatly decreased after G418 was supplemented at the 38th generation (Figure 1D). The plasmid loss rates of the 40th generation were lower than those of the 20th generation, and the plasmid loss rates of p2μ-derived plasmids were still much lower than those of pRS423-derived plasmids.
To demonstrate that p2μM could be applied for the optimization of natural product biosynthesis, the tyrosol biosynthetic pathway was chosen as an example. The WT strain CEN.PK2-1C was fermented in YPD medium. Engineered strains containing individual p2μM-based plasmids and pRS423M-based plasmids with different sizes of tyrosol biosynthesis-related modules were simultaneously fermented in both non-selective medium and selective medium.
As demonstrated in Figure 2A, after fermentation in YPD medium, tyrosol productions of the WT strain were 45.11 ± 0.85 mg/L at the 20th generation and 48.53 ± 0.98 mg/L at the 40th generation. In non-selective YPD medium, strain CEN.PK2-1C with p2μM produced 39.39 ± 0.97 mg/L tyrosol after 20 generations and 44.78 ± 0.64 mg/L tyrosol after 40 generations (Figure 2B), which were lower than those of the WT strain. When the plasmid p2μM-small-module was transformed into the strain CEN.PK2-1C, the tyrosol production was 47.79 ± 0.64 mg/L at the 20th generation and 54.46 ± 0.21 mg/L at the 40th generation, 12.2% greater than that of the WT strain and 9.7% greater than that of the strain with pRS423M-small-module. The strain CEN.PK2-1C carrying plasmid p2μM-medium-module accumulated 50.59 ± 1.12 mg/L tyrosol after 40 generations of fermentation. In the strain CEN.PK2-1C with p2μM-large-module, the tyrosol titer of 48.03 ± 0.45 mg/L was obtained, which was not as good as the WT strain but 7.3% higher than that of CEN.PK2-1C carrying p2μM. CEN.PK2-1C carrying plasmid pRS423M produced 35.99 ± 0.35 mg/L tyrosol at the 20th generation and 43.41 ± 0.94 mg/L tyrosol at the 40th generation, which were lower than those of the strain with p2μM and the WT strain. Tyrosol productions in strain CEN.PK2-1C with pRS423M-medium-module and pRS423M-large-module at each generation were all much lower than those of the strains carrying p2μM -based plasmids.
Figure 2. Tyrosol production of strains containing a single plasmid with modules of different sizes after fermentation for 20 and 40 generations. (A) Tyrosol production of the WT strain CEN.PK2-1C. (B) Tyrosol production of strains CEN.PK2-1C with p2μM-based plasmids and CEN.PK2-1C with pRS423M-based plasmids after fermentation in YPD medium. (C) Tyrosol production of strains CEN.PK2-1C with p2μM-based plasmids and CEN.PK2-1C with pRS423M-based plasmids after fermentation in YPD medium with 200 μg/ml G418.
According to Figure 2C, after shake flask cultivation in YPD + G418 medium, the strain harboring p2μM generated tyrosol titer of 44.75 ± 0.83 mg/L at the 20th generation. At the 40th generation, tyrosol production was 49.05 ± 0.90 mg/L, which was higher than that of the WT strain and CEN.PK2-1C with p2μM fermented in non-selective medium; 71.11 ± 0.71 and 98.39 ± 0.41 mg/L tyrosol was produced in the strain containing p2μM-small-module after fermentation for 20 and 40 generations, respectively, which were much higher than that of CEN.PK2-1C with pRS423M-small-module (59.55 ± 0.16 mg/L). Tyrosol productions accumulated in the strain with p2μM-medium-module (47.71 ± 0.72 and 54.95 ± 0.50 mg/L) and p2μM-large-module (46.44 ± 0.65 and 50.20 ± 0.34 mg/L) after fermentation for 20 and 40 generations in selective medium were lower than those of the strain containing p2μM-small-module, but they were higher than those of CEN.PK2-1C with pRS423M-based plasmids. Strains carrying pRS423M produced 47.72 ± 0.18 mg/L tyrosol at the 40th generation, 2.8% lower than that of the strain with p2μM and 1.7% lower than that of the WT strain. The tyrosol yields of the strain containing plasmids pRS423M-small-module (59.55 ± 0.13 mg/L), pRS423M-medium-module (44.65 ± 1.46 mg/L), and pRS423M-large-module (25.64 ± 0.80 mg/L) at the 40th generation were all lower than those of the strains of p2μM-based plasmids with modules of the same size.
