- 1Department of Periodontics and Oral Medicine, School of Dentistry, University of Michigan, Ann Arbor, MI, United States
- 2Department of Pathology, Medical School, University of Michigan, Ann Arbor, MI, United States
- 3Rogel Cancer Center, University of Michigan, Ann Arbor, MI, United States
- 4Department of Biological Sciences, School of Dental Medicine, Case Western Reserve University, Cleveland, OH, United States
- 5Department of Pathology, Case Western Reserve University, Cleveland, OH, United States
- 6Center for AIDS Research, Case Western Reserve University, Cleveland, OH, United States
- 7Case Comprehensive Cancer Center, School of Medicine, Case Western Reserve University, Cleveland, OH, United States
Inflammation is a process that is associated with the activation of distal immunosuppressive pathways that have evolved to restore homeostasis and prevent excessive tissue destruction. However, long-term immunosuppression resulting from systemic and local inflammation that may stem from dysbiosis, infections, or aging poses a higher risk for cancers. Cancer incidence and progression dramatically increase with chronic infections including HIV infection. Thus, studies on pro-tumorigenic effects of microbial stimulants from resident microbiota and infections in the context of inflammation are needed and underway. Here, we discuss chronic infections and potential neuro-immune interactions that could establish immunomodulatory programs permissive for tumor growth and progression.
Introduction
Chronic infections have been identified as a significant risk factor for the development of various types of cancer. Helicobacter pylori, human papillomavirus (HPV), hepatitis B virus (HBV), hepatitis C virus(HCV), and Epstein–Barr virus(EBV) play prominent roles (1). Other infections such as Candida are also associated with cancers (2). Infectious agents and inflammation modulate a broad range of host immune responses, which in turn may promote carcinogenesis and progression (3). However, mechanisms related to infection-mediated immunomodulation in tumor development have not been completely understood. Local inflammatory signals in tissues provide a positive feedback loop to nerve fibers, glial, and immune cells which dynamically reciprocate in a recurrent fashion in the tumor microenvironment (4, 5). Here we discuss some of these interactions in intra-tumoral immunosuppressive milieu and cellular crosstalk at the neural-immune interface that may contribute to oral cancer initiation, progression, and metastasis.
CD8 exhaustion and CD4+CD25+FOXP3+ regulatory cells (Tregs) – at the crossroads of immune-homeostasis, chronic infections, and tumor evasion
Chronic and persistent inflammatory stimuli (due to ongoing HIV replication, microbial translocation, or co-infections) have been shown to stimulate the expression of pro-inflammatory cytokines in people living with HIV (PLWH) (6–11). Accumulating evidence suggests that HIV may lead, at least in part, to an accelerated aging phenotype in immune cells and immunomodulatory/inflammaging phenotype (12–17). With regards to the oral mucosa, opportunistic infections (18) and altered oral microbiome/mycobiome profiles (19–22) are also important features of oral inflammation in PLWH under treatment (23–26). Local and systemic inflammation in PLWH are linked to a wide range of co-morbidities, the most significant being increased propensity to malignancy (27–30). Some oral malignancies in PLWH are linked to HPV, EBV, and Kaposi’s sarcoma herpesvirus, as well as the HIV itself (31). However, the immune mechanisms of increased propensity to Head and neck cancers (HNC) in PLWH are not fully understood. HNC are the sixth most common cancers, accounting for 450,000 GLOBOCAN estimated deaths each year (32, 33). Over two-thirds of all new cancers are diagnosed among adults aged ≥60 years. Oral squamous cell carcinoma (OSCC) are aggressive tumors constituting ~90% of all oral cancers, with a global incidence of ~350,000 new cases and 177,000 deaths annually. The treatment of OSCC mainly includes surgery, radiotherapy, and chemotherapy. The prognosis of OSCC is poor due to tumor recurrence (50%) or lymph node metastasis within 3 years, and the 5-year survival rate is ~50% (34, 35). Although our understanding of underlying oncogenic processes of OSCC is evolving and has led to Epidermal Growth Factor Receptor (EGFR) and PD-1 targeted therapies, major roadblocks exist. Several cancers, including a large proportion of oral cancers, do not respond to immune checkpoint inhibitors (36–40). Varied treatment efficacy between patients, inadvertent side effects, overtreatment, worsening prognosis, and increasing treatment costs contribute to treatment challenges. Therefore, there has been great interest in understanding the mechanisms that govern immunosuppression in cancer including the contributions of components of the tumor immune microenvironment (TIME).
Due to their crucial role in the anti-tumoral immune response, CD8+ T lymphocytes and their dysfunction have been a major focus of attention. In the context of chronic infections, inflammation, or cancer progression, persisting antigen stimulation of CD8+ T cells drives progressive loss of functionality and eventual deletion instead of memory formation (41, 42). The cells in TIME are known to release cytokines to engage checkpoints on immune cells, induce an increase in exhausted cytotoxic T cells, thereby disrupting the anti-tumoral immune response. Also, unceasing antigen stimulation in tumors profoundly alters T cell differentiation trajectories, leading to CD8+ precursors of exhausted T cells (TPEX cells), which share several common features with those in chronic infections (43). Exhausted CD8 T cells may comprise heterogeneous cell populations expressing a multitude of exhaustion markers such as TOX, PD1 and lymphocyte-activation gene 3 protein (LAG3) and memory cell markers including T cell factor 1 (TCF1), B cell lymphoma 6 protein (BCL6), inhibitor of DNA binding 3 (ID3) and SLAMF6 (also known as LY108), C–C chemokine receptor type 7 (CCR7), CD62L, CD127, CD69 and eomesodermin (EOMES) (44–46) and are present in both lymphoid and non-lymphoid tissues. Apart from TPEX cells, effector-like, tumor-reactive, exhausted CD8+ T cells (TEX cells) that are distinct from resting memory T cells are also found in TIME (47, 48). These subsets undergo gradual exhaustion in the face of persisting antigen stimulation giving rise to effector-like cytolytic exhausted T cells (TEEF cells), transient or intermediate differentiation state exhausted T cells (TINT cells) with antitumoral functions, as well as terminally exhausted T cells (TTEX cells) along this trajectory. It will be of considerable interest to determine the functional relevance of these stem cell–like and exhausted CD8 T cells and factors that may drive their proliferation or exhaustion in the context of chronic infections and OSCC.
FOXP3+Tregs are central to immune homeostasis but have been implicated in cancer immune evasion and angiogenesis (49–53). These cells, along with tumor-associated macrophages (TAM), and myeloid-derived suppressive cells (MDSC) accumulate in tumors and contribute to poor immunologic response against the tumor (54, 55). Tregs display a broad degree of functional heterogeneity and phenotypic plasticity within tissues and tumors Indeed we (17, 27, 56–58) and others have shown distinct populations of Tregs namely, T-bet+FOXP3+cells (that may be dysfunctional; TregDys), ROR-γt+FOXP3+ (Treg17) cells, and PD-1+FOXP3+ cells (16, 17, 59), whose functions are significantly altered by microbiome, IL-6, and IL-1β in an mTOR dependent manner in oral mucosa (58). In solid tumors of nonlymphoid origin, Tregs may constitute 30–45% of CD4+ T cells, depending on the tumor type (55, 60), and can also hinder the success of α-PD1 cancer immunotherapy (61). Having a high Treg infiltration and highest Treg/CD8+ T cell ratio among all cancers, OSCCs are poised to benefit from Treg-targeted approaches (60). Intra-tumoral FOXP3 + Treg: CD8 ratio is associated with poor prognosis and survival in human OSCC (62) and is linked to pro-tumorigenic functions of Tregs (55). Besides being recruited into tumors via chemotaxis, FOXP3+ Tregs can be induced in situ in tumors by mediators released from tumor cells, TAM and MDSC (63). Increased accrual of Tregs is also observed in aging (age > 60) oral mucosa compared to younger mucosa (58, 64). In a 4-Nitroquinoline 1-oxide (4-NQO) mouse model of oral carcinogenesis, Candida albicans infection and zymosan exacerbate and accelerate dysplasia and hyperplasia demonstrating the role of fungal ligands in exacerbating tumor growth and progression. Our prior studies have established the requirement of TGF-β1 and microbiome in Treg cell induction and viability during Candida infection (59, 65–68). Several studies have suggested a link between oral fungi and the development of OSCC (2, 55, 69). Yet, the underlying molecular mechanisms of OSCC initiation and progression are unknown. A combination of inflammaging and impaired immunity contributes to increased susceptibility to infections and cancer in elderly individuals. Candida infection is effectively cleared in young mice but causes oral inflammation in aged mice (58). Immunopathology involved the loss of anti-inflammatory function by IL-1β dependent Treg17, but an accumulation of IL-6 and TregDys in aged oral mucosa (58). These data suggest that aging may lead to loss of homeostatic mechanisms that maintain Candida in a non-inflammatory commensal state. Also, aged mice show increased fungal abundance and early filtration of Tregs and MDSC cells than young mice during oral tumorigenesis (55). Tumors in aged animals further show higher PD-1 expression (exhaustion marker) in CD8+ T cells coinciding with accelerated incidence of dysplasia, hyperplasia, and OSCC development when compared to younger mice. Elevated resident fungal abundance in saliva implies the role of resident mycobiome dysbiosis in promoting immune dysfunction and tumorigenesis, although the cause versus consequence effect of the mycobiome is unknown. In summary, while evidence point to a link between inflammaging and immunomodulation mechanisms, the process by which mucosal cells are precisely poised for tumor growth in different contexts needs further exploration.
Fungal recognition receptors such as TLR-2 and dectin-1 are expressed by myeloid dendritic cells, monocytes, and macrophages. Dectin-1 binds specifically to β-1,3 glucans in fungi, as well as to endogenous galectins and annexins on apoptotic cells (70, 71). It has a C-type lectin-like carbohydrate recognition domain, whose stimulation leads to phosphorylation of Syk (p-SYK) and IL-1β secretion. Fungi can also induce inflammasome activation by signaling through MyD88/NF-κB and Caspase recruitment domain-containing protein-9/SYK pathways and engage pyrin domain-containing protein 3 (NLRP3), which activates the protease caspase-1 in infected macrophages and other immune cells (72, 73). IL-1β is a typical cancer-inflammation-associated cytokine up-regulated in saliva derived from OSCC patients and is linked to poor prognosis for esophageal cancer (74). HIV can also activate inflammasome pathway and IL-1β secretion, which are linked to AKT activation, T cell dysfunction and Treg enrichment seen in oral mucosa of PLWH (16). Candida can further potentiate inflammasome pathway in the context of HIV (15, 16). IL-1β is also involved in maintaining immunomodulatory Foxp3+ROR-γt+ (Treg17) cells (56–58, 68, 74), which contribute to mucosal homeostasis, tumor immune evasion and autoimmunity control (75). Inflammasome activation can significantly alter the population size and functions of immune cells (76, 77), and are linked to tumor initiation and development (78). NLRP3 is also implicated in promoting Th1 responses and anti-tumor immune functions (79). Inflammasome activation and metabolic pathways are intricately connected and regulate each other through feed-back loop mechanisms. Activated caspase-1 can mediate multiple processes including (1) release of IL-1β, (2) pyroptosis (80), (3) mitochondrial damage (81), (4) cleavage of glycolytic enzymes (82) causing alterations in glycolytic metabolites (83), and (5) degradation of innate immune sensor proteins (84). For example, succinate, an intermediate of the tricarboxylic acid (TCA) cycle can activate NLRP3 through HIF-1α stabilization and reactive oxygen species production (85). Similarly, K+-depletion/efflux-induced canonical NLRP3 response is associated with increased glycolytic flux, which is dependent on the AKT/PI3-K/ mammalian target of rapamycin (mTOR) pathway, and upregulation hexokinase 1 the primary glycolytic enzyme (86). Therefore, immunometabolism and inflammasome pathways play pivotal roles in integrating growth signals and functions in T cells including Tregs and govern tumor permissive pathways (87–93). Understanding them will pave the way to new combinatorial strategies in the face of resistance to PD-1 immunotherapy, leading to improved patient outcomes.
Interactions between immune cells and neural cells in inflammation
The oral mucosa is richly innervated with sensory afferents for physiological sensory perception (94, 95). Innervation for the oral mucosa is from the maxillary and mandibular branches of the trigeminal nerves, facial, glossopharyngeal, vagus, and hypoglossal nerves; and by the spinal accessory nerve (96–98). The tongue receives additional sensory innervation from the glossopharyngeal nerve, and the chorda tympani branch of the facial nerve (97, 99). Bidirectional signals between tissue-resident immune cells and nerve fiber terminals form an integrated network coordinating and modulating antimicrobial immunity, inflammation and pain signals during infection, and tissue homeostasis (100–102). For example, microglia were found to cross-present antigen after acquisition from adjacent olfactory sensory neurons and provide a front-line defense against a neuroinvasive nasal viral infection (103). Residential macrophages play a homeostatic role in the control of tissue innervation of brown-adipose tissue (104). Tissue-residential Tregs promote myelin regeneration upon damage of the central nervous system mediated by CCN2 (105). Infective agents, damaged host cells, and activated immune cells may initiate inflammatory signals in the nerve fiber environment (106). Such inflammatory chemical signals interacting with sensory nerve fiber terminals strongly associate with pain (107–109). It occurs via the synthesis and release of inflammatory mediators such as prostaglandin (PG) and interactions with neurotransmitters and their receptors (107, 110). Arachidonic acid is a key lipid mediator driving pain and inflammatory responses (111, 112) and is metabolized by cyclooxygenase and 5-lipoxygenase, resulting in the synthesis of PG and leukotrienes. This pathway is involved in the release of PGE2, IL-1β and ATP (113) and neuronal nociceptor activation (113–115). Thus, emerging evidence demonstrate how tissue resident immune cells, mucosal and submucosal glial cells, and neurons are actively involved in tissue homeostasis, inflammation, and pain pathophysiology (114–116). Even stress-susceptible cellular and behavioral phenotypes are causally mediated by dectin-1, an innate immune receptor expressed in intestinal γδ T cells (117). Nerves also have an extensive and well recognized role in immune regulation via neurotransmitter neuropeptides such as calcitonin gene-related peptide (CGRP) and substance P (118). Glial cells surrounding trigeminal neurons (119, 120), also produce PGE2 during tissue injury or inflammation and may regulate the sensory neuronal function both in paracrine and autocrine manners (121–123), involving CGRP and SP (124–126). CGRP is a crucial neurotransmitter of sensory neurons innervating the mucosa (127, 128), although it’s modulatory role in oral mucosal immunity needs new exploration.
Interactions between immune cells and neural cells in tumor progression
In the last two decades, cancer neuroscience has revealed the significant role of nerves in cancer progression (129). Since nerves regulate tumor progression and immunity, there is emerging interest in the nerve-immune-cancer axis. Studies in cutaneous cancers suggest that the increased innervation and damage of nerves in cancer can promote adverse outcomes by favoring pro-tumoral immunity. Nerves in oral squamous cell carcinomas exhibit damage (97, 130, 131). Moreover, increased nerve density is associated with poor outcomes (132). Cytotoxic CD8+ T lymphocytes were recently shown to express RAMP1 (receptor activity-modifying protein 1), the receptor for the neuropeptide CGRP, which is produced by transcription of the Calca gene in mice (133). Balood et al. showed that nociceptors release CGRP that induce RAMP1 on CD8+ T cells thereby leading to functional exhaustion (133). These CD8+ T cells exhibited concurrent expression of exhaustion markers such as PD-1+LAG3+TIM3+ and suppression of effector functions, resulting in tumor progression. Importantly, ablation of the nociceptor neurons inhibited tumor growth, which was reversed by intra-tumoral injection of CGRP. The importance of these findings lies in the ability to sensitize tumors to immunotherapy by interrupting the cancer-neuro-immune axis. Perineural Invasion (PNI) is another phenomenon that is highly correlated with poor prognosis, increased likelihood of metastasis, higher recurrence rates, node involvement, and decreased survival in OSCC (97, 130, 131, 134, 135). Although it is a high-risk adverse feature in OSCC, there are currently no treatments targeting PNI, which is important route of tumor dissemination in OSCC. It is seen in most of OSCC and provides a challenge to complete resection due to neural extension away from the primary tumor that is missed during surgical margin evaluation. PNI requires crosstalk between multiple cells, paracrine signaling, and direct matrix remodeling in the perineural niche (136–138). TIME-produced mediators activate and sensitize primary afferent neurons, contributing to inflammation, peripheral nerve injury, and sensitization that also underlie pain associated with PNI (128, 139–141). The heterogeneity in levels of neurotropism and the predictive value of nerve-tumor distance for survival among N0 patients emphasizes the need for mechanistic studies and characterization of interactions between immune cells, nerves, and cancer cells in TIME. Recent findings in cutaneous squamous cell carcinoma lend support to the importance of nerve-immune interactions in tumor resistance to immunotherapy (142). Non responders to anti-PD-1 therapy are more prone to nerve damage and immunosuppression. In mice, denervation enhanced tumor sensitivity to anti-PD-1 therapies. In a syngeneic orthotopic mouse model of oral squamous cell carcinoma, tumors in Calca knockout mice were smaller than in wild type mice, and had an increased anti-tumor immune response, including CD8+ T cells and CD4+ T cells (143). In a subsequent study, surgical denervation of the lingual nerve in mice, inhibited tumor growth, enhanced cytotoxic activity of CD8+ T cells and improved response to anti-PD1 immunotherapy (144). Findings from these oral cancer studies were corroborated in a recent in vitro and in vivo study (145).
Neuregulins (NRGs) and neurotrophic factors such as nerve growth factor (NGF) are expressed by leukocytes and can be involved in neuro-immune crosstalk. Amphiregulin (AREG) is a glycoprotein that was originally named Schwannoma-derived Growth Factor (SDGF) and is known to be neurotrophic (146). Schwann cell-derived AREG enhances nerve regeneration during peripheral nerve injury. AREG triggers EGFR signaling activating MAPK/ERK, PI3K/AKT, mTOR and STAT pathways in leukocytes (147). It is vital for tissue repair and the suppression of inflammation but is overexpressed in cancers (100, 148). AREG is also upregulated in Tregs in oral mucosa under chronic HIV infection, and may enhance their proliferation (16). GAL-1 is present in cytosolic compartments and as secreted form. It is known to promote cell–cell and cell-matrix communications and interact with glycoconjugates in TIME (149). GAL-1 overexpression is observed in the lymphocyte populations adjacent to areas of perineural spread and is associated with poor disease-free survival and overall survival (150). GAL-1 is also known to be upregulated in FOXP3+ Tregs supporting their differentiation, expansion, recruitment, and immunosuppressive potential (151). However, much work remains to define precise cellular sources of these proteins and their functions at the neuroimmune interface during PNI (Figure 1).
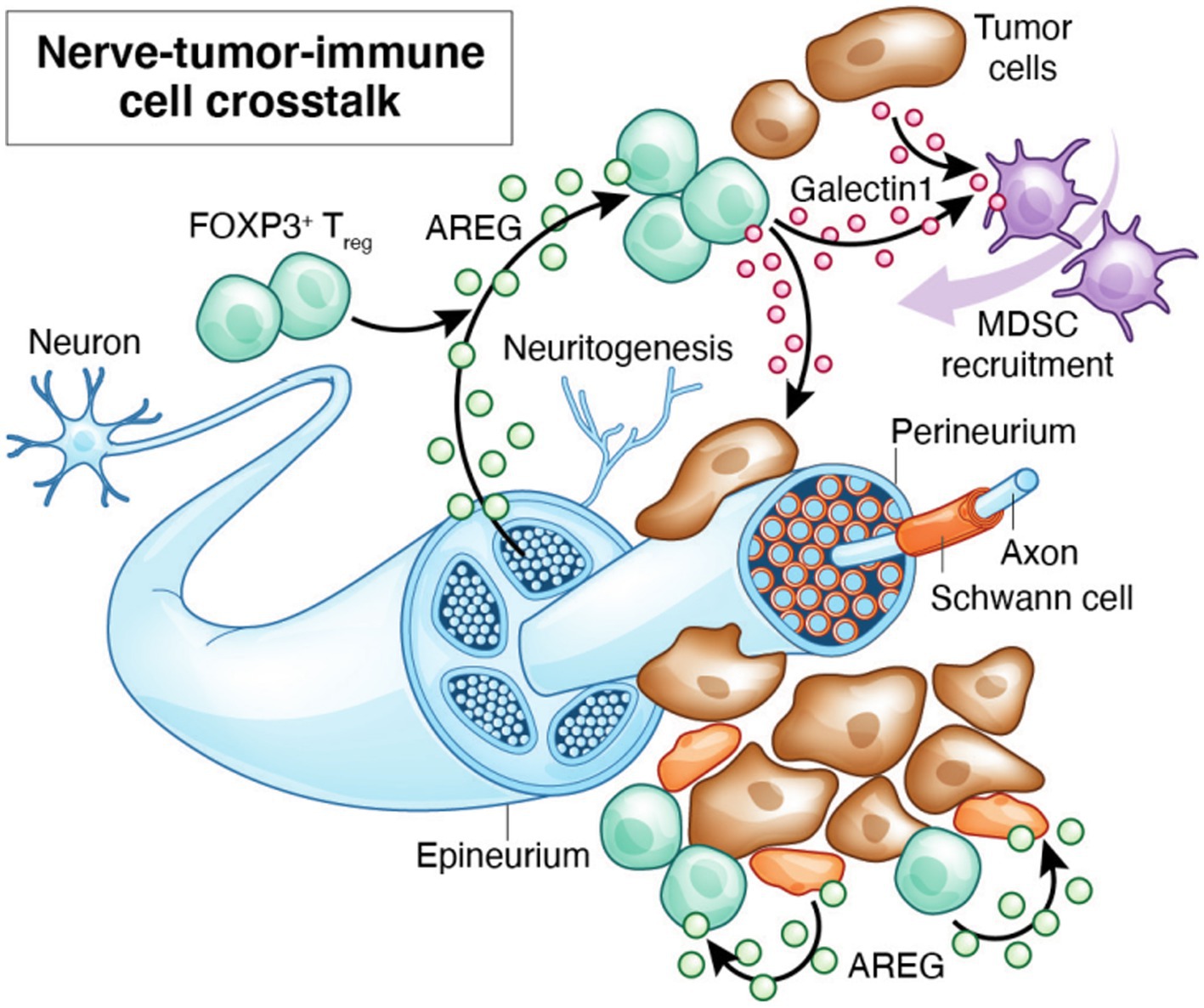
Figure 1. By releasing inflammatory mediators, tissue resident glial cells such as mucosal glia and Schwann cells and Tregs may modulate the synthesis and release of neurotransmitters and their receptors. Local cytokines may provide a positive feedback loop through which these cells interact in a reciprocal and recurrent fashion to induce neuropathological and immunosuppressive signals in PNI. These signals may be amplified by infections.
Schwann cells (SC) are glial-type cells that nurture neurons during development, promote myelination of mature peripheral nerves, and play a crucial role in neural regeneration and mediate bidirectional interactions between inflammation and pain (136, 137, 139, 152, 153). An increase in non-myelinating SC, like those responding to nerve injury, is observed in close proximity to pancreatic cancer cells in patient specimens and correlates with tumor invasion and diminished survival in patients (136–138). While GFAP+ and S100β+ glial cells can be found in oral mucosa (154, 155), it will be crucial to study if these cells support and modulate bidirectional neuronal and immune signaling in TIME (141, 156). Tumor cells can activate c-Jun–dependent reprogramming and kynurenine metabolism changes inducing them into non-myelinating/repair SC, which are involved in tumorigenesis (157). Except these recent reports in pancreatic cancer, the role of SC remains largely unexplored but could be an important cell-type explaining the heterogeneity of OSCC and amenable to therapeutic targeting in OSCC. Taken together, these studies suggest that the nerves and nerve-glia-immune interactions significantly impact cancer progression and could have a crucial role in the outcome of immunotherapy in oral cancer (Table 1).
Discussion
Cellular dysregulation related to microbiome dysbiosis, infections, and chronic inflammation that could result in malfunction at the neuroimmune interface contributes to initiation and progression of cancers. While infections are associated with tumors, precise mechanisms linking infection responses, immuno-senescence, immunosuppression in tumors are yet to be studied. A new understanding of neurons and glial cells in immune exhaustion and suppression, and tumor development and dissemination of squamous cell cancers are crucial for opening new avenues of investigation leading to better treatment selection and developing new treatment strategies.
Data availability statement
The original contributions presented in the study are included in the article/supplementary material, further inquiries can be directed to the corresponding author.
Ethics statement
The studies involving humans were approved by Institutional review board, UH. The studies were conducted in accordance with the local legislation and institutional requirements. The participants provided their written informed consent to participate in this study. The animal study was approved by Institutional Animal Care and Use Committee (IACUC). The study was conducted in accordance with the local legislation and institutional requirements.
Author contributions
ND'S: Writing – original draft, Writing – review & editing. PP: Conceptualization, Writing – original draft, Writing – review & editing.
Funding
The author(s) declare financial support was received for the research, authorship, and/or publication of this article. This work was supported by grants RO1DE026923 NIH/NIDCR funding (PP) and NIH/NIDCR R35 DE027551 and NIH/NCI R01 CA250214 (ND’S).
Conflict of interest
The authors declare that the research was conducted in the absence of any commercial or financial relationships that could be construed as a potential conflict of interest.
The author(s) declared that they were an editorial board member of Frontiers, at the time of submission. This had no impact on the peer review process and the final decision.
Publisher’s note
All claims expressed in this article are solely those of the authors and do not necessarily represent those of their affiliated organizations, or those of the publisher, the editors and the reviewers. Any product that may be evaluated in this article, or claim that may be made by its manufacturer, is not guaranteed or endorsed by the publisher.
References
1. Plummer, M, de Martel, C, Vignat, J, Ferlay, J, Bray, F, and Franceschi, S. Global burden of cancers attributable to infections in 2012: a synthetic analysis. Lancet Glob Health. (2016) 4:e609–16. doi: 10.1016/S2214-109X(16)30143-7
2. Mahalingam, SS, Jayaraman, S, and Pandiyan, P. Fungal colonization and infections-interactions with other human diseases. Pathogens. (2022) 11:212. doi: 10.3390/pathogens11020212
3. Greten, FR, and Grivennikov, SI. Inflammation and Cancer: triggers, mechanisms, and consequences. Immunity. (2019) 51:27–41. doi: 10.1016/j.immuni.2019.06.025
4. Bernaus, A, Blanco, S, and Sevilla, A. Glia crosstalk in Neuroinflammatory diseases. Front Cell Neurosci. (2020) 14:209. doi: 10.3389/fncel.2020.00209
5. Liebig, C, Ayala, G, Wilks, JA, Berger, DH, and Albo, D. Perineural invasion in cancer: a review of the literature. Cancer. (2009) 115:3379–91. doi: 10.1002/cncr.24396
6. Deeks, SG, Kitchen, CMR, Liu, L, Guo, H, Gascon, R, Narváez, AB, et al. Immune activation set point during early HIV infection predicts subsequent CD4+ T-cell changes independent of viral load. Blood. (2004) 104:942–7. doi: 10.1182/blood-2003-09-3333
7. Hunt, PW . Role of immune activation in HIV pathogenesis. Curr HIV/AIDS Rep. (2007) 4:42–7. doi: 10.1007/s11904-007-0007-8
8. Klatt, NR, Chomont, N, Douek, DC, and Deeks, SG. Immune activation and HIV persistence: implications for curative approaches to HIV infection. Immunol Rev. (2013) 254:326–42. doi: 10.1111/imr.12065
9. Brenchley, JM . Mucosal immunity in human and simian immunodeficiency lentivirus infections. Mucosal Immunol. (2013) 6:657–65. doi: 10.1038/mi.2013.15
10. Brenchley, JM, Price, DA, and Douek, DC. HIV disease: fallout from a mucosal catastrophe? Nat Immunol. (2006) 7:235–9. doi: 10.1038/ni1316
11. Klatt, NR, Funderburg, NT, and Brenchley, JM. Microbial translocation, immune activation, and HIV disease. Trends Microbiol. (2013) 21:6–13. doi: 10.1016/j.tim.2012.09.001
12. Ghosh, SK, McCormick, TS, Eapen, BL, Yohannes, E, Chance, MR, and Weinberg, A. Comparison of epigenetic profiles of human oral epithelial cells from HIV-positive (on HAART) and HIV-negative subjects. Epigenetics. (2013) 8:703–9. doi: 10.4161/epi.25028
13. Yohannes, E, Ghosh, SK, Jiang, B, McCormick, TS, Weinberg, A, Hill, E, et al. Proteomic signatures of human oral epithelial cells in HIV-infected subjects. PLoS One. (2011) 6:e27816. doi: 10.1371/journal.pone.0027816
14. George, J, Wagner, W, Lewis, MG, and Mattapallil, JJ. Significant depletion of CD4(+) T cells occurs in the Oral mucosa during simian immunodeficiency virus infection with the infected CD4(+) T cell reservoir continuing to persist in the Oral mucosa during antiretroviral therapy. J Immunol Res. (2015) 2015:673815. doi: 10.1155/2015/673815
15. Mahalingam, SS, Jayaraman, S, Bhaskaran, N, Schneider, E, Faddoul, F, Paes da Silva, A, et al. Polyamine metabolism impacts T cell dysfunction in the oral mucosa of people living with HIV. Nat Commun. (2023) 14:399. doi: 10.1038/s41467-023-36163-2
16. Bhaskaran, N, Schneider, E, Faddoul, F, Paes da Silva, A, Asaad, R, Talla, A, et al. Oral immune dysfunction is associated with the expansion of FOXP3+PD-1+Amphiregulin+ T cells during HIV infection. Nat Commun. (2021) 12:5143. doi: 10.1038/s41467-021-25340-w
17. Pandiyan, P, Younes, SA, Ribeiro, SP, Talla, A, McDonald, D, Bhaskaran, N, et al. Mucosal regulatory T cells and T helper 17 cells in HIV associated immune activation. Front Immunol. (2016) 7:228. doi: 10.3389/fimmu.2016.00228
18. González, OA, Li, M, Ebersole, JL, and Huang, CB. HIV-1 reactivation induced by the periodontal pathogens Fusobacterium nucleatum and Porphyromonas gingivalis involves toll-like receptor 2 [corrected] and 9 activation in monocytes/macrophages. Clin Vaccine Immunol. (2010) 17:1417–27. doi: 10.1128/CVI.00009-10
19. Mukherjee, PK, Chandra, J, Retuerto, M, Sikaroodi, M, Brown, RE, Jurevic, R, et al. Oral mycobiome analysis of HIV-infected patients: identification of Pichia as an antagonist of opportunistic fungi. PLoS Pathog. (2014) 10:e1003996. doi: 10.1371/journal.ppat.1003996
20. Ghannoum, MA, Jurevic, RJ, Mukherjee, PK, Cui, F, Sikaroodi, M, Naqvi, A, et al. Characterization of the oral fungal microbiome (mycobiome) in healthy individuals. PLoS Pathog. (2010) 6:e1000713. doi: 10.1371/journal.ppat.1000713
21. Aas, JA, Paster, BJ, Stokes, LN, Olsen, I, and Dewhirst, FE. Defining the normal bacterial flora of the oral cavity. J Clin Microbiol. (2005) 43:5721–32. doi: 10.1128/JCM.43.11.5721-5732.2005
22. Aas, JA, Barbuto, SM, Alpagot, T, Olsen, I, Dewhirst, FE, and Paster, BJ. Subgingival plaque microbiota in HIV positive patients. J Clin Periodontol. (2007) 34:189–95. doi: 10.1111/j.1600-051X.2006.01034.x
23. Robinson, PG . The oral manifestations of HIV infection. Int J STD AIDS. (1997) 8:668–74. doi: 10.1258/0956462971919039
24. Robinson, PG, Sheiham, A, Challacombe, SJ, and Zakrzewska, JM. Periodontal health and HIV infection. Oral Dis. (1997) 3:S149–52. doi: 10.1111/j.1601-0825.1997.tb00349.x
25. Robinson, PG, Challacombe, SJ, Sheiham, A, and Zakrzewska, JM. Is erythematous candidiasis associated with advanced HIV disease? Oral Dis. (1997) 3:S116–8. doi: 10.1111/j.1601-0825.1997.tb00339.x
26. Patton, LL . Oral lesions associated with human immunodeficiency virus disease. Dent Clin N Am. (2013) 57:673–98. doi: 10.1016/j.cden.2013.07.005
27. Weinberg, A, Tugizov, S, Pandiyan, P, Jin, G, Rakshit, S, Vyakarnam, A, et al. Innate immune mechanisms to oral pathogens in oral mucosa of HIV-infected individuals. Oral Dis. (2020) 26:69–79. doi: 10.1111/odi.13470
28. Catalfamo, M, Le Saout, C, and Lane, HC. The role of cytokines in the pathogenesis and treatment of HIV infection. Cytokine Growth Factor Rev. (2012) 23:207–14. doi: 10.1016/j.cytogfr.2012.05.007
29. Tenorio, AR, Zheng, Y, Bosch, RJ, Krishnan, S, Rodriguez, B, Hunt, PW, et al. Soluble markers of inflammation and coagulation but not T-cell activation predict non-AIDS-defining morbid events during suppressive antiretroviral treatment. J Infect Dis. (2014) 210:1248–59. doi: 10.1093/infdis/jiu254
30. Gabuzda, D, Jamieson, BD, Collman, RG, Lederman, MM, Burdo, TH, Deeks, SG, et al. Pathogenesis of aging and age-related comorbidities in people with HIV: highlights from the HIV ACTION workshop. Pathog Immun. (2020) 5:143–74. doi: 10.20411/pai.v5i1.365
31. Chen, L, Feng, Z, Yue, H, Bazdar, D, Mbonye, U, Zender, C, et al. Exosomes derived from HIV-1-infected cells promote growth and progression of cancer via HIV TAR RNA. Nat Commun. (2018) 9:4585. doi: 10.1038/s41467-018-07006-2
32. Ellington, TD, Henley, SJ, Senkomago, V, O’Neil, ME, Wilson, RJ, Singh, S, et al. Trends in incidence of cancers of the Oral cavity and pharynx-United States 2007-2016. MMWR Morb Mortal Wkly Rep. (2020) 69:433–8. doi: 10.15585/mmwr.mm6915a1
33. Barsouk, A, Aluru, JS, Rawla, P, Saginala, K, and Barsouk, A. Epidemiology, risk factors, and prevention of head and Neck squamous cell carcinoma. Med Sci (Basel). (2023) 11:42. doi: 10.3390/medsci11020042
34. Coletta, RD, Yeudall, WA, and Salo, T. Grand challenges in Oral cancers. Front Oral Health. (2020) 1:3. doi: 10.3389/froh.2020.00003
35. Feng, L, Yin, K, Zhang, S, Chen, Z, Bao, Y, and Li, T. Anti-PD-1 therapy is beneficial for the survival of patients with Oral squamous cell carcinoma. Cancer Manag Res. (2022) 14:2723–31. doi: 10.2147/CMAR.S368738
36. Seiwert, TY, Burtness, B, Mehra, R, Weiss, J, Berger, R, Eder, JP, et al. Safety and clinical activity of pembrolizumab for treatment of recurrent or metastatic squamous cell carcinoma of the head and neck (KEYNOTE-012): an open-label, multicentre, phase 1b trial. Lancet Oncol. (2016) 17:956–65. doi: 10.1016/S1470-2045(16)30066-3
37. Ferris, RL, Blumenschein, G Jr, Fayette, J, Guigay, J, Colevas, AD, Licitra, L, et al. Nivolumab for recurrent squamous-cell carcinoma of the head and Neck. N Engl J Med. (2016) 375:1856–67. doi: 10.1056/NEJMoa1602252
38. Hamid, O, Robert, C, Daud, A, Hodi, FS, Hwu, WJ, Kefford, R, et al. Five-year survival outcomes for patients with advanced melanoma treated with pembrolizumab in KEYNOTE-001. Ann Oncol. (2019) 30:582–8. doi: 10.1093/annonc/mdz011
39. Antonia, SJ, Borghaei, H, Ramalingam, SS, Horn, L, de Castro Carpeño, J, Pluzanski, A, et al. Four-year survival with nivolumab in patients with previously treated advanced non-small-cell lung cancer: a pooled analysis. Lancet Oncol. (2019) 20:1395–408. doi: 10.1016/S1470-2045(19)30407-3
40. Robert, C, Schachter, J, Long, GV, Arance, A, Grob, JJ, Mortier, L, et al. Pembrolizumab versus Ipilimumab in Advanced Melanoma. N Engl J Med. (2015) 372:2521–32. doi: 10.1056/NEJMoa1503093
41. McLane, LM, Abdel-Hakeem, MS, and Wherry, EJ. CD8 T cell exhaustion during chronic viral infection and Cancer. Annu Rev Immunol. (2019) 37:457–95. doi: 10.1146/annurev-immunol-041015-055318
42. Hashimoto, M, Kamphorst, AO, Im, SJ, Kissick, HT, Pillai, RN, Ramalingam, SS, et al. CD8 T cell exhaustion in chronic infection and Cancer: opportunities for interventions. Annu Rev Med. (2018) 69:301–18. doi: 10.1146/annurev-med-012017-043208
43. Rosato, PC, Wijeyesinghe, S, Stolley, JM, Nelson, CE, Davis, RL, Manlove, LS, et al. Virus-specific memory T cells populate tumors and can be repurposed for tumor immunotherapy. Nat Commun. (2019) 10:567. doi: 10.1038/s41467-019-08534-1
44. Eberhardt, CS, Kissick, HT, Patel, MR, Cardenas, MA, Prokhnevska, N, Obeng, RC, et al. Functional HPV-specific PD-1(+) stem-like CD8 T cells in head and neck cancer. Nature. (2021) 597:279–84. doi: 10.1038/s41586-021-03862-z
45. Alfei, F, Kanev, K, Hofmann, M, Wu, M, Ghoneim, HE, Roelli, P, et al. TOX reinforces the phenotype and longevity of exhausted T cells in chronic viral infection. Nature. (2019) 571:265–9. doi: 10.1038/s41586-019-1326-9
46. Ren, X, Zhang, L, Zhang, Y, Li, Z, Siemers, N, and Zhang, Z. Insights gained from single-cell analysis of immune cells in the tumor microenvironment. Annu Rev Immunol. (2021) 39:583–609. doi: 10.1146/annurev-immunol-110519-071134
47. Gebhardt, T, Park, SL, and Parish, IA. Stem-like exhausted and memory CD8(+) T cells in cancer. Nat Rev Cancer. (2023) 23:780–98. doi: 10.1038/s41568-023-00615-0
48. van der Leun, AM, Thommen, DS, and Schumacher, TN. CD8(+) T cell states in human cancer: insights from single-cell analysis. Nat Rev Cancer. (2020) 20:218–32. doi: 10.1038/s41568-019-0235-4
49. Pandiyan, P, Zheng, L, and Lenardo, MJ. The molecular mechanisms of regulatory T cell immunosuppression. Front Immunol. (2011) 2:60. doi: 10.3389/fimmu.2011.00060
50. Gratz, IK, Rosenblum, MD, and Abbas, AK. The life of regulatory T cells. Ann N Y Acad Sci. (2013) 1283:8–12. doi: 10.1111/nyas.12011
51. Barron, L, Dooms, H, Hoyer, KK, Kuswanto, W, Hofmann, J, O’Gorman, WE, et al. Cutting edge: mechanisms of IL-2-dependent maintenance of functional regulatory T cells. J Immunol. (2010) 185:6426–30. doi: 10.4049/jimmunol.0903940
52. Tai, X, Erman, B, Alag, A, Mu, J, Kimura, M, Katz, G, et al. Foxp3 transcription factor is proapoptotic and lethal to developing regulatory T cells unless counterbalanced by cytokine survival signals. Immunity. (2013) 38:1116–28. doi: 10.1016/j.immuni.2013.02.022
53. Facciabene, A, Motz, GT, and Coukos, G. T-regulatory cells: key players in tumor immune escape and angiogenesis. Cancer Res. (2012) 72:2162–71. doi: 10.1158/0008-5472.CAN-11-3687
54. Bronte, V, Brandau, S, Chen, SH, Colombo, MP, Frey, AB, Greten, TF, et al. Recommendations for myeloid-derived suppressor cell nomenclature and characterization standards. Nat Commun. (2016) 7:12150. doi: 10.1038/ncomms12150
55. Bhaskaran, N, Jayaraman, S, Quigley, C, Mamileti, P, Ghannoum, M, Weinberg, A, et al. The role of Dectin-1 signaling in altering tumor immune microenvironment in the context of aging. Front Oncol. (2021) 11:669066. doi: 10.3389/fonc.2021.669066
56. Bhaskaran, N, Quigley, C, Paw, C, Butala, S, Schneider, E, and Pandiyan, P. Role of short chain fatty acids in controlling Tregs and immunopathology during mucosal infection. Front Microbiol. (2018) 9:1995. doi: 10.3389/fmicb.2018.01995
57. Pandiyan, P, Bhaskaran, N, Zou, M, Schneider, E, Jayaraman, S, and Huehn, J. Microbiome dependent regulation of Tregs and Th17 cells in mucosa. Front Immunol. (2019) 10:78–94. doi: 10.3389/fimmu.2019.00426
58. Bhaskaran, N, Faddoul, F, Paes da Silva, A, Jayaraman, S, Schneider, E, Mamileti, P, et al. IL-1beta-MyD88-mTOR Axis promotes immune-protective IL-17A(+)Foxp3(+) cells during mucosal infection and is dysregulated with aging. Front Immunol. (2020) 11:595936. doi: 10.3389/fimmu.2020.595936
59. Bhaskaran, N, Quigley, C, Weinberg, A, Huang, A, Popkin, D, and Pandiyan, P. Transforming growth factor-beta1 sustains the survival of Foxp3(+) regulatory cells during late phase of oropharyngeal candidiasis infection. Mucosal Immunol. (2016) 9:1015–26. doi: 10.1038/mi.2015.115
60. Mandal, R, Şenbabaoğlu, Y, Desrichard, A, Havel, JJ, Dalin, MG, Riaz, N, et al. The head and neck cancer immune landscape and its immunotherapeutic implications. JCI Insight. (2016) 1:e89829. doi: 10.1172/jci.insight.89829
61. Tay, C, Qian, Y, and Sakaguchi, S. Hyper-progressive disease: the potential role and consequences of T-regulatory cells foiling anti-PD-1 Cancer immunotherapy. Cancers (Basel). (2020) 13:48. doi: 10.3390/cancers13010048
62. Watanabe, Y, Katou, F, Ohtani, H, Nakayama, T, Yoshie, O, and Hashimoto, K. Tumor-infiltrating lymphocytes, particularly the balance between CD8(+) T cells and CCR4(+) regulatory T cells, affect the survival of patients with oral squamous cell carcinoma. Oral Surg Oral Med Oral Pathol Oral Radiol Endod. (2010) 109:744–52. doi: 10.1016/j.tripleo.2009.12.015
63. Chu, M, Su, YX, Wang, L, Zhang, TH, Liang, YJ, Liang, LZ, et al. Myeloid-derived suppressor cells contribute to oral cancer progression in 4NQO-treated mice. Oral Dis. (2012) 18:67–73. doi: 10.1111/j.1601-0825.2011.01846.x
64. Shive, C, and Pandiyan, P. Inflammation, immune senescence, and dysregulated immune regulation in the elderly. Front Aging. (2022) 3:840827. doi: 10.3389/fragi.2022.840827
65. Bhaskaran, N, Cohen, S, Zhang, Y, Weinberg, A, and Pandiyan, P. TLR-2 signaling promotes IL-17A production in CD4+CD25+Foxp3+ regulatory cells during oropharyngeal candidiasis. Pathogens. (2015) 4:90–110. doi: 10.3390/pathogens4010090
66. Bhaskaran, N, Weinberg, A, and Pandiyan, P. Th17 inflammation model of oropharyngeal candidiasis in immunodeficient mice. J Vis Exp. (2015) 96:e52538. doi: 10.3791/52538
67. Pandiyan, P, and Lenardo, M. Comment on "cutting edge: regulatory T cells do not mediate suppression via programmed cell death pathways". J Immunol. (2012) 188:5203–4; author reply 5204-5. doi: 10.4049/jimmunol.1290023
68. Pandiyan, P, and Zhu, J. Origin and functions of pro-inflammatory cytokine producing Foxp3(+) regulatory T cells. Cytokine. (2015) 76:13–24. doi: 10.1016/j.cyto.2015.07.005
69. O'Grady, JF, and Reade, PC. Candida albicans as a promoter of oral mucosal neoplasia. Carcinogenesis. (1992) 13:783–6. doi: 10.1093/carcin/13.5.783
70. Bode, K, Bujupi, F, Link, C, Hein, T, Zimmermann, S, Peiris, D, et al. Dectin-1 binding to Annexins on apoptotic cells induces peripheral immune tolerance via NADPH Oxidase-2. Cell Rep. (2019) 29:4435–4446.e9. doi: 10.1016/j.celrep.2019.11.086
71. Deerhake, ME, and Shinohara, ML. Emerging roles of Dectin-1 in noninfectious settings and in the CNS. Trends Immunol. (2021) 42:891–903. doi: 10.1016/j.it.2021.08.005
72. Swanson, KV, Deng, M, and Ting, JP. The NLRP3 inflammasome: molecular activation and regulation to therapeutics. Nat Rev Immunol. (2019) 19:477–89. doi: 10.1038/s41577-019-0165-0
73. Gross, O, Poeck, H, Bscheider, M, Dostert, C, Hannesschläger, N, Endres, S, et al. Syk kinase signalling couples to the Nlrp3 inflammasome for anti-fungal host defence. Nature. (2009) 459:433–6. doi: 10.1038/nature07965
74. Wu, T, Hong, Y, Jia, L, Wu, J, Xia, J, Wang, J, et al. Modulation of IL-1beta reprogrammes the tumor microenvironment to interrupt oral carcinogenesis. Sci Rep. (2016) 6:20208. doi: 10.1038/srep20208
75. Downs-Canner, S, Berkey, S, Delgoffe, GM, Edwards, RP, Curiel, T, Odunsi, K, et al. Suppressive IL-17A(+)Foxp3(+) and ex-Th17 IL-17A(neg)Foxp3(+) Treg cells are a source of tumour-associated Treg cells. Nat Commun. (2017) 8:14649. doi: 10.1038/ncomms14649
76. MacIver, NJ, Michalek, RD, and Rathmell, JC. Metabolic regulation of T lymphocytes. Annu Rev Immunol. (2013) 31:259–83. doi: 10.1146/annurev-immunol-032712-095956
77. Prochnicki, T, and Latz, E. Inflammasomes on the crossroads of innate immune recognition and metabolic control. Cell Metab. (2017) 26:71–93. doi: 10.1016/j.cmet.2017.06.018
78. Karki, R, and Kanneganti, TD. Diverging inflammasome signals in tumorigenesis and potential targeting. Nat Rev Cancer. (2019) 19:197–214. doi: 10.1038/s41568-019-0123-y
79. Hamarsheh, S, and Zeiser, R. NLRP3 Inflammasome activation in Cancer: a double-edged sword. Front Immunol. (2020) 11:1444. doi: 10.3389/fimmu.2020.01444
80. Ding, J, Wang, K, Liu, W, She, Y, Sun, Q, Shi, J, et al. Pore-forming activity and structural autoinhibition of the gasdermin family. Nature. (2016) 535:111–6. doi: 10.1038/nature18590
81. Yu, J, Nagasu, H, Murakami, T, Hoang, H, Broderick, L, Hoffman, HM, et al. Inflammasome activation leads to Caspase-1-dependent mitochondrial damage and block of mitophagy. Proc Natl Acad Sci USA. (2014) 111:15514–9. doi: 10.1073/pnas.1414859111
82. Sanman, LE, Qian, Y, Eisele, NA, Ng, TM, van der Linden, WA, Monack, DM, et al. Disruption of glycolytic flux is a signal for inflammasome signaling and pyroptotic cell death. eLife. (2016) 5:e13663. doi: 10.7554/eLife.13663
83. Yang, Q, Liu, R, Yu, Q, Bi, Y, and Liu, G. Metabolic regulation of inflammasomes in inflammation. Immunology. (2019) 157:95–109. doi: 10.1111/imm.13056
84. Wang, Y, Ning, X, Gao, P, Wu, S, Sha, M, Lv, M, et al. Inflammasome activation triggers Caspase-1-mediated cleavage of cGAS to regulate responses to DNA virus infection. Immunity. (2017) 46:393–404. doi: 10.1016/j.immuni.2017.02.011
85. Lin, HC, Chen, YJ, Wei, YH, Lin, HA, Chen, CC, Liu, TF, et al. Lactic acid fermentation is required for NLRP3 Inflammasome activation. Front Immunol. (2021) 12:630380. doi: 10.3389/fimmu.2021.630380
86. Moon, JS, Hisata, S, Park, MA, DeNicola, GM, Ryter, SW, Nakahira, K, et al. mTORC1-induced HK1-dependent glycolysis regulates NLRP3 Inflammasome activation. Cell Rep. (2015) 12:102–15. doi: 10.1016/j.celrep.2015.05.046
87. Ouyang, W, Beckett, O, Ma, Q, Paik, JH, DePinho, RA, and Li, MO. Foxo proteins cooperatively control the differentiation of Foxp3+ regulatory T cells. Nat Immunol. (2010) 11:618–27. doi: 10.1038/ni.1884
88. Datta, SR, Brunet, A, and Greenberg, ME. Cellular survival: a play in three Akts. Genes Dev. (1999) 13:2905–27. doi: 10.1101/gad.13.22.2905
89. So, T, and Croft, M. Regulation of PI-3-kinase and Akt signaling in T lymphocytes and other cells by TNFR family molecules. Front Immunol. (2013) 4:139. doi: 10.3389/fimmu.2013.00139
90. Pandiyan, P, Gärtner, D, Soezeri, O, Radbruch, A, Schulze-Osthoff, K, and Brunner-Weinzierl, MC. CD152 (CTLA-4) determines the unequal resistance of Th1 and Th2 cells against activation-induced cell death by a mechanism requiring PI3 kinase function. J Exp Med. (2004) 199:831–42. doi: 10.1084/jem.20031058
91. Shrestha, S, Yang, K, Guy, C, Vogel, P, Neale, G, and Chi, H. Treg cells require the phosphatase PTEN to restrain TH1 and TFH cell responses. Nat Immunol. (2015) 16:178–87. doi: 10.1038/ni.3076
92. Araki, K, Turner, AP, Shaffer, VO, Gangappa, S, Keller, SA, Bachmann, MF, et al. mTOR regulates memory CD8 T-cell differentiation. Nature. (2009) 460:108–12. doi: 10.1038/nature08155
93. Ron-Harel, N, Ghergurovich, JM, Notarangelo, G, LaFleur, MW, Tsubosaka, Y, Sharpe, AH, et al. T cell activation depends on extracellular alanine. Cell Rep. (2019) 28:3011–3021.e4. doi: 10.1016/j.celrep.2019.08.034
94. Muller, T . Morphological differences among nerve fiber endings in the rat oral mucosa as revealed by methylene blue staining. Histol Histopathol. (1996) 11:659–66.
95. Moayedi, Y, Duenas-Bianchi, LF, and Lumpkin, EA. Somatosensory innervation of the oral mucosa of adult and aging mice. Sci Rep. (2018) 8:9975. doi: 10.1038/s41598-018-28195-2
96. Kamrani, P., and Sadiq, N.M., Anatomy, head and neck, oral cavity (mouth), in StatPearls. (2024): Treasure Island (FL) ineligible companies. Disclosure: Nazia Sadiq declares no relevant financial relationships with ineligible companies.
97. D'Silva, NJ, Perez-Pacheco, C, and Schmitd, LB. The 3D's of neural phenotypes in Oral Cancer: distance, diameter, and density. Adv Biol (Weinh). (2023) 7:e2200188. doi: 10.1002/adbi.202200188
98. Koller, A., and Sapra, A., Anatomy, head and neck, Oral Gingiva, in StatPearls. (2024): Treasure Island (FL) ineligible companies. Disclosure: Amit Sapra declares no relevant financial relationships with ineligible companies.
99. Sharabi, A.F., and Winters, R., Glossitis, in StatPearls. (2024): Treasure Island (FL) ineligible companies. Disclosure: Ryan Winters declares no relevant financial relationships with ineligible companies.
100. Tsou, AM, Yano, H, Parkhurst, CN, Mahlakõiv, T, Chu, C, Zhang, W, et al. Neuropeptide regulation of non-redundant ILC2 responses at barrier surfaces. Nature. (2022) 611:787–93. doi: 10.1038/s41586-022-05297-6
101. Chiu, IM, Heesters, BA, Ghasemlou, N, von Hehn, CA, Zhao, F, Tran, J, et al. Bacteria activate sensory neurons that modulate pain and inflammation. Nature. (2013) 501:52–7. doi: 10.1038/nature12479
102. Talbot, S, Abdulnour, REE, Burkett, PR, Lee, S, Cronin, SJF, Pascal, MA, et al. Silencing nociceptor neurons reduces allergic airway inflammation. Neuron. (2015) 87:341–54. doi: 10.1016/j.neuron.2015.06.007
103. Moseman, EA, Blanchard, AC, Nayak, D, and McGavern, DB. T cell engagement of cross-presenting microglia protects the brain from a nasal virus infection. Sci Immunol. (2020) 5:eabb1817. doi: 10.1126/sciimmunol.abb1817
104. Wolf, Y, Boura-Halfon, S, Cortese, N, Haimon, Z, Sar Shalom, H, Kuperman, Y, et al. Brown-adipose-tissue macrophages control tissue innervation and homeostatic energy expenditure. Nat Immunol. (2017) 18:665–74. doi: 10.1038/ni.3746
105. Dombrowski, Y, O'Hagan, T, Dittmer, M, Penalva, R, Mayoral, SR, Bankhead, P, et al. Regulatory T cells promote myelin regeneration in the central nervous system. Nat Neurosci. (2017) 20:674–80. doi: 10.1038/nn.4528
106. Chavan, SS, Pavlov, VA, and Tracey, KJ. Mechanisms and therapeutic relevance of neuro-immune communication. Immunity. (2017) 46:927–42. doi: 10.1016/j.immuni.2017.06.008
107. Vanderwall, AG, and Milligan, ED. Cytokines in pain: harnessing endogenous anti-inflammatory signaling for improved pain management. Front Immunol. (2019) 10:3009. doi: 10.3389/fimmu.2019.03009
108. Mailhot, B, Christin, M, Tessandier, N, Sotoudeh, C, Bretheau, F, Turmel, R, et al. Neuronal interleukin-1 receptors mediate pain in chronic inflammatory diseases. J Exp Med. (2020) 217:e20191430. doi: 10.1084/jem.20191430
109. Zhang, R, Lai, M, and Wang, D. Psychologic impacts on diabetic neuropathic pain. Curr Pain Headache Rep. (2022) 26:423–7. doi: 10.1007/s11916-022-01040-y
110. Basbaum, AI, Bautista, DM, Scherrer, G, and Julius, D. Cellular and molecular mechanisms of pain. Cell. (2009) 139:267–84. doi: 10.1016/j.cell.2009.09.028
111. Enyedi, B, Jelcic, M, and Niethammer, P. The cell nucleus serves as a Mechanotransducer of tissue damage-induced inflammation. Cell. (2016) 165:1160–70. doi: 10.1016/j.cell.2016.04.016
112. Das, UN . Essential fatty acids and their metabolites in the pathobiology of inflammation and its resolution. Biomol Ther. (2021) 11:1873. doi: 10.3390/biom11121873
113. Wang, B, Wu, L, Chen, J, Dong, L, Chen, C, Wen, Z, et al. Metabolism pathways of arachidonic acids: mechanisms and potential therapeutic targets. Signal Transduct Target Ther. (2021) 6:94. doi: 10.1038/s41392-020-00443-w
114. Wang, H, Foong, JPP, Harris, NL, and Bornstein, JC. Enteric neuroimmune interactions coordinate intestinal responses in health and disease. Mucosal Immunol. (2022) 15:27–39. doi: 10.1038/s41385-021-00443-1
115. Wang, S, Nie, X, Siddiqui, Y, Wang, X, Arora, V, Fan, X, et al. Nociceptor neurons magnify host responses to aggravate periodontitis. J Dent Res. (2022) 101:812–20. doi: 10.1177/00220345211069956
116. Seguella, L, and Gulbransen, BD. Enteric glial biology, intercellular signalling and roles in gastrointestinal disease. Nat Rev Gastroenterol Hepatol. (2021) 18:571–87. doi: 10.1038/s41575-021-00423-7
117. Zhu, X, Sakamoto, S, Ishii, C, Smith, MD, Ito, K, Obayashi, M, et al. Dectin-1 signaling on colonic gammadelta T cells promotes psychosocial stress responses. Nat Immunol. (2023) 24:625–36. doi: 10.1038/s41590-023-01447-8
118. Jacobson, A, Yang, D, Vella, M, and Chiu, IM. The intestinal neuro-immune axis: crosstalk between neurons, immune cells, and microbes. Mucosal Immunol. (2021) 14:555–65. doi: 10.1038/s41385-020-00368-1
119. Shankland, WE . Nociceptive trigeminal inhibition--tension suppression system: a method of preventing migraine and tension headaches. Compend Contin Educ Dent. (2001) 22:1075–1080, 1082.
120. Shankland, WE 2nd . The trigeminal nerve. Part IV: the mandibular division. Cranio. (2001) 19:153–61. doi: 10.1080/08869634.2001.11746164
121. Capuano, A, De Corato, A, Lisi, L, Tringali, G, Navarra, P, and Dello Russo, C. Proinflammatory-activated trigeminal satellite cells promote neuronal sensitization: relevance for migraine pathology. Mol Pain. (2009) 5:43. doi: 10.1186/1744-8069-5-43
122. Jang, Y, Kim, M, and Hwang, SW. Molecular mechanisms underlying the actions of arachidonic acid-derived prostaglandins on peripheral nociception. J Neuroinflammation. (2020) 17:30. doi: 10.1186/s12974-020-1703-1
123. Mense, S., and Gerwin, R.D. (eds.), Functional Anatomy of muscle: muscle, nociceptors and afferent fibers. Textbook: muscle pain: understanding the mechanisms, (2010). Chapter 2. p. 17–48.
124. Suzuki, N, Hardebo, JE, and Owman, C. Origins and pathways of cerebrovascular nerves storing substance P and calcitonin gene-related peptide in rat. Neuroscience. (1989) 31:427–38. doi: 10.1016/0306-4522(89)90385-0
125. Suzuki, N, Hardebo, JE, and Owman, C. Trigeminal fibre collaterals storing substance P and calcitonin gene-related peptide associate with ganglion cells containing choline acetyltransferase and vasoactive intestinal polypeptide in the sphenopalatine ganglion of the rat. An axon reflex modulating parasympathetic ganglionic activity? Neuroscience. (1989) 30:595–604.
126. Edvinsson, L, Ekman, R, Jansen, I, McCulloch, J, and Uddman, R. Calcitonin gene-related peptide and cerebral blood vessels: distribution and vasomotor effects. J Cereb Blood Flow Metab. (1987) 7:720–8. doi: 10.1038/jcbfm.1987.126
127. Terenghi, G, Polak, JM, Rodrigo, J, Mulderry, PK, and Bloom, SR. Calcitonin gene-related peptide-immunoreactive nerves in the tongue, epiglottis and pharynx of the rat: occurrence, distribution and origin. Brain Res. (1986) 365:1–14. doi: 10.1016/0006-8993(86)90716-X
128. Scheff, NN, Ye, Y, Bhattacharya, A, MacRae, J, Hickman, DN, Sharma, AK, et al. Tumor necrosis factor alpha secreted from oral squamous cell carcinoma contributes to cancer pain and associated inflammation. Pain. (2017) 158:2396–409. doi: 10.1097/j.pain.0000000000001044
129. Monje, M, Borniger, JC, D’Silva, NJ, Deneen, B, Dirks, PB, Fattahi, F, et al. Roadmap for the emerging field of Cancer neuroscience. Cell. (2020) 181:219–22. doi: 10.1016/j.cell.2020.03.034
130. Schmitd, LB, Perez-Pacheco, C, Bellile, EL, Wu, W, Casper, K, Mierzwa, M, et al. Spatial and transcriptomic analysis of Perineural invasