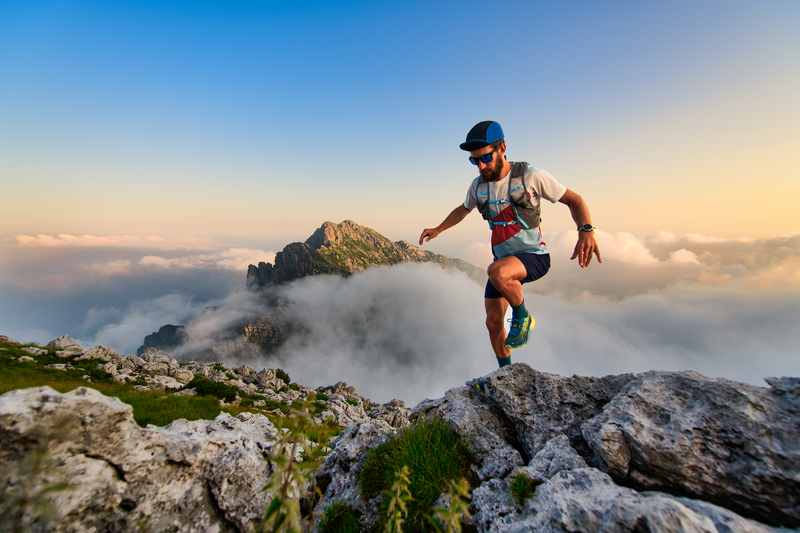
95% of researchers rate our articles as excellent or good
Learn more about the work of our research integrity team to safeguard the quality of each article we publish.
Find out more
REVIEW article
Front. Med. , 04 April 2025
Sec. Intensive Care Medicine and Anesthesiology
Volume 12 - 2025 | https://doi.org/10.3389/fmed.2025.1535673
This article is part of the Research Topic Exploring Endothelial Injury Syndromes: Mechanisms, Markers, and Therapeutic Potential View all 7 articles
Sepsis-induced acute kidney injury (S-AKI) is a common complication of sepsis. It occurs at high incidence and is associated with a high level of mortality in the intensive care unit (ICU). The pathophysiologic mechanisms underlying S-AKI are complex, and include renal vascular endothelial cell dysfunction. The endothelial glycocalyx (EG) is a polysaccharide/protein complex located on the cell membrane at the luminal surface of vascular endothelial cells that has anti-inflammatory, anti-thrombotic, and endothelial protective effects. Recent studies have shown that glycocalyx damage plays a causal role in S-AKI progression. In this review, we first describe the structure, location, and basic function of the EG. Second, we analyze the underlying mechanisms of EG degradation in sepsis and S-AKI. Finally, we provide a summary of the potential therapeutic strategies that target the EG.
Sepsis is a life-threatening condition caused by an uncontrolled response to infection (1). Sepsis-induced acute kidney injury (S-AKI) occurs within 7 days of the onset of sepsis, and is extremely common in the intensive care units (ICU), being present in approximately half of the patients with acute kidney injury (AKI) in this environment (2). An epidemiologic study performed in South Korea showed that from September 2019 to December 2022, 5,100 patients were admitted to the ICU with a diagnosis of sepsis, of whom 3,177 (62.3%) developed S-AKI. A total of 613 (19.3%), 721 (22.7%), and 1,843 (58.0%) patients had stage 1, stage 2, and stage 3 S-AKI, respectively. Severe S-AKI (stages 2 and 3 combined) was associated with a higher risk of in-hospital mortality (3); however, in general, the risks of chronic kidney disease, cardiovascular events, and death are much higher in patients with S-AKI (4–6).
The pathophysiologic mechanisms underpinning S-AKI are complex, and recent studies have shown that acute tubular necrosis, oxidative stress (7), mitochondrial dysfunction, inflammatory responses (8), microvascular dysfunction (9), and ischemia are involved (Figure 1).
Figure 1. S-AKI is associated with a complex array of mechanisms. The pathophysiologic mechanisms included acute tubular necrosis, oxidative stress, inflammatory responses, mitochondrial dysfunction, microvascular dysfunction, and ischemic. Sepsis-related injury factors cause the degradation of the glycocalyx, which leads to vascular endothelial dysfunction and is an important mechanism causing acute tubular necrosis during S-AKI. S-AKI, Sepsis-induced acute kidney injury.
Dysfunction of the vascular endothelial barrier is one of the most important mechanisms of S-AKI. The glycocalyx is an important component of the vascular endothelial barrier. It is complex and fragile, protects endothelial barrier integrity, and plays a crucial role in maintaining microcirculatory homeostasis and blood–tissue exchange (10). It is a gel-like polysaccharide/protein complex that is synthesized by endothelial cells and is rich in heparan sulfate (HS). It maintains vascular endothelial structure and function, protects the integrity of the vessel wall, inhibits thrombosis, and restricts leukocyte adherence to endothelial cells (11). Previous studies have shown that endothelial glycocalyx (EG) damage is closely associated with the development of S-AKI, and the specific structure and function of the glycocalyx and its role in S-AKI have been studied in recent years (12) (Figure 1).
The glycocalyx, also known as the polysaccharide envelope, is approximately 0.1–1.0 μm thick, as demonstrated through electron microscopy by Luft et al. in 1966 (13). Twamley et al. used immuno-electron microscopy and confocal laser scanning microscopy to study the glycocalyx of a human acute monocytic leukemia cell line, and found that its average length was 6.45 ± 0.26 μm (14). The thickness of the EG is within the range of 0.2–2 μm, and its composition and thickness vary according to the type of blood vessel, the location of the blood vessel within the organism, and the physiologic state (15). It lies between the blood and the vessel wall, is synthesized and secreted by vascular endothelial cells, and is a dynamic natural barrier that is principally composed of proteoglycans, membrane glycoproteins, and plasma proteins, of which proteoglycans and glycoproteins are the main components (16). Glycosaminoglycans are the most abundant constituent of proteoglycans, accounting for 95% of its structure (17). The principal components of glycosaminoglycans are HS, chondroitin sulfate, dermatan sulfate, keratin sulfate, and hyaluronic acid (HA), and the HS and chondroitin sulfate are important for vascular permeability (18–20). Glycoproteins are located on the surface of the endothelial cells and are covered by the EG under normal conditions. Glycoproteins include short, covalently-bound, branched oligosaccharides (21), which are principally E-selectin, P-selectin, intercellular adhesion molecule (ICAM), and vascular cell adhesion molecule (VCAM). Unlike the continuous capillaries in the heart (22) and the sinusoidal capillaries in the liver (23), the capillaries in the glomerulus are fenestrated, including pores that allow the penetration of small molecules but restrict the diffusion of proteins. The EG on the luminal surface of the glomerular capillaries reduces the size of these pores, almost blocking them (24).
The glycocalyx forms a barrier on the vascular endothelium and is a dynamic structure. The basic structure and function of the glycocalyx have been thoroughly studied, but some of the mechanisms involved have not been fully elucidated (25). Recent studies have shown that the glycocalyx plays an important role in the regulation of vascular permeability, cell–endothelial interactions (inflammation and coagulation) (26, 27), signaling, and molecular bioavailability (21). For example, fibroblast growth factor (FGF) signaling is entirely dependent on interactions between ligands, receptors, and the glycocalyx (28, 29). Furthermore, the binding of plasma-derived molecules to the glycocalyx can result in a local concentration gradient, which is a common feature of the transcriptional and developmental processes that are regulated by growth factors (30, 31).
In recent years, with the further development of medical technology, interest in the structure and function of the glycocalyx has been steadily increasing (32). The structure of the glycocalyx has become increasingly clear, and new technologies such as scanning probe microscopy have been used to better characterize the structure and function of the glycocalyx under physiologic conditions (33). The EG plays an important role in the maintenance of vascular homeostasis, and it is therefore a key player in a variety of systemic diseases (34).
The glycocalyx has been shown to be closely associated with various cardiovascular diseases. In aortic aneurysm, degradation products of the glycocalyx, syndecans, may serve as biomarkers and therapeutic targets (35). During acute myocardial infarction, the dysregulation of complement activation can lead to the degradation of the EG, causing endothelial dysfunction, and therefore this may provide new therapeutic targets (36). In coronary atherosclerosis, damage to the EG accelerates plaque formation (37). Furthermore, cardiac surgery can affect the function of the EG, predisposing toward disease progression (38). Thus, the glycocalyx has become a potential therapeutic target for cardiovascular disease (39).
Recent research has also demonstrated the role of the glycocalyx in respiratory diseases (40). For example, there is a complex interaction between coronavirus disease 2019 and the EG (41), and the glycocalyx plays a crucial role in the pathogenesis, progression, and complications of this disease (42, 43). In acute respiratory distress syndrome, the shedding of the epithelial glycocalyx contributes to the development and progression of lung injury, including excessive alveolar permeability, disruption of surfactant function, greater bacterial virulence, and impaired epithelial cell repair (44). The HS of the glycocalyx plays an important role in preserving endothelial barrier function and preventing the development of injury, acting in concert with tight junctions through signal transducer and activator of transcription 3 signaling (45).
The glycocalyx also plays important roles in other diseases. In patients with tumors, the glycocalyx, through extracellular vesicles, plays central roles in angiogenesis, the tumor microenvironment, and metastasis (46). The disruption of the EG has also been identified in diabetic nephropathy and retinopathy, and it plays a role in their development (47, 48). Moreover, the glycocalyx maintains the integrity of the blood–brain barrier and the vascular health of the central nervous system, influencing key processes such as blood flow regulation, inflammation, and vascular permeability (49).
Some common drugs have also been shown to affect the glycocalyx. For example, rivaroxaban protects the EG against damage caused by oxidative stress (50), doxycycline protects against sepsis-induced EG damage (51), and excessive aldosterone can cause EG damage (52).
Sepsis is a systemic disease, but the vascular endothelium is the first site that is exposed to bacterial endotoxin. As early as a few decades ago, it was shown that the glycocalyx covers the surface of most vascular lumens and that microcirculatory dysfunction and endothelial damage play important roles in the multiorgan failure associated with sepsis (53), and also that endothelial dysfunction and microcirculatory impairment are determinants of the severity and duration of sepsis (54).
