- Department of Pharmaceutical Sciences, Irma Lerma Rangel College of Pharmacy, Texas A&M University, College Station, TX, United States
Human immunodeficiency virus (HIV) infection is the cause of acquired immunodeficiency syndrome (AIDS). Combination antiretroviral therapy (cART) has successfully controlled AIDS, but HIV-associated neurocognitive disorders (HANDs) remain prevalent among people with HIV. HIV infection is often associated with substance use, which promotes HIV transmission and viral replication and exacerbates HANDs even in the era of cART. Thus, the comorbid effects of substance use exacerbate the neuropathogenesis of HANDs. Unraveling the mechanism(s) of this comorbid exacerbation at the molecular, cell-type, and brain region levels may provide a better understanding of HAND persistence. This review aims to highlight the comorbid effects of HIV and substance use in specific brain regions and cell types involved in the persistence of HANDs. This review includes an overview of post-translational modifications, alterations in microglia-specific biomarkers, and possible mechanistic pathways that may link epigenomic modifications to functional protein alterations in microglia. The impairment of the microglial proteins that are involved in neural circuit function appears to contribute to the breakdown of cellular communication and neurodegeneration in HANDs. The epigenetic modification of N-terminal acetylation is currently understudied, which is discussed in brief to demonstrate the important role of this epigenetic modification in infected microglia within specific brain regions. The discussion also explores whether combined antiretroviral therapy is effective in preventing HIV infection or substance-use-mediated post-translational modifications and protein alterations in the persistence of neuropathogenesis in HANDs.
1 Introduction
Recent advances in combined antiretroviral therapy (cART) have visibly reduced the levels of HIV infection, replication, and HIV/AIDS progression. Moreover, these advances have contributed to gaining a better understanding of central nervous system (CNS) viral invasion, persistence, and neuropathogenesis (1–4). It is evident that while cART can control viral replication, a cure for HIV/AIDS remains elusive due to the persistence of the HIV-1 latent reservoir in microglia and monocytes/macrophages, as well as the limited penetration of cART across the blood-brain barrier (BBB) and its confinement within the restricted skull cavity (5–8). However, it has been shown that there is a direct correlation between ART concentrations and HIV-1 viral load in the brain (9), which indicates that cART can penetrate the BBB and enter the brain. Penetration of cART into the brain has been shown to cause adverse effects, including neurological complications (10–12). An equally important concern regarding HIV infection is the multifaceted complications fueled by substance use, such as cocaine, amphetamine, alcohol, and opioids. Opioids alone consist of several drugs of abuse, such as morphine, heroin, buprenorphine, oxycodone, fentanyl, methadone, and nalorphine (12). The interactions of these drugs with HIV-1 and their mechanisms of action are different for each drug. This review focuses on the role of long-term substance use that could contribute to the development and progression of HANDs arising from immune cell suppression, neuroinflammation, and neurodegeneration. Although these risk factors contribute to the development of HANDs, there are currently limited discussions regarding the interactive role of substance use that may contribute to risk factors of HIV transmission, infection, and non-adherence to cART regimens.
One of the common factors in substance use that contributes to neuro-AIDS development is undoubtedly damage to the BBB, which promotes the infiltration of infected cells into the brain for the progression of HANDs (2). Substance use, such as alcohol, is indeed a risk factor for HIV infection (13, 14) that contributes to the reduction in CD4+ T cells in HIV patients (15, 16). The comorbid effects of substance use in HANDs include depressive symptoms (17), memory loss (18), increased neuropathy (19), and an elevated mortality rate among HIV/AIDS patients (20). Thus, the BBB disruption, oxidative stress, cytokine release, and neuroinflammation mediated by HIV infection and substance use are thought to be due to the persistence of HANDs (21–24). Moreover, the cure/prevention of this fatal disease is hampered by the persistence of the HIV reservoir. The stability of the latent HIV-1 reservoir is harbored in the central memory CD4+ T cell genotype, and the integrated HIV DNA is harbored in the transitional memory CD4+ T cell genotype (25, 26). Together with these CD4+ T cells, the persistence of HIV-1 latency and HIV reservoirs in microglia, macrophages, and inflammatory monocytes within the restricted skull cavity appear to play a crucial role in the recurrence of HIV-1 self-renewal, reinfection upon cART withdrawal (27–29), and perhaps the chronic prevalence of HANDs. In fact, the use of highly suppressive cART has significantly controlled HIV/AIDS into a manageable chronic disease (2), but the prevalence of HANDs remains at 40–50% among people with HIV (30–32). In addition to the neuropsychological diagnosis of HANDs, it is also crucial to delve deeper into the specific brain region harboring the cell types that are explicitly linked to HIV resurgence and neuropathogenesis of HANDs. Understanding this knowledge gap could pave the path for uncovering the underlying molecular and cellular mechanisms and facilitate successful viral purging, thus making the cell types linked to HIV resurgence more accessible targets for cART.
2 Brain region affected in HANDs
Despite advancements in antiretroviral therapy (ART), the prevalence of HIV-associated neurocognitive disorders (HAND) and grey matter atrophy remains concerningly high among people with HIV (PWH), even with effective viral suppression and restored CD4+ counts (30, 32). Grey matter atrophy predominantly affects regions such as the frontal and temporal cortices, hippocampus, and basal ganglia. These changes are driven by viral proteins (e.g., Tat, gp120), chronic neuroinflammation, oxidative stress, and neuronal dysregulation. Substance use disorders (SUDs) further exacerbate these effects, intensifying neurodegeneration in vulnerable brain regions.
Understanding the specific brain regions and cell types affected by HAND and SUDs is critical for developing targeted therapies. Neuroimaging studies using powerful magnetic resonance imaging (MRI), computed tomography (CT) scanning, and positron emission tomography (PET) scans have demonstrated a strong correlation between regional brain atrophy and cognitive impairments, offering valuable insights into the pathophysiology of HAND. This complex interplay underscores the urgent need for a deeper understanding of the mechanisms driving selective grey matter atrophy and its interactions with comorbid SUDs. Such knowledge is essential for designing effective therapeutic interventions to mitigate HAND and its associated cognitive deficits.
Neuroimaging techniques have claimed to have the advantage of confirming the diagnosis of HANDs because they can exclude other diseases that can mimic them (33), but it is reasonable to state that the integration of information gathered from neuropsychiatric testing, CSF analytical markers, and neuroimaging findings is expected to provide a better diagnosis. Neuroimaging techniques have observed brain atrophy in all brain regions in PWH, which include the frontal, subcortical, temporal, parietal, occipital, and cerebellum, as shown by the various studies cited below. However, there are also some conflicting results, particularly regarding the effects of HIV infection on the brain volume of PWH. The findings include a reduced brain volume (34, 35), an increased brain volume (36), and no change in brain amygdala volume (37–39). To this end, Israel et al. (40) identify two distinct patterns of grey matter atrophy in HIV: frontal and anterior cingulate cortex (ACC) atrophy, which is associated with disease progression, and caudate/striatum atrophy, which is linked to neurocognitive impairments. These regions play distinct roles in the development of HIV-associated neurocognitive disorders (HANDs). Furthermore, this model generates several testable predictions and provides a framework for understanding HANDs within the broader context of behaviorally based models in people with HIV. A consistent finding is frontal or subcortical atrophy, observed in HIV-1 infection with/without substance use, such as cocaine, methamphetamine, alcohol, or opioid drugs in HANDs/SUDs (37, 40–46). Most neuroimaging studies have employed image- or coordinate-based MRI meta-analyses (47, 48). The former used full statistical maps and the latter used the reported coordinates of peak locations from the available original studies. The regions-of-interest-based colocalization likelihood estimation (CLE) approach was introduced to quantify atrophy at specific brain regions in PWH and people without HIV, which improves the limitations of these two voxel-based approaches (40). These studies showed that the two distinct atrophied brain regions affected in adults infected with HIV were the frontal subcortical and caudate/striatum, which were clearly differentiated from those without HIV-infected controls. The hallmark of frontal subcortical atrophy was attributed to the early development of HANDs, while caudate/striatum atrophy was attributed to the progression of neurocognitive impairment in HANDs. These findings were in line with those of other MRI-based findings that showed that HIV infection selectively damaged the cortex, even during the period in which patients received cART (49). Further, coordinate-based MRI meta-analysis showed that caudate/striatum atrophy in HANDs correlated with a decrease in cortical thickness and impairment of neurocognitive function (48–50). Although most brain regions are affected by HANDs/SUDs, it is reasonable to state that frontal subcortical and caudate/striatum may be the prominent brain regions selectively affected and atrophied by HANDs/SUDs. Supporting these neuroimaging observations, neuropathological examinations of postmortem brain tissues from individuals with HIV infection and substance use have confirmed significant brain damage in the frontal cortex, substantia nigra, and cerebellum. This damage is characterized by synaptic and dendritic density loss and pronounced neuroinflammation (51–53). Further studies have corroborated these findings, noting an increased number of infected macrophages and microglia in the perivascular space and the presence of multinucleated giant cells—hallmark features of HIV-associated neurocognitive disorders (HANDs) (54, 55).
3 Specific cell types in affected brain regions
It is pertinent to identify whether microglia, astrocytes, or infiltrated monocytes/macrophages actively participate in promoting the propagation and progression of atrophy in HANDs in these two distinct brain regions affected by HIV infection and substance use. Together with the role of astrocytes and oligodendrocytes, microglial cells are considered one of the key players in maintaining brain homeostasis and protecting the well-being of neuronal function in the context of HIV infection. Microglia are the brain’s resident innate immune cells that act as immune surveillance, phagocytose the unhealthy dying cells, and scavenge dead cell debris or toxic proteins for the healthy maintenance of the brain environment. As such, microglial cells are well fortified with immune defense mechanisms, well-orchestrated neurotransmitter molecules, receptors for cellular communication, and xenobiotic sensors for the elimination of toxic molecules in the brain (56). Microglia and infiltrating inflammatory monocytes/macrophages are likely key drivers of neuroinflammation and neurodegeneration in the context of HIV infection and substance use disorders. HIV infection disrupts microglial function, and these effects are exacerbated by substance use, resulting in the release of various inflammatory cytokines and subsequent neuroinflammation, ultimately contributing to neurodegeneration. It is now well established that without the direct involvement of oligodendrocytes (57), the HIV-infected microglia, astrocytes, and monocytes/macrophages serve as the viral reservoir, viral latency, and viral resurgence cells in the CNS (30, 31, 58, 59). These factors serve as a roadblock to the eradication of HIV/AIDS and effective prevention of HANDs; therefore, microglia and monocytes/macrophages have attracted considerable interest in the study of the persistence of HANDs in substance use disorders. The rationale is that HIV-infected innate immune cells have been shown to increase the production of inflammatory cytokines TNF-α, IL-1β, IL-4, L-6, IL-2, IFN-γ, and IL-8 (60–64) in addition to the release of neurotoxic HIV proteins (65–67). Similarly, the role of microglia in the brain emerged as novel targets in opioid use research because microglia play an important role in shaping neural circuitry (68). Thus, identification of the impaired neurotransmitter molecules in the infected microglia is likely to afford a better strategy to protect these neurotransmitters for improved microglia-neuron communication in HIV/SUDs.
On the question of whether substance use impacts HIV infection, neuropathogenesis, and latency reversal, there seem to be contradictory reports, mostly on the use of opioids. Opioids such as morphine, fentanyl, and methadone facilitate HIV infectivity by suppressing antiviral genes and altering immune responses, particularly in CD4+ T cells and monocyte-derived macrophages (MDMs). This includes the upregulation of pro-inflammatory cytokines like TNF-α and IL-6, which promote a microenvironment conducive to HIV replication (69–71). Methadone, commonly used in opioid replacement therapy, compromises T cell responses, weakening host resistance to HIV (72, 73). Additionally, opioids modulate CXCR4, a co-receptor for HIV-1 entry, enhancing viral susceptibility in CD4+ T cells (74). Chronic opioid use increases latent HIV reservoirs, with studies showing higher viral loads and latency reversal, particularly in heroin users who inject drugs (70, 75, 76). These clinical and epidemiological findings were also supported by in vitro studies that demonstrated that HIV reactivation was observed mostly in opioid users (77).
Buprenorphine, a partial opioid agonist, increases HIV infection in CD4+ T cells but does not reactivate latent HIV-1 in resting CD4+ T cells, likely due to its unique pharmacological profile (78). Opioids also exacerbate HIV-associated neuropathogenesis through neuroinflammation and glial activation. Astrocytes and microglia are key players in these processes, contributing to neuronal injury and amplifying neurodegeneration in HIV-infected individuals (70, 77). Despite substantial evidence supporting the detrimental effects of opioids on HIV, some studies suggest that their immunosuppressive properties might reduce inflammation-driven replication, highlighting the complexity of these interactions (79). If HIV-infected monocytes fail to migrate into the CNS, the virus may remain in peripheral circulation, increasing systemic viral load as these cells act as reservoirs and release new virions. These monocytes may migrate to other tissues, such as the liver, spleen, or lymph nodes, establishing reservoirs or causing local inflammation. Reduced migration to the CNS may limit reservoir establishment in the brain, potentially decreasing direct CNS inflammation but allowing systemic inflammation to affect the CNS indirectly. Recent studies indicate that opioid use does not directly affect HIV replication, latency, or reactivation but indirectly shapes the HIV reservoir, reducing the reactivation of HIV latency (80, 81).
On the other hand, methamphetamine is considered mostly stimulatory by activating CD4 T cells, which has been suggested to be a contributing factor to HIV replication and disease progression (82, 83). Notably, methamphetamine exposure contributed to enhanced migration of HIV-infected monocytes into the brain for the establishment of HIV reservoirs, persistence, and a source of inflammation (84). Moreover, poor adherence to cART regimens by methamphetamine users contributed to HIV disease progression (85). Furthermore, methamphetamine exposure has been shown to suppress T cell proliferation by altering the G-phase cell cycle, thereby impairing the innate immune system (86). Moreover, methamphetamine treatment significantly suppressed the levels of IL-2, IFN-γ, IL-10, and MCP-1 production in mice (87, 88).
In contrast, cannabinoid THC has contradictory effects on CD4 T cells and intermediate monocytes. The use of THC, which is considered anti-inflammatory, in HIV infection is associated with lower T cell activation, a potential protective mechanism against HIV replication (89, 90). However, clinical studies have shown a significant increase in intermediate monocyte populations and a notable decrease in TNF-α levels in these cell types in cannabis users compared to controls (91, 92). Conversely, a substantial increase in the levels of cytokines IL-6, IL-8, TNF-α, and IL-10 has been observed in cannabis smokers (93). Nevertheless, cannabis may alter immune responses in ways that are not always beneficial, particularly if it affects the immune system’s ability to control HIV replication or repair immune dysfunction. Cannabis has been shown to reduce T cell activation and TNF-α levels in monocytes, potentially providing a protective mechanism against HIV replication. However, clinical studies have also reported an increase in intermediate monocyte populations among cannabis users, highlighting the complex and potentially dual role of cannabinoids in modulating immune responses during HIV infection.
4 Identification of cell phenotypes
Neuroimaging techniques have proven to be powerful tools for analyzing region-specific brain atrophy and the detection of microglia phenotypes in affected regions of the brain in HIV/SUDs. Among these techniques, positron emission tomography (PET) and MRI are widely utilized for assessing microglial inflammation. PET scanning has emerged as a potential technique for investigating neuroinflammation resulting from microgliosis. PET enables the identification of microglial phenotypes and the assessment of alterations in functional proteins within the identified phenotypes at the cellular and molecular levels, respectively (94, 95). Precision is achieved via PET by using radiotracers that target the proinflammatory M1 phenotype and various biomarkers at the cellular and molecular levels, respectively.
Microglia-specific biomarkers used in PET imaging include colony-stimulating factor 1 receptor (CSF1R), mitochondrial 18 kDa translocator protein, an array of purinergic receptors (such as the P2X7 receptor, involved in immune signaling and NLRP3 activation), cannabinoid receptor type 2, cyclooxygenase-2 (COX-2), and reactive oxygen species (ROS). For example, the PET radiotracer [11C] CPPC targeting the CSF1R marker in microglia has demonstrated increased uptake in murine and non-primate models of neuroinflammation (96), murine models of Alzheimer’s disease (AD) (97), AD postmortem brain tissues (98), epilepsy seizure imaging (99), and PET imaging of microglial activation in patients with multiple sclerosis or cerebellar ataxia in HIV infection (100, 101). Similarly, the use of an 11C-CB184 PET scan revealed elevated uptake of 11C-CB184 in cerebellar microglia of a gp120 mouse model of HIV infection (102) and in humans with HIV (103). Specifically, CSF1R, TSPO, P2X7, and CB2 are particularly relevant for imaging microglial activation in neuroinflammatory conditions like HIV. COX-2 and ROS are broader indicators of neuroinflammation involving various cell types and activated microglia. Identifying the ability of PET imaging to identify neuronal cells, astrocytes, oligodendrocytes, and microglial phenotypes is a significant achievement, unraveling the cellular mechanisms associated with HANDs (46, 94, 95). A recent article emphasized the importance of neuroimaging analysis in people with HIV (PWH), suggesting that an improved, common neuroimaging approach may enhance our understanding of CNS complications in PWH (104), potentially refining the characterization of HAND persistence. Emerging advancements in PET neuroimaging techniques targeting activated microglia contribute to a better understanding of neurochemical-stimulated neurophysiological alterations in HANDs (46).
In addition, the advancement in capturing the presence of macrophage/microglia M1/M2 phenotypes, CD8+ T cell CD4dim CD8bright T cell phenotypes, and astrocyte A1/A2 phenotypes in the restricted skull cavity, perivascular, and meningeal space provides an important predictor of HIV infection/invasion in the brain. This comprehensive assessment may contribute to the understanding of neuroinflammation, neurodegeneration, and persistence of HANDs in PWH. In the context of HIV and substance use disorders, increased expression of M1 macrophage or microglia phenotypes, the astrocyte type A1 subset, and CD8+ T cells in these brain regions would suggest HIV/SUD-induced neuroinflammation in HANDs and other neurological diseases (105, 106). In contrast, elevated expression of M2 microglia or macrophage phenotypes (107, 108), the astrocyte type A2 subset (109, 110), and the CD8+ T cell CD4dim CD8bright subset (111–113) would indicate an anti-inflammatory response to HIV neuro-invasion.
While HIV primarily infects and activates proinflammatory cell phenotypes in microglia and monocytes/macrophages, the question of astrocyte infection has been debated. Earlier studies demonstrated HIV infection in astrocytes by observing the integration of HIV-1 DNA in the astrocyte genome (114, 115). Most recent studies have concluded that astrocytes are not directly infected by HIV-1 in the brain but are activated by the virus. HIV infection in the brain occurs primarily in microglia and migrated monocytes/macrophages (116). It was previously believed that HIV infects only differentiated macrophages, not monocytes (117–119); however, recent findings have demonstrated that HIV can infect monocytes, which also act as reservoirs in the brain (120, 121). In light of these findings, it is safe to assert that neuroimaging techniques, particularly those utilizing PET radioligand binding methods, play a crucial role in assessing glial and monocyte inflammatory phenotypes during neuroinflammation (29, 122, 123). Therefore, delving into the underlying molecular mechanisms involved in inflammatory phenotypes becomes essential, which may offer insights into the supportive infection of bystander neuroimmune cells and potentially contribute to the severity of HANDs.
5 Molecular events in HIV/SUDs
While noninvasive neuroimaging techniques offer insights into immune cell response and longitudinal tracking in specific brain regions, understanding the molecular mechanistic events that may occur within the infected cells or activated cells surpasses the capabilities of neuroimaging techniques. It is well established that infected microglia or activated astrocytes produce a cascade of inflammatory cytokines, chemokines, ROS, and toxic HIV proteins (60–67). Concurrently, a decrease in anti-inflammatory cytokines and interferons is observed in both HIV infection (124, 125) and substance use disorders, such with alcohol, opioids, cannabinoids, and other drugs of abuse (126–128). Consequently, the production of proinflammatory agents outweighs the retention of anti-inflammatory molecules in HIV-infected or substance-exposed microglia. Therefore, it is crucial to understand the balance of these functional molecules within these affected cells during the inflammatory process.
Microglia, the brain’s innate immune cells, are equipped with immune surveillance, phagocytic scavenging capabilities, and functional receptor proteins that are essential for various neurophysiological functions. Some of these molecules that are predominantly expressed in microglia include ATP-dependent ionotropic purinergic receptor (P2X7R) (129, 130), metabotropic purinergic receptor (P2Y12R) (131, 132), colony-stimulating factor 1 receptor (CSF1R) (96, 133), cyclooxygenase-1/2 (134, 135), triggering receptor expressed in myeloid cells (TREM1) (136–138), cannabinoid type 2 receptor (CB2R) (139, 140), and γ-aminobutyric acid type B (GABA-B) receptors (141, 142). These biomarker receptors are mostly expressed in microglia and regulate cell proliferation, survival, differentiation, activation, and neurotransmission, and they also serve as sensors for apoptotic cell death. It should be noted that the above-cited biomarkers are not just limited to microglia; they are also expressed in other brain cell types, including monocytes/macrophages.
Recent findings highlighted that activation of microglia GABA-B receptor and CSF1R by astrocytic GABA release plays an important role in the microglia-neuron communication pathway (143, 144). While these microglia receptors remain inactive under normal physiological conditions, their significant up-regulation occurs during HIV-associated neuroinflammation (137, 145, 146). The activation of microglia and its receptors undoubtedly plays a vital role in the progression of neuroinflammation in HANDs and substance use disorders, such as with the long-term use of cocaine, morphine, amphetamine, alcohol, and opioids (146–149). Radiotracers that target these microglia receptors as biomarkers for inflammatory and neurodegenerative diseases have garnered considerable interest in the longitudinal visualization of disease progression (96, 128, 132, 135, 138). Beyond targeting these functional receptors, it is imperative to discuss the involvement of epigenomic, genomic, and transcriptomic factors in HIV/SUD-activated microglia that may contribute to the development of neuroinflammatory diseases.
6 Impact of epigenetics in HANDs
Recent interest has surged regarding research into understanding the effects of microglial epigenetic and transcriptomic modifications on the pathophysiology and neuropathogenesis of substance use disorders (69). Notably, these epigenetic or transcriptomic landscape modifications in microglial phenotypes exert control over neurophysiological functions and offer potential molecular mechanisms in neurological diseases (150, 151). This discussion focuses on the epigenetic modifications occurring in brain-resident microglia, which appear to exhibit a distinct epigenome, transcriptome, and chromatin landscape that differs from engrafted parenchymal brain macrophages (150), ex vivo cell cultures (152, 153), or microglia during the neurodevelopmental stage (154–156). It is rather challenging to distinguish between microglia and migrated macrophages in the brain because of the shared lineage and similar functional receptors (157, 158). To this end, a recently identified microglia-specific biomarker, transmembrane protein 119 (TMEM119), appears to be a promising authentic microglia-specific biomarker for cell phenotyping, as demonstrated by an investigation of transcriptional identity and developmental heterogeneity in single-cell sequencing (159–163). Recently, the chromatin landscape for HIV-1 integration determinants in microglia was identified at the CTCF-enriched domain (164), and region-specific epigenetic and transcriptomic alterations induced by HIV infection and substance use disorders in microglia were observed (165). It has been reported that HIV infection and substance use can modulate DNA methylation, histone modification, and chromatin conformation in microglia, with the underlying mechanisms of epigenetic alterations in human disease (164–168).
Advancements in various epigenetic modifications have led to a new therapeutic approach targeting the inhibition of these alterations as a potential cure for HIV/AIDS (169). Strategies include the “shock and kill” approach, aiming to shock the provirus into expression and then eliminate the cells by the immune system or neutralize them with antibodies (170); stimulating latently infected cells and challenging them with epigenetic inhibitors of HDACs, DNMTs, or combinations (171–173); and the “lock-and-block” approach, which focuses on silencing provirus reactivation using inhibitors of Tat (174), mTOR inhibitors (175), or inhibitors of an epigenetic reader BRD4 (176).
Epigenetic interventions for an HIV cure primarily revolve around the inhibition of lysine methylation or acetylation, given their demonstrated requirement for maintaining HIV-1 latency (177, 178). The role of N-terminal acetylation, catalyzed by N-terminal acetyltransferase (NAT), has been underexplored in HIV infection and substance use disorders. However, recent findings highlighted the critical role in regulating chromatin function and control of various gene expressions that influence functional protein dysregulation (179, 180). N-terminal acetylation has been implicated in HIV-host interactions and mitochondrial epigenetic modification as a cause of HIV neuropathogenesis (181). Activation of N-α-acetyltransferase 60 (NAT60) has been shown to promote influenza A viral infection, which inhibited the interferon-α signaling pathway (182), while NAT-B-mediated N-terminal acetylation has been shown to inactivate influenza virus PA-X and viral polymerase activity (183). Given the critical regulatory roles and emerging impact of N-terminal acetylation in health and disease, there is undoubtedly considerable interest and prospects for research exploring the pivotal role of NAT and its inhibitors, in particular, unraveling the epigenetic regulation of neurocognitive functional alterations in HANDs/SUDs.
7 Impact of antiretroviral therapy in HANDs
The introduction of highly active combination antiretroviral therapy (cART) has significantly transformed HIV/AIDS from a fatal to manageable chronic disease. However, the impact of cART on the prevalence of HANDs remains a subject of ongoing debate. The Frascati subclassification of HANDs encompasses asymptomatic neurocognitive impairment (ANI), minor neurocognitive disorder (MND/HAND), and severe HIV-associated dementia (HAD) (184). In the pre-cART era, HAD was associated with high viral loads and CD4 counts less than 200 cells/μL, while HANDs developed in patients with normal CD4 counts (30). In the cART era, there has been a substantial reduction in HAD, but the prevalence of HANDs remains noteworthy among people with HIV (30–32). Both HANDs and HAD share the same root cause, that is, HIV infection followed by chronic inflammation and neurotoxicity. The distinguishing factor appears to be viremia and CD4 counts, which are effectively managed in the cART era. Mild neurocognitive dysfunction in HANDs persists, suggesting that while cART effectively inhibits HIV replication, it may not entirely counteract the effects of shed neurotoxic viral proteins, secreted inflammatory agents, or ongoing oxidative stress in HIV patients. This persistent dysfunction may also be attributed to the “legacy effect,” where neuropsychological impairments result from brain damage caused by HIV infection during the pre-cART era, adverse side effects of cART, or comorbid substance use disorders (185, 186). Despite its success, cART has limitations in addressing the multifaceted aspects of HANDs. Although it effectively suppresses viral replication, cART exerts beneficial and adverse effects on neurocognitive function in HANDs. However, during the pre-cART era, HIV infection often led to more severe immune system damage, and many people with HIV developed neurological impairments such as HANDs due to the lack of effective viral suppression (10); however, this did not occur without HIV in people with SUDs. We propose that chronic inflammation, driven by persistent HIV resurgence and compounded by the adverse impact of cART and substance use disorders, contributes to the persistence of mild neurocognitive dysfunction in HANDs. However, there is limited evidence of neurocognitive effects or brain atrophy from PrEP use, as most studies focus on its efficacy for HIV prevention. Neurological impacts have been observed in ART users, such as cognitive impairments and brain changes, but these findings are not well documented in PrEP users without HIV.
Concerns arise from the broad spectrum of adverse effects associated with cART, including renal toxicity, mitochondrial metabolic impairment, gastrointestinal symptoms, cardiovascular effects, hypersensitivity, skin reactions, insomnia, and neuropsychiatric symptoms (187–189). These side effects often lead to interruptions in cART adherence by people with HIV (190). It is well documented in the literature that long-term cART use alone is associated with reduced levels of brain-derived neurotrophic factor (BDNF), astrogliosis, and increased release of proinflammatory cytokines (IL-1β and TNF-α), suggesting potential contributions to HAND development even in the absence of HIV infection (191). The co-occurrence of substance abuse in HIV infection further complicates cART efficacy and exacerbates HAND progression (54). The co-occurrence of substance abuse with HIV infection further complicates cART efficacy and exacerbates HAND progression (192). Regarding the ability of cART to penetrate the blood-brain barrier, evidence suggests that while cART affects brain functions, supporting its potential penetration into the CNS, its pharmacokinetics vary. Some cART drugs are metabolized quickly within the CNS, whereas others, particularly those with high CNS penetration effectiveness (CPE) scores, may persist longer. Furthermore, cART metabolites or low concentrations of these drugs can remain in the CNS for weeks to months post treatment. However, their functional effects, such as residual toxicity or viral suppression, may differ. Adverse effects on BDNF levels, induction of astrogliosis, upregulation of proinflammatory cytokines, and resurgence of HIV in microglia upon cART interruption reinforce the complex interplay between cART, HIV, and HANDs. The persistence of HIV infection in CNS reservoir cells and chronic inflammation may significantly contribute to HAND development. To effectively manage HANDs in the context of substance use, it is crucial to target HIV reservoir cells in the CNS with improved cART to prevent HIV resurgence. HIV reservoirs in the CNS primarily include microglia and astrocytes, while neurons are indirectly affected by inflammation and viral proteins. Improved long-acting cART aims to enhance CNS penetration and reduce dosing frequency. However, it faces challenges with HIV latency and potential resurgence, increasing the risk of neuroinflammation and cognitive decline. Additionally, exploring adjuvant therapeutic approaches to mitigate chronic neuroinflammation and neurotoxicity resulting from cART adverse effects, toxic viral proteins, secreted proinflammatory agents, and continuous oxidative stress remains a critical avenue for future research.
Current understandings of CNS HIV reservoirs focus predominantly on microglia, but this perspective is limited. Astrocytes also play a critical role as reservoirs, contributing to viral persistence and CNS dysfunction. While neurons are not directly infected, they experience indirect effects such as neurotoxicity, inflammation, and oxidative stress. Expanding research beyond microglia is essential to fully understand and address the multifaceted impact of HIV in the CNS.
8 Concluding remarks and future perspectives
In the complex landscape of HANDs, the coexistence of SUDs adds layers of complexity to the neuropathogenesis, potentially through oxidative stress and prolonged inflammatory processes driven by microglia phenotypes and migration of HIV-infected monocytes in specific brain regions. In the era of combination antiretroviral therapy (cART), it is crucial to delve into the brain-region-specific and cell-type-specific molecular mechanisms triggering chronic inflammation to develop preventive approaches to each of the mechanistic events associated with the persistence of HANDs, as described in Figure 1. Neuroimaging techniques have provided evidence that frontal subcortical and caudate striatum atrophies have been linked to the initial development of HIV disease and subsequent neurocognitive impairment in HANDs. Neuroimaging methods are believed to have the advantage of differentiating HANDs from other diseases that might mimic their symptoms, even in the presence of cART. Therefore, improvements to combined imaging tools offer hope for the accurate identification of specific brain regions affected in HANDs/SUDs and the specific types of cells that are involved in the progression of chronic inflammation within the identified brain region(s).
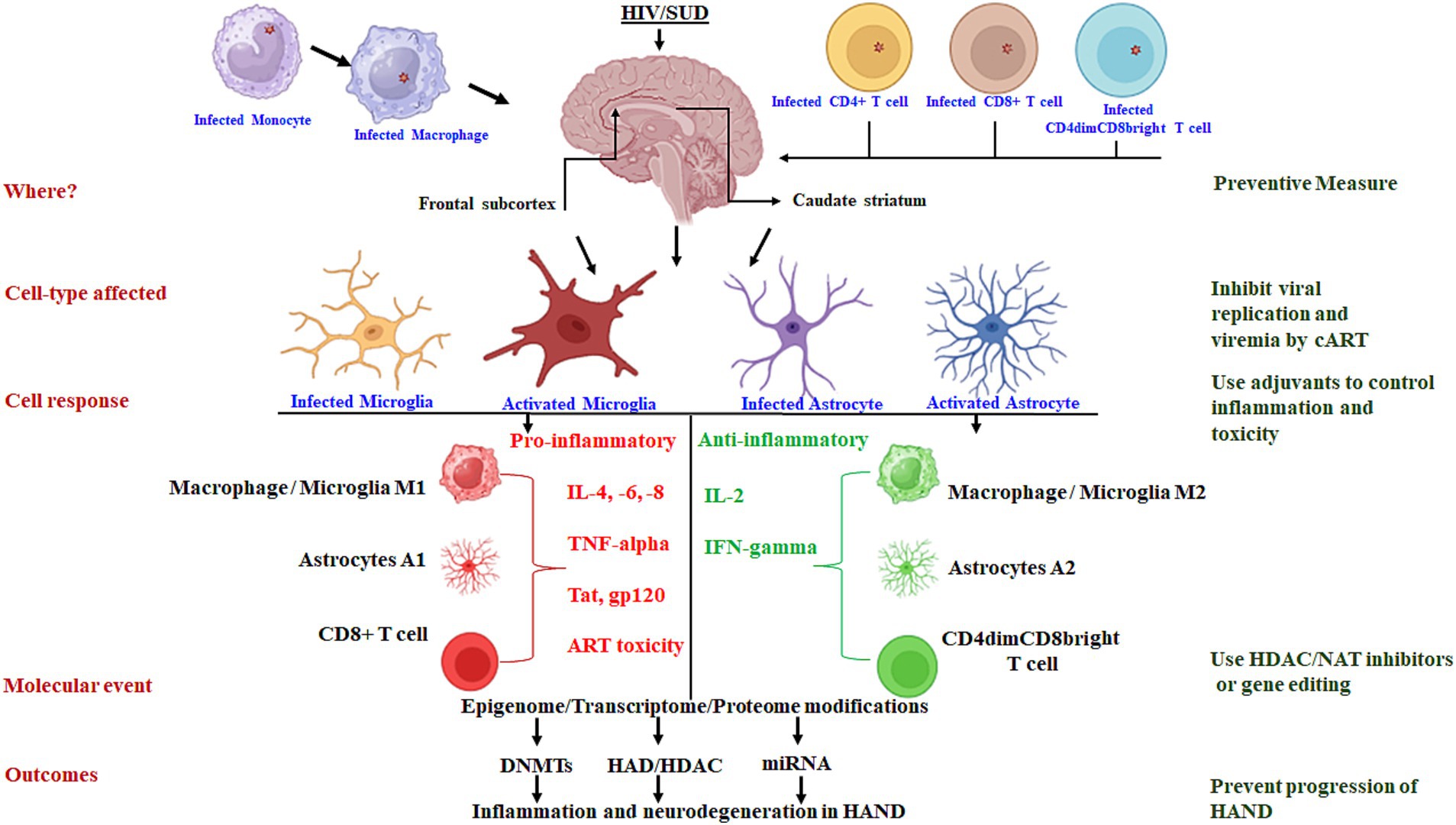
Figure 1. Schematic presentation of the molecular mechanisms driving chronic inflammation in brain-region-specific and cell-type-specific contexts: targets for preventive approaches.
Microglia, as brain-resident cells, emerge as key players in CNS HIV infection, serving as viral reservoirs and potential sources of inflammation for the propagation of chronic neuroinflammation. Recent findings showed that substance use exacerbates immune activation, neuroinflammation, and mitochondrial dysfunction, and increases HIV replication, which are intimately linked to the pathology of HANDs. Thus, the identification of inflammatory microglia phenotypes in specific brain areas through PET imaging and targeting of microglial-specific biomarkers hold promise in understanding HAND prevalence in people with HIV. As such, microglia are significant contributors to HAND persistence in the context of substance use. Thus, exploring the molecular mechanisms and the contributing factors of substance use in HAND prevalence through microglial epigenetic modifications studies may be an important step to unravel the inflammatory phenotypes for inducing neurophysiological defects. The distinct epigenome, transcriptome, and chromatin landscape of microglia is of prime research interest. In particular, the epigenetic regulation and reprogramming of the “innate immunity” of brain-resident cell microglia and monocyte-differentiated macrophage brain homing cells can address the knowledge gap in the development and progression of HANDs. In this regard, the focus of research will be to evaluate whether interruption and re-adherence of cART in chronic HIV infection will impact trained/tolerized microglia/macrophages more during epigenetic modifications and metabolic switch, which are the two pillars of reprogramming innate immunity. Interest in epigenetic interventions for a HIV cure, such as inhibiting modifications, “shock and kill,” or reprogramming of innate immunity strategies, has increased with recent findings that highlight the crucial role of mitochondrial N-terminal acetylation in HIV-host interactions.
On the cART front, new antiviral drugs and immune activation therapies specifically targeting the latently infected cells (microglia/macrophages/monocytes that serve as HIV reservoirs) could bridge the current knowledge gap. Such drug and therapy advancements could include the formulation of improved antiretroviral drugs that can easily penetrate the blood–brain barrier, tissue-specific cART delivery, and purging of latently infected cells from anatomical HIV reservoirs. Despite successful viral load suppression by cART, the persistence of latent HIV reservoirs in the CNS impedes the possibility of HIV eradication. Chronic neuroinflammation and neurotoxicity from both latently infected microglia HIV reservoirs and cART adverse effects are suspected to be the factors contributing to HAND persistence. In conclusion, until the development of a new, improved, and less toxic antiviral drug, there is an urgent need for neuroprotective and anti-inflammatory adjuvant therapies along with cART regimens to control HAND progression in chronic HIV disease.
Author contributions
JH: Writing – original draft, Writing – review & editing, Conceptualization. SM: Project administration, Writing – review & editing, Data curation. HI: Project administration, Formal analysis, Writing – review & editing. TS: Project administration, Conceptualization, Funding acquisition, Investigation, Supervision, Writing – original draft, Writing – review & editing.
Funding
The author(s) declare that financial support was received for the research, authorship, and/or publication of this article. The present study is supported by a grant from the National Institute of Health//National Institute of Drug Abuse (NIDA): DA044872.
Acknowledgments
BioRender.com was used to create the figures.
Conflict of interest
The authors declare that the research was conducted in the absence of any commercial or financial relationships that could be construed as a potential conflict of interest.
Generative AI statement
The authors declare that no Gen AI was used in the creation of this manuscript.
Publisher’s note
All claims expressed in this article are solely those of the authors and do not necessarily represent those of their affiliated organizations, or those of the publisher, the editors and the reviewers. Any product that may be evaluated in this article, or claim that may be made by its manufacturer, is not guaranteed or endorsed by the publisher.
Abbreviations
HIV, Human immunodeficiency virus; SUDs, Substance use disorders; HANDs, HIV-associated neurocognitive disorders; cART, Combined antiretroviral therapy; BBB, Blood-brain barrier; PET, Positron emission tomography; CNS, Central nervous system; NAT, N-terminal acetyltransferase.
References
1. Price, RW, and Spudich, S. Antiretroviral therapy and central nervous system HIV type 1 infection. J Infect Dis. (2008) 197:S294–306. doi: 10.1086/533419
2. McArthur, JC, Brew, BJ, and Nath, A. Neurological complications of HIV infection. Lancet Neurol. (2005) 4:543–55. doi: 10.1016/S1474-4422(05)70165-4
3. Kaul, M, Garden, GA, and Lipton, SA. Pathways to neuronal injury and apoptosis in HIV-associated dementia. Nature. (2001) 410:988–94. doi: 10.1038/35073667
4. Nath, A, and Sacktor, N. Influence of highly active antiretroviral therapy on persistence of HIV in the central nervous system. Curr Opin Neurol. (2006) 19:358–61. doi: 10.1097/01.wco.0000236614.51592.ca
5. Eisfeld, C, Reichelt, D, Evers, S, and Husstedt, I. CSF penetration by antiretroviral drugs. CNS Drugs. (2013) 27:31–55. doi: 10.1007/s40263-012-0018-x
6. Varatharajan, L, and Thomas, SA. The transport of anti-HIV drugs across blood-CNS interfaces: summary of current knowledge and recommendations for further research. Antivir Res. (2009) 82:A99–A109. doi: 10.1016/j.antiviral.2008.12.013
7. Strazielle, N, and Ghersi-Egea, JF. Factors affecting delivery of antiviral drugs to the brain. Rev Med Virol. (2005) 15:105–33. doi: 10.1002/rmv.454
8. Gibbs, JE, and Thomas, SA. The distribution of the anti-HIV drug, 2′3′-dideoxycytidine (ddC), across the blood-brain and blood-cerebrospinal fluid barriers and the influence of organic anion transport inhibitors. J Neurochem. (2002) 80:392–404. doi: 10.1046/j.0022-3042.2001.00711.x
9. Letendre, S, Marquie-Beck, J, Capparelli, E, Best, B, Clifford, D, Collier, AC, et al. Validation of the CNS penetration-effectiveness rank for quantifying antiretroviral penetration into the central nervous system. Arch Neurol. (2008) 65:65–70. doi: 10.1001/archneurol.2007.31
10. Yuan, NY, and Kaul, M. Beneficial and adverse effects of cART affect neurocognitive function in HIV-1 infection: balancing viral suppression against neuronal stress and injury. J Neuroimmune Pharmacol. (2021) 16:90–112. doi: 10.1007/s11481-019-09868-9
11. Peluso, MJ, Ferretti, F, Peterson, J, Lee, E, Fuchs, D, Boschini, A, et al. Cerebrospinal fluid HIV escape associated with progressive neurologic dysfunction in patients on antiretroviral therapy with well controlled plasma viral load. AIDS. (2012) 26:1765–74. doi: 10.1097/QAD.0b013e328355e6b2
12. Ren, M, and Lotfipour, S. The role of the gut microbiome in opioid use. Behav Pharmacol. (2020) 31:113–21. doi: 10.1097/FBP.0000000000000538
13. Freiberg, MS, McGinnis, K, Kraemer, K, Samet, JH, Conigliaro, J, Curtis Ellison, R, et al. The association between alcohol consumption and prevalent cardiovascular diseases among HIV-infected and HIV-uninfected men. J Acquir Immune Defic Syndr. (2010) 53:247–53. doi: 10.1097/QAI.0b013e3181c6c4b7
14. Clary, CR, Guidot, DM, Bratina, MA, and Otis, JS. Chronic alcohol ingestion exacerbates skeletal muscle myopathy in HIV-1 transgenic rats. AIDS Res Ther. (2011) 8:30. doi: 10.1186/1742-6405-8-30
15. Baum, MK, Rafie, C, Lai, S, Sales, S, Page, JB, and Campa, A. Alcohol use accelerates HIV disease progression. AIDS Res Hum Retrovir. (2010) 26:511–8. doi: 10.1089/aid.2009.0211
16. Shuper, PA, Neuman, M, Kanteres, F, Baliunas, D, Joharchi, N, and Rehm, J. Causal considerations on alcohol and HIV/AIDS—a systematic review. Alcohol Alcohol. (2010) 45:159–66. doi: 10.1093/alcalc/agp091
17. Sullivan, LE, Goulet, JL, Justice, AC, and Fiellin, DA. Alcohol consumption and depressive symptoms over time: a longitudinal study of patients with and without HIV infection. Drug Alcohol Depend. (2011) 117:158–63. doi: 10.1016/j.drugalcdep.2011.01.014
18. Fama, R, Rosenbloom, MJ, Nichols, BN, Pfefferbaum, A, and Sullivan, EV. Working and episodic memory in HIV infection, alcoholism, and their comorbidity: baseline and 1-year follow-up examinations. Alcohol Clin Exp Res. (2009) 33:1815–24. doi: 10.1111/j.1530-0277.2009.01020.x
19. Ferrari, LF, and Levine, JD. Alcohol consumption enhances antiretroviral painful peripheral neuropathy by mitochondrial mechanisms. Eur J Neurosci. (2010) 32:811–8. doi: 10.1111/j.1460-9568.2010.07355.x
20. DeLorenze, GN, Weisner, C, Tsai, AL, Satre, DD, and Quesenberry Jr, CP. Excess mortality among HIV-infected patients diagnosed with substance use dependence or abuse receiving care in a fully integrated medical care program. Alcohol Clin Exp Res. (2011) 35:203–10. doi: 10.1111/j.1530-0277.2010.01335.x
21. Potula, R, Haorah, J, Knipe, B, Leibhart, J, Chrastil, J, Heilman, D, et al. Alcohol abuse enhances neuroinflammation and impairs immune responses in an animal model of human immunodeficiency virus-1 encephalitis. Am J Pathol. (2006) 168:1335–44. doi: 10.2353/ajpath.2006.051181
22. Huang, Y, Zhao, L, Jia, B, Wu, L, Li, Y, Curthoys, N, et al. Glutaminase dysregulation in HIV-1-infected human microglia mediates neurotoxicity: relevant to HIV-1-associated neurocognitive disorders. J Neurosci. (2011) 31:15195–204. doi: 10.1523/JNEUROSCI.2051-11.2011
23. Persidsky, Y, Heilman, D, Haorah, J, Zelivyanskaya, M, Persidsky, R, Weber, GA, et al. Rho-mediated regulation of tight junctions during monocyte migration across the blood-brain barrier in HIV-1 encephalitis (HIVE). Blood. (2006) 107:4770–80. doi: 10.1182/blood-2005-11-4721
24. Persidsky, Y, Ho, W, Ramirez, SH, Potula, R, Abood, ME, Unterwald, E, et al. HIV-1 infection and alcohol abuse: neurocognitive impairment, mechanisms of neurodegeneration and therapeutic interventions. Brain Behav Immun. (2011) 25:S61–70. doi: 10.1016/j.bbi.2011.03.001
25. Chomont, N, El-Far, M, Ancuta, P, Trautmann, L, Procopio, FA, Yassine-Diab, B, et al. HIV reservoir size and persistence are driven by T cell survival and homeostatic proliferation. Nat Med. (2009) 15:893–900. doi: 10.1038/nm.1972
26. Vandergeeten, C, Fromentin, R, DaFonseca, S, Lawani, MB, Sereti, I, Lederman, MM, et al. Interleukin-7 promotes HIV persistence during antiretroviral therapy. Blood. (2013) 121:4321–9. doi: 10.1182/blood-2012-11-465625
27. Wallet, C, de Rovere, M, van Assche, J, Daouad, F, de Wit, S, Gautier, V, et al. Microglial cells: the main HIV-1 reservoir in the brain. Front Cell Infect Microbiol. (2019) 9:362. doi: 10.3389/fcimb.2019.00362
28. Donoso, M, D’Amico, D, Valdebenito, S, Hernandez, CA, Prideaux, B, and Eugenin, EA. Identification, quantification, and characterization of HIV-1 reservoirs in the human brain. Cells. (2022) 11:2379. doi: 10.3390/cells11152379
29. Veenstra, M, León-Rivera, R, Li, M, Gama, L, Clements, JE, and Berman, JW. Mechanisms of CNS viral seeding by HIV+ CD14+ CD16+ monocytes: establishment and reseeding of viral reservoirs contributing to HIV-associated neurocognitive disorders. mBio. (2017) 8:e01280. doi: 10.1128/mBio.01280-17
30. Heaton, RK, Clifford, DB, Franklin DR Jr,, Woods, SP, Ake, C, Vaida, F, et al. HIV-associated neurocognitive disorders persist in the era of potent antiretroviral therapy: CHARTER study. Neurology. (2010) 75:2087–96. doi: 10.1212/WNL.0b013e318200d727
31. Heaton, RK, Franklin, DR, Ellis, RJ, McCutchan, J, Letendre, SL, Leblanc, S, et al. HIV-associated neurocognitive disorders before and during the era of combination antiretroviral therapy: differences in rates, nature, and predictors. J Neurovirol. (2011) 17:3–16. doi: 10.1007/s13365-010-0006-1
32. Sacktor, N, Skolasky, RL, Seaberg, E, Munro, C, Becker, JT, Martin, E, et al. Prevalence of HIV-associated neurocognitive disorders in the multicenter AIDS cohort study. Neurology. (2016) 86:334–40. doi: 10.1212/WNL.0000000000002277
33. Vastag, Z, Fira-Mladinescu, O, and Rosca, EC. HIV-associated neurocognitive disorder (HAND): obstacles to early neuropsychological diagnosis. Int J Gen Med. (2022) 15:4079–90. doi: 10.2147/IJGM.S295859
34. Ances, BM, Ortega, M, Vaida, F, Heaps, J, and Paul, R. Independent effects of HIV, aging, and HAART on brain volumetric measures. J Acquir Immune Defic Syndr. (2012) 59:469–77. doi: 10.1097/QAI.0b013e318249db17
35. Spies, G, Ahmed-Leitao, F, Fennema-Notestine, C, Cherner, M, and Seedat, S. Effects of HIV and childhood trauma on brain morphometry and neurocognitive function. J Neurovirol. (2016) 22:149–58. doi: 10.1007/s13365-015-0379-2
36. Clark, US, Walker, KA, Cohen, RA, Devlin, KN, Folkers, AM, Pina, MJ, et al. Facial emotion recognition impairments are associated with brain volume abnormalities in individuals with HIV. Neuropsychologia. (2015) 70:263–71. doi: 10.1016/j.neuropsychologia.2015.03.003
37. Jernigan, TL, Gamst, AC, Archibald, SL, Fennema-Notestine, C, Mindt, MR, Marcotte, TL, et al. Effects of methamphetamine dependence and HIV infection on cerebral morphology. Am J Psychiatry. (2005) 162:1461–72. doi: 10.1176/appi.ajp.162.8.1461
38. Ragin, AB, Du, H, Ochs, R, Wu, Y, Sammet, CL, Shoukry, A, et al. Structural brain alterations can be detected early in HIV infection. Neurology. (2012) 79:2328–34. doi: 10.1212/WNL.0b013e318278b5b4
39. Wade, BS, Valcour, V, Busovaca, E, Esmaeili-Firidouni, P, Joshi, SH, Wang, Y, et al. Subcortical shape and volume abnormalities in an elderly HIV+ cohort. Proc SPIE Int Soc Opt Eng. (2015) 9417:94171S. doi: 10.1117/12.2082241
40. Israel, SM, Hassanzadeh-Behbahani, S, Turkeltaub, PE, Moore, DJ, Ellis, RJ, and Jiang, X. Different roles of frontal versus striatal atrophy in HIV-associated neurocognitive disorders. Hum Brain Mapp. (2019) 40:3010–26. doi: 10.1002/hbm.24577
41. Nestler, EJ. The neurobiology of cocaine addiction. Sci Pract Perspect. (2005) 3:4–10. doi: 10.1151/spp05314
42. Volkow, ND, Wang, GJ, Fowler, JS, Tomasi, D, and Telang, F. Addiction: beyond dopamine reward circuitry. Proc Natl Acad Sci USA. (2011) 108:15037–42. doi: 10.1073/pnas.1010654108
43. Coughlin, JM, Wang, Y, Ma, S, Yue, C, Kim, PK, Adams, AV, et al. Regional brain distribution of translocator protein using [11C]DPA-713 PET in individuals infected with HIV. J Neurovirol. (2014) 20:219–32. doi: 10.1007/s13365-014-0239-5
44. Becker, JT, Sanders, J, Madsen, SK, Ragin, A, Kingsley, L, Maruca, V, et al. Subcortical brain atrophy persists even in HAART-regulated HIV disease. Brain Imaging Behav. (2011) 5:77–85. doi: 10.1007/s11682-011-9113-8
45. Sinharay, S, and Hammoud, DA. Brain PET imaging: value for understanding the pathophysiology of HIV-associated neurocognitive disorder (HAND). Curr HIV/AIDS Rep. (2019) 16:66–75. doi: 10.1007/s11904-019-00419-8
46. Vera, JH, Ridha, B, Gilleece, Y, Amlani, A, Thorburn, P, and Dizdarevic, S. PET brain imaging in HIV-associated neurocognitive disorders (HAND) in the era of combination antiretroviral therapy. Eur J Nucl Med Mol Imaging. (2017) 44:895–902. doi: 10.1007/s00259-016-3602-3
47. Salimi-Khorshidi, G, Smith, SM, Keltner, JR, Wager, TD, and Nichols, TE. Meta-analysis of neuroimaging data: a comparison of image-based and coordinate-based pooling of studies. NeuroImage. (2009) 45:810–23. doi: 10.1016/j.neuroimage.2008.12.039
48. Tench, CR, Tanasescu, R, Constantinescu, CS, Auer, DP, and Cottam, WJ. Easy to interpret coordinate based meta-analysis of neuroimaging studies: analysis of brain coordinates (ABC). J Neurosci Methods. (2022) 372:109556. doi: 10.1016/j.jneumeth.2022.109556
49. Thompson, PM, Dutton, RA, Hayashi, KM, Toga, AW, Lopez, OL, Aizenstein, HJ, et al. Thinning of the cerebral cortex visualized in HIV/AIDS reflects CD4+ T lymphocyte decline. Proc Natl Acad Sci USA. (2005) 102:15647–52. doi: 10.1073/pnas.0502548102
50. Eickhoff, SB, Laird, AR, Grefkes, C, Wang, LE, Zilles, K, and Fox, PT. Coordinate-based activation likelihood estimation meta-analysis of neuroimaging data: a random-effects approach based on empirical estimates of spatial uncertainty. Hum Brain Mapp. (2009) 30:2907–26. doi: 10.1002/hbm.20718
51. Ketzler, S, Weis, S, Haug, H, and Budka, H. Loss of neurons in the frontal cortex in AIDS brains. Acta Neuropathol. (1990) 80:92–4. doi: 10.1007/BF00294228
52. Reyes, MG, Faraldi, F, Senseng, CS, Flowers, C, and Fariello, R. Nigral degeneration in acquired immune deficiency syndrome (AIDS). Acta Neuropathol. (1991) 82:39–44. doi: 10.1007/BF00310921
53. Graus, F, Ribalta, T, Abos, J, Alom, J, Cruz-Sanchez, F, Mallolas, J, et al. Subacute cerebellar syndrome as the first manifestation of AIDS dementia complex. Acta Neurol Scand. (1990) 81:118–20. doi: 10.1111/j.1600-0404.1990.tb00945.x
54. Sanchez, AB, and Kaul, M. Neuronal stress and injury caused by HIV-1, cART and drug abuse: converging contributions to HAND. Brain Sci. (2017) 7:25. doi: 10.3390/brainsci7030025
55. Gonzalez-Scarano, F, and Martin-Garcia, J. The neuropathogenesis of AIDS. Nat Rev Immunol. (2005) 5:69–81. doi: 10.1038/nri1527
56. Stolero, N, and Frenkel, D. The dialog between neurons and microglia in Alzheimer’s disease: the neurotransmitters view. J Neurochem. (2021) 158:1412–24. doi: 10.1111/jnc.15262
57. Nath, A. Eradication of human immunodeficiency virus from brain reservoirs. J Neurovirol. (2015) 21:227–34. doi: 10.1007/s13365-014-0291-1
58. Minagar, A, Shapshak, P, Fujimura, R, Ownby, R, Heyes, M, and Eisdorfer, C. The role of macrophage/microglia and astrocytes in the pathogenesis of three neurologic disorders: HIV-associated dementia, Alzheimer disease, and multiple sclerosis. J Neurol Sci. (2002) 202:13–23. doi: 10.1016/S0022-510X(02)00207-1
59. Li, GH, Henderson, L, and Nath, A. Astrocytes as an HIV reservoir: mechanism of HIV infection. Curr HIV Res. (2016) 14:373–81. doi: 10.2174/1570162X14666161006121455
60. Ngcobo, S, Molatlhegi, RP, Osman, F, Ngcapu, S, Samsunder, N, Garrett, NJ, et al. Pre-infection plasma cytokines and chemokines as predictors of HIV disease progression. Sci Rep. (2022) 12:2437. doi: 10.1038/s41598-022-06532-w
61. Kedzierska, K, and Crowe, SM. Cytokines and HIV-1: interactions and clinical implications. Antivir Chem Chemother. (2001) 12:133–50. doi: 10.1177/095632020101200301
62. Brabers, NA, and Nottet, HS. Role of the pro-inflammatory cytokines TNF-alpha and IL-1beta in HIV-associated dementia. Eur J Clin Investig. (2006) 36:447–58. doi: 10.1111/j.1365-2362.2006.01657.x
63. Tyor, WR, Glass, JD, Griffin, JW, Becker, PS, McArthur, JC, Bezman, L, et al. Cytokine expression in the brain during the acquired immunodeficiency syndrome. Ann Neurol. (1992) 31:349–60. doi: 10.1002/ana.410310402
64. Uzasci, L, Nath, A, and Cotter, R. Oxidative stress and the HIV-infected brain proteome. J Neuroimmune Pharmacol. (2013) 8:1167–80. doi: 10.1007/s11481-013-9444-x
65. Yeung, MC, Pulliam, L, and Lau, AS. The HIV envelope protein gp120 is toxic to human brain-cell cultures through the induction of interleukin-6 and tumor necrosis factor-alpha. AIDS. (1995) 9:137–44. doi: 10.1097/00002030-199509020-00004
66. Nicolini, A, Ajmone-Cat, MA, Bernardo, A, Levi, G, and Minghetti, L. Human immunodeficiency virus type-1 tat protein induces nuclear factor (NF)-kappaB activation and oxidative stress in microglial cultures by independent mechanisms. J Neurochem. (2001) 79:713–6. doi: 10.1046/j.1471-4159.2001.00568.x
67. Borrajo, A, Spuch, C, Penedo, MA, Olivares, JM, and Agís-Balboa, RC. Important role of microglia in HIV-1 associated neurocognitive disorders and the molecular pathways implicated in its pathogenesis. Ann Med. (2021) 53:43–69. doi: 10.1080/07853890.2020.1814962
68. Vilca, SJ, Margetts, AV, Pollock, TA, and Tuesta, LM. Transcriptional and epigenetic regulation of microglia in substance use disorders. Mol Cell Neurosci. (2023) 125:103838. doi: 10.1016/j.mcn.2023.103838
69. Azzoni, L, Metzger, D, and Montaner, LJ. Effect of opioid use on immune activation and HIV persistence on ART. J Neuroimmune Pharmacol. (2020) 15:643–57. doi: 10.1007/s11481-020-09959-y
70. Madhuravasal Krishnan, J, Kong, L, Karns, R, Medvedovic, M, Sherman, KE, and Blackard, JT. The synthetic opioid fentanyl increases HIV replication and chemokine co-receptor expression in lymphocyte cell lines. Viruses. (2023) 15:1027. doi: 10.3390/v15041027
71. Kong, L, Shata, MTM, Brown, JL, Lyons, MS, Sherman, KE, and Blackard, JT. The synthetic opioid fentanyl increases HIV replication and chemokine co-receptor expression in vitro. J Neurovirol. (2022) 28:583–94. doi: 10.1007/s13365-022-01090-3
72. Wang, MR, Wu, DD, Luo, F, Zhong, CJ, Wang, X, Zhu, N, et al. Methadone inhibits viral restriction factors and facilitates HIV infection in macrophages. Front Immunol. (2020) 11:1253. doi: 10.3389/fimmu.2020.01253
73. Karagiannis, TT, Cleary, JP Jr, Gok, B, Henderson, AJ, Martin, NG, Yajima, M, et al. Single cell transcriptomics reveals opioid usage evokes widespread suppression of antiviral gene program. Nat Commun. (2020) 11:2611. doi: 10.1038/s41467-020-16159-y
74. Ma, H, Wang, H, Li, M, Barreto-de-Souza, V, Reinecke, BA, Gunta, R, et al. Bivalent ligand aiming putative mu opioid receptor and chemokine receptor CXCR4 dimers in opioid enhanced HIV-1 entry. ACS Med Chem Lett. (2020) 11:2318–24. doi: 10.1021/acsmedchemlett.0c00444
75. Sonti, S, Tyagi, K, Pande, A, Daniel, R, Sharma, AL, and Tyagi, M. Crossroads of drug abuse and HIV infection: neurotoxicity and CNS reservoir. Vaccines. (2022) 10:202. doi: 10.3390/vaccines10020202
76. Bachmann, N, von Siebenthal, C, Vongrad, V, Turk, T, Neumann, K, Beerenwinkel, N, et al. Determinants of HIV-1 reservoir size and long-term dynamics during suppressive ART. Nat Commun. (2019) 10:3193. doi: 10.1038/s41467-019-10884-9
77. Prottengeier, J, Koutsilieri, E, and Scheller, C. The effects of opioids on HIV reactivation in latently-infected T-lymphoblasts. AIDS Res Ther. (2014) 11:17. doi: 10.1186/1742-6405-11-17
78. Gornalusse, GG, Vojtech, LN, Levy, CN, Hughes, SM, Kim, Y, Valdez, R, et al. Buprenorphine increases HIV-1 infection in vitro but does not reactivate HIV-1 from latency. Viruses. (2021) 13:1472. doi: 10.3390/v13081472
79. Murphy, AJ, Kelschenbach, J, He, H, Chao, W, Kim, BH, Volsky, DJ, et al. Buprenorphine reverses neurocognitive impairment in EcoHIV infected mice: a potential therapy for HIV-NCI. Front Immunol. (2022) 13:1004985. doi: 10.3389/fimmu.2022.1004985
80. Basukala, B, Rossi, S, Bendiks, S, Gnatienko, N, Patts, G, Krupitsky, E, et al. Virally suppressed people living with HIV who use opioids have diminished latency reversal. Viruses. (2023) 15:415. doi: 10.3390/v15020415
81. Trunfio, M, Chaillon, A, Beliakova-Bethell, N, Deiss, R, Letendre, SL, Riggs, PK, et al. Beyond the syndemic of opioid use disorders and HIV: the impact of opioids on viral reservoirs. Viruses. (2023) 15:1712. doi: 10.3390/v15081712
82. Prasad, A, Kulkarni, R, Shrivastava, A, Jiang, S, Lawson, K, and Groopman, JE. Methamphetamine functions as a novel CD4+ T-cell activator via the sigma-1 receptor to enhance HIV-1 infection. Sci Rep. (2019) 9:958. doi: 10.1038/s41598-018-35757-x
83. Mata, MM, Napier, TC, Graves, SM, Mahmood, F, Raeisi, S, and Baum, LL. Methamphetamine decreases CD4 T cell frequency and alters pro-inflammatory cytokine production in a model of drug abuse. Eur J Pharmacol. (2015) 752:26–33. doi: 10.1016/j.ejphar.2015.02.002
84. Chilunda, V, Weiselberg, J, Martinez-Meza, S, Mhamilawa, LE, Cheney, L, and Berman, JW. Methamphetamine induces transcriptional changes in cultured HIV-infected mature monocytes that may contribute to HIV neuropathogenesis. Front Immunol. (2022) 13:952183. doi: 10.3389/fimmu.2022.952183
85. Lawson, KS, Prasad, A, and Groopman, JE. Methamphetamine enhances HIV-1 replication in CD4+ T-cells via a novel IL-1beta auto-regulatory loop. Front Immunol. (2020) 11:136. doi: 10.3389/fimmu.2020.00136
86. Potula, R, Haldar, B, Cenna, JM, Sriram, U, and Fan, S. Methamphetamine alters T cell cycle entry and progression: role in immune dysfunction. Cell Death Discov. (2018) 4:44. doi: 10.1038/s41420-018-0045-6
87. Loftis, JM, Ramani, S, Firsick, EJ, Hudson, R, Le-Cook, A, Murnane, KS, et al. Immunotherapeutic treatment of inflammation in mice exposed to methamphetamine. Front Psychiatry. (2023) 14:1259041. doi: 10.3389/fpsyt.2023.1259041
88. Yu, Q, Zhang, D, Walston, M, Zhang, J, Liu, Y, and Watson, RR. Chronic methamphetamine exposure alters immune function in normal and retrovirus-infected mice. Int Immunopharmacol. (2002) 2:951–62. doi: 10.1016/S1567-5769(02)00047-4
89. Manuzak, JA, Gott, TM, Kirkwood, JS, Coronado, E, Hensley-McBain, T, Miller, C, et al. Heavy cannabis use associated with reduction in activated and inflammatory immune cell frequencies in antiretroviral therapy-treated human immunodeficiency virus-infected individuals. Clin Infect Dis. (2018) 66:1872–82. doi: 10.1093/cid/cix1116
90. Falcinelli, SD, Cooper-Volkheimer, AD, Semenova, L, Wu, E, Richardson, A, Ashokkumar, M, et al. Impact of cannabis use on immune cell populations and the viral reservoir in people with HIV on suppressive antiretroviral therapy. J Infect Dis. (2023) 228:1600–9. doi: 10.1093/infdis/jiad364
91. Mboumba Bouassa, RS, Comeau, E, Alexandrova, Y, Pagliuzza, A, Yero, A, Samarani, S, et al. Effects of oral cannabinoids on systemic inflammation and viral reservoir markers in people with HIV on antiretroviral therapy: results of the CTN PT028 pilot clinical trial. Cells. (2023) 12:1811. doi: 10.3390/cells12141811
92. Lisano, JK, Kisiolek, J, Flores, V, Smoak, P, Pullen, NA, and Stewart, LK. Chronic cannabis use is associated with altered monocyte phenotype, immune response, and depression in physically active individuals. Can J Physiol Pharmacol. (2023) 101:316–26. doi: 10.1139/cjpp-2022-0451
93. Maggirwar, SB, and Khalsa, JH. The link between cannabis use, immune system, and viral infections. Viruses. (2021) 13:1099. doi: 10.3390/v13061099
94. Zammit, M, Tao, Y, Olsen, ME, Metzger, J, Vermilyea, SC, Bjornson, K, et al. [18F]FEPPA PET imaging for monitoring CD68-positive microglia/macrophage neuroinflammation in nonhuman primates. EJNMMI Res. (2020) 10:93. doi: 10.1186/s13550-020-00683-5
95. Janssen, B, Vugts, D, Windhorst, A, and Mach, R. PET imaging of microglial activation-beyond targeting TSPO. Molecules. (2018) 23:93. doi: 10.3390/molecules23030607
96. Horti, AG, Naik, R, Foss, CA, Minn, I, Misheneva, V, Du, Y, et al. PET imaging of microglia by targeting macrophage colony-stimulating factor 1 receptor (CSF1R). Proc Natl Acad Sci USA. (2019) 116:1686–91. doi: 10.1073/pnas.1812155116
97. Airas, L, Rissanen, E, and Rinne, J. Imaging of microglial activation in MS using PET: research use and potential future clinical application. Mult Scler. (2017) 23:496–504. doi: 10.1177/1352458516674568
98. Shen, Z, Bao, X, and Wang, R. Clinical PET imaging of microglial activation: implications for microglial therapeutics in Alzheimer’s disease. Front Aging Neurosci. (2018) 10:314. doi: 10.3389/fnagi.2018.00314
99. Jain, P, Chaney, AM, Carlson, ML, Jackson, IM, Rao, A, and James, ML. Neuroinflammation PET imaging: current opinion and future directions. J Nucl Med. (2020) 61:1107–12. doi: 10.2967/jnumed.119.229443
100. Scott, G, Mahmud, M, Owen, DR, and Johnson, MR. Microglial positron emission tomography (PET) imaging in epilepsy: applications, opportunities and pitfalls. Seizure. (2017) 44:42–7. doi: 10.1016/j.seizure.2016.10.023
101. Ishibashi, K, Miura, Y, Imamura, A, Toyohara, J, and Ishii, K. Microglial activation on 11C-CB184 PET in a patient with cerebellar ataxia associated with HIV infection. Clin Nucl Med. (2018) 43:e82–4. doi: 10.1097/RLU.0000000000001936
102. Young, JW, Barback, CV, Stolz, LA, Groman, SM, Vera, DR, Hoh, C, et al. MicroPET evidence for a hypersensitive neuroinflammatory profile of gp120 mouse model of HIV. Psychiatry Res Neuroimaging. (2022) 321:111445. doi: 10.1016/j.pscychresns.2022.111445
103. Schlachetzki, JCM, Zhou, Y, and Glass, CK. Human microglia phenotypes in the brain associated with HIV infection. Curr Opin Neurobiol. (2022) 77:102637. doi: 10.1016/j.conb.2022.102637
104. O’Connor, EE, Sullivan, EV, Chang, L, Hammoud, DA, Wilson, TW, Ragin, AB, et al. Imaging of brain structural and functional effects in people with human immunodeficiency virus. J Infect Dis. (2023) 227:S16–29. doi: 10.1093/infdis/jiac387
105. Guo, S, Wang, H, and Yin, Y. Microglia polarization from M1 to M2 in neurodegenerative diseases. Front Aging Neurosci. (2022) 14:815347. doi: 10.3389/fnagi.2022.815347
106. Tang, Y, and Le, W. Differential roles of M1 and M2 microglia in neurodegenerative diseases. Mol Neurobiol. (2016) 53:1181–94. doi: 10.1007/s12035-014-9070-5
107. Cherry, JD, Olschowka, JA, and O’Banion, MK. Neuroinflammation and M2 microglia: the good, the bad, and the inflamed. J Neuroinflammation. (2014) 11:98. doi: 10.1186/1742-2094-11-98
108. Orihuela, R, McPherson, CA, and Harry, GJ. Microglial M1/M2 polarization and metabolic states. Br J Pharmacol. (2016) 173:649–65. doi: 10.1111/bph.13139
109. Fan, YY, and Huo, J. A1/A2 astrocytes in central nervous system injuries and diseases: angels or devils? Neurochem Int. (2021) 148:105080. doi: 10.1016/j.neuint.2021.105080
110. Lawrence, JM, Schardien, K, Wigdahl, B, and Nonnemacher, MR. Roles of neuropathology-associated reactive astrocytes: a systematic review. Acta Neuropathol Commun. (2023) 11:42. doi: 10.1186/s40478-023-01526-9
111. Richards, MH, Narasipura, SD, Seaton, MS, Lutgen, V, and Al-Harthi, L. Migration of CD8+ T cells into the central nervous system gives rise to highly potent anti-HIV CD4dim CD8bright T cells in a Wnt signaling-dependent manner. J Immunol. (2016) 196:317–27. doi: 10.4049/jimmunol.1501394
112. Al-Harthi, L. Comment on “CD4+ CD8+ T cells represent a significant portion of the anti-HIV T cell response to acute HIV infection”. J Immunol. (2012) 188:5809. doi: 10.4049/jimmunol.1290028
113. Zloza, A, Schenkel, JM, Tenorio, AR, Martinson, JA, Jeziorczak, PM, and Al-Harthi, L. Potent HIV-specific responses are enriched in a unique subset of CD8+ T cells that coexpresses CD4 on its surface. Blood. (2009) 114:3841–53. doi: 10.1182/blood-2009-02-202481
114. Churchill, MJ, Wesselingh, SL, Cowley, D, Pardo, CA, McArthur, JC, Brew, BJ, et al. Extensive astrocyte infection is prominent in human immunodeficiency virus-associated dementia. Ann Neurol. (2009) 66:253–8. doi: 10.1002/ana.21697
115. Churchill, MJ, Gorry, PR, Cowley, D, Lal, L, Sonza, S, Purcell, DFJ, et al. Use of laser capture microdissection to detect integrated HIV-1 DNA in macrophages and astrocytes from autopsy brain tissues. J Neurovirol. (2006) 12:146–52. doi: 10.1080/13550280600748946
116. Ko, A, Kang, G, Hattler, JB, Galadima, HI, Zhang, J, Li, Q, et al. Macrophages but not astrocytes harbor HIV DNA in the brains of HIV-1-infected aviremic individuals on suppressive antiretroviral therapy. J Neuroimmune Pharmacol. (2019) 14:110–9. doi: 10.1007/s11481-018-9809-2
117. Bergamaschi, A, and Pancino, G. Host hindrance to HIV-1 replication in monocytes and macrophages. Retrovirology. (2010) 7:31. doi: 10.1186/1742-4690-7-31
118. Dong, C, Kwas, C, and Wu, L. Transcriptional restriction of human immunodeficiency virus type 1 gene expression in undifferentiated primary monocytes. J Virol. (2009) 83:3518–27. doi: 10.1128/JVI.02665-08
119. Triques, K, and Stevenson, M. Characterization of restrictions to human immunodeficiency virus type 1 infection of monocytes. J Virol. (2004) 78:5523–7. doi: 10.1128/JVI.78.10.5523-5527.2004
120. León-Rivera, R, Veenstra, M, Donoso, M, Tell, E, Eugenin, E, Morgello, S, et al. Central nervous system (CNS) viral seeding by mature monocytes and potential therapies to reduce CNS viral reservoirs in the cART era. mBio. (2021) 12:e03633. doi: 10.1128/mBio.03633-20
121. Veenstra, M, Byrd, DA, Inglese, M, Buyukturkoglu, K, Williams, DW, Fleysher, L, et al. CCR2 on peripheral blood CD14+ CD16+ monocytes correlates with neuronal damage, HIV-associated neurocognitive disorders, and peripheral HIV DNA: reseeding of CNS reservoirs? J Neuroimmune Pharmacol. (2019) 14:120–33. doi: 10.1007/s11481-018-9792-7
122. Crawshaw, AA, and Robertson, NP. The role of TSPO PET in assessing neuroinflammation. J Neurol. (2017) 264:1825–7. doi: 10.1007/s00415-017-8565-1
123. Tournier, BB, Tsartsalis, S, Ceyzériat, K, Garibotto, V, and Millet, P. In vivo TSPO signal and neuroinflammation in Alzheimer’s disease. Cells. (2020) 9:1941. doi: 10.3390/cells9091941
124. Valdivia, A, Ly, J, Gonzalez, L, Hussain, P, Saing, T, Islamoglu, H, et al. Restoring cytokine balance in HIV-positive individuals with low CD4 T cell counts. AIDS Res Hum Retrovir. (2017) 33:905–18. doi: 10.1089/aid.2016.0303
125. Nosik, M, Ryzhov, K, Rymanova, I, Sobkin, A, Kravtchenko, A, Kuimova, U, et al. Dynamics of plasmatic levels of pro- and anti-inflammatory cytokines in HIV-infected individuals with M. tuberculosis co-infection. Microorganisms. (2021) 9:2291. doi: 10.3390/microorganisms9112291
126. Martinez, P, Lien, L, Zemore, S, Bramness, JG, and Neupane, SP. Circulating cytokine levels are associated with symptoms of depression and anxiety among people with alcohol and drug use disorders. J Neuroimmunol. (2018) 318:80–6. doi: 10.1016/j.jneuroim.2018.02.011
127. Morcuende, A, Navarrete, F, Nieto, E, Manzanares, J, and Femenía, T. Inflammatory biomarkers in addictive disorders. Biomolecules. (2021) 11:12. doi: 10.3390/biom11121824
128. Mednova, IA, Levchuk, LA, Boiko, AS, Roschina, OV, Simutkin, GG, Bokhan, NA, et al. Cytokine level in patients with mood disorder, alcohol use disorder and their comorbidity. World J Biol Psychiatry. (2023) 24:243–53. doi: 10.1080/15622975.2022.2095439
129. Tewari, M, and Seth, P. Emerging role of P2X7 receptors in CNS health and disease. Ageing Res Rev. (2015) 24:328–42. doi: 10.1016/j.arr.2015.10.001
130. Koole, M, Schmidt, ME, Hijzen, A, Ravenstijn, P, Vandermeulen, C, van Weehaeghe, D, et al. 18F-JNJ-64413739, a novel PET ligand for the P2X7 ion channel: radiation dosimetry, kinetic modeling, test-retest variability, and occupancy of the P2X7 antagonist JNJ-54175446. J Nucl Med. (2019) 60:683–90. doi: 10.2967/jnumed.118.216747
131. Beaino, W, Janssen, B, Kooij, G, van der Pol, SMA, van Het Hof, B, van Horssen, J, et al. Purinergic receptors P2Y12R and P2X7R: potential targets for PET imaging of microglia phenotypes in multiple sclerosis. J Neuroinflammation. (2017) 14:259. doi: 10.1186/s12974-017-1034-z
132. Walker, DG, Tang, TM, Mendsaikhan, A, Tooyama, I, Serrano, GE, Sue, LI, et al. Patterns of expression of purinergic receptor P2RY12, a putative marker for non-activated microglia, in aged and Alzheimer’s disease brains. Int J Mol Sci. (2020) 21:678. doi: 10.3390/ijms21020678
133. Chitu, V, Gokhan, Ş, Nandi, S, Mehler, MF, and Stanley, ER. Emerging roles for CSF-1 receptor and its ligands in the nervous system. Trends Neurosci. (2016) 39:378–93. doi: 10.1016/j.tins.2016.03.005
134. Ohnishi, A, Senda, M, Yamane, T, Mikami, T, Nishida, H, Nishio, T, et al. Exploratory human PET study of the effectiveness of 11C-ketoprofen methyl ester, a potential biomarker of neuroinflammatory processes in Alzheimer’s disease. Nucl Med Biol. (2016) 43:438–44. doi: 10.1016/j.nucmedbio.2016.04.005
135. Narayanaswami, V, Dahl, K, Bernard-Gauthier, V, Josephson, L, Cumming, P, and Vasdev, N. Emerging PET radiotracers and targets for imaging of neuroinflammation in neurodegenerative diseases: outlook beyond TSPO. Mol Imaging. (2018) 17:1536012118792317. doi: 10.1177/1536012118792317
136. Wu, K, Liu, YY, Shao, S, Song, W, Chen, XH, Dong, YT, et al. The microglial innate immune receptors TREM-1 and TREM-2 in the anterior cingulate cortex (ACC) drive visceral hypersensitivity and depressive-like behaviors following DSS-induced colitis. Brain Behav Immun. (2023) 112:96–117. doi: 10.1016/j.bbi.2023.06.003
137. Campbell, GR, Rawat, P, To, RK, and Spector, SA. HIV-1 tat upregulates TREM1 expression in human microglia. J Immunol. (2023) 211:429–42. doi: 10.4049/jimmunol.2300152
138. Xu, P, Zhang, X, Liu, Q, Xie, Y, Shi, X, Chen, J, et al. Microglial TREM-1 receptor mediates neuroinflammatory injury via interaction with SYK in experimental ischemic stroke. Cell Death Dis. (2019) 10:555. doi: 10.1038/s41419-019-1777-9
139. Yamagishi, S, Iga, Y, Nakamura, M, Takizawa, C, Fukumoto, D, Kakiuchi, T, et al. Upregulation of cannabinoid receptor type 2, but not TSPO, in senescence-accelerated neuroinflammation in mice: a positron emission tomography study. J Neuroinflammation. (2019) 16:208. doi: 10.1186/s12974-019-1604-3
140. Savonenko, AV, Melnikova, T, Wang, Y, Ravert, H, Gao, Y, Koppel, J, et al. Cannabinoid CB2 receptors in a mouse model of Aβ amyloidosis: immunohistochemical analysis and suitability as a PET biomarker of neuroinflammation. PLoS One. (2015) 10:e0129618. doi: 10.1371/journal.pone.0129618
141. Logiacco, F, Xia, P, Georgiev, SV, Franconi, C, Chang, YJ, Ugursu, B, et al. Microglia sense neuronal activity via GABA in the early postnatal hippocampus. Cell Rep. (2021) 37:110128. doi: 10.1016/j.celrep.2021.110128
142. Liu, J, Feng, X, Wang, Y, Xia, X, and Zheng, JC. Astrocytes: GABAceptive and GABAergic cells in the brain. Front Cell Neurosci. (2022) 16:892497. doi: 10.3389/fncel.2022.892497
143. Knight, AC, Brill, SA, Queen, SE, Tarwater, PM, and Mankowski, JL. Increased microglial CSF1R expression in the SIV/macaque model of HIV CNS disease. J Neuropathol Exp Neurol. (2018) 77:199–206. doi: 10.1093/jnen/nlx115
144. Irons, DL, Meinhardt, T, Allers, C, Kuroda, MJ, and Kim, WK. Overexpression and activation of colony-stimulating factor 1 receptor in the SIV/macaque model of HIV infection and neuroHIV. Brain Pathol. (2019) 29:826–36. doi: 10.1111/bpa.12731
145. Bai, R, Song, C, Lv, S, Chang, L, Hua, W, Weng, W, et al. Role of microglia in HIV-1 infection. AIDS Res Ther. (2023) 20:16. doi: 10.1186/s12981-023-00511-5
146. Van Weehaeghe, D, Van Schoor, E, De Vocht, J, Koole, M, Attili, B, Celen, S, et al. TSPO versus P2X7 as a target for neuroinflammation: an in vitro and in vivo study. J Nucl Med. (2020) 61:604–7. doi: 10.2967/jnumed.119.231985
147. Van Weehaeghe, D, Koole, M, Schmidt, ME, Deman, S, Jacobs, AH, Souche, E, et al. [11C]JNJ54173717, a novel P2X7 receptor radioligand as marker for neuroinflammation: human biodistribution, dosimetry, brain kinetic modelling and quantification of brain P2X7 receptors in patients with Parkinson’s disease and healthy volunteers. Eur J Nucl Med Mol Imaging. (2019) 46:2051–64. doi: 10.1007/s00259-019-04369-6
148. McGrath, AG, and Briand, LA. A potential role for microglia in stress- and drug-induced plasticity in the nucleus accumbens: a mechanism for stress-induced vulnerability to substance use disorder. Neurosci Biobehav Rev. (2019) 107:360–9. doi: 10.1016/j.neubiorev.2019.09.007
149. Melbourne, JK, Chandler, CM, van Doorn, CE, Bardo, MT, Pauly, JR, Peng, H, et al. Primed for addiction: a critical review of the role of microglia in the neurodevelopmental consequences of adolescent alcohol drinking. Alcohol Clin Exp Res. (2021) 45:1908–26. doi: 10.1111/acer.14694
150. Shemer, A, Grozovski, J, Tay, TL, Tao, J, Volaski, A, Süß, P, et al. Engrafted parenchymal brain macrophages differ from microglia in transcriptome, chromatin landscape and response to challenge. Nat Commun. (2018) 9:5206. doi: 10.1038/s41467-018-07548-5
151. Yeh, H, and Ikezu, T. Transcriptional and epigenetic regulation of microglia in health and disease. Trends Mol Med. (2019) 25:96–111. doi: 10.1016/j.molmed.2018.11.004
152. Gosselin, D, Link, VM, Romanoski, CE, Fonseca, GJ, Eichenfield, DZ, Spann, NJ, et al. Environment drives selection and function of enhancers controlling tissue-specific macrophage identities. Cell. (2014) 159:1327–40. doi: 10.1016/j.cell.2014.11.023
153. Bohlen, CJ, Bennett, FC, Tucker, AF, Collins, HY, Mulinyawe, SB, and Barres, BA. Diverse requirements for microglial survival, specification, and function revealed by defined-medium cultures. Neuron. (2017) 94:759–773.e8. doi: 10.1016/j.neuron.2017.04.043
154. Matcovitch-Natan, O, Winter, DR, Giladi, A, Vargas Aguilar, S, Spinrad, A, Sarrazin, S, et al. Microglia development follows a stepwise program to regulate brain homeostasis. Science. (2016) 353:aad8670. doi: 10.1126/science.aad8670
155. Mass, E, Ballesteros, I, Farlik, M, Halbritter, F, Günther, P, Crozet, L, et al. Specification of tissue-resident macrophages during organogenesis. Science. (2016) 353. doi: 10.1126/science.aaf4238
156. Komada, M, and Nishimura, Y. Epigenetics and neuroinflammation associated with neurodevelopmental disorders: a microglial perspective. Front Cell Dev Biol. (2022) 10:852752. doi: 10.3389/fcell.2022.852752
157. Li, Q, and Barres, BA. Microglia and macrophages in brain homeostasis and disease. Nat Rev Immunol. (2018) 18:225–42. doi: 10.1038/nri.2017.125
158. Amici, SA, Dong, J, and Guerau-de-Arellano, M. Molecular mechanisms modulating the phenotype of macrophages and microglia. Front Immunol. (2017) 8:1520. doi: 10.3389/fimmu.2017.01520
159. Bennett, ML, Bennett, FC, Liddelow, SA, Ajami, B, Zamanian, JL, Fernhoff, NB, et al. New tools for studying microglia in the mouse and human CNS. Proc Natl Acad Sci USA. (2016) 113:E1738–46. doi: 10.1073/pnas.1525528113
160. Ruan, C, and Elyaman, W. A new understanding of TMEM119 as a marker of microglia. Front Cell Neurosci. (2022) 16:902372. doi: 10.3389/fncel.2022.902372
161. Van Hove, H, Martens, L, Scheyltjens, I, De Vlaminck, K, Pombo Antunes, AR, De Prijck, S, et al. A single-cell atlas of mouse brain macrophages reveals unique transcriptional identities shaped by ontogeny and tissue environment. Nat Neurosci. (2019) 22:1021–35. doi: 10.1038/s41593-019-0393-4
162. Masuda, T, Sankowski, R, Staszewski, O, Böttcher, C, Amann, L, Sagar,, et al. Spatial and temporal heterogeneity of mouse and human microglia at single-cell resolution. Nature. (2019) 566:388–92. doi: 10.1038/s41586-019-0924-x
163. Li, Q, Cheng, Z, Zhou, L, Darmanis, S, Neff, NF, Okamoto, J, et al. Developmental heterogeneity of microglia and brain myeloid cells revealed by deep single-cell RNA sequencing. Neuron. (2019) 101:207–223.e10. doi: 10.1016/j.neuron.2018.12.006
164. Rheinberger, M, Costa, AL, Kampmann, M, Glavas, D, Shytaj, IL, Sreeram, S, et al. Genomic profiling of HIV-1 integration in microglia cells links viral integration to the topologically associated domains. Cell Rep. (2023) 42:112110. doi: 10.1016/j.celrep.2023.112110
165. Miao, B, Xing, X, Bazylianska, V, Madden, P, Moszczynska, A, and Zhang, B. Methamphetamine-induced region-specific transcriptomic and epigenetic changes in the brain of male rats. Commun Biol. (2023) 6:991. doi: 10.1038/s42003-023-05355-3
166. Abdel-Hameed, EA, Ji, H, and Shata, MT. HIV-induced epigenetic alterations in host cells. Adv Exp Med Biol. (2016) 879:27–38. doi: 10.1007/978-3-319-24738-0_2
167. Portela, A, and Esteller, M. Epigenetic modifications and human disease. Nat Biotechnol. (2010) 28:1057–68. doi: 10.1038/nbt.1685
168. Periyasamy, P, Thangaraj, A, Kannan, M, Oladapo, A, and Buch, S. The epigenetic role of miR-124 in HIV-1 tat- and cocaine-mediated microglial activation. Int J Mol Sci. (2022) 23:15017. doi: 10.3390/ijms232315017
169. Svensson, JP. Targeting epigenetics to cure HIV-1: lessons from (and for) cancer treatment. Front Cell Infect Microbiol. (2021) 11:668637. doi: 10.3389/fcimb.2021.668637
170. Sok, D, Pauthner, M, Briney, B, Lee, JH, Saye-Francisco, KL, Hsueh, J, et al. A prominent site of antibody vulnerability on HIV envelope incorporates a motif associated with CCR5 binding and its camouflaging glycans. Immunity. (2016) 45:31–45. doi: 10.1016/j.immuni.2016.06.026
171. Jiang, C, Lian, X, Gao, C, Sun, X, Einkauf, KB, Chevalier, JM, et al. Distinct viral reservoirs in individuals with spontaneous control of HIV-1. Nature. (2020) 585:261–7. doi: 10.1038/s41586-020-2651-8
172. Moron-Lopez, S, Kim, P, Søgaard, OS, Tolstrup, M, Wong, JK, and Yukl, SA. Characterization of the HIV-1 transcription profile after romidepsin administration in ART-suppressed individuals. AIDS. (2019) 33:425–31. doi: 10.1097/QAD.0000000000002083
173. Bouchat, S, Delacourt, N, Kula, A, Darcis, G, van Driessche, B, Corazza, F, et al. Sequential treatment with 5-aza-2′-deoxycytidine and deacetylase inhibitors reactivates HIV-1. EMBO Mol Med. (2016) 8:117–38. doi: 10.15252/emmm.201505557
174. Kessing, CF, Nixon, CC, Li, C, Tsai, P, Takata, H, Mousseau, G, et al. In vivo suppression of HIV rebound by didehydro-cortistatin A, a “block-and-lock” strategy for HIV-1 treatment. Cell Rep. (2017) 21:600–11. doi: 10.1016/j.celrep.2017.09.080
175. Besnard, E, Hakre, S, Kampmann, M, Lim, HW, Hosmane, NN, Martin, A, et al. The mTOR complex controls HIV latency. Cell Host Microbe. (2016) 20:785–97. doi: 10.1016/j.chom.2016.11.001
176. Niu, Q, Liu, Z, Alamer, E, Fan, X, Chen, H, Endsley, J, et al. Structure-guided drug design identifies a BRD4-selective small molecule that suppresses HIV. J Clin Invest. (2019) 129:3361–73. doi: 10.1172/JCI120633
177. Nguyen, K, das, B, Dobrowolski, C, Karn, J, Rice, A, Zack, J, et al. Multiple histone lysine methyltransferases are required for the establishment and maintenance of HIV-1 latency. MBio. (2017) 8:e00133. doi: 10.1128/mBio.00133-17
178. Khan, S, Iqbal, M, Tariq, M, Baig, SM, and Abbas, W. Epigenetic regulation of HIV-1 latency: focus on polycomb group (PcG) proteins. Clin Epigenetics. (2018) 10:14. doi: 10.1186/s13148-018-0441-z
179. Constantinou, M, Klavaris, A, Koufaris, C, and Kirmizis, A. Cellular effects of NAT-mediated histone N-terminal acetylation. J Cell Sci. (2023) 136:jcs260801. doi: 10.1242/jcs.260801
180. Gottlieb, L, and Marmorstein, R. Biochemical and structural analysis of N-terminal acetyltransferases. Methods Enzymol. (2019) 626:271–99. doi: 10.1016/bs.mie.2019.07.016
181. Sundar, V, McLaughlin, JP, and Samikkannu, T. Epigenetic signature of N-terminal acetyltransferases: a probable mediator of immune and neuropathogenesis in HIV infection. Mol Brain. (2022) 15:69. doi: 10.1186/s13041-022-00946-3
182. Ahmed, F, and Husain, M. Human N-alpha-acetyltransferase 60 promotes influenza a virus infection by dampening the interferon alpha signaling. Front Immunol. (2021) 12:771792. doi: 10.3389/fimmu.2021.771792
183. Oishi, K, Yamayoshi, S, Kozuka-Hata, H, Oyama, M, and Kawaoka, Y. N-terminal acetylation by NatB is required for the shutoff activity of influenza a virus PA-X. Cell Rep. (2018) 24:851–60. doi: 10.1016/j.celrep.2018.06.078
184. Antinori, A, Arendt, G, Becker, JT, Brew, BJ, Byrd, DA, Cherner, M, et al. Updated research nosology for HIV-associated neurocognitive disorders. Neurology. (2007) 69:1789–99. doi: 10.1212/01.WNL.0000287431.88658.8b
185. Qu, Y, Weinstein, A, Wang, Z, Cheng, Y, Kingsley, L, Levine, A, et al. Legacy effect on neuropsychological function in HIV-infected men on combination antiretroviral therapy. AIDS. (2022) 36:19–27. doi: 10.1097/QAD.0000000000003071
186. Canestri, A, Lescure, FX, Jaureguiberry, S, Moulignier, A, Amiel, C, Marcelin, AG, et al. Discordance between cerebral spinal fluid and plasma HIV replication in patients with neurological symptoms who are receiving suppressive antiretroviral therapy. Clin Infect Dis. (2010) 50:773–8. doi: 10.1086/650538
187. Vidal, F, Gutiérrez, F, Gutiérrez, M, Olona, M, Sánchez, V, Mateo, G, et al. Pharmacogenetics of adverse effects due to antiretroviral drugs. AIDS Rev. (2010) 12:15–30.
188. Montessori, V, Press, N, Harris, M, Akagi, L, and Montaner, JS. Adverse effects of antiretroviral therapy for HIV infection. CMAJ. (2004) 170:229–38.
189. Fernandez-Montero, JV, Eugenia, E, Barreiro, P, Labarga, P, and Soriano, V. Antiretroviral drug-related toxicities—clinical spectrum, prevention, and management. Expert Opin Drug Saf. (2013) 12:697–707. doi: 10.1517/14740338.2013.806480
190. O’Brien, ME, Clark, RA, Besch, CL, Myers, L, and Kissinger, P. Patterns and correlates of discontinuation of the initial HAART regimen in an urban outpatient cohort. J Acquir Immune Defic Syndr. (2003) 34:407–14. doi: 10.1097/00126334-200312010-00008
191. Zulu, SS, Simola, N, Mabandla, MV, and Daniels, WMU. Effect of long-term administration of antiretroviral drugs (tenofovir and nevirapine) on neuroinflammation and neuroplasticity in mouse hippocampi. J Chem Neuroanat. (2018) 94:86–92. doi: 10.1016/j.jchemneu.2018.10.003
Keywords: HIV, drugs of abuse, microglia, CART, HANDs
Citation: Haorah J, Malaroviyam S, Iyappan H and Samikkannu T (2025) Neurological impact of HIV/AIDS and substance use alters brain function and structure. Front. Med. 11:1505440. doi: 10.3389/fmed.2024.1505440
Edited by:
Joseph Bryant, University of Maryland, United StatesReviewed by:
Debashis Dutta, University of Nebraska Medical Center, United StatesAndrew MacLean, Tulane University, United States
Copyright © 2025 Haorah, Malaroviyam, Iyappan and Samikkannu. This is an open-access article distributed under the terms of the Creative Commons Attribution License (CC BY). The use, distribution or reproduction in other forums is permitted, provided the original author(s) and the copyright owner(s) are credited and that the original publication in this journal is cited, in accordance with accepted academic practice. No use, distribution or reproduction is permitted which does not comply with these terms.
*Correspondence: Thangavel Samikkannu, dGhhbmdhdmVsQHRhbXUuZWR1