All results showed that the tyrosol yields of the strains with p2μM -based plasmids were higher than those of the strains with pRS423M-based plasmids both in non-selective medium and selective medium, which could be due to the instability of plasmid pRS423.
In this study, an endogenous 2μ-based expression vector with enhanced stability was developed in S. cerevisiae. The site between the RAF1 promoter and REP1 promoter on this plasmid was chosen as the insertion site for the gene of interest, which would not affect the functional elements and stability of the plasmid.
The plasmid loss rates were calculated on the strains harboring plasmids with inserted fragments of different sizes by culturing in non-selective YPD medium and YPD medium with selective pressure. After culturing without selective pressure for 40 generations, the loss rates of p2μM and pRS423M were about 73 and 100%, respectively. For plasmids containing modules of about 4 kb, the plasmid loss rates of p2μM-small-module and pRS423M-small-module in non-selective YPD medium were about 90 and 100%, respectively. All strains lost their plasmids by fermentation in YPD medium for 50 generations. Culturing in YPD + G418 medium for 50 generations, plasmid loss rate of p2μM was about 27% and that of pRS423M was about 85%. Plasmid pRS423M-large-module was all lost after 40 generations of cultivation, while merely 57% of the plasmid p2μM-large-module was lost. Continuous supplementation of G418 in YPD + G418 medium could help maintain the stability of plasmids, especially for p2μM-based plasmids. The plasmid loss rate of p2μM-large-module after 40 generations of cultivation was about 31%, which was much lower than that of pRS423M-large-module (about 82%). Although the selection pressure was conducive to the stable existence and inheritance of plasmids, a large number of pRS423M-based plasmids were lost during long-time fermentation. The results showed that the stabilities of the p2μM-based plasmids were higher than those of the pRS423M-based plasmids. It is estimated that an inserted fragment of 10 kb is acceptable for p2μM when there is no selection in the medium, and the inserted fragment of 14 kb is acceptable for p2μM under condition with selection. Zeng et al. (2021) moved the essential gene TPI1 from chromosome to p2μ plasmid. With auxotrophic complementation of TPI1, the resulting plasmid pE2μRT could undergo cultivation of 90 generations without loss under non-selective conditions.
Tyrosol biosynthetic pathway was introduced to demonstrate that the expression level of the p2μM-based plasmids was superior to that of the controls. After 40 generations of shake flask cultivation in YPD medium, the tyrosol yield of strain CEN.PK2-1C carrying plasmid p2μM-small-module was 54.46 ± 0.21 mg/L, about 9.7% higher than that of CEN.PK2-1C with pRS423M-small-module (49.64 ± 0.71 mg/L). The tyrosol titer of CEN.PK2-1C with p2μM-medium-module was 82.0% higher than that of strains carrying pRS423M-medium-module. The yield of tyrosol harvested from strains with p2μM-large-module was about threefold higher than that from strains with pRS423M-large-module. However, strains containing large module accumulated less tyrosol than strains containing small module and medium module, which was probably due to the instability of p2μM containing large module. Tyrosol production of the strain with p2μM-small-module at the 40th generation was 98.39 ± 0.41 mg/L with selective pressure, which was 80.7% greater than the strain with p2μM-small-module in non-selective medium and 65.2% higher than that of the strain with pRS423M-small-module in selective medium. The tyrosol yields of the strain containing plasmids pRS423M-medium-module and pRS423M-large-module at the 40th generation were all lower than those of the strains of p2μM-based plasmids with modules of the same size.