Recent studies of the heart, lungs, and small blood vessels of the kidneys have shown that the EG is involved in some manifestations of endothelial dysfunction in sepsis (53, 55), and Song et al. have shown that measures taken to prevent glycocalyx degradation (subcutaneous injection of sulodexide (SDX), a highly purified patented product prepared from the porcine intestinal mucosa, consisting of 80% iduronylglycosaminoglycan sulfate, known as “fast-moving heparin,” and 20% dermatan sulfate) improves the survival of mice with sepsis (56). Furthermore, there may be a vicious circle involving endothelial cell dysfunction and glycocalyx damage. Zhang et al. found that endothelial cell dysfunction, which is characterized by low nitric oxide (NO) bioavailability, greater reactive oxygen species production, the activation of abscission enzymes, and impaired extracellular lysosome-associated organelle function, triggers the degradation of the EG (57), leading to shear stress defects. The glycocalyx, which covers endothelial cells, is the first component of the barrier to sense the mechanical signals associated with blood flow and transduce mechanical and biological signals to endothelial cells. During sepsis, changes in hemodynamics and shear stress can lead to glycocalyx damage and endothelial cell dysfunction. In turn, endothelial cell dysfunction affects the synthesis and secretion of the glycocalyx, thereby forming a vicious circle (58).
In general, there is greater catabolism of proteins, lipids, and carbohydrates in sepsis (59), but recent studies have shown that there is also greater polysaccharide synthesis in endothelial cells in the presence of sepsis. This is accompanied by loss of the glycocalyx and a 60% increase in permeability of the vascular endothelium, a change that is even more pronounced in patients who die as a result of sepsis, indirectly demonstrating that sepsis leads to the degradation of the glycocalyx and a compensatory increase in glycocalyx synthesis by endothelial cells (60). There is normally a feedback mechanism that regulates glycocalyx synthesis and degradation, but the rate of degradation is much higher than the rate of synthesis in the pathologic state. Enzymatic degradation of the glycocalyx is mediated by catabolic enzymes, catabolites, metalloproteinases, matrix metalloproteinases (MMPs), disintegrin and metalloproteinase domain-containing proteins (ADAMs), heparanase-1, hyaluronidase, and GPI-specific PLC AMs (61–63).
The expression of the gene encoding heparanase is regulated epigenetically and by tumor suppressor p53, but can also be induced by early growth response stimulation of transcription factor-1, reactive oxygen species, and inflammatory cytokines (64). Heparanase is also overexpressed in some malignant tumors and is activated in sepsis, causing partial degradation of the glycocalyx, which further exacerbates the loss of glycocalyx components (65). Schmidt et al. used heparinase inhibitors and a heparinase-deficient mouse model to demonstrate the pathogenic role of heparanase activation in sepsis-induced respiratory distress (53), and a similar phenomenon was identified in a model of ischemic acute kidney injury (66, 67).
HA has a wide variety of receptors, including cluster of differentiation 44 (CD44), HA-mediated motility receptor, lymphatic endothelial receptor-1, endocytosis-activated HA receptor, and Toll-like receptor 4, and is thus a potent signaling molecule with a range of effects that plays a crucial role in the development of sepsis (68). Under normal circumstances, the high-molecular weight form of HA predominates, and this maintains tissue stability and immune balance (69). When sepsis develops, inflammatory stimuli cause the degradation of high-molecular weight HA to the low-molecular weight form, and the accumulation of this form triggers an inflammatory response with the aim of eliminating pathogens (70). If low-molecular weight HA is produced in excessive amounts or cannot be cleared promptly an uncontrolled inflammatory response develops, which aggravates tissue damage. High-molecular weight HA can inhibit this inflammatory response to a certain extent by inhibiting the effects of the low-molecular weight form (71) through competition for receptors or interference with the associated signal transduction pathway (72). Thus, the balance between the two forms plays key roles in the development and outcome of sepsis. A disruption of this balance leads to the progression of the disease (73).
Sepsis has been shown to affect HA metabolism. The circulating concentrations of HA and HS are four-fold higher than normal in patients with sepsis and 29-fold higher in patients who survive for more than 90 days. Furthermore, the HA concentration correlates with the severity of renal and hepatic impairment (74). In rats with unilateral renal ischemia/reperfusion injury, the circulating HA concentration is high on day 1, and the excess largely comprises the high-molecular weight species (75). This phenomenon is associated with 35- to 50-fold higher hyaluronan synthase 1 mRNA expression in the outer and inner medulla of the kidney and a sustained increase in hyaluronan synthase 2 mRNA expression. However, the activities of hyaluronidase 1 and 2 are inhibited for 24 h following ischemia/reperfusion (76).
Lysosome-associated organelles also induce degradation of the glycocalyx. Lysosomes, as well as late endosomes and autophagosomes, have a thin layer of lysosomal glycocalyx on their inner surfaces (77) that protects the membranes from being digested by the hydrolytic enzymes they contain (78). It has been suggested that secreted lysosomes may contribute to the repair of the glycocalyx on cellular membranes during cytosolization (79), but the opposite has been identified in patients with sepsis (55). Lysosomes contain approximately 60 different soluble hydrolases that hydrolyze glycosaminoglycans, phospholipids, and a range of proteins, and cytosolization results in the release of a large number of stored substances from secreted lysosomes (80). Zullo et al. found that patchy degradation of the EG occurs after 10–15 min of exposure to lipopolysaccharide (LPS) and that this phenomenon is associated with an increase in histone B concentration in the culture medium, consistent with the export of lysosomal contents. Furthermore, when the binding of NO donors to lysosome-associated organelles is inhibited, this loss of EG is attenuated (55). Thus, lysosomes may also mediate early glycocalyx loss (Figure 2).
Figure 2. (a) Normal glycocalyx structure. (b) Mechanisms of sepsis-induced glycocalyx damage: shear stress, lysosome-related organelles, inflammatory response, oxidative stress, and some metallohydrolases. MMPs, matrix metalloproteinases; ADAMs, A Disintegrin And Metalloproteinase Domain-containing Proteins; IL-1β, Interleukin-1β; IL-6, Interleukin-6; IL-10, Interleukin-10; TNF-α, Tumor Necrosis Factor-α; CD44, Cluster of Differentiation 44; vWF, Von Willebrand Factor; SOD, Reactive Oxygen Species; ICAM-1, Intercellular Adhesion Molecule-1; VCAM-1, Vascular Cell Adhesion Molecule-1.
The EG layer lines the open fenestrae and covers the surface of the podocytes. The capillary EG covers 16.7% ± 1.8% of…. These structures are key to kidney function (15). Recent research has shown that an endothelial-specific glycocalyx component, endomucin (EMCN), is highly expressed in the glomerular endothelium and plays a key role supporting the normal structure and function of the glomerular filtration barrier by maintaining the tight junctions, homeostasis of the glomerular endothelium, and function of podocytes (81). The microvasculature of the kidney has similar functions to the microvascular systems of other organs, including the delivery of sufficient oxygen and nutrients to tissue cells, maintenance of the integrity of the endothelial monolayer, maintenance of the anticoagulant/procoagulant balance, and promotion of leukocyte recruitment (82). The vascular EG can determine vascular permeability, weaken the interaction between blood cells and the vessel wall, mediate shear stress sensing, achieve balanced signal transduction, and play a role in vascular protection. However, when it is disrupted, these properties are lost (16, 83). In the kidney, the control of vascular homeostasis and renal microvascular permeability is further regulated by the glycocalyx (84). The heterogeneity of the behavior of renal capillary endothelial cells is partly determined by the microenvironment in which the cells are located (85). Because sepsis rapidly changes the microenvironment and endothelial cells are one of the cell types that first sense the changes caused by sepsis and undergo extensive molecular adaptations (83), further research into the renal vascular EG could help physicians diagnose sepsis as early as possible, thereby providing the opportunity for early intervention.
More than half of patients in the ICU develop AKI, of those admitted because of sepsis, 62.3% develop S-AKI, and severe S-AKI is associated with a higher risk of death (3, 86). The systemic hypotension associated with sepsis involves reflex vasoconstriction, which leads to a decrease in renal blood perfusion, ultimately resulting in ischemia and hypoxia in the kidney (87). The subsequent pathophysiologic mechanisms can be summarized as acute tubular apoptosis, oxidative stress, inflammation, microcirculatory dysfunction, vascular barrier function disorder, local hypoxia, and tissue edema (84). Any of these factors, alone or in combination, can lead to AKI and damage to the vascular endothelium, including shedding of the glycocalyx. Damage to the tubular endothelial cells can increase leukocyte adhesion, platelet aggregation, and vasoconstriction, reduce blood flow to nephrons, and damage the glomerular barrier and the extensive network of peritubular capillaries (88). The glycocalyx is a negatively charged gel that covers endothelial cells and forms a molecular sieve, preventing the passage of macromolecules and protecting endothelial cells (89). Therefore, damage to the glycocalyx is a crucial promoter of S-AKI progression (90).
In all models of sepsis, loss of the EG rapidly leads to the accumulation of high circulating concentrations of soluble glycocalyx components (91). In mice, the plasma concentration of syndecan-1 begins to increase within 6 h of high-dose LPS (20 mg/kg) administration and peaks after approximately 24 h (92). Electron microscopic analysis has shown that 48 h after LPS treatment, the glycocalyx of glomerular endothelial cells and podocytes begins to disappear, and endothelial fenestrations are lost or edematous, such that a gap forms between the podocytes and the basement membrane (15). In addition, heparanase expression is high, principally in the glomerulus, 24–48 h after LPS treatment, which leads to the glomerular loss of HS and glycosaminoglycans, and is associated with high blood urea nitrogen (BUN), high urinary albumin/creatinine ratio, and glomerular injury (93, 94). Furthermore, in rats that are subjected to cecum ligation and puncture (CLP) induced-AKI, early glomerular syndecan-1 loss is followed by the loss of hyaluronic acid 7 h after CLP is initiated, and this is accompanied by alterations in glomerular sialic acids and the molecular composition of the glomerular filtration barrier (95). Although the circulating creatinine concentration and creatinine clearance are normal, the urinary albumin/creatinine ratio of such rats is high, suggesting a loss of the glomerular filtration barrier, rather than a loss of renal function. Furthermore, in mice subjected to CLP, the expression of active heparanase increases within the first 4 h of AKI, most markedly in the glomerulus and small periglomerular arteries (96).
