- 1State Key Laboratory of Conservation and Utilization of Subtropical Agro-bioresources, Guangdong Laboratory of Lingnan Modern Agriculture, Guangdong Key Laboratory for Innovative Development and Utilization of Forest Plant Germplasm, College of Forestry and Landscape Architecture, South China Agricultural University, Guangzhou, China
- 2College of Forestry, Northwest A&F University, Yangling, China
- 3Key Laboratory of State Forestry and Grassland Administration on Forest Ecosystem Protection and Restoration of Poyang Lake Watershed, College of Forestry, Jiangxi Agricultural University, Nanchang, China
- 4School of Life Science, Shanxi Datong University, Datong, China
Plants and other organisms in the ecosystem compete for the limited nitrogen (N) in the soil. Formation of a symbiotic relationship with arbuscular mycorrhizal fungi (AMF) may influence plant competitiveness for N. However, the effects of AMF on plant nitrate (NO3–) uptake capacity remain unknown. In this study, a pot experiment was conducted to investigate the effects of N application and Rhizophagus irregularis inoculation on the root absorbing area, uptake kinetics of NO3–, and the expression of NO3– transporter (NRT) genes in Populus × canadensis ‘Neva’. The results showed that R. irregularis colonized more than 70% of the roots of the poplar and increased root active absorbing area/total absorbing area. The uptake kinetics of NO3– by poplar fitted the Michaelis–Menten equation. Mycorrhizal plants had a higher maximum uptake rate (Vmax) value than non-mycorrhizal plants, indicating that R. irregularis enhanced the NO3– uptake capacity of poplar. The expression of NRTs in roots, namely, NRT1;2, NRT2;4B, NRT2;4C, NRT3;1A, NRT3;1B, and NRT3;1C, was decreased by R. irregularis under conditions of 0 and 1 mM NH4NO3. This study demonstrated that the improved NO3– uptake capacity by R. irregularis was not achieved by up-regulating the expression of NRTs in roots. The mycorrhizal pathway might repress root direct pathway in the NO3– uptake by mycorrhizal plants.
Introduction
Nitrogen (N) is an essential component of nucleic acids, proteins, and many other critical biomolecules of living organisms (Castro-Rodríguez et al., 2017). Limited N in terrestrial ecosystems generally causes strong competition between different players, such as plants and/or soil microorganisms (Geisseler et al., 2010). Microorganisms are considered to be more competitive than plants in regard to N uptake due to their unique properties, such as faster growth rates and larger surface-area-to-volume ratios (Kuzyakov and Xu, 2013). In order to acquire enough N for growth, plants have evolved several N uptake strategies, including changing their root physiological characteristics and gene expression levels in N uptake systems, and establishing symbiosis with microorganisms (Kraiser et al., 2011).
Several different forms of N can be available to plant roots, such as ammonium (NH4+), nitrate (NO3–), and amino acids (Fan et al., 2017). Nitrate is usually the primary form of inorganic N absorbed by plants in aerobic soils (Xu et al., 2012). The NO3– uptake rate is mainly determined by NO3– uptake kinetics, which can be modeled by the Michaelis–Menten equation, which denotes the absorption rate of NO3– as a function of external NO3– concentration (McMurtrie and Näsholm, 2017). Two parameters of Michaelis–Menten kinetics, the maximum uptake rate (Vmax) and the Michaelis constant (Km), can describe N absorption characteristics (York et al., 2016). The Vmax value is the maximum influx rate of NO3–, whereas the Km value is the external NO3– concentration when the absorption rate is half of Vmax and presents the affinity of transporters (York et al., 2016).
Uptake of NO3– from soil is mediated by low- (LATS) and high-affinity transport systems (HATS) (Fan et al., 2017). The molecular basis of LATS and HATS mainly includes two families of NO3– transporters (NRT): NRT1/PTR (renamed NPF by Léran et al., 2014) family and NRT2 family (Castro-Rodríguez et al., 2017). The NPF family members are responsible for the LATS, except for NPF6;3/NRT1;1, which has a dual low and high affinity for NO3– (von Wittgenstein et al., 2014). Among the 53 known NPF family members in Arabidopsis, only AtNPF6;2/AtNRT1;1 and AtNPF4;6/AtNRT1;2 are involved in root NO3– influx (Kant, 2018). The NRT2 family has seven members and is responsible for the HATS (von Wittgenstein et al., 2014). The coding genes are induced by a low concentration of NO3– and repressed by a high concentration of NO3–. AtNRT2;1, AtNRT2;2, AtNRT2;4 and AtNRT2;5 have been found playing a role in root uptake of NO3– from soil (Kant, 2018). Of these, AtNRT2;1, in interaction with a NO3– assimilation-related protein (NAR2/NRT3), accounts for approximately 75% of the high-affinity NO3– absorption (Filleur et al., 2001).
Arbuscular mycorrhizal fungi (AMF), belonging to the monophyletic phylum Glomeromycota, are the most widespread root symbionts and can colonize at least 80% of terrestrial plants (Smith and Read, 2008). AMF acquire up to 20% of their host photosynthates and provide water and mineral nutrients in return (Bago et al., 2000; Kiers et al., 2011). Many 15N-labeling experiments confirmed the ability of AMF to transport N from soil to plants (Govindarajulu et al., 2005; Jin et al., 2005). Plants can acquire large amounts of N through the mycorrhizal pathway (Smith and Smith, 2011). The effects of AMF on the expression of NRT genes have been observed in several plant species, for example, in grapevine (Balestrini et al., 2017), Solanum lycopersicum (Ruzicka et al., 2012), and Lotus japonicus (Guether et al., 2009). The arbuscular mycorrhizal (AM)-induced alterations in NRTs expression suggest that AMF may affect plant uptake capacity for NO3–. However, the impacts of AMF on plant NO3– uptake kinetics are poorly understood.
Poplars have great ecological and economic values and can form symbiotic relationships with AMF (Wu et al., 2017). With the publication of the genome of Populus trichocarpa (Tuskan et al., 2006), poplar has become a model specie for woody plant research (Jansson and Douglas, 2007). In the genome database of P. trichocarpa, 68 NPF/NRT1 and 11 NRT2/NRT3 have been putatively identified (Willmann et al., 2014). The genes NRT1;1, NRT1;2, NRT2;4B, and NRT2;4C of poplar were the closest homologs to AtNRT1;1, AtNRT1;2, AtNRT2;4, and AtNRT2;1 of Arabidopsis, respectively; and NRT3;1A, NRT3;1B, and NRT3;1C of poplar were the closest homologs to AtNRT3;1 of Arabidopsis (Li et al., 2012; Luo et al., 2013). These genes may play important roles in NO3– uptake by poplar because their expression levels are markedly affected by changes in available N (Li et al., 2012; Gan et al., 2015). The objectives of the current research were to (i) evaluate the effects of AMF on NO3– uptake kinetics of poplar and (ii) investigate the influence of AMF on NRTs expression under different N levels. The results may provide new insights into the parts AMF play in plant N uptake capacity.
Materials and Methods
Plant Material and Fungal Inoculum
Cuttings (6 cm long) of Populus × canadensis ‘Neva’ were obtained from a plant nursery in Yangling, Shaanxi Province, China. The cuttings were surface sterilized with 75% ethanol and rinsed with sterile water. After surface sterilization, each cutting was planted in a plastic pot (10 × 8 cm) containing 450 g of growth substrate.
Rhizophagus irregularis (B109) was commercially provided by the Institute of Plant Nutrition and Resources, Beijing Academy of Agriculture and Forestry Sciences, China. The fungal inoculum contained colonized root fragments, hyphae, spores (approximately 50 spores g–1), and cultured sands.
Growth Substrates
The growth substrate consisted of topsoil (0–20 cm) collected from a poplar field in Yangling, Shaanxi Province, China. Before being put into the pots, the growth substrate was sieved (2 mm) and autoclaved (0.11 MPa, 121°C, 2 h). The soil contained soil organic matter, 18.97 mg kg–1; available N, 38.62 mg kg–1; available phosphorus, 12.31 mg kg–1; and available potassium, 140.26 mg kg–1. The pH value was 7.7 (1:5, soil:water).
Experimental Design and Growth Conditions
The experiment was conducted in a greenhouse using a randomized complete block design with two AM status (inoculated with R. irregularis or not inoculated with R. irregularis) and five N levels (0, 1, 5, 10, or 15 mM NH4NO3), with 30 replicates per treatment. For AM fungal inoculation, each pot received 20 g of inoculum, whereas the non-mycorrhizal treatment received 20 g of autoclaved inoculum and the filtrate (<20 μm) of the inoculum to provide a typical microbial population free of AM propagules (Wu et al., 2017). The cuttings were grown at a temperature of 25–30°C with 12 h of light per day. Ninety days after planting, N application started. NH4NO3 solution was applied to the corresponding treatments every other day. The N treatment lasted for 28 days. The pots were kept at field capacity during the experiment.
AM Colonization
The fresh roots (<2-mm diameter) were cut into 1- to 2-cm fragments and stained with trypan blue according to the method from Phillips and Hayman (1970). The proportion of root length colonized by AMF was estimated under a light microscope (×200 magnification; Olympus, Tokyo, Japan) using the gridline intersection method (McGonigle et al., 1990). The arbuscular, vesicular, hyphal, and total colonization values were expressed as a percentage of the length of root fragments containing arbuscules, vesicles, hyphae, and all AMF structures, respectively.
Root Absorbing Area
The root absorbing area was determined by a methylene blue colorimetric method (Liu et al., 2014). The roots were sequentially immersed for 90 s in three beakers containing a known volume of 0.2 mM methylene blue solution. Then, the concentration and volume of the remaining methylene blue solution in each beaker were determined by a spectrophotometer (UV mini 1240; Shimadzu, Kyoto, Japan) at 660 nm. Methylene blue was used as the standard. The equations are as follows:
Total absorbing area (m2) = [(C - C1) × V1] + [(C - C2) × V2] × 1.1
Active absorbing area (m2) = [(C - C3) × V3] × 1.1
where C (mg mL–1) was the initial concentration of the methylene blue solution; C1, C2, and C3 (mg mL–1) were the concentrations of the methylene blue solution after three root immersions; V1, V2, and V3 (mL) were the volumes of the methylene blue solution after three root immersions.
Determination of NO3– Uptake Kinetics
Plant NO3– uptake was measured using the solution depletion method (Gobert and Plassard, 2002) with some modifications. The roots of the intact plants were carefully washed and transferred to a 0.2 mM CaSO4 solution for pretreatment for 48 h. After pretreatment, the plants were transferred to a Ca(NO3)2 solution (containing 0, 0.01, 0.05, 0.1, 0.2, 0.5, or 1 mM NO3–) for 6 h. The NO3– concentration in the Ca(NO3)2 solution was determined by an AA3 continuous flow analytical system (AA3 autoanalyzer; Bran + Luebbe GmbH, Norderstedt, Germany) (Hu et al., 2016). The uptake rates were calculated as follows:
Uptake rates = (C1 - C2)/(t × m)
where C1 is the initial concentration of NO3– (μmol mL–1), C2 is the final concentration of NO3– (μmol mL–1), t is the absorption time (h), and m is the root dry weight (g). The kinetic parameters, Vmax and Km, were calculated using a non-linear curve-fitting model (Michaelis–Menten kinetics) using the Enzyme Kinetics Module of SigmaPlot 13.0 (SPSS Inc., Chicago, IL, United States).
Quantitative Real-Time PCR Analysis
At harvest, the root and leaf samples were immediately snap-frozen in liquid N and stored at −80°C. RNA was extracted using the cetyl trimethyl ammonium bromide method (Shao et al., 2014). A DNAse treatment was performed to remove residual DNA by using RNase-free DNase I (Invitrogen, Carlsbad, CA, United States). The quantity and quality of RNA were analyzed by measuring the A260/A280 ratio with a spectrophotometer (NanoDrop 2000c; Thermo Scientific, Pittsburgh, PA, United States). The first-strand cDNA was synthesized with 2 μg of total RNA, using a RevertAid first-strand cDNA Synthesis Kit (Thermo Fisher Scientific, Waltham, MA, United States). The gene-specific primers used are listed in Table 1. Quantitative real-time polymerase chain reaction (PCR) was performed on a Bio-Rad CFX96 real-time PCR instrument (Bio-Rad, Hercules, CA, United States). The reactions were performed in 20-μL volumes containing 10 μL of SYBR Premix Ex Taq II (TaKaRa, Dalian, China), 0.8 μL of gene-specific primers, 1.0 μL of cDNA template and 8.2 μL of sterile distilled water. The PCR amplification conditions were 94°C for 10 s and then 40 cycles at 58°C for 20 s and 72°C for 30 s. The ubiquitin gene (UBQ) was selected as an internal standard (Couturier et al., 2007). Four biological replicates were utilized for each assay. The relative RNA levels of each gene were determined using the 2–ΔΔCT method (Livak and Schmittgen, 2001).
Statistical Analyses
Experimental data were statistically analyzed using the statistical software package SPSS 13.0 (SPSS Inc., Chicago, IL, United States). The data were tested for normality and homogeneity of variances. A two-way analysis of variance (ANOVA) was used to analyze the effects of N treatment and AMF treatment and their interactions on the measured parameters, at a significance level of P = 0.05. The means were compared by Tukey’s multiple comparison test (P = 0.05). Figures were drawn using SigmaPlot 10.0 (Systat Software, San Jose, CA, United States).
Results
AM Colonization
AM colonization structures were observed in more than 70% of the poplar roots after inoculation with R. irregularis, whereas no AM structure was observed in NM plants (Figure 1). The hyphal colonization was prominent in mycorrhizal poplar roots (Figure 1C). With increasing N level, the arbuscular, hyphal, and total colonization rates increased substantially. The highest arbuscular and hyphal colonization rates (58.85 and 90.42%, respectively) were observed in plants treated with 15 mM NH4NO3.
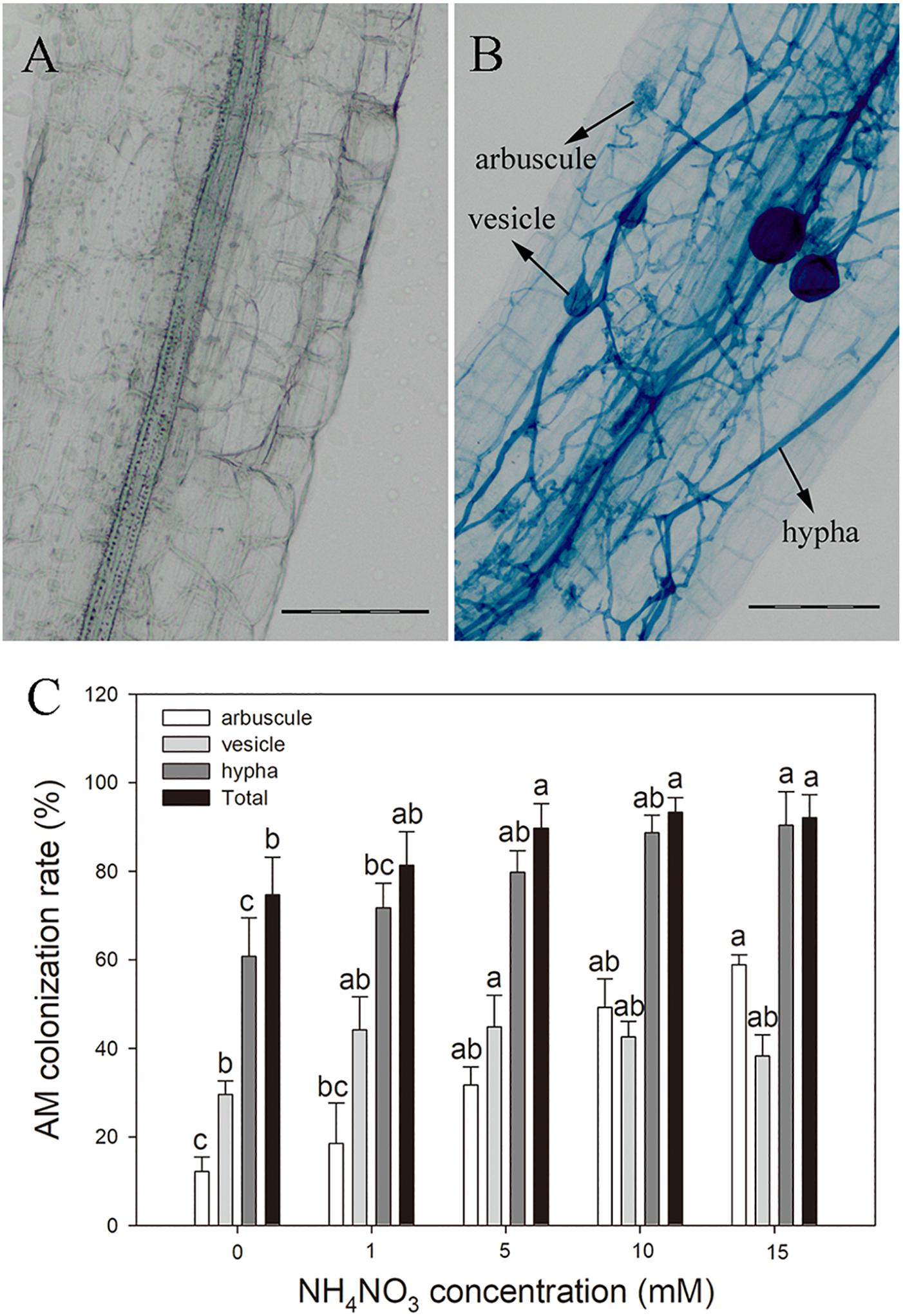
Figure 1. Arbuscular mycorrhizal fungi structures in non-inoculated roots (A) and Rhizophagus irregularis–inoculated roots of poplar (B) and the AM colonization rates under different nitrogen levels (C). Values are presented as means ± SD (n = 3). Means followed by the same letter do not differ significantly at P < 0.05 by Tukey’s test. Scale bar = 100 μm.
Root Absorbing Area
According to the two-way ANOVA, the total absorbing area was notably affected by N treatment and the interaction of N treatment and AMF treatment (Table 2). The active absorbing area was affected by N treatment, AMF treatment, and their interaction. The ratio of active absorbing area to total absorbing area was affected by AMF treatment. Nitrogen fertilization increased the total absorbing area and active absorbing area in both NM and AM plants (Table 3). The active absorbing area/total absorbing area of AM plants was higher than that of NM plants under each N level (3.47, 2.51, 2.73, 3.65, and 2.21% under 0, 1, 5, 10, and 15 mM NH4NO3, respectively).
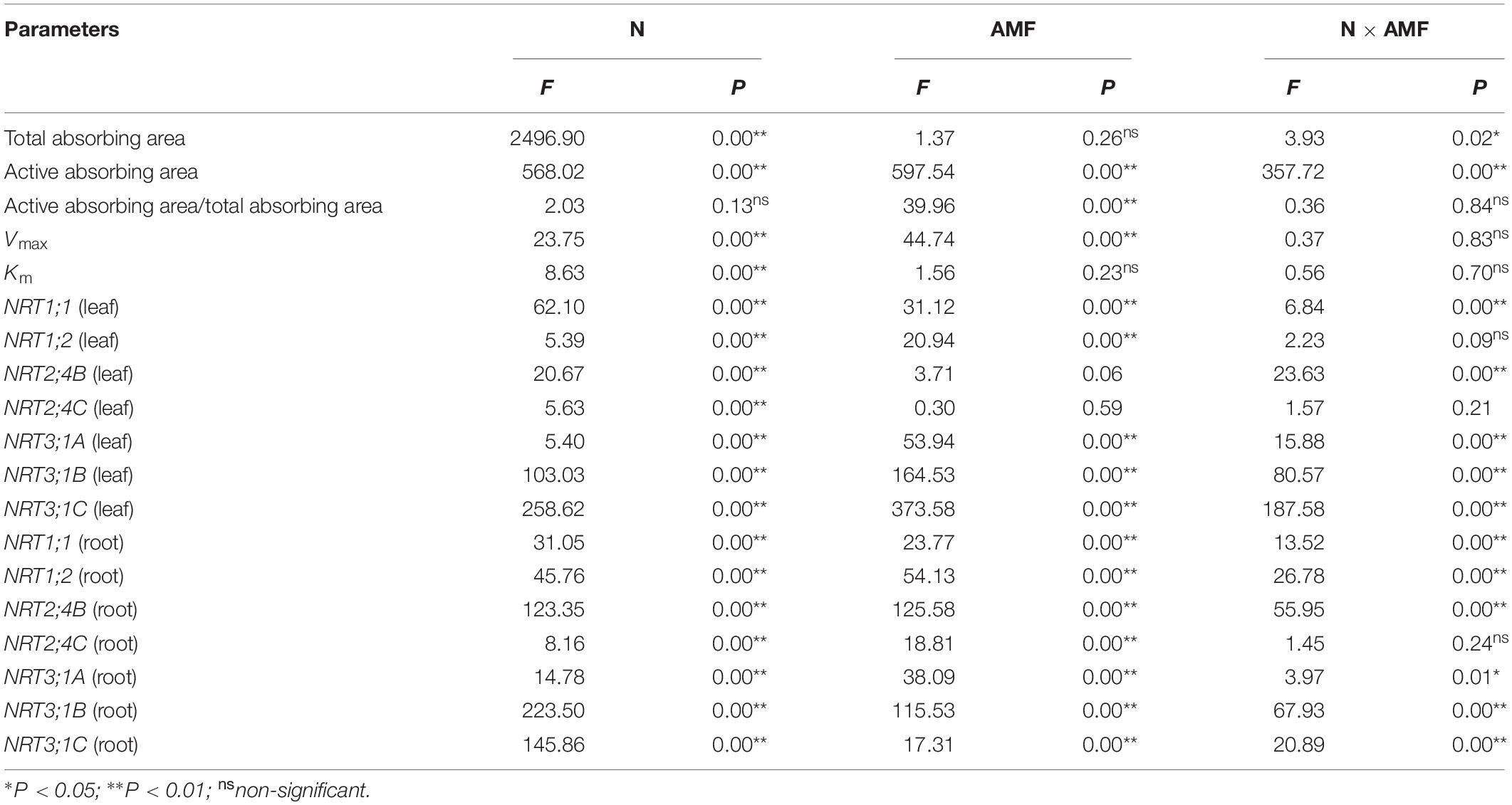
Table 2. Effects of nitrogen treatment (N), AMF treatment (AMF), and N × AMF on the physiological parameters and expression levels of nitrate transporter genes of poplar.
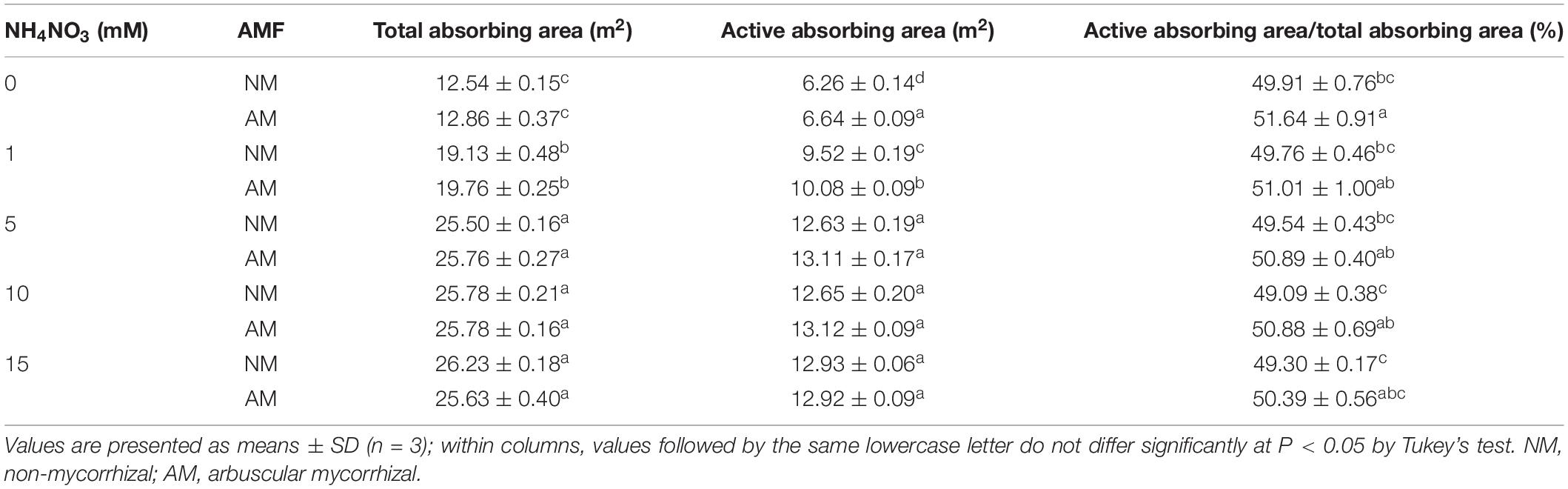
Table 3. Effects of N fertilization and AMF inoculation on root total absorbing area, active absorbing area, and active absorbing area/total absorbing area of poplar.
NO3– Uptake Kinetics
The NO3– uptake rates of both AM and NM plants increased with increasing external NO3– concentrations (Figure 2). The NO3– uptake rates were fitted using a non-linear regression of the Michaelis–Menten equation (V = Vmax/(Km + [NO3–]). According to the two-way ANOVA results, Vmax values were affected by N treatment and AMF treatment, whereas Km values were affected by N treatment (Table 2). The N × AMF interaction had no effect on the Vmax and Km values. Nitrogen decreased Vmax values but increased Km values (Table 4). Vmax values were higher in AM plants than in NM plants under each N level (46.83, 37.24, 67.07, 86.11, and 64.02% under 0, 1, 5, 10, and 15 mM NH4NO3, respectively). Km values were not significantly different between AM and NM plants.
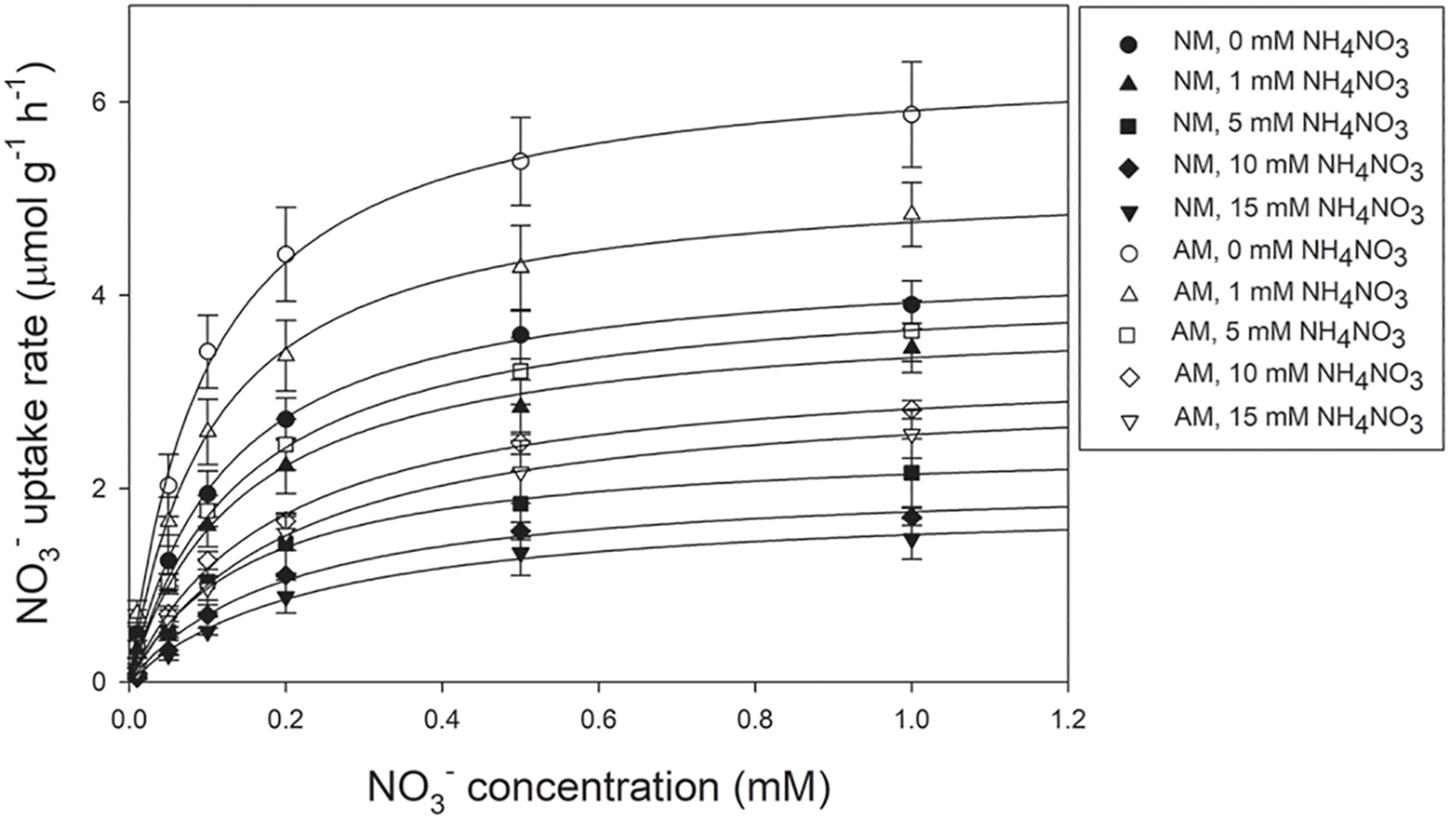
Figure 2. The NO3 – uptake rates of poplar as a function of NO3 – solution concentration. Values are presented as means ± SD (n = 3). NM, non-mycorrhizal; AM, arbuscular mycorrhizal.
NRTs Expression in Leaves and Roots
Nitrogen treatment significantly affected the expression of all the tested N transporter genes (Table 2). AMF treatment significantly affected the expression of NRT1;1, NRT1;2, NRT3;1A, NRT3;1B, and NRT3;1C in leaves and NRT1;1, NRT1;2, NRT2;4B, NRT2;4C, NRT3;1A, NRT3;1B, and NRT 3;1C in roots. The interaction of N treatment and AMF treatment significantly affected the expression of NRT1;1, NRT2;4B, NRT3;1A, NRT3;1B, and NRT 3;1C in leaves and NRT1;1, NRT1;2, NRT2;4B, NRT3;1A, NRT3;1B, and NRT3;1C in roots. In roots, the expression of NRT2;4B, NRT2;4C, NRT3;1A, NRT3;1B, and NRT3;1C in NM plants decreased with increasing N application (Figure 3). In leaves, the expression of NRT1;1 and NRT2;4B increased with increasing N application (Figure 4). AMF reduced the expression of NRT1;2, NRT2;4B, NRT2;4C, NRT3;1A, NRT3;1B, and NRT3;1C in roots (Figure 3) and up-regulated the expression of NRT2;4B in leaves under 0 and 1 mM NH4NO3 (Figure 4). Under 10 and 15 mM NH4NO3, AMF up-regulated the expression of NRT1;1 in roots and reduced the expression of NRT1;1, NRT1;2, and NRT2;4B in leaves. Correlation analysis showed that the expression levels of NRT3;1A, NRT3;1B, and NRT3;1C were positively correlated with the expression levels of NRT1;2, NRT2;4B, and NRT2;4C (Table 5).
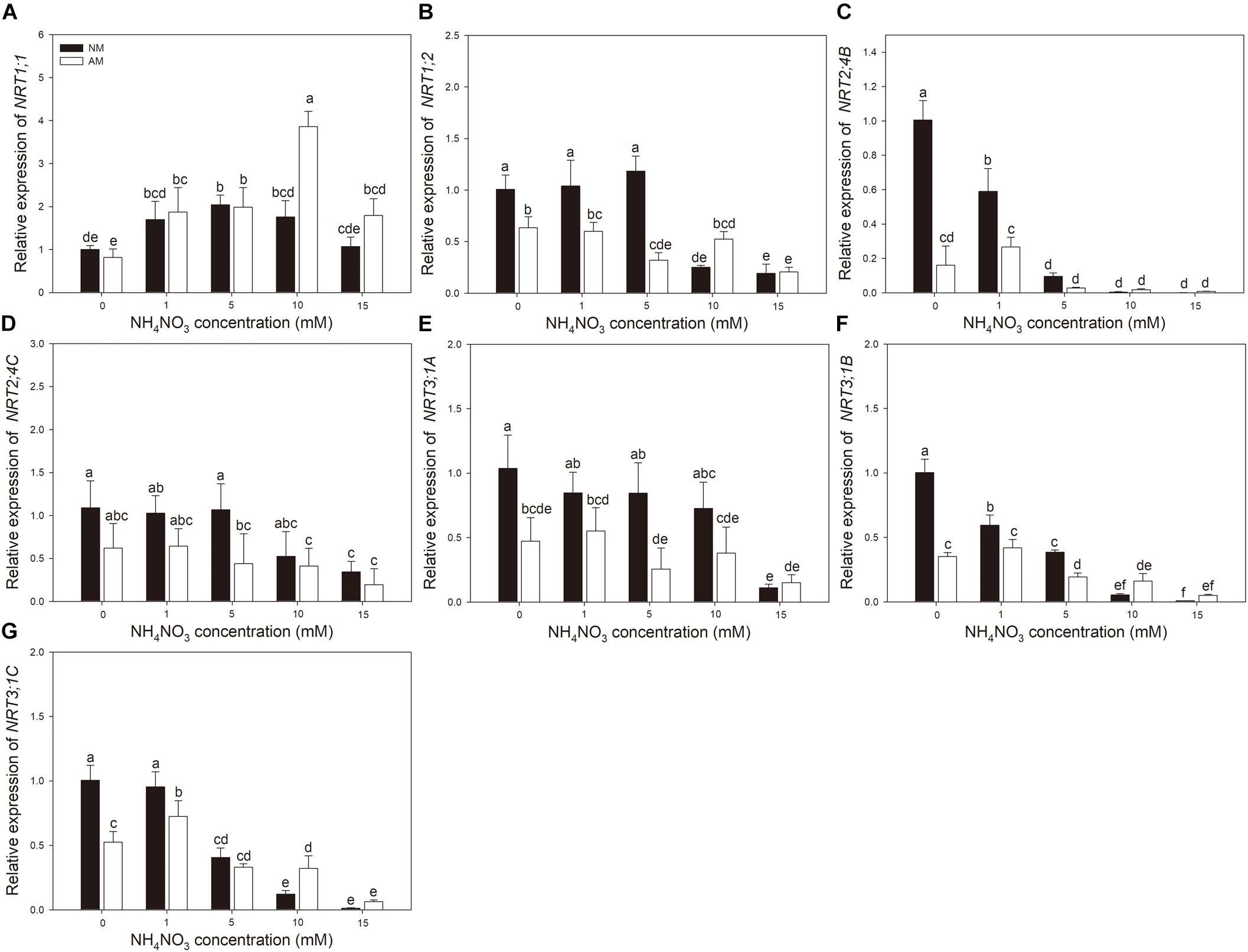
Figure 3. (A–G) NRT1;1, NRT1;2, NRT2;4B, NRT2;4C, NRT3;1A, NRT3;1B, and NRT3;1C expression levels in roots of mycorrhizal and non-mycorrhizal poplar in response to increasing nitrogen concentrations. Values are presented as means ± SD (n = 4). Means followed by the same letter do not differ significantly at P < 0.05 by Tukey’s test. NM, non-mycorrhizal; AM, arbuscular mycorrhizal.
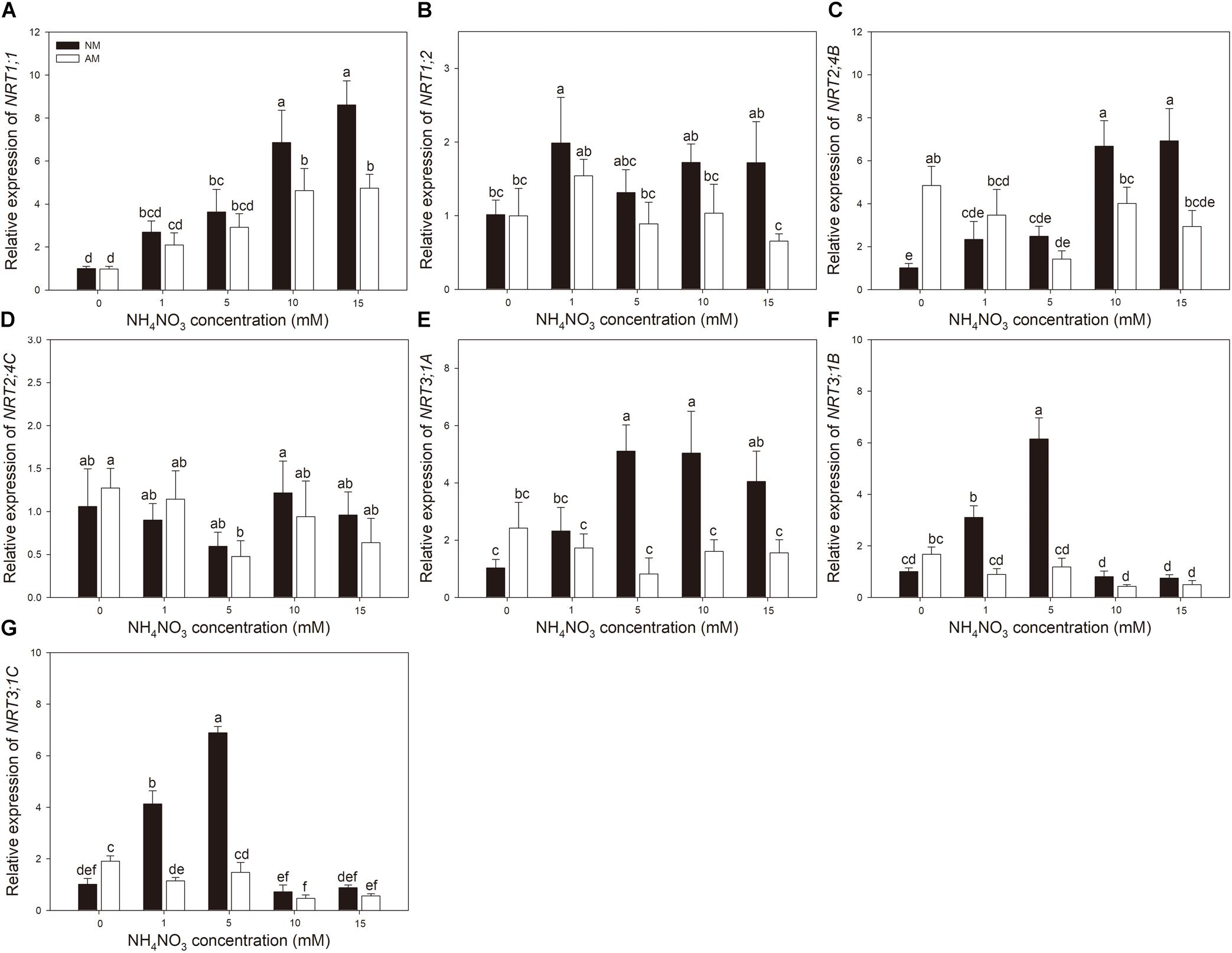
Figure 4. (A–G) NRT1;1, NRT1;2,NRT2;4B, NRT2;4C, NRT3;1A, NRT3;1B, and NRT3;1C expression levels in leaves of mycorrhizal and non-mycorrhizal poplar in response to increasing nitrogen concentrations. Values are presented as means ± SD (n = 4). Means followed by the same letter do not differ significantly at P < 0.05 by Tukey’s test. NM, non-mycorrhizal; AM, arbuscular mycorrhizal.
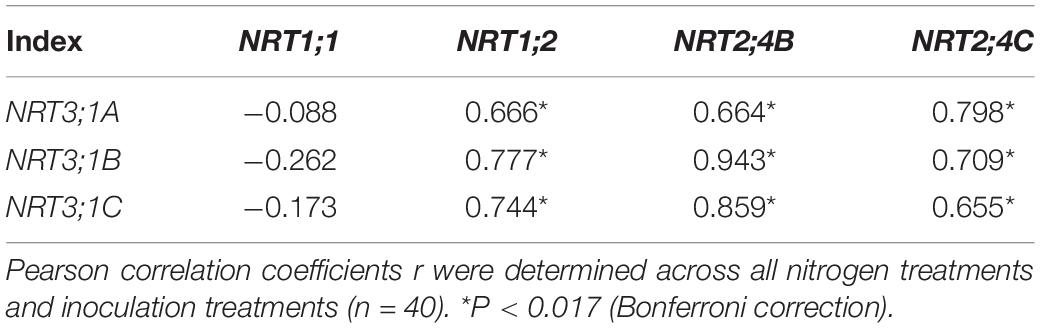
Table 5. Correlation coefficients between the expression levels of the nitrate transporter genes in roots of poplar.
Discussion
The formation of symbiosis is the starting point of AMF to improve plant nutrient uptake and stress resistance (Zhang et al., 2017). In this study, the structures of arbuscules, vesicles, and internal hyphae were clearly observed inside the roots of mycorrhizal poplar (Figure 1). More than 70% of the roots of the P. × canadensis ‘Neva’ cuttings were colonized by R. irregularis, which accords with previous findings that P. × canadensis ‘Neva’ was capable of establishing AM symbiosis (Liu et al., 2014). Nitrogen application influences AMF colonization rate, but inconsistent results have been reported (Calabrese et al., 2017; Wheeler et al., 2017). Using the same intersection method as this study, Wheeler et al. (2017) found a negative effect of chronic N application on the AMF colonization rate of Acer rubrum, whereas Calabrese et al. (2017) observed the opposite result in P. trichocarpa. The higher photosynthetic capacity in N-applied plants may provide more photosynthates for AMF and may promote AMF colonization (Wu et al., 2017).
The establishment of AM in roots can trigger the changes in root activities and gene expression patterns of root systems, for example, in grapevine (Balestrini et al., 2017; Torres et al., 2018), Robinia pseudoacacia (Huang et al., 2019), L. japonicus (Guether et al., 2009), and Lycopersicon esculentum (Hildebrandt et al., 2002). The results of this study showed that the active absorbing area/total absorbing area ratios of AM plants were higher than those in NM plants, which was in keeping with observations by Liu et al. (2014) and Huang et al. (2019). An increase in root activities suggests a stronger element uptake capacity (Yang et al., 2008). Uptake of NO3– by plants relies on NO3– transport systems (Garnett et al., 2010). In this study, the absorption of NO3– by P. × canadensis ‘Neva’ followed Michaelis–Menten kinetics, as with other plant species, for example, Solanum tuberosum (Sharifi and Zebarth, 2006), Fagus sylvatica (Kreuzwieser et al., 2000), Populus tremuloides Michx., and Pinus contorta Dougl. ex Loud. var. latifolia Engelm. (Min et al., 2000). Nitrogen application decreased the Vmax value and increased the Km value, which was consistent with previous studies showing that HATS was under feedback repression by N levels, and was down-regulated by N supply and up-regulated by N limitation (Bassirirad, 2000; Rothstein et al., 2000). Inoculation with R. irregularis increased the Vmax value of poplar in absorption of NO3–, irrespective of N treatment. So far, the effects of AMF on the kinetic analysis of NO3– uptake have never been reported. In ectomycorrhizal research, inoculation with Rhizopogon roseolus increased the Vmax of Pinus pinaster for NO3– absorption, indicating that ectomycorrhiza conferred plants with higher ability to utilize NO3– (Gobert and Plassard, 2002). Kuzyakov and Xu (2013) found a higher Vmax and smaller Km for NO3– in microorganisms than in plants, indicating that microorganisms have an advantage in the absorption of NO3–, especially at low concentrations. In general, microorganisms absorb the majority of N immediately after N is mineralized from soil organic matter (Kuzyakov and Xu, 2013). The higher NO3– uptake rates in AM plants than in NM plants were observed regardless of the external NO3– concentration, suggesting that AMF increased the competitive ability of the host to use fluctuating concentrations of NO3– in the soil solution (Gobert and Plassard, 2002; Kuzyakov and Xu, 2013).