Taking these results into account, in order to improve the stability of endogenous 2μ-based expression vector in yeast, an essential gene could be introduced into the plasmid while knocking out the same essential gene in the genome to ensure the existence of engineered endogenous 2μ plasmid in yeast (Zeng et al., 2021). In the future, researchers could apply the CRISPR/Cas9 system to directly integrate metabolic pathways into the endogenous 2μ plasmid with an essential gene in vivo (Dean-Johnson and Henry, 1989; Zheng et al., 1993; Wang et al., 2020; Yang et al., 2021). In summary, our endogenous 2μ-based expression vector p2μM has improved stability than the commonly used YEp pRS423, so it could be applied in S. cerevisiae for genetic manipulations.
The original contributions presented in the study are included in the article/Supplementary Material, further inquiries can be directed to the corresponding author/s.
YT, JY, and YL conceived the study and carried out the molecular genetic studies as well as the strain construction. HL, YK, YZ, and YW participated in the design and coordination of the study. YT and JY performed the experiments and drafted the manuscript. YL supervised the whole research and revised the manuscript. All the authors read and approved the final manuscript.
We gratefully acknowledge the financial support from the Key-Area Research and Development Program of Guangdong Province (2020B0303070002), the National Natural Science Foundation of China (Grant No. 32071426), the National Key R&D Program of China (2018YFA0903300), and the Natural Science Foundation of Tianjin Province (19JCYBJC24200).
The authors declare that the research was conducted in the absence of any commercial or financial relationships that could be construed as a potential conflict of interest.
The Supplementary Material for this article can be found online at: https://www.frontiersin.org/articles/10.3389/fmicb.2021.679665/full#supplementary-material
Benedetto, R., Varì, R., Scazzocchio, B., Filesi, C., Santangelo, C., Giovannini, C., et al. (2007). Tyrosol, the major extra virgin olive oil compound, restored intracellular antioxidant defences in spite of its weak antioxidative effectiveness. Nutr. Metab. Cardiovasc. Dis. 17, 535–545. doi: 10.1016/j.numecd.2006.03.005
Bera, A. K., Ho, N. W., Khan, A., and Sedlak, M. (2011). A genetic overhaul of Saccharomyces cerevisiae 424A(LNH-ST) to improve xylose fermentation. J. Ind. Microbiol. Biotechnol. 38, 617–626. doi: 10.1007/s10295-010-0806-6
Boulet, A., Vest, K., Maynard, M., Gammon, M., Russell, A., Mathews, A., et al. (2017). The mammalian phosphate carrier SLC25A3 is a mitochondrial copper transporter required for cytochrome c oxidase biogenesis. J. Biol. Chem. 293:jbc.RA117.000265. doi: 10.1074/jbc.RA117.000265
Broach, J. R., and Hicks, J. B. (1980). Replication and recombination functions associated with the yeast plasmid, 2 mu circle. Cell 21, 501–508. doi: 10.1016/0092-8674(80)90487-0
Cao, M., Gao, M., Suastegui, M., Mei, Y., and Shao, Z. (2020). Building microbial factories for the production of aromatic amino acid pathway derivatives: from commodity chemicals to plant-sourced natural products. Metab. Eng. 58, 94–132. doi: 10.1016/j.ymben.2019.08.008
Cao, X., Yang, S., Cao, C., and Zhou, Y. J. (2020). Harnessing sub-organelle metabolism for biosynthesis of isoprenoids in yeast. Synth. Syst. Biotechnol. 5, 179–186. doi: 10.1016/j.synbio.2020.06.005
Chlebowicz-Śledziewska, E., and Śledziewski, A. Z. (1985). Construction of multicopy yeast plasmids with regulated centromere function. Gene 39, 25–31. doi: 10.1016/0378-1119(85)90103-9
Choe, K. I., Kwon, J. H., Park, K. H., Oh, M. H., Kim, M. H., Kim, H. H., et al. (2012). The antioxidant and anti-inflammatory effects of phenolic compounds isolated from the root of Rhodiola sachalinensis A. BOR. Molecules 17, 11484–11494. doi: 10.3390/molecules171011484
Christianson, T., Sikorski, R., Dante, M., Shero, J., and Hieter, P. (1992). Mulfunctional yeast high-copy-number shuttle vectors. Gene 110, 119–122. doi: 10.1016/0378-1119(92)90454-W
Dean-Johnson, M., and Henry, S. (1989). Biosynthesis of inositol in yeast. Primary structure of myo-inositol-1-phosphate synthase (EC 5.5.1.4) and functional analysis of its structural gene, the INO1 locus. J. Biol. Chem. 264, 1274–1283.
Gao, M., Cao, M., Suastegui, M., Walker, J., Rodriguez Quiroz, N., Wu, Y., et al. (2017). Innovating a Nonconventional Yeast Platform for Producing Shikimate as the Building Block of High-Value Aromatics. ACS Synth. Biol. 6, 29–38. doi: 10.1021/acssynbio.6b00132
Gerbaud, C., Fournier, P., Blanc, H., Aigle, M., Heslot, H., and Guerineau, M. (1979). High frequency of yeast transformation by plasmids carrying part or entire 2-micron yeast plasmid. Gene 5, 233–253. doi: 10.1016/0378-1119(79)90080-5
González, M., Brito, N., Hernández Bolaños, E., and Gonzalez, C. (2018). New tools for high-throughput expression of fungal secretory proteins in Saccharomyces cerevisiae and Pichia pastoris. Microb. Biotechnol. 12, 1139–1153. doi: 10.1111/1751-7915.13322
Hartley, J., and Donelson, J. (1980). Nucleotide sequence of the yeast plasmid. Nature 286, 860–865. doi: 10.1038/286860a0
Hohnholz, R., Pohlmann, K. J., and Achstetter, T. (2017). A set of isomeric episomal plasmids for systematic examination of mitotic stability in Saccharomyces cerevisiae. Yeast 34, 267–275. doi: 10.1002/yea.3231
Huang, M., Wang, G., Qin, J., Petranovic, D., and Nielsen, J. (2018). Engineering the protein secretory pathway of Saccharomyces cerevisiae enables improved protein production. Proc. Natl. Acad. Sci. U. S. A. 115, E11025–E11032. doi: 10.1073/pnas.1809921115
Jayaram, M., Li, Y. Y., and Broach, J. R. (1983). The yeast plasmid 2mu circle encodes components required for its high copy propagation. Cell 34, 95–104. doi: 10.1016/0092-8674(83)90139-3
Jensen, N. B., Strucko, T., Kildegaard, K. R., David, F., Maury, J., Mortensen, U. H., et al. (2014). EasyClone: method for iterative chromosomal integration of multiple genes in Saccharomyces cerevisiae. FEMS Yeast Res. 14, 238–248. doi: 10.1111/1567-1364.12118
Karković Marković, A., Torić, J., Barbarić, M., and Jakobusic Brala, C. (2019). Hydroxytyrosol, Tyrosol and Derivatives and Their Potential Effects on Human Health. Molecules 24:2001. doi: 10.3390/molecules24102001
Kikuchi, Y. (1983). Yeast plasmid requires a cis-acting locus and two plasmid proteins for its stable maintenance. Cell 35, 487–493. doi: 10.1016/0092-8674(83)90182-4
Kondo, H., Nakamura, Y., Dong, Y.-X., Nikawa, I., and Sueda, S. (2004). Pyridoxine biosynthesis in yeast: participation of ribose 5-phosphate ketol-isomerase. Biochem. J. 379, 65–70. doi: 10.1042/BJ20031268
Lee, D., Lloyd, N. D. R., Pretorius, I. S., and Borneman, A. R. (2016). Heterologous production of raspberry ketone in the wine yeast Saccharomyces cerevisiae via pathway engineering and synthetic enzyme fusion. Microb. Cell Fact. 15:49. doi: 10.1186/s12934-016-0446-2
Liu, H., Tian, Y., Zhou, Y., Kan, Y., Wu, T., Xiao, W., et al. (2020). Multi-modular engineering of Saccharomyces cerevisiae for high-titre production of tyrosol and salidroside. Microb. Biotechnol. doi: 10.1111/1751-7915.13667 [Epub Online ahead of print].
Liu, Q., Liu, Y., Chen, Y., and Nielsen, J. (2020). Current state of aromatics production using yeast: achievements and challenges. Curr. Opin. Biotechnol. 65, 65–74. doi: 10.1016/j.copbio.2020.01.008
Ludwig, D. L., and Bruschi, C. V. (1991). The 2-micron plasmid as a nonselectable, stable, high copy number yeast vector. Plasmid 25, 81–95. doi: 10.1016/0147-619x(91)90019-s
Luo, Y., Li, B. Z., Liu, D., Zhang, L., Chen, Y., Jia, B., et al. (2015). Engineered biosynthesis of natural products in heterologous hosts. Chem. Soc. Rev. 44, 5265–5290. doi: 10.1039/c5cs00025d
McQuaid, M. E., Polvi, E. J., and Dobson, M. J. (2019). DNA sequence elements required for partitioning competence of the Saccharomyces cerevisiae 2-micron plasmid STB locus. Nucleic Acids Res. 47, 716–728. doi: 10.1093/nar/gky1150
Misumi, Y., Nishioka, S., Fukuda, A., Uemura, T., Nakamura, M., Hoshida, H., et al. (2018). YHp as a highly stable, hyper-copy, hyper-expression plasmid constructed using a full 2-mum circle sequence in cir(0) strains of Saccharomyces cerevisiae. Yeast 36, 249–257. doi: 10.1002/yea.3371
Murray, A. W., and Szostak, J. W. (1983). Pedigree analysis of plasmid segregation in yeast. Cell 34, 961–970. doi: 10.1016/0092-8674(83)90553-6
Murray, J. A., and Cesareni, G. (1986). Functional analysis of the yeast plasmid partition locus STB. EMBO J. 5, 3391–3399. doi: 10.1002/j.1460-2075.1986.tb04655.x
Petes, T. D., and Williamson, D. H. (1975). Replicating circular DNA molecules in yeast. Cell 4, 249–253. doi: 10.1016/0092-8674(75)90172-5
Reider Apel, A., d’Espaux, L., Wehrs, M., Sachs, D., Li, R. A., Tong, G. J., et al. (2017). A Cas9-based toolkit to program gene expression in Saccharomyces cerevisiae. Nucleic Acids Res. 45, 496–508. doi: 10.1093/nar/gkw1023
Ren, Y., Liu, S., Jin, G., Yang, X., and Zhou, Y. J. (2020). Microbial production of limonene and its derivatives: achievements and perspectives. Biotechnol. Adv. 44:107628. doi: 10.1016/j.biotechadv.2020.107628
Rizvi, S. M. A., Prajapati, H. K., and Ghosh, S. K. (2018). The 2 micron plasmid: a selfish genetic element with an optimized survival strategy within Saccharomyces cerevisiae. Curr. Genet. 64, 25–42. doi: 10.1007/s00294-017-0719-2
Rizvi, S. M. A., Prajapati, H. K., Nag, P., and Ghosh, S. K. (2017). The 2-mum plasmid encoded protein Raf1 regulates both stability and copy number of the plasmid by blocking the formation of the Rep1-Rep2 repressor complex. Nucleic Acids Res. 45, 7167–7179. doi: 10.1093/nar/gkx316
Romanos, M. A., Scorer, C. A., and Clare, J. J. (1992). Foreign gene expression in yeast: a review. Yeast 8, 423–488. doi: 10.1002/yea.320080602
Sadowski, P. D. (1995). The Flp recombinase of the 2-microns plasmid of Saccharomyces cerevisiae. Prog. Nucleic Acid Res. Mol. Biol. 51, 53–91. doi: 10.1016/s0079-6603(08)60876-4
Stevens, B. J., and Moustacchi, E. (1971). Gamma satellite DNA and small twisted circular molecules in yeast Saccharomyces cerevisiae. Exp. Cell Res. 64, 259–266.