In pathologic conditions, the loss of HS disrupts the charge selectivity of the glomerular filtration barrier, which increases the filtration of proteins and leads to proteinuria (97). This increases the reabsorption burden of the renal tubules, resulting in impaired excretion of renal metabolic waste and contributing to the increase in BUN. However, this increase in BUN is also affected by other factors: the concomitant loss of HS at least in part explains the increase in BUN and fully explains the CLP-induced decrease in glomerular filtration rate (GFR) (98). CLP-induced sepsis leads to the degradation of HS in the endothelial glycocalyx (99). Hemodynamically, the loss of HS impairs its mechanotransduction function, resulting in abnormal regulation of vascular tone and lower renal perfusion; and in terms of vascular barrier function, the loss of HS exposes adhesion molecules, promoting the adhesion of leukocytes and platelets, triggering inflammation and thrombosis, and further impairing renal blood supply and glomerular filtration, ultimately leading to a decrease in GFR (100). Thus, in CLP-induced sepsis, the loss of HS is the key factor causing the decrease in GFR and can fully explain this effect. The increase in renal microvascular permeability becomes evident 8–24 h after CLP, which is a delayed response compared with the early increases in the systemic concentrations of glycocalyx components (101). The structure of the glycocalyx is gradually degraded, and therefore it retains some of its barrier function for a time, thereby restricting the increase in microvascular permeability. Furthermore, the intervals between an inflammatory stimulus, the massive generation and complete activation of lytic enzymes, and the accumulation of glycocalyx degradation products, which causes changes in microvascular permeability that can be relatively prolonged (102). There are multiple compensatory mechanisms for glycocalyx damage, such as an increase in the expression of tight junction proteins and changes in cytoskeletal structure, which help maintain the normal function of microvessels (103) and further delay the effects.
The effects of the sepsis-associated loss of the vascular EG on renal leukocyte recruitment are less clear than those on permeability. In the presence of LPS, the lung capillaries of mice rapidly lose HS, leading to greater neutrophil recruitment (53). However, in the kidney, 48 h after LPS administration, monocyte and macrophage accumulation is dependent on the glycocalyx, whereas neutrophil endocytosis in the glomerulus is not (93). In mice with CLP-induced sepsis, no relationship was identified of the loss of HS in glomeruli with small periglomerular arteriolar microvessel function and neutrophil endocytosis (96). However, in antiglomerular basement membrane glomerulonephritis, neutrophil recruitment to the glomerulus is dependent on the loss of HS (104). These data suggest that in S-AKI, the glomerular EG restricts immune cell recruitment. Findings made in other organs cannot be fully extrapolated to the kidney, and therefore the role of the glycocalyx in inflammation and leukocyte recruitment in S-AKI requires further investigation (105).
The EG also plays important roles in renal microvascular homeostasis. We evaluated the role of heparanase using two experimental models of glomerulonephritis, in wild-type and heparan-deficient mice, and found that normal glomerular levels of HS are necessary for the maintenance of normal renal function (93). HS is an important component of the glomerular filtration barrier (106), and in experimental diabetic nephropathy, low expression of HS leads to impaired function of the glomerular filtration barrier, greater protein filtration, and therefore an impairment in renal function (107). Therefore, normal glomerular levels of HS are necessary for renal function in general and the control of glomerular permeability in particular (93, 108), and HS in small arterioles is necessary for the appropriate control of GFR (96) because it helps maintain the normal structure and function of blood vessels. Hemodynamically, as a major component of the EG, HS converts fluid shear stress into signals, regulates vascular tone, and ensures the normal contraction and relaxation of arterioles (106). During sepsis, HS is degraded, leading to abnormal regulation of vascular tone, which affects renal blood perfusion and GFR (109). When HS is damaged, the adhesion of leukocytes and platelets increases, which may lead to thrombosis and inflammation in arterioles, affect the blood supply to the kidneys, and impair GFR. Furthermore, appropriate glomerular levels of syndecan and HS are necessary for normal glomerular filtration barrier function (95). In S-AKI, the microvascular levels of these glycocalyx components change in a heterogeneous manner, and along with changes in other structural components of the endothelial surface layer, these underlie the loss of microvascular function (85). A recent study has shown that the absence of EMCN disrupts endothelial homeostasis. Along with infiltration by inflammatory cells, it also causes dysfunction of the glomerular filtration barrier, and therefore albuminuria (81). These findings reveal the crucial role of the glycocalyx molecule EMCN in the permeability of renal blood vessels and the integrity of the glomerular filtration barrier; and provide more compelling evidence for the relationship between S-AKI and the glycocalyx.
Endothelial dysfunction and glycocalyx damage have become a focus of sepsis research, and markers of glycocalyx degradation are being identified. This is evidenced by a recently published bibliometric analysis that counted articles regarding endothelial dysfunction in sepsis that were published between 2003 and 2023, which showed a rapidly increasing number. Key areas of future research will include the signaling pathways and molecular mechanisms involved, endothelial repair, and interactions between endothelial cells and other cell types in sepsis (110). In addition, Nelson et al. have shown that patients with septic shock who are admitted to the ICU (N = 18) have a significantly higher median expression of syndecan-1 than healthy individuals (111). Furthermore, Schmidt et al. measured the urinary HS concentration, which may reflect renal glycocalyx degradation, and found that HS was the only glycosaminoglycan that was significantly associated with mortality (hyaluronic acid and chondroitin sulfate were not) and that patients who died of sepsis had significantly higher mean HA concentrations at the start of the study than survivors (112).
Currently, the fundamental problem regarding the treatment or prevention of S-AKI is that it is typically diagnosed late. The diagnostic criteria for AKI are based on changes in urine output and serum creatinine concentration (2). However, urine output is not a very reliable index in patients who are taking medication or are perioperative, and other commonly used surrogate indices, such as the serum creatinine concentration, creatinine clearance, or GFR, can only show that AKI and functional impairment is already present, rather than being predictors of their development at an early stage (113). By contrast, the troponin concentration can be used to identify cardiac conditions before dysfunction develops (114). Therefore, the identification of a high troponin concentration permits earlier treatment and prevention, thereby halving the mortality rate associated with non-ST-segment elevation-associated myocardial infarction (115). Thus, there is an urgent need to identify a marker of structural damage to the kidney before functional impairment and the vicious cycle of inflammation, microcirculatory disturbances, and local ischemia are established (116).
Several biomarkers that have the potential to overcome the limitations of the use of creatinine concentration have been identified (91). For example, cystatin C is suitable for the detection of renal impairment in patients with GFRs in the “creatinine-blind” range, and it is not significantly influenced by muscle mass, age, or ethnicity (117). The cystatin C concentration begins to rise 24–48 h after AKI, and this response is much faster than that of creatinine, the concentration of which increases 2–7 days after AKI, depending on the extent of the pre-existing kidney damage (118). Another new biomarker is neutrophil gelatinase-associated lipocalcin (NGAL), which shows changes in concentration that are comparable to those of troponin, with a peak 6 h after the onset of AKI. This is a reliable predictor of AKI in critically ill patients, but the utility of NGAL for the diagnosis of sepsis is more questionable because it is partly derived from neutrophils (119). Cut-off values have been established for these biomarkers, but although they may help physicians identify the most appropriate time to start treatment for kidney damage, no corresponding treatment exists (84).
The findings of studies of the tubular EG may be able to compensate for these diagnostic and therapeutic deficiencies. Previous studies have identified several predictors of glycocalyx injury or degradation in patients with sepsis, including high plasma HS and heparanase concentrations in children (20) and high plasma HA and syndecan-1 concentrations in adults (120). These glycocalyx components are released into the bloodstream and may represent useful markers for the prediction of sepsis and even S-AKI. Some preliminary studies have demonstrated that high concentrations of syndecan-1, CD44, and glycosaminoglycans are associated with microvascular injury in resuscitated patients with septic shock (91, 121). However, the relationships between these markers and S-AKI have yet to be fully characterized. One study has shown that in patients with septic shock, the plasma concentrations of syndecan-1 and VE-cadherin increase significantly within 7 days (122). In addition, in patients with organ failure, the syndecan-1 and VE-cadherin concentrations increase, whereas that of sphingosine 1-phosphate (S1P) decreases. The syndecan-1 and VE-cadherin concentrations are independently associated with the need for renal replacement therapy during hospitalization in the ICU, but only syndecan-1 is predictive of its development (122). A few previous studies have shown associations between these markers and S-AKI. Saoraya et al. showed that in the emergency department, the circulating concentration of syndecan-1, a marker of glycocalyx degradation, correlates with the fluid requirement, severity of sepsis, extent of organ dysfunction, and risk of mortality, but a direct relationship with glycocalyx degradation has not been identified (123). The serum syndecan-1 concentration may indirectly reflect the extent of glycocalyx degradation because a study performed in humans showed that syndecan-1 may be a potential biomarker of the quality of donor kidneys (124). However, Hahn et al. found that the renal elimination of syndecan-1 and HS differed significantly. The changes in renal function that are common after trauma and major surgery may lead to several-fold changes in the plasma concentrations of these substances (125), which renders the reliability of these markers questionable. Schmidt et al. found that in septic shock, the glycosaminoglycans fragmentation index is associated with the development of renal insufficiency and in-hospital mortality within 72 h of urine collection (112). Finally, Ying et al. found that syndecan-1 predicts the prognosis of children with septic shock, and SDX may have therapeutic potential for sepsis-associated endothelial dysfunction (126). The search for markers of glycocalyx injury continues.