Regulation of NO3– transporters is an essential factor for elucidating NO3– uptake and assimilation. Nitrate transport systems have been well identified in Arabidopsis (Kant, 2018). AtNPF6;2/AtNRT1;1 was found to be a dual-affinity NO3– transporter, participating in low- and high-affinity NO3– uptake and function as a NO3– sensor (Liu et al., 1999; Ho et al., 2009). AtNPF4;6/AtNRT1;2 was found to be a low-affinity NO3– transporter, and AtNPF4;6/AtNRT1;2 has been observed being constitutively expressed in root NO3– uptake (Huang et al., 1999). AtNRT2;1 is the main NO3– inducible member of the HATS, whereas NRT2;2 plays a minor role in NO3– absorption (Li et al., 2007). AtNRT2;5 has a similar role to AtNRT2;4, which plays a main role in NO3– uptake under N-starvation condition (Kiba et al., 2012; Lezhneva et al., 2014). Moreover, the interaction of NRT2 members with NAR2;1 (NRT3;1) is necessary to function in the NO3– transport capacity of NRT2 (Okamoto et al., 2006; Tsay et al., 2007). The results of this study showed that the expression levels of NRT2;4B, NRT2;4C, NRT3;1A, NRT3;1B, and NRT3;1C in the roots of poplar were negatively correlated with external NO3– concentrations, supporting the suggestion that NRT2 family members are high-affinity transporters (Li et al., 2012). The positive correlations between the expression levels of NRT3;1A, NRT3;1B, or NRT3;1C and the expression levels of NRT1;2, NRT2;4B, or NRT2;4C indicated that they might combine to function in NO3– absorption (Tsay et al., 2007). In leaves, the expression of NRT1;1 and NRT2;4B were up-regulated by N application, which may be due to a higher amount of N transferred to leaves with increasing N application. Under N-limiting condition, the transcript levels of NRTs in roots increased, and the expression of NRTs in leaves decreased, which may be a possible mechanism for poplar adaptation to an N-deficient environment (Luo et al., 2013).
Previous studies reported that AMF can influence NO3– uptake of plants by regulating the transcript levels of NRTs (Chen et al., 2018; Hildebrandt et al., 2002). With transcriptomics techniques, Balestrini et al. (2017) demonstrated that Funneliformis mosseae up-regulated the expression of two putative NO3– transporters in the roots of grapevine. In L. japonicus, four NRTs showed fourfold to 18-fold up-regulation by Gigaspora margarita colonization (Guether et al., 2009). Tian et al. (2017) found both up- and down-regulated expression of NRTs in the roots of Triticum aestivum by R. irregularis colonization. The differential responses of NRTs to AM colonization may be related to the different functions of NRTs. Some NRTs induced by AMF were expressed in arbuscule-containing cortical cells and may be involved in symbiosis development or N transfer through the periarbuscular membrane (Gaude et al., 2012). Hildebrandt et al. (2002) found that LeNRT2;3 was expressed in inner cortical cells of L. esculentum, in which arbuscules and vesicles were mainly distributed. The expression of LeNRT2;3 was higher in AMF-colonized roots than in non-colonized controls, which probably mediated the positive effects of AMF on NO3– uptake from soil and the distribution of NO3– to the host (Hildebrandt et al., 2002). In this research, the absorption capacity of poplar for NO3– was improved by R. irregularis, but no AM-specific NRT was detected. The expression levels of NRT1;2, NRT2;4B, NRT2;4C, NRT3;1A, NRT3;1B, and NRT3;1C were lower in R. irregularis–colonized poplar roots compared with non-colonized roots at 0 and 1 mM NH4NO3. The mycorrhizal plants have two N absorption pathways, the root direct pathway and the mycorrhizal pathway (Smith and Smith, 2011). Plants that form AM may use the mycorrhizal pathway to absorb NO3– and down-regulate the transcript levels of NRTs involved in the root direct pathway (Tian et al., 2017). Ruzicka et al. (2012) demonstrated that, under low inorganic N concentrations in the soil, the N uptake of mycorrhizal tomatoes shifted toward mycorrhizal-mediated N uptake over the root direct pathway. NRT1;2, NRT2;4B, NRT2;4C, NRT3;1A, NRT3;1B, and NRT3;1C may be involved in the root direct pathway and repressed in the presence of the mycorrhizal pathway (Ruzicka et al., 2012).
The diverse N sources and N conversion reactions lead to complex N pools in the field, of which organic N is the largest N pool (Kuypers et al., 2018). Most organisms prefer bioavailable N forms, such as NH4+ and NO3–, for which production is mainly controlled by microbial reactions (Kuypers et al., 2018). Nitrate is the main inorganic N form in aerobic soils (Xu et al., 2012). Compared with microorganisms, plants have shown a lower Vmax and higher Km for NO3–, which indicated a disadvantage in competing for NO3– (Kuzyakov and Xu, 2013). Previous studies demonstrated that AMF could change the microbial community that degrades organic matter and promote plant uptake of inorganic N produced during the degradation of organic matter (Hodge and Fitter, 2010; Nuccio et al., 2013). This study showed that AMF improved the ratio of root active absorbing area/total absorbing area and increased the Vmax value of the Michaelis–Menten equation for NO3– uptake of poplar, resulting in a promotion in NO3– uptake capacity. The key genes in NO3– uptake of poplar were repressed by AMF under 0 and 1 mM NH4NO3, indicating that the mycorrhizal pathway may have an impact on the root direct pathway for NO3– uptake. These results implied that the formation of AM symbiosis may be an efficient strategy for plants to compete with microorganisms for the finite available N in the soil. However, the NO3– uptake kinetics of R. irregularis remains unclear (Kuzyakov and Xu, 2013). Further studies are needed to investigate the NO3– uptake characteristics of AMF, in order to elucidate the mechanism of AMF in plant N uptake.
Data Availability Statement
All datasets generated for this study are included in the manuscript.
Author Contributions
FW, FF, and MT designed the experiments. FW, FF, NW, and LL performed the experiments. FW and FF analyzed the data. FW and MT wrote the manuscript.
Conflict of Interest
The authors declare that the research was conducted in the absence of any commercial or financial relationships that could be construed as a potential conflict of interest.
Funding
This study was supported by the National Natural Science Foundation of China (41671268, http://www.nsfc.gov.cn/), National Key Research and Development Program of China (2018YFD0600203) and Natural Science Foundation of Jiangxi Province, China (20192BAB214020).
Acknowledgments
The authors thank the referees and editors for their valuable advice.
References
Bago, B., Pfeffer, P. E., and Shachar-Hill, Y. (2000). Carbon metabolism and transport in arbuscular mycorrhizas. Plant Physiol. 124, 949–958. doi: 10.1104/pp.124.3.949
Balestrini, R., Salvioli, A., Molin, A. D., Novero, M., Gabelli, G., Paparelli, E., et al. (2017). Impact of an arbuscular mycorrhizal fungus versus a mixed microbial inoculum on the transcriptome reprogramming of grapevine roots. Mycorrhiza 27, 417–430. doi: 10.1007/s00572-016-0754-8
Bassirirad, H. (2000). Kinetics of nutrient uptake by roots: responses to global change. New Phytol. 147, 155–169. doi: 10.1046/j.1469-8137.2000.00682.x
Calabrese, S., Kohler, A., Niehl, A., Veneaultfourrey, C., Boller, T., and Courty, P. E. (2017). Transcriptome analysis of the Populus trichocarpa-Rhizophagus irregularis mycorrhizal symbiosis: regulation of plant and fungal transportomes under nitrogen starvation. Plant Cell Physiol. 58, 1003–1017. doi: 10.1093/pcp/pcx044
Castro-Rodríguez, V., Cañas, R. A., Fn, D. L. T., Pascual, M. B., Avila, C., and Cánovas, F. M. (2017). Molecular fundamentals of nitrogen uptake and transport in trees. J. Exp. Bot. 68, 2489–2500. doi: 10.1093/jxb/erx037
Chen, A., Gu, M., Wang, S., Chen, J., and Xu, G. (2018). Transport properties and regulatory roles of nitrogen in arbuscular mycorrhizal symbiosis. Semin. Cell Dev. Biol. 74, 80–88. doi: 10.1016/j.semcdb.2017.06.015
Couturier, J., Montanini, B., Martin, F., Brun, A., Blaudez, D., and Chalot, M. (2007). The expanded family of ammonium transporters in the perennial poplar plant. New Phytol. 174, 137–150. doi: 10.1111/j.1469-8137.2007.01992.x
Fan, X., Naz, M., Fan, X., Xuan, W., Miller, A. J., and Xu, G. (2017). Plant nitrate transporters: from gene function to application. J. Exp. Bot. 68, 2463–2475. doi: 10.1093/jxb/erx011
Filleur, S., Dorbe, M. F., Cerezo, M., Orsel, M., Granier, F., Gojon, A., et al. (2001). An Arabidopsis T-DNA mutant affected in Nrt2 genes is impaired in nitrate uptake. FEBS Lett. 489, 220–224. doi: 10.1016/S0014-5793(01)02096-8
Gan, H., Jiao, Y., Jia, J., Wang, X., Li, H., Shi, W., et al. (2015). Phosphorus and nitrogen physiology of two contrasting poplar genotypes when exposed to phosphorus and/or nitrogen starvation. Tree Physiol. 36, 22–38. doi: 10.1093/treephys/tpv093
Garnett, T., Conn, V., and Kaiser, B. N. (2010). Root based approaches to improving nitrogen use efficiency in plants. Plant Cell. Environ. 32, 1272–1283. doi: 10.1111/j.1365-3040.2009.02011.x
Gaude, N., Bortfeld, S., Duensing, N., Lohse, M., and Krajinski, F. (2012). Arbuscule-containing and non-colonized cortical cells of mycorrhizal roots undergo extensive and specific reprogramming during arbuscular mycorrhizal development. Plant J. 69, 510–528. doi: 10.1111/j.1365-313X.2011.04810.x
Geisseler, D., Horwath, W. R., Joergensen, R. G., and Ludwig, B. (2010). Pathways of nitrogen utilization by soil microorganisms–a review. Soil Biol. Biochem. 42, 2058–2067. doi: 10.1016/j.soilbio.2010.08.021
Gobert, A., and Plassard, C. (2002). Differential NO3- dependent patterns of NO3- uptake in Pinus pinaster, Rhizopogon roseolus and their ectomycorrhizal association. New Phytol. 154, 509–516. doi: 10.1046/j.1469-8137.2002.00378.x
Govindarajulu, M., Pfeffer, P. E., Jin, H., Abubaker, J., Douds, D. D., Allen, J. W., et al. (2005). Nitrogen transfer in the arbuscular mycorrhizal symbiosis. Nature 435, 819–823. doi: 10.1038/nature03610
Guether, M., Balestrini, R., Hannah, M., He, J., Udvardi, M. K., and Bonfante, P. (2009). Genome-wide reprogramming of regulatory networks, transport, cell wall and membrane biogenesis during arbuscular mycorrhizal symbiosis in Lotus japonicus. New Phytol. 182, 200–212. doi: 10.1111/j.1469-8137.2008.02725.x
Hildebrandt, U., Schmelzer, E., and Bothe, H. (2002). Expression of nitrate transporter genes in tomato colonized by an arbuscular mycorrhizal fungus. Physiol. Plant 115, 125–136. doi: 10.1034/j.1399-3054.2002.1150115.x
Ho, C. H., Lin, S. H., Hu, H. C., and Tsay, Y. F. (2009). CHL1 functions as a nitrate sensor in plants. Cell 138, 1184–1194. doi: 10.1016/j.cell.2009.07.004
Hodge, A., and Fitter, H. (2010). Substantial nitrogen acquisition by arbuscular mycorrhizal fungi from organic material has implications for N cycling. Proc. Natl. Acad. Sci. U.S.A. 107, 13754–13759. doi: 10.1073/pnas.1005874107
Hu, G., He, H., Zhang, W., Zhao, J., Cui, J., Li, B., et al. (2016). The transformation and renewal of soil amino acids induced by the availability of extraneous C and N. Soil Biol. Biochem. 96, 86–96. doi: 10.1016/j.soilbio.2016.01.019
Huang, L., Chen, D., Zhang, H., Song, Y., Chen, H., and Tang, M. (2019). Funneliformis mosseae enhances root development and Pb phytostabilization in Robinia pseudoacacia in Pb-contaminated soil. Front. Microbiol. 10:2591. doi: 10.3389/fmicb.2019.02591
Huang, N. C., Liu, K. H., Lo, H. J., and Tsay, Y. F. (1999). Cloning and functional characterization of an Arabidopsis nitrate transporter gene that encodes a constitutive component of low-affinity uptake. Plant Cell 11, 1381–1392. doi: 10.1105/tpc.11.8.1381
Jansson, S., and Douglas, C. J. (2007). Populus: a model system for plant biology. Annu. Rev. Plant Biol. 58, 435–458. doi: 10.1146/annurev.arplant.58.032806.103956
Jin, H., Pfeffer, P. E., Douds, D. D., Piotrowski, E., Lammers, P. J., and Shachar-Hill, Y. (2005). The uptake, metabolism, transport and transfer of nitrogen in an arbuscular mycorrhizal symbiosis. New Phytol. 168, 687–696. doi: 10.1111/j.1469-8137.2005.01536.x
Kant, S. (2018). Understanding nitrate uptake, signaling and remobilisation for improving plant nitrogen use efficiency. Semin. Cell Dev. Biol. 74, 89–96. doi: 10.1093/jxb/erq297
Kiba, T., Feria-Bourrellier, A. B., Lafouge, F., Lezhneva, L., Boutet-Mercey, S., Orsel, M., et al. (2012). The Arabidopsis nitrate transporter NRT2.4 plays a double role in roots and shoots of nitrogen-starved plants. Plant Cell 24, 245–258. doi: 10.1105/tpc.111.092221
Kiers, E. T., Duhamel, M., Beesetty, Y., Mensah, J. A., Franken, O., Verbruggen, E., et al. (2011). Reciprocal rewards stabilize cooperation in the mycorrhizal symbiosis. Science 333, 880–882. doi: 10.1126/science.1208473
Kraiser, T., Gras, D. E., Gutiérrez, A. G., González, B., and Gutiérrez, R. A. (2011). A holistic view of nitrogen acquisition in plants. J. Exp. Bot. 62, 1455–1466. doi: 10.1093/jxb/erq425
Kreuzwieser, J., Stulen, I., Wiersema, P., Vaalburg, W., and Rennenberg, H. (2000). Nitrate transport processes in Fagus-Laccaria-Mycorrhizae. Plant Soil 220, 107–117. doi: 10.1023/A:1004775230952
Kuypers, M., Marchant, H., and Kartal, B. (2018). The microbial nitrogen-cycling network. Nat. Rev. Microbiol. 16, 263–276. doi: 10.1038/nrmicro.2018.9
Kuzyakov, Y., and Xu, X. (2013). Competition between roots and microorganisms for nitrogen: mechanisms and ecological relevance. New Phytol. 198, 656–669. doi: 10.1111/nph.12235
Léran, S., Varala, K., Boyer, J. C., Chiurazzi, M., Crawford, N., Daniel-Vedele, F., et al. (2014). A unified nomenclature of NITRATE TRANSPORTER 1/PEPTIDE TRANSPORTER family members in plants. Trends Plant Sci. 19, 5–9. doi: 10.1016/j.tplants.2013.08.008
Lezhneva, L., Kiba, T., Feria-Bourrellier, A. B., Lafouge, F., Boutet−Mercey, S., and Zoufan, P. (2014). The Arabidopsis nitrate transporter NRT2.5 plays a role in nitrate acquisition and remobilization in nitrogen−starved plants. Plant J. 80, 230–241. doi: 10.1111/tpj.12626
Li, H., Li, M., Luo, J., Cao, X., Qu, L., Gai, Y., et al. (2012). N-fertilization has different effects on the growth, carbon and nitrogen physiology, and wood properties of slow- and fast-growing Populus species. J. Exp. Bot. 63, 6173–6185. doi: 10.1093/jxb/ers271
Li, W., Wang, Y., Okamoto, M., Crawford, N. M., Siddiqi, M. Y., and Glass, A. D. (2007). Dissection of the AtNRT2.1:AtNRT2.2 inducible high-affinity nitrate transporter gene cluster. Plant physiol. 143, 425–433. doi: 10.1104/pp.106.091223
Liu, K. H., Huang, C. Y., and Tsay, Y. F. (1999). CHL1 is a dual-affinity nitrate transporter of Arabidopsis involved in multiple phases of nitrate uptake. Plant Cell 11, 865–874. doi: 10.1105/tpc.11.5.865
Liu, T., Wang, C. Y., Chen, H., Fang, F. R., Zhu, X. Q., and Tang, M. (2014). Effects of arbuscular mycorrhizal colonization on the biomass and bioenergy production of Populus × canadensis ‘Neva’ in sterilized and unsterilized soil. Acta Physiol. Plant. 36, 871–880. doi: 10.1007/s11738-013-1465-9
Livak, K. J., and Schmittgen, T. D. (2001). Analysis of relative gene expression data using real-time quantitative PCR and the 2-ΔΔCT Method. Methods 25, 402–408. doi: 10.1006/meth.2001.1262
Luo, J., Li, H., Liu, T. X., Polle, A., Peng, C. H., and Luo, Z. B. (2013). Nitrogen metabolism of two contrasting poplar species during acclimation to limiting nitrogen availability. J. Exp. Bot. 64, 4207–4224. doi: 10.1093/jxb/ert234
McGonigle, T., Miller, M., Evans, D., Fairchild, G., and Swan, J. (1990). A new method which gives an objective measure of colonization of roots by vesicular-arbuscular mycorrhizal fungi. New Phytol. 115, 495–501. doi: 10.1111/j.1469-8137.1990.tb00476.x
McMurtrie, R. E., and Näsholm, T. (2017). Quantifying the contribution of mass flow to nitrogen acquisition by an individual plant root. New Phytol. 218, 119–130. doi: 10.1111/nph.14927
Min, X. J., Siddiqi, M. Y., Guy, R. D., Glass, A. D. M., and Kronzucker, H. J. (2000). A comparative kinetic analysis of nitrate and ammonium influx in two early-successional tree species of temperate and boreal forest ecosystems. Plant Cell Environ. 23, 321–328. doi: 10.1046/j.1365-3040.2000.00546.x
Nuccio, E. E., Hodge, A., Pett−Ridge, J., Herman, D. J., Weber, P. K., and Firestone, M. K. (2013). An arbuscular mycorrhizal fungus significantly modifies the soil bacterial community and nitrogen cycling during litter decomposition. Environ. Microbiol. 15, 1870–1881. doi: 10.1111/1462-2920.12081
Okamoto, M., Kumar, A., Li, W., Wang, Y., Siddiqi, M. Y., Crawford, N. M., et al. (2006). High-affinity nitrate transport in roots of Arabidopsis depends on expression of the NAR2-like gene AtNRT3.1. Plant Physiol. 140, 1036–1046. doi: 10.1104/pp.105.074385
Phillips, J., and Hayman, D. (1970). Improved procedures for clearing roots and staining parasitic and vesicular-arbuscular mycorrhizal fungi for rapid assessment of infection. Transact. Br. Mycol. Soc. 55, 158–161. doi: 10.1016/S0007-1536(70)80110-3
Rothstein, D. E., Zak, D. R., Pregitzer, K. S., and Curtis, P. S. (2000). Kinetics of nitrogen uptake by Populus tremuloides in relation to atmospheric CO2 and soil nitrogen availability. Tree Physiol. 20, 265–270. doi: 10.1093/treephys/20.4.265
Ruzicka, D. R., Hausmann, N. T., Barrios-Masias, R. H., Jackson, L. E., and Schachtman, D. P. (2012). Transcriptomic and metabolic responses of mycorrhizal roots to nitrogen patches under field conditions. Mycorrhiza 350, 145–162. doi: 10.1007/s11104-011-0890-z
Shao, Y., Qin, Y., Zou, Y., and Ma, F. (2014). Genome-wide identification and expression profiling of the SnRK2 gene family in Malus prunifolia. Gene 552, 87–97. doi: 10.1016/j.gene.2014.09.017
Sharifi, M., and Zebarth, B. J. (2006). Nitrate influx kinetic parameters of five potato cultivars during vegetative growth. Plant Soil 288, 91–99. doi: 10.1007/s11104-006-9092-5
Smith, S. E., and Smith, F. A. (2011). Roles of arbuscular mycorrhizas in plant nutrition and growth: new paradigms from cellular to ecosystem scales. Annu. Rev. Plant. Biol. 62, 227–250. doi: 10.1146/annurev-arplant-042110-103846
Tian, H., Yuan, X., Duan, J., Li, W., Zhai, B., and Gao, Y. (2017). Influence of nutrient signals and carbon allocation on the expression of phosphate and nitrogen transporter genes in winter wheat (Triticum aestivum L.) roots colonized by arbuscular mycorrhizal fungi. PLoS One 12:e0172154. doi: 10.1371/journal.pone.0172154
Torres, N., Antolín, M. C., and Goicoechea, N. (2018). Arbuscular mycorrhizal symbiosis as a promising resource for improving berry quality in grapevines under changing environments. Front. Plant Sci. 9:897. doi: 10.3389/fpls.2018.00897
Tsay, Y. F., Chiu, C. C., Tsai, C. B., Ho, C. H., and Hsu, P. K. (2007). Nitrate transporters and peptide transporters. FEBS Lett. 581, 2290–2300. doi: 10.1016/j.febslet.2007.04.047
Tuskan, G. A., Difazio, S., Jansson, S., Bohlmann, J., Grigoriev, I., Hellsten, U., et al. (2006). The genome of black cottonwood. Populus trichocarpa (Torr. & Gray). Science 313, 1596–1604. doi: 10.1126/science.1128691
von Wittgenstein, N. J., Le, C. H., Hawkins, B. J., and Ehlting, J. (2014). Evolutionary classification of ammonium, nitrate, and peptide transporters in land plants. BMC Evol. Biol. 14:11. doi: 10.1186/1471-2148-14-11
Wheeler, J. A., Frey, S. D., and Stinson, K. A. (2017). Tree seedling responses to multiple environmental stresses: interactive effects of soil warming, nitrogen fertilization, and plant invasion. Forest Ecol. Manag. 403, 44–51. doi: 10.1016/j.foreco.2017.08.010
Willmann, A., Thomfohrde, S., Haensch, R., and Nehls, U. (2014). The poplar NRT2 gene family of high affinity nitrate importers: impact of nitrogen nutrition and ectomycorrhiza formation. Environ. Exp. Bot. 108, 79–88. doi: 10.1016/j.envexpbot.2014.02.003
Wu, F., Zhang, H., Fang, F., Wu, N., Zhang, Y., and Tang, M. (2017). Effects of nitrogen and exogenous Rhizophagus irregularis on the nutrient status, photosynthesis and leaf anatomy of Populus × canadensis ‘Neva’. J. Plant Growth Regul. 36, 824–835. doi: 10.1007/s00344-017-9686-6
Xu, G., Fan, X., and Miller, A. J. (2012). Plant nitrogen assimilation and use efficiency. Annu. Rev. Plant Biol. 63, 153–182. doi: 10.1146/annurev-arplant-042811-105532
Yang, L., Wang, Y., Kobayashi, K., Zhu, J., Huang, J., Yang, H., et al. (2008). Seasonal changes in the effects of free−air CO2 enrichment (FACE) on growth, morphology and physiology of rice root at three levels of nitrogen fertilization. Global Change Biol. 14, 1844–1853. doi: 10.1111/j.1365-2486.2008.01624.x
York, L. M., Silberbush, M., and Lynch, J. P. (2016). Spatiotemporal variation of nitrate uptake kinetics within the maize (Zea mays. L) root system is associated with greater nitrate uptake and interactions with architectural phenes. J. Exp. Bot. 67, 3763–3775. doi: 10.1093/jxb/erw133
Keywords: arbuscular mycorrhiza, poplar, nitrate transporter gene, nitrate uptake kinetics, nitrogen fertilization
Citation: Wu F, Fang F, Wu N, Li L and Tang M (2020) Nitrate Transporter Gene Expression and Kinetics of Nitrate Uptake by Populus × canadensis ‘Neva’ in Relation to Arbuscular Mycorrhizal Fungi and Nitrogen Availability. Front. Microbiol. 11:176. doi: 10.3389/fmicb.2020.00176
Received: 27 July 2019; Accepted: 24 January 2020;
Published: 28 February 2020.
Edited by:
Andrea Genre, University of Turin, ItalyReviewed by:
Raffaella Balestrini, Institute for Sustainable Plant Protection (CNR), ItalyGuido Lingua, University of Eastern Piedmont, Italy
Copyright © 2020 Wu, Fang, Wu, Li and Tang. This is an open-access article distributed under the terms of the Creative Commons Attribution License (CC BY). The use, distribution or reproduction in other forums is permitted, provided the original author(s) and the copyright owner(s) are credited and that the original publication in this journal is cited, in accordance with accepted academic practice. No use, distribution or reproduction is permitted which does not comply with these terms.
*Correspondence: Ming Tang, tangmingyl@163.com
†These authors have contributed equally to this work