Suastegui, M., and Shao, Z. (2016). Yeast factories for the production of aromatic compounds: from building blocks to plant secondary metabolites. J. Ind. Microb. Biotechnol. 43, 1611–1624. doi: 10.1007/s10295-016-1824-9
Walfridsson, M., Hallborn, J., Penttilä, M., Keränen, S., and Hahn-Hägerdal, B. (1996). Xylose-metabolizing Saccharomyces cerevisiae strains overexpressing the TKL1 and TAL1 genes encoding the pentose phosphate pathway enzymes transketolase and transaldolase. Appl. Enviro. Microbiol. 61, 4184–4190. doi: 10.1128/AEM.61.12.4184-4190.1995
Wang, X., Chen, L., Liu, J., Sun, T., and Zhang, W. (2020). Light-Driven Biosynthesis of myo-Inositol Directly From CO2 in Synechocystis sp. PCC 6803. Front. Microbiol. 11:566117. doi: 10.3389/fmicb.2020.566117
Yang, Y., Hu, Y., Wu, L., Zhang, P., and Shang, J. (2021). dnm1 deletion blocks mitochondrial fragmentation in Deltafzo1 cells. Yeast 38, 197–205. doi: 10.1002/yea.3524
Zeng, B.-X., Yao, M.-D., Xiao, W.-H., Luo, Y.-Z., Wang, Y., and Yuan, Y.-J. (2021). Endogenous 2μ Plasmid Editing for Pathway Engineering in Saccharomyces cerevisiae. Front. Microbiol. 12:631462. doi: 10.3389/fmicb.2021.631462
Zhang, J., Chen, Y., Fu, L., Guo, E., Wang, B., Dai, L., et al. (2021). Accelerating strain engineering in biofuel research via build and test automation of synthetic biology. Curr. Opin. Biotechnol. 67, 88–98. doi: 10.1016/j.copbio.2021.01.010
Zhang, Y., Wang, J., Wang, Z., Zhang, Y., Shi, S., Nielsen, J., et al. (2019). A gRNA-tRNA array for CRISPR-Cas9 based rapid multiplexed genome editing in Saccharomyces cerevisiae. Nat. Commun. 10:1053. doi: 10.1038/s41467-019-09005-3
Keywords: 2μ plasmid, plasmid stability, tyrosol, pRS423, Saccharomyces cerevisiae
Citation: Yang J, Tian Y, Liu H, Kan Y, Zhou Y, Wang Y and Luo Y (2021) Harnessing the Endogenous 2μ Plasmid of Saccharomyces cerevisiae for Pathway Construction. Front. Microbiol. 12:679665. doi: 10.3389/fmicb.2021.679665
Received: 12 March 2021; Accepted: 18 May 2021;
Published: 18 June 2021.
Edited by:
Song Yang, Qingdao Agricultural University, ChinaCopyright © 2021 Yang, Tian, Liu, Kan, Zhou, Wang and Luo. This is an open-access article distributed under the terms of the Creative Commons Attribution License (CC BY). The use, distribution or reproduction in other forums is permitted, provided the original author(s) and the copyright owner(s) are credited and that the original publication in this journal is cited, in accordance with accepted academic practice. No use, distribution or reproduction is permitted which does not comply with these terms.
*Correspondence: Yunzi Luo, bHVveXVuemk4MjdAYWxpeXVuLmNvbQ==
†These authors have contributed equally to this work
Disclaimer: All claims expressed in this article are solely those of the authors and do not necessarily represent those of their affiliated organizations, or those of the publisher, the editors and the reviewers. Any product that may be evaluated in this article or claim that may be made by its manufacturer is not guaranteed or endorsed by the publisher.
Research integrity at Frontiers
Learn more about the work of our research integrity team to safeguard the quality of each article we publish.