Concerning the treatment of glycocalyx injury, Urban et al. found that colivelin, a synthetic derivative of the mitochondrial peptide humanin, reduces the plasma syndecan-1, tumor necrosis factor-α (TNF-α), and interleukin-10 (IL-10) concentrations. In addition, the glycocalyx in colivelin-treated mice has thicker and more complex glycosaminoglycan bundles than that in vehicle-treated septic mice (127). Furthermore, the intravenous administration of interferon-β (IFN-β; 1,000 units/20 g) 6 and 18 h after CLP increases the survival rate of mice by 40% (128). Vitamin C (VC) and recombinant thrombomodulin have also been studied for their potential to protect the glycocalyx and improve the prognosis associated with sepsis. In humans, the administration of high doses of VC intravenously for 48 h (50 mg/kg every 6 h) has been shown to reduce the plasma syndecan-1 concentration (129). In addition, the plasma syndecan-1 concentration of septic mice is reduced by recombinant thrombomodulin, suggesting that it ameliorates glycocalyx injury (130). The search for markers of glycocalyx damage that can predict S-AKI is ongoing.
Measures that protect or restore the glycocalyx may reduce the vascular hyperpermeability, inflammation, and organ dysfunction associated with sepsis (131). S1P is a sphingolipid that may help improve glycocalyx integrity by inhibiting syndecan-1 shedding. It binds to the S1P1 receptor, which is most abundantly expressed on vascular endothelial cells, and the activation of this receptor attenuates the activity of MMPs, causing syndecan-1 ectodomain shedding (132). Because the activation of heparanase can increase MMP expression, heparin also may attenuate the increase of MMP expression by inhibiting heparanase activity (133). Fernández-Sarmiento et al. found that children with sepsis, and particularly those who are administered unbalanced crystalloid solutions during resuscitation, demonstrate a loss or deterioration of the EG, and this effect peaks approximately 6 h after the infusion and is often associated with metabolic acidosis and AKI (134). Duan et al. demonstrated that IFN-β alleviates sepsis through the sirtuin 1 (SIRT1)-mediated blockade of EG shedding and that IFN-β plus nicotinamide riboside prevents endothelial damage during sepsis through activation of the SIRT1/heparanase 1 pathway (128). More recently, it has been demonstrated that 10–12 weeks of dietary supplementation with Endocalyx, which contains high-molecular weight HA and other glycocalyx components, restores the glycocalyx and ameliorates age-related vascular dysfunction in aged mice (135). Although this finding is promising, many glycocalyx enzyme-targeting therapies require the oral administration of dietary supplements for a few weeks, which is virtually impossible for patients with sepsis. Instead, glycocalyx-targeting therapies for sepsis and other acute health conditions need to rapidly restore the glycocalyx and be administered parenterally.
Ishiko et al. demonstrated that the intravenous infusion of liposomal nanocarriers of pre-assembled glycocalyx (LNPG) leads to the restoration of the glycocalyx in LPS-treated mice (136). This study had a couple of key strengths that are worth highlighting. First, LNPG administration is a novel means of restoring the glycocalyx, and importantly, LNPG infusion in septic mice restores the glycocalyx within 30 min and maintains its integrity thereafter. Second, the therapeutic effect of LNPG was shown both in vivo and ex vivo, when it was delivered to endothelial cells lacking a glycocalyx (137). Although other therapeutic agents, such as colivelin, have been shown to restore the glycocalyx and reduce the plasma concentration of syndecan-1 in sepsis (127), it is not clear whether these agents are directly integrated into the glycocalyx from the circulation or whether the glycocalyx is restored by de novo synthesis by endothelial cells. To the best of our knowledge, this was the first study of a potential therapy targeting the glycocalyx in sepsis to demonstrate that it can be restored by an exogenous substance (138). Another study showed that hydrogen-rich saline can upregulate the SIRT1/nuclear factor-κB signaling pathway, thus reducing the shedding of vascular EG in S-AKI (139). Furthermore, Xing et al. discovered that knocking down hyaluronidase-1 in mice with LPS-induced sepsis significantly alleviates kidney inflammation, oxidative stress, apoptosis, and renal dysfunction in AKI (140). It also mitigates the damage to the renal EG by preventing the release of hyaluronic acid into the bloodstream. The beneficial effects of hyaluronidase-1 blockade are closely related to activation of the 5′-AMP-activated protein kinase/mechanistic target of rapamycin (AMPK/mTOR) signaling pathway (141). However, the mechanism involved and the therapeutic significance require further investigation. A recent study has shown that tacrolimus reduces apoptosis, maintains the integrity of the glycocalyx, regulates neutrophil infiltration, and alleviates kidney injury in AKI caused by brain death (142). Nevertheless, its effect on S-AKI remains unclear and requires further research. Another study showed that plasma infusion may prevent and treat the degradation of the EG in sepsis, but the current level of evidence is insufficient to prove that this effect is mediated by the glycocalyx. Thus, further research is needed before this approach could be used clinically (143).
Renal tubular endothelial cells and the glycocalyx are closely associated with the development of S-AKI. However, the extent to which glycocalyx restoration improves the prognosis of sepsis needs to be further investigated. This may lead to the discovery of new therapies for sepsis and septic renal injury, as well as other disorders that lead to glycocalyx degradation (Figure 3).
Figure 3. Potential therapeutic approaches and targets for sepsis-induced acute kidney injury, including reducing the production of inflammatory factors, weakening the activity of metallohydrolases, SIRT1-related treatments, inhibiting the shedding of glycocalyx components, and repairing the glycocalyx. VC, Vitamin C; IFN-β, Interferon-β; SIRT-1, Sirtuin 1; MMPs, matrix metalloproteinases; ADAMs, A Disintegrin And Metalloproteinase Domain-containing Proteins; IL-1β, Interleukin-1β; IL-6, Interleukin-6; IL-10, Interleukin-10; TNF-α, Tumor Necrosis Factor-α; FGF, Fibroblast Growth Factor; LNPG, liposomal nanocarriers of pre-assembled glycocalyx; SIRT1, Sirtuin 1; SDX, sulodexide; ROS, Reactive Oxygen Species.
In recent years there has been a gradual increase in research on the glycocalyx, which is an important substance that is synthesized and secreted by vascular endothelial cells and is involved in the maintenance of endothelial structure and function. Under pathologic conditions, glycocalyx degradation indicates damage to the vascular endothelium, which is associated with the development of various pathologies, such as vascular leakage, interstitial edema, the dissemination of inflammation, oxidative stress, vasoconstriction, and even disseminated intravascular coagulation. A large amount of evidence suggests that glycocalyx plays a critical role in S-AKI. To date, research on the role of the EG in S-AKI has mostly been performed in cells or animals, and there have been very few clinical studies. This severe shortage of clinical data has greatly hindered the identification of EG-related molecules as diagnostic markers and therapeutic targets for S-AKI. Even though knowledge regarding the important roles of the glycocalyx has accumulated, the relationship between the impairment of the EG and sepsis or S-AKI has not been fully elucidated. Therefore, further in-depth research is required to explore potential therapeutic strategies targeting the EG.
YW: Writing – original draft. ZZ: Writing – review & editing. XQ: Writing – review & editing. GZ: Writing – review & editing.
The author(s) declare that financial support was received for the research and/or publication of this article. This work was supported by the Beijing Natural Science Foundation (7232126), a Special scientific research project of the Beijing Critical Care Ultrasound Research Association (2023-CCUSG-A-03), and the Medical health research project of Yichang (A24-2-011).
We thank Mark Cleasby, PhD from Liwen Bianji (Edanz; www.liwenbianji.cn) for editing the language of a draft of this manuscript. Figure 1 in this manuscript was created with figdraw.com.
The authors declare that the research was conducted in the absence of any commercial or financial relationships that could be construed as a potential conflict of interest.
The author(s) declare that no Gen AI was used in the creation of this manuscript.
All claims expressed in this article are solely those of the authors and do not necessarily represent those of their affiliated organizations, or those of the publisher, the editors and the reviewers. Any product that may be evaluated in this article, or claim that may be made by its manufacturer, is not guaranteed or endorsed by the publisher.
1. Singer, M, Deutschman, CS, Seymour, CW, Shankar-Hari, M, Annane, D, Bauer, M, et al. The third international consensus definitions for Sepsis and septic shock (Sepsis-3). JAMA. (2016) 315:801–10. doi: 10.1001/jama.2016.0287
2. Bellomo, R, Kellum, JA, Ronco, C, Wald, R, Martensson, J, Maiden, M, et al. Acute kidney injury in sepsis. Intensive Care Med. (2017) 43:816–28. doi: 10.1007/s00134-017-4755-7
3. Song, MJ, Jang, Y, Legrand, M, Park, S, Ko, R, Suh, GY, et al. Epidemiology of sepsis-associated acute kidney injury in critically ill patients: a multicenter, prospective, observational cohort study in South Korea. Crit Care Lond Engl. (2024) 28:383. doi: 10.1186/s13054-024-05167-9
4. Zarbock, A, Nadim, MK, Pickkers, P, Gomez, H, Bell, S, Joannidis, M, et al. Sepsis-associated acute kidney injury: consensus report of the 28th acute disease quality initiative workgroup. Nat Rev Nephrol. (2023) 19:401–17. doi: 10.1038/s41581-023-00683-3
5. Stanski, NL, Cvijanovich, NZ, Fitzgerald, JC, Bigham, MT, and Wong, HR. Genomics of pediatric septic shock investigators. Severe acute kidney injury is independently associated with mortality in children with septic shock. Intensive Care Med. (2020) 46:1050–1. doi: 10.1007/s00134-020-05940-8
6. Schuler, A, Wulf, DA, Lu, Y, Iwashyna, TJ, Escobar, GJ, Shah, NH, et al. The impact of acute organ dysfunction on long-term survival in Sepsis. Crit Care Med. (2018) 46:843–9. doi: 10.1097/CCM.0000000000003023
7. Mas-Font, S, Ros-Martinez, J, Pérez-Calvo, C, Villa-Díaz, P, Aldunate-Calvo, S, Moreno-Clari, E, et al. Prevention of acute kidney injury in intensive care units. Med Int. (2017) 41:116–26. doi: 10.1016/j.medin.2016.12.004
8. Fani, F, Regolisti, G, Delsante, M, Cantaluppi, V, Castellano, G, Gesualdo, L, et al. Recent advances in the pathogenetic mechanisms of sepsis-associated acute kidney injury. J Nephrol. (2018) 31:351–9. doi: 10.1007/s40620-017-0452-4
9. Kataoka, H, Ushiyama, A, Akimoto, Y, Matsubara, S, Kawakami, H, and Iijima, T. Structural behavior of the endothelial Glycocalyx is associated with pathophysiologic status in septic mice: an integrated approach to analyzing the behavior and function of the Glycocalyx using both Electron and fluorescence Intravital microscopy. Anesth Analg. (2017) 125:874–83. doi: 10.1213/ANE.0000000000002057
10. Villalba, N, Baby, S, and Yuan, SY. The endothelial Glycocalyx as a double-edged sword in microvascular homeostasis and pathogenesis. Front Cell Dev Biol. (2021) 9:711003. doi: 10.3389/fcell.2021.711003
11. Joffre, J, Hellman, J, Ince, C, and Ait-Oufella, H. Endothelial responses in Sepsis. Am J Respir Crit Care Med. (2020) 202:361–70. doi: 10.1164/rccm.201910-1911TR
12. Song, JW, Zullo, J, Lipphardt, M, Dragovich, M, Zhang, FX, Fu, B, et al. Endothelial glycocalyx-the battleground for complications of sepsis and kidney injury. Nephrol Dial Transplant Off Publ Eur Dial Transpl Assoc - Eur Ren Assoc. (2018) 33:203–11. doi: 10.1093/ndt/gfx076
13. Luft, JH. Fine structures of capillary and endocapillary layer as revealed by ruthenium red. Fed Proc. (1966) 25:1773–83.
14. Twamley, SG, Stach, A, Heilmann, H, Söhl-Kielczynski, B, Stangl, V, Ludwig, A, et al. Immuno-Electron and confocal laser scanning microscopy of the Glycocalyx. Biology. (2021) 10:402. doi: 10.3390/biology10050402
15. Okada, H, Takemura, G, Suzuki, K, Oda, K, Takada, C, Hotta, Y, et al. Three-dimensional ultrastructure of capillary endothelial glycocalyx under normal and experimental endotoxemic conditions. Crit Care Lond Engl. (2017) 21:261. doi: 10.1186/s13054-017-1841-8
16. Reitsma, S, Slaaf, DW, Vink, H, van Zandvoort, MAMJ, and MGA, o E. The endothelial glycocalyx: composition, functions, and visualization. Pflugers Arch. (2007) 454:345–59. doi: 10.1007/s00424-007-0212-8
17. Leonova, EI, and Galzitskaya, OV. Structure and functions of syndecans in vertebrates. Biochem Biokhimiia. (2013) 78:1071–85. doi: 10.1134/S0006297913100015
18. Gao, L, and Lipowsky, HH. Composition of the endothelial glycocalyx and its relation to its thickness and diffusion of small solutes. Microvasc Res. (2010) 80:394–401. doi: 10.1016/j.mvr.2010.06.005
19. Oshima, K, King, SI, McMurtry, SA, and Schmidt, EP. Endothelial Heparan sulfate proteoglycans in Sepsis: the role of the Glycocalyx. Semin Thromb Hemost. (2021) 47:274–82. doi: 10.1055/s-0041-1725064
20. Richter, RP, Ashtekar, AR, Zheng, L, Pretorius, D, Kaushlendra, T, Sanderson, RD, et al. Glycocalyx heparan sulfate cleavage promotes endothelial cell angiopoietin-2 expression by impairing shear stress-related AMPK/fox O1 signaling. JCI Insight. (2022) 7:e155010. doi: 10.1172/jci.insight.155010
21. Gaudette, S, Hughes, D, and Boller, M. The endothelial glycocalyx: structure and function in health and critical illness. J Vet Emerg Crit Care San Antonio Tex 2001. (2020) 30:117–34. doi: 10.1111/vec.12925
23. Wisse, E. An electron microscopic study of the fenestrated endothelial lining of rat liver sinusoids. J Ultrastruct Res. (1970) 31:125–50. doi: 10.1016/s0022-5320(70)90150-4
24. Rhodin, J. Electron microscopy of the glomerular capillary wall. Exp Cell Res. (1955) 8:572–4. doi: 10.1016/0014-4827(55)90136-1
25. Zhan, J-H, Wei, J, Liu, Y-J, Wang, P-X, and Zhu, X-Y. Sepsis-associated endothelial glycocalyx damage: a review of animal models, clinical evidence, and molecular mechanisms. Int J Biol Macromol. (2025) 295:139548. doi: 10.1016/j.ijbiomac.2025.139548
26. Aw, M, and Hh, L. Role of glycocalyx in leukocyte-endothelial cell adhesion. Am J Physiol Heart Circ Physiol. (2002) 283:H1282–91. doi: 10.1152/ajpheart.00117.2002
27. Chappell, D, Brettner, F, Doerfler, N, Jacob, M, Rehm, M, Bruegger, D, et al. Protection of glycocalyx decreases platelet adhesion after ischaemia/reperfusion: an animal study. Eur J Anaesthesiol. (2014) 31:474–81. doi: 10.1097/EJA.0000000000000085
28. Allen, BL, Filla, MS, and Rapraeger, AC. Role of heparan sulfate as a tissue-specific regulator of FGF-4 and FGF receptor recognition. J Cell Biol. (2001) 155:845–58. doi: 10.1083/jcb.200106075
29. Fromm, JR, Hileman, RE, Weiler, JM, and Linhardt, RJ. Interaction of fibroblast growth factor-1 and related peptides with heparan sulfate and its oligosaccharides. Arch Biochem Biophys. (1997) 346:252–62. doi: 10.1006/abbi.1997.0299
30. Lin, X. Functions of heparan sulfate proteoglycans in cell signaling during development. Dev Camb Engl. (2004) 131:6009–21. doi: 10.1242/dev.01522
31. Perrimon, N, and Bernfield, M. Specificities of heparan sulphate proteoglycans in developmental processes. Nature. (2000) 404:725–8. doi: 10.1038/35008000
32. Shi, Y, Ji, S, Xu, Y, Ji, J, Yang, X, Ye, B, et al. Global trends in research on endothelial cells and sepsis between 2002 and 2022: A systematic bibliometric analysis. Heliyon. (2024) 10:e23599. doi: 10.1016/j.heliyon.2023.e23599
33. Kolesov, D, Astakhova, A, Galdobina, M, Moskovtsev, A, Kubatiev, A, Sokolovskaya, A, et al. Scanning probe microscopy techniques for studying the cell Glycocalyx. Cells. (2023) 12:2778. doi: 10.3390/cells12242778
34. Zeng, Y, and Fu, BM. Angiogenesis and microvascular permeability. Cold Spring Harb Perspect Med. (2025) 15:a041163. doi: 10.1101/cshperspect.a041163
35. Pisano, C, Asta, L, Sbrigata, A, and Balistreri, CR. A narrative review: Syndecans in aortic aneurysm pathogenesis and course-biomarkers and targets? Int J Mol Sci. (2025) 26:1211. doi: 10.3390/ijms26031211
36. Vahldieck, C, Löning, S, Hamacher, C, Fels, B, Rudzewski, B, Nickel, L, et al. Dysregulated complement activation during acute myocardial infarction leads to endothelial glycocalyx degradation and endothelial dysfunction via the C5a: C5a-receptor 1 axis. Front Immunol. (2024) 15:1426526. doi: 10.3389/fimmu.2024.1426526
37. Kei, CY, Singh, K, Dautov, RF, Nguyen, TH, Chirkov, YY, and Horowitz, JD. Coronary “microvascular dysfunction”: evolving understanding of pathophysiology, clinical implications, and potential therapeutics. Int J Mol Sci. (2023) 24:11287. doi: 10.3390/ijms241411287
38. Kršek, A, Batičić, L, Ćurko-Cofek, B, Batinac, T, Laškarin, G, Miletić-Gršković, S, et al. Insights into the molecular mechanism of endothelial Glycocalyx dysfunction during heart surgery. Curr Issues Mol Biol. (2024) 46:3794–809. doi: 10.3390/cimb46050236
39. Milusev, A, Rieben, R, and Sorvillo, N. The endothelial Glycocalyx: A possible therapeutic target in cardiovascular disorders. Front Cardiovasc Med. (2022) 9:897087. doi: 10.3389/fcvm.2022.897087
40. Zhou, T, Long, K, Chen, J, Zhi, L, Zhou, X, and Gao, P. Global research progress of endothelial cells and ALI/ARDS: A bibliometric analysis. Front Physiol. (2024). 15:1326392. doi: 10.3389/fphys.2024.1326392
41. Zha, D, Fu, M, and Qian, Y. Vascular endothelial Glycocalyx damage and potential targeted therapy in COVID-19. Cells. (2022) 11:1972. doi: 10.3390/cells11121972
42. Xu, S-W, Ilyas, I, and Weng, J-P. Endothelial dysfunction in COVID-19: an overview of evidence, biomarkers, mechanisms and potential therapies. Acta Pharmacol Sin. (2023) 44:695–709. doi: 10.1038/s41401-022-00998-0
43. Wu, X, Xiang, M, Jing, H, Wang, C, Novakovic, VA, and Shi, J. Damage to endothelial barriers and its contribution to long COVID. Angiogenesis. (2024) 27:5–22. doi: 10.1007/s10456-023-09878-5
44. Rizzo, AN, and Schmidt, EP. The role of the alveolar epithelial glycocalyx in acute respiratory distress syndrome. Am J Phys Cell Phys. (2023) 324:C799–806. doi: 10.1152/ajpcell.00555.2022
45. Zhang, D, Qi, B, Peng, Z, Huang, X, Chen, Y, Sun, T, et al. Heparan sulfate acts in synergy with tight junction through STAT3 signaling to maintain the endothelial barrier and prevent lung injury development. Int Immunopharmacol. (2025) 147:113957. doi: 10.1016/j.intimp.2024.113957
46. Zeng, Y, Qiu, Y, Jiang, W, and Fu, BM. Glycocalyx acts as a central player in the development of tumor microenvironment by extracellular vesicles for angiogenesis and metastasis. Cancer. (2022) 14:5415. doi: 10.3390/cancers14215415
47. Kaur, G, and Harris, NR. Endothelial glycocalyx in retina, hyperglycemia, and diabetic retinopathy. Am J Phys Cell Phys. (2023) 324:C1061–77. doi: 10.1152/ajpcell.00188.2022
48. Yu, H, Song, Y-Y, and Li, X-H. Early diabetic kidney disease: focus on the glycocalyx. World J Diabetes. (2023) 14:460–80. doi: 10.4239/wjd.v14.i5.460
49. Dancy, C, Heintzelman, KE, and Katt, ME. The Glycocalyx: the importance of sugar coating the blood-brain barrier. Int J Mol Sci. (2024) 25:8404. doi: 10.3390/ijms25158404
50. Kitasato, L, Yamaoka-Tojo, M, Iwaya, T, Murayama, Y, Ikeda, Y, Hashikata, T, et al. Rivaroxaban as a protector of oxidative stress-induced vascular endothelial Glycocalyx damage via the IQGAP1/PAR1-2/PI3K/Akt pathway. J Vasc Res. (2025) 62:1–15. doi: 10.1159/000542419
51. de Oliveira, JGCG, and Miranda, CH. Doxycycline protects against sepsis-induced endothelial glycocalyx shedding. Sci Rep. (2024) 14:10477. doi: 10.1038/s41598-024-60919-5
52. Crompton, M, Skinner, LJ, Satchell, SC, and Butler, MJ. Aldosterone: essential for life but damaging to the vascular endothelium. Biomol Ther. (2023) 13:1004. doi: 10.3390/biom13061004
53. Schmidt, EP, Yang, Y, Janssen, WJ, Gandjeva, A, Perez, MJ, Barthel, L, et al. The pulmonary endothelial glycocalyx regulates neutrophil adhesion and lung injury during experimental sepsis. Nat Med. (2012) 18:1217–23. doi: 10.1038/nm.2843
54. Becker, BF, Chappell, D, and Jacob, M. Endothelial glycocalyx and coronary vascular permeability: the fringe benefit. Basic Res Cardiol. (2010) 105:687–701. doi: 10.1007/s00395-010-0118-z
55. Zullo, JA, Fan, J, Azar, TT, Yen, W, Zeng, M, Chen, J, et al. Exocytosis of endothelial lysosome-related organelles hair-triggers a patchy loss of Glycocalyx at the onset of Sepsis. Am J Pathol. (2016) 186:248–58. doi: 10.1016/j.ajpath.2015.10.001
56. Song, JW, Zullo, JA, Liveris, D, Dragovich, M, Zhang, XF, and Goligorsky, MS. Therapeutic restoration of endothelial Glycocalyx in Sepsis. J Pharmacol Exp Ther. (2017) 361:115–21. doi: 10.1124/jpet.116.239509
57. Lira Chavez, FM, Gartzke, LP, van Beuningen, FE, Wink, SE, Henning, RH, Krenning, G, et al. Restoring the infected powerhouse: mitochondrial quality control in sepsis. Redox Biol. (2023) 68:102968. doi: 10.1016/j.redox.2023.102968
58. Zhang, X, Sun, D, Song, JW, Zullo, J, Lipphardt, M, Coneh-Gould, L, et al. Endothelial cell dysfunction and glycocalyx - A vicious circle. Matrix Biol J Int Soc Matrix Biol. (2018) 71-72:421–31. doi: 10.1016/j.matbio.2018.01.026
59. Van Wyngene, L, Vandewalle, J, and Libert, C. Reprogramming of basic metabolic pathways in microbial sepsis: therapeutic targets at last? EMBO Mol Med. (2018) 10:e8712. doi: 10.15252/emmm.201708712
60. McGarrity, S, Anuforo, Ó, Halldórsson, H, Bergmann, A, Halldórsson, S, Palsson, S, et al. Metabolic systems analysis of LPS induced endothelial dysfunction applied to sepsis patient stratification. Sci Rep. (2018) 8:6811. doi: 10.1038/s41598-018-25015-5
61. Goligorsky, MS, and Sun, D. Glycocalyx in Endotoxemia and Sepsis. Am J Pathol. (2020) 190:791–8. doi: 10.1016/j.ajpath.2019.06.017
62. Mishra, HK, Ma, J, and Walcheck, B. Ectodomain shedding by ADAM17: its role in neutrophil recruitment and the impairment of this process during Sepsis. Front Cell Infect Microbiol. (2017) 7:138. doi: 10.3389/fcimb.2017.00138
63. Lin, J-J, Chan, O-W, Hsiao, H-J, Wang, Y, Hsia, S-H, and Chiu, C-H. Decreased ADAMTS 13 activity is associated with disease severity and outcome in pediatric severe Sepsis. Medicine (Baltimore). (2016) 95:e3374. doi: 10.1097/MD.0000000000003374
64. Goldberg, R, Meirovitz, A, Hirshoren, N, Bulvik, R, Binder, A, Rubinstein, AM, et al. Versatile role of heparanase in inflammation. Matrix Biol J Int Soc Matrix Biol. (2013) 32:234–40. doi: 10.1016/j.matbio.2013.02.008
65. Ilan, N, Elkin, M, and Vlodavsky, I. Regulation, function and clinical significance of heparanase in cancer metastasis and angiogenesis. Int J Biochem Cell Biol. (2006) 38:2018–39. doi: 10.1016/j.biocel.2006.06.004
66. Masola, V, Zaza, G, Gambaro, G, Onisto, M, Bellin, G, Vischini, G, et al. Heparanase: A potential new factor involved in the renal epithelial mesenchymal transition (EMT) induced by ischemia/reperfusion (I/R) injury. PLoS One. (2016) 11:e0160074. doi: 10.1371/journal.pone.0160074
67. Masola, V, Zaza, G, Bellin, G, and Dall'Olmo, L. Heparanase regulates the M1 polarization of renal macrophages and their crosstalk with renal epithelial tubular cells after ischemia/reperfusion injury. FASEB J Off Publ Fed Am Soc Exp Biol. (2018) 32:742–56. doi: 10.1096/fj.201700597R
68. Powell, JD, and Horton, MR. Threat matrix: low-molecular-weight hyaluronan (HA) as a danger signal. Immunol Res. (2005) 31:207–18. doi: 10.1385/IR:31:3:207
69. Jiang, D, Liang, J, and Noble, PW. Hyaluronan as an immune regulator in human diseases. Physiol Rev. (2011) 91:221–64. doi: 10.1152/physrev.00052.2009
70. Day, AJ, and de la Motte, CA. Hyaluronan cross-linking: a protective mechanism in inflammation? Trends Immunol. (2005) 26:637–43. doi: 10.1016/j.it.2005.09.009
71. D’Agostino, A, Stellavato, A, Corsuto, L, Diana, P, Filosa, R, La Gatta, A, et al. Is molecular size a discriminating factor in hyaluronan interaction with human cells? Carbohydr Polym. (2017) 157:21–30. doi: 10.1016/j.carbpol.2016.07.125
72. Tenhunen, AB, van der Heijden, J, Skorup, P, Maccarana, M, Larsson, A, Larsson, A, et al. Fluid restrictive resuscitation with high molecular weight hyaluronan infusion in early peritonitis sepsis. Intensive Care Med Exp. (2023) 11:63. doi: 10.1186/s40635-023-00548-w
73. Stern, R, Asari, AA, and Sugahara, KN. Hyaluronan fragments: an information-rich system. Eur J Cell Biol. (2006) 85:699–715. doi: 10.1016/j.ejcb.2006.05.009
74. Nelson, A, Berkestedt, I, and Bodelsson, M. Circulating glycosaminoglycan species in septic shock. Acta Anaesthesiol Scand. (2014) 58:36–43. doi: 10.1111/aas.12223
75. Declèves, A-E, Caron, N, Voisin, V, Legrand, A, Bouby, N, Kultti, A, et al. Synthesis and fragmentation of hyaluronan in renal ischaemia. Nephrol Dial Transplant Off Publ Eur Dial Transpl Assoc - Eur Ren Assoc. (2012) 27:3771–81. doi: 10.1093/ndt/gfs098
76. Jiang, D, Liang, J, and Noble, PW. Hyaluronan in tissue injury and repair. Annu Rev Cell Dev Biol. (2007) 23:435–61. doi: 10.1146/annurev.cellbio.23.090506.123337
77. Wilke, S, Krausze, J, and Büssow, K. Crystal structure of the conserved domain of the DC lysosomal associated membrane protein: implications for the lysosomal glycocalyx. BMC Biol. (2012) 10:62. doi: 10.1186/1741-7007-10-62
78. Settembre, C, Fraldi, A, Medina, DL, and Ballabio, A. Signals from the lysosome: a control Centre for cellular clearance and energy metabolism. Nat Rev Mol Cell Biol. (2013) 14:283–96. doi: 10.1038/nrm3565
79. Kuo, M-C, Patschan, D, Patschan, S, Cohen-Gould, L, Park, H-C, Ni, J, et al. Ischemia-induced exocytosis of Weibel-Palade bodies mobilizes stem cells. J Am Soc Nephrol JASN. (2008) 19:2321–30. doi: 10.1681/ASN.2007111200
80. Freeman, CG, Parish, CR, Knox, KJ, Blackmore, JL, Lobov, SA, King, DW, et al. The accumulation of circulating histones on heparan sulphate in the capillary glycocalyx of the lungs. Biomaterials. (2013) 34:5670–6. doi: 10.1016/j.biomaterials.2013.03.091
81. Hu, Z, Cano, I, Lei, F, Liu, J, Bossardi Ramos, R, Gordon, H, et al. Loss of the endothelial Glycocalyx component EMCN leads to glomerular impairment. Circ Res. (2025) 136:59–74. doi: 10.1161/CIRCRESAHA.124.325218
82. Molema, G, and Aird, WC. Vascular heterogeneity in the kidney. Semin Nephrol. (2012) 32:145–55. doi: 10.1016/j.semnephrol.2012.02.001
83. Iba, T, and Levy, JH. Derangement of the endothelial glycocalyx in sepsis. J Thromb Haemost. (2019) 17:283–94. doi: 10.1111/jth.14371
84. Jedlicka, J, Becker, BF, and Chappell, D. Endothelial Glycocalyx. Crit Care Clin. (2020) 36:217–32. doi: 10.1016/j.ccc.2019.12.007
85. Molema, G, Zijlstra, JG, van Meurs, M, and Kamps, JAAM. Renal microvascular endothelial cell responses in sepsis-induced acute kidney injury. Nat Rev Nephrol. (2022) 18:95–112. doi: 10.1038/s41581-021-00489-1
86. Hoste, EA, Bagshaw, SM, and Bellomo, R. Epidemiology of acute kidney injury in critically ill patients: the multinational AKI-EPI study. Intensive Care Med. (2015) 41:1411–23. doi: 10.1007/s00134-015-3934-7
87. Ishikawa, K, May, CN, Gobe, G, Langenberg, C, and Bellomo, R. Pathophysiology of septic acute kidney injury: a different view of tubular injury. Contrib Nephrol. (2010) 165:18–27. doi: 10.1159/000313740
88. Jourde-Chiche, N, Fakhouri, F, Dou, L, Bellien, J, Burtey, S, Frimat, M, et al. Endothelium structure and function in kidney health and disease. Nat Rev Nephrol. (2019) 15:87–108. doi: 10.1038/s41581-018-0098-z
89. Rabelink, TJ, and de Zeeuw, D. The glycocalyx--linking albuminuria with renal and cardiovascular disease. Nat Rev Nephrol. (2015) 11:667–76. doi: 10.1038/nrneph.2015.162
90. Li, L, and Bonventre, JV. Endothelial Glycocalyx: not just a sugar coat. Am J Respir Crit Care Med. (2016) 194:390–3. doi: 10.1164/rccm.201603-0624ED
91. Li, Z, Jiang, X, Li, J, and Wang, Y. Correlation of Hyaluronic Acid (HA), Syndecan-1 (SDC-1), Heparan sulfate (HS) with early stage end organ dysfunction in Sepsis patients. J Cardiovasc Pharmacol. (2025) 85:129–36. doi: 10.1097/FJC.0000000000001654
92. Zafrani, L, Payen, D, Azoulay, E, and Ince, C. The microcirculation of the septic kidney. Semin Nephrol. (2015) 35:75–84. doi: 10.1016/j.semnephrol.2015.01.008
93. Garsen, M, Benner, M, Dijkman, HB, van Kuppevelt, TH, Li, J-P, Rabelink, TJ, et al. Heparanase is essential for the development of acute experimental glomerulonephritis. Am J Pathol. (2016) 186:805–15. doi: 10.1016/j.ajpath.2015.12.008
94. Xu, C, Chang, A, Hack, BK, Eadon, MT, Alper, SL, and Cunningham, PN. TNF-mediated damage to glomerular endothelium is an important determinant of acute kidney injury in sepsis. Kidney Int. (2014). 85:72–81. doi: 10.1038/ki.2013.286
95. Adembri, C, Sgambati, E, Vitali, L, Selmi, V, Margheri, M, Tani, A, et al. Sepsis induces albuminuria and alterations in the glomerular filtration barrier: a morphofunctional study in the rat. Crit Care Lond Engl. (2011) 15:R277. doi: 10.1186/cc10559
96. Lygizos, MI, Yang, Y, Altmann, CJ, Okamura, K, Hernando, AA, Perez, MJ, et al. Heparanase mediates renal dysfunction during early sepsis in mice. Phys Rep. (2013) 1:e00153. doi: 10.1002/phy2.153
97. Maciej-Hulme, ML, Van Gemst, JJ, Sanderson, P, Rops, ALWMM, Berden, JH, Smeets, B, et al. Glomerular endothelial glycocalyx-derived heparan sulfate inhibits glomerular leukocyte influx and attenuates experimental glomerulonephritis. Front Mol Biosci. (2023) 10:1177560. doi: 10.3389/fmolb.2023.1177560
98. Salmon, AHJ, Ferguson, JK, Burford, JL, Gevorgyan, H, Nakano, D, Harper, SJ, et al. Loss of the endothelial glycocalyx links albuminuria and vascular dysfunction. J Am Soc Nephrol JASN. (2012) 23:1339–50. doi: 10.1681/ASN.2012010017
99. Hogwood, J, Gray, E, and Mulloy, B. Heparin, Heparan Sulphate and Sepsis: potential new options for treatment. Pharm Basel Switz. (2023) 16:271. doi: 10.3390/ph16020271
100. Li, J-C, Wang, L-J, Feng, F, Chen, T-T, Shi, W-G, and Liu, L-P. Role of heparanase in sepsis-related acute kidney injury (review). Exp Ther Med. (2023) 26:379. doi: 10.3892/etm.2023.12078
101. Margraf, A, Herter, JM, Kühne, K, Stadtmann, A, Ermert, T, Wenk, M, et al. 6% hydroxyethyl starch (HES 130/0.4) diminishes glycocalyx degradation and decreases vascular permeability during systemic and pulmonary inflammation in mice. Crit Care Lond Engl. (2018) 22:111. doi: 10.1186/s13054-017-1846-3
102. Cusack, R, Leone, M, Rodriguez, AH, and Martin-Loeches, I. Endothelial damage and the microcirculation in critical illness. Biomedicine. (2022) 10:3150. doi: 10.3390/biomedicines10123150
103. Simons, M, Gordon, E, and Claesson-Welsh, L. Mechanisms and regulation of endothelial VEGF receptor signalling. Nat Rev Mol Cell Biol. (2016) 17:611–25. doi: 10.1038/nrm.2016.87
104. Rops, ALWMM, Loeven, MA, van Gemst, JJ, Eversen, I, Van Wijk, XM, Dijkman, HB, et al. Modulation of heparan sulfate in the glomerular endothelial glycocalyx decreases leukocyte influx during experimental glomerulonephritis. Kidney Int. (2014) 86:932–42. doi: 10.1038/ki.2014.115
105. Cox, LAE, van Eijk, LT, Ramakers, BPC, Dorresteijn, MJ, Gerretsen, J, Kox, M, et al. Inflammation-induced increases in plasma endocan levels are associated with endothelial dysfunction in humans in vivo. Shock Augusta Ga. (2015) 43:322–6. doi: 10.1097/SHK.0000000000000320
106. Suzuki, A, Tomita, H, and Okada, H. Form follows function: the endothelial glycocalyx. Transl Res J Lab Clin Med. (2022) 247:158–67. doi: 10.1016/j.trsl.2022.03.014
107. Gil, N, Goldberg, R, Neuman, T, Garsen, M, Zcharia, E, Rubinstein, AM, et al. Heparanase is essential for the development of diabetic nephropathy in mice. Diabetes. (2012) 61:208–16. doi: 10.2337/db11-1024
108. Santacruz, CA, Pereira, AJ, Celis, E, and Vincent, J-L. Which multicenter randomized controlled trials in critical care medicine have shown reduced mortality? A Systematic Review. Crit Care Med. (2019) 47:1680–91. doi: 10.1097/CCM.0000000000004000
109. Wang, J, Ma, L, Fang, Y, Ye, T, Li, H, and Lan, P. Factors influencing glycocalyx degradation: a narrative review. Front Immunol. (2024) 15:1490395. doi: 10.3389/fimmu.2024.1490395
110. Wei, J, Mo, H, Zhang, Y, Deng, W, Zheng, S, Mao, H, et al. Evolutionary trend analysis and knowledge structure mapping of endothelial dysfunction in sepsis: a bibliometrics study. World J Emerg Med. (2024) 15:386–96. doi: 10.5847/wjem.j.1920-8642.2024.083
111. Nelson, A, Berkestedt, I, Schmidtchen, A, Ljunggren, L, and Bodelsson, M. Increased levels of glycosaminoglycans during septic shock: Relation to mortality and the antibacterial actions of plasma. Shock. (2008). 30:623–627. doi: 10.1097/SHK.0b013e3181777da3
112. Schmidt, EP, Overdier, KH, Sun, X, Lin, L, Liu, X, Yang, Y, et al. Urinary Glycosaminoglycans predict outcomes in septic shock and acute respiratory distress syndrome. Am J Respir Crit Care Med. (2016) 194:439–49. doi: 10.1164/rccm.201511-2281OC
113. Zhang, Z. Biomarkers, diagnosis and management of sepsis-induced acute kidney injury: a narrative review. Heart Lung Vessels. (2015) 7:64–73.
114. Roffi, M, Patrono, C, Collet, J-P, Mueller, C, Valgimigli, M, Andreotti, F, et al. 2015 ESC guidelines for the management of acute coronary syndromes in patients presenting without persistent ST-segment elevation: task force for the Management of Acute Coronary Syndromes in patients presenting without persistent ST-segment elevation of the European Society of Cardiology (ESC). Eur Heart J. (2016) 37:267–315. doi: 10.1093/eurheartj/ehv320
115. Morrow, DA, Cannon, CP, Rifai, N, Frey, MJ, Vicari, R, Lakkis, N, et al. Ability of minor elevations of troponins I and T to predict benefit from an early invasive strategy in patients with unstable angina and non-ST elevation myocardial infarction: results from a randomized trial. JAMA. (2001) 286:2405–12. doi: 10.1001/jama.286.19.2405
116. Yang, J, Miao, X, Guan, Y, Chen, C, Chen, S, Zhang, X, et al. Microbubble functionalization with platelet membrane enables targeting and early detection of Sepsis-induced acute kidney injury. Adv Healthc Mater. (2021) 10:e2101628. doi: 10.1002/adhm.202101628
117. Rickli, H, Benou, K, Ammann, P, Fehr, T, Brunner-La Rocca, HP, Petridis, H, et al. Time course of serial cystatin C levels in comparison with serum creatinine after application of radiocontrast media. Clin Nephrol. (2004) 61:98–102. doi: 10.5414/cnp61098
118. Waikar, SS, and Bonventre, JV. Creatinine kinetics and the definition of acute kidney injury. J Am Soc Nephrol JASN. (2009) 20:672–9. doi: 10.1681/ASN.2008070669
119. Klementa, V, Petejova, N, Zadrazil, J, Horak, P, Proskova, J, Langova, K, et al. Prediction of acute kidney injury development in critically ill septic patients based on NGAL determination. Physiol Res. (2024) 73:1001–11. doi: 10.33549/physiolres.935336
120. Macdonald, S, Bosio, E, Shapiro, NI, Balmer, L, Burrows, S, Hibbs, M, et al. No association between intravenous fluid volume and endothelial glycocalyx shedding in patients undergoing resuscitation for sepsis in the emergency department. Sci Rep. (2022). 12:8733. doi: 10.1038/s41598-022-12752-x
121. Soubihe Neto, N, de Almeida, MCV, de O, CH, and Miranda, CH. Biomarkers of endothelial glycocalyx damage are associated with microvascular dysfunction in resuscitated septic shock patients. Microvasc Res. (2024) 154:104683. doi: 10.1016/j.mvr.2024.104683
122. Piotti, A, Novelli, D, Meessen, JMTA, Ferlicca, D, Coppolecchia, S, Marino, A, et al. Endothelial damage in septic shock patients as evidenced by circulating syndecan-1, sphingosine-1-phosphate and soluble VE-cadherin: a substudy of ALBIOS. Crit Care Lond Engl. (2021) 25:113. doi: 10.1186/s13054-021-03545-1
123. Saoraya, J, Wongsamita, L, Srisawat, N, and Musikatavorn, K. Plasma syndecan-1 is associated with fluid requirements and clinical outcomes in emergency department patients with sepsis. Am J Emerg Med. (2021) 42:83–9. doi: 10.1016/j.ajem.2021.01.019
124. Navratil, P, Sahi, S, Hruba, P, Ticha, A, Timkova, K, Viklicky, O, et al. Syndecan-1 in the serum of deceased kidney donors as a potential biomarker of kidney function. Transplant Proc. (2025) 57:187–93. doi: 10.1016/j.transproceed.2024.12.031
125. Hahn, RG, Zdolsek, M, and Zdolsek, J. Plasma concentrations of syndecan-1 are dependent on kidney function. Acta Anaesthesiol Scand. (2021) 65:809–15. doi: 10.1111/aas.13801
126. Ying, J, Zhang, C, Wang, Y, Liu, T, Yu, Z, Wang, K, et al. Sulodexide improves vascular permeability via glycocalyx remodelling in endothelial cells during sepsis. Front Immunol. (2023) 14:1172892. doi: 10.3389/fimmu.2023.1172892
127. Urban, C, Hayes, HV, Piraino, G, Wolfe, V, Lahni, P, O’Connor, M, et al. Colivelin, a synthetic derivative of humanin, ameliorates endothelial injury and glycocalyx shedding after sepsis in mice. Front Immunol. (2022) 13:984298. doi: 10.3389/fimmu.2022.984298
128. Duan, S, Kim, S-G, Lim, H-J, Song, H-R, and Han, M-K. Interferon-β alleviates sepsis by SIRT1-mediated blockage of endothelial glycocalyx shedding. BMB Rep. (2023) 56:314–9. doi: 10.5483/BMBRep.2023-0030
129. Qiao, X, Kashiouris, MG, L’Heureux, M, Fisher, BJ, Leichtle, SW, Truwit, JD, et al. Biological effects of intravenous vitamin C on neutrophil extracellular traps and the endothelial Glycocalyx in patients with Sepsis-induced ARDS. Nutrients. (2022) 14:4415. doi: 10.3390/nu14204415
130. Watanabe, E, Akamatsu, T, Ohmori, M, Kato, M, Takeuchi, N, Ishiwada, N, et al. Recombinant thrombomodulin attenuates hyper-inflammation and glycocalyx damage in a murine model of Streptococcus pneumoniae-induced sepsis. Cytokine. (2022) 149:155723. doi: 10.1016/j.cyto.2021.155723
131. Martin, L, Koczera, P, Zechendorf, E, and Schuerholz, T. The endothelial Glycocalyx: new diagnostic and therapeutic approaches in Sepsis. Biomed Res Int. (2016) 2016:3758278. doi: 10.1155/2016/3758278
132. Zeng, Y, Adamson, RH, Curry, FRE, and Tarbell, JM. Sphingosine-1-phosphate protects endothelial glycocalyx by inhibiting syndecan-1 shedding. Am J Physiol Heart Circ Physiol. (2014) 306:H363–72. doi: 10.1152/ajpheart.00687.2013
133. Purushothaman, A, Chen, L, Yang, Y, and Sanderson, RD. Heparanase stimulation of protease expression implicates it as a master regulator of the aggressive tumor phenotype in myeloma. J Biol Chem. (2008) 283:32628–36. doi: 10.1074/jbc.M806266200
134. Fernández-Sarmiento, J, Salazar-Peláez, LM, Acevedo, L, Niño-Serna, LF, Flórez, S, Alarcón-Forero, L, et al. Endothelial and glycocalyx biomarkers in children with sepsis after one bolus of unbalanced or balanced crystalloids. Pediatr Crit Care Med. (2023). 24:213–221. doi: 10.1097/PCC.0000000000003123
135. Machin, DR, Trott, DW, Gogulamudi, VR, Islam, MT, Bloom, SI, Vink, H, et al. Glycocalyx-targeted therapy ameliorates age-related arterial dysfunction. GeroScience. (2023) 45:2351–65. doi: 10.1007/s11357-023-00745-1
136. Ishiko, S, Ben Rahoma, G, Kandhi, S, Huang, A, and Sun, D. Liposomal nanocarriers of preassembled glycocalyx expeditiously restore endothelial glycocalyx in endotoxemia. Am J Physiol Heart Circ Physiol. (2023) 325:H645–55. doi: 10.1152/ajpheart.00196.2023
137. Ishiko, S, Huang, A, and Sun, D. Long-term efficacy of liposomal nanocarriers of preassembled glycocalyx in restoring cerebral endothelial glycocalyx in sepsis. Microvasc Res. (2024) 154:104684. doi: 10.1016/j.mvr.2024.104684
138. Zheng, X, and Machin, DR. Patching up a degraded endothelial glycocalyx in sepsis. Am J Physiol Heart Circ Physiol. (2023) 325:H673–4. doi: 10.1152/ajpheart.00499.2023
139. Lin, L, Qiu, D, Yang, F, Xia, Y, Cai, S, Liao, X, et al. Hydrogen-rich saline upregulates the sirt1/nf-κb signaling pathway and reduces vascular endothelial glycocalyx shedding in sepsis-induced acute kidney injury. Shock Augusta Ga. (2024) 62:416–25. doi: 10.1097/SHK.0000000000002404
140. Colombaro, V, Jadot, I, Declèves, A-E, Voisin, V, Giordano, L, Habsch, I, et al. Lack of hyaluronidases exacerbates renal post-ischemic injury, inflammation, and fibrosis. Kidney Int. (2015) 88:61–71. doi: 10.1038/ki.2015.53
141. Xing, H, Li, S, Fu, Y, Wan, X, Zhou, A, Cao, F, et al. HYAL1 deficiency attenuates lipopolysaccharide-triggered renal injury and endothelial glycocalyx breakdown in septic AKI in mice. Ren Fail. (2023) 45:2188966. doi: 10.1080/0886022X.2023.2188966
142. Idouz, K, Belhaj, A, Rondelet, B, Dewachter, L, Flamion, B, Kirschvink, N, et al. Cascading renal injury after brain death: unveiling glycocalyx alteration and the potential protective role of tacrolimus. Front Cell Dev Biol. (2024) 12:1449209. doi: 10.3389/fcell.2024.1449209
143. Kravitz, MS, Kattouf, N, Stewart, IJ, Ginde, AA, Schmidt, EP, and Shapiro, NI. Plasma for prevention and treatment of glycocalyx degradation in trauma and sepsis. Crit Care Lond Engl. (2024) 28:254. doi: 10.1186/s13054-024-05026-7
S-AKI – sepsis-induced acute kidney injury
ICUs – intensive care units
EG – endothelial glycocalyx
AKI – acute kidney injury
HS – heparan sulfate
ICAM – intercellular adhesion molecule
VCAM – vascular cell adhesion molecule
FGF – Fibroblast Growth Factor
NO – nitric oxide
MMPs – matrix metalloproteinases
ADAMs – A Disintegrin And Metalloproteinase Domain-containing Proteins
CD44 – Cluster of Differentiation 44
HA – hyaluronic acid
LPS – lipopolysaccharide
EMCN – endo mucin
BUN – blood urea nitrogen
CLP – cecum ligation and puncture
GFR – glomerular filtration rate
NGAL – neutrophil gelatinase-associated lipocalcin
S1P – Sphingosine 1-Phosphate
TNF-
IL-10 – Interleukin-10
IL-1β – Interleukin-1β
VC – Vitamin C
SIRT1 – Sirtuin 1
LNPG – liposomal nanocarriers of pre-assembled glycocalyx
AMPK/mTOR – 5′-Adenosine Monophosphate-Activated Protein Kinase/mammalian Target Of Rapamycin
IL-6 – Interleukin-6
vWF – Von Willebrand Factor
SOD – Reactive Oxygen Species
SDX – sulodexide
ROS – Reactive Oxygen Species
Keywords: glycocalyx, sepsis, endothelium, kidney, inflammation
Citation: Wang Y, Zhang Z, Qu X and Zhou G (2025) Role of the endothelial cell glycocalyx in sepsis-induced acute kidney injury. Front. Med. 12:1535673. doi: 10.3389/fmed.2025.1535673
Received: 27 November 2024; Accepted: 25 March 2025;
Published: 04 April 2025.
Edited by:
Vasilios Kotsis, Aristotle University of Thessaloniki, GreeceReviewed by:
Panagiota Anyfanti, Aristotle University of Thessaloniki, GreeceCopyright © 2025 Wang, Zhang, Qu and Zhou. This is an open-access article distributed under the terms of the Creative Commons Attribution License (CC BY). The use, distribution or reproduction in other forums is permitted, provided the original author(s) and the copyright owner(s) are credited and that the original publication in this journal is cited, in accordance with accepted academic practice. No use, distribution or reproduction is permitted which does not comply with these terms.
*Correspondence: Xingguang Qu, cXV4aW5nZ3VhbmcxOTYxQGN0Z3UuZWR1LmNu; Gaosheng Zhou, Z3N6aG91MjAxMkAxNjMuY29t
Disclaimer: All claims expressed in this article are solely those of the authors and do not necessarily represent those of their affiliated organizations, or those of the publisher, the editors and the reviewers. Any product that may be evaluated in this article or claim that may be made by its manufacturer is not guaranteed or endorsed by the publisher.
Research integrity at Frontiers
Learn more about the work of our research integrity team to safeguard the quality of each article we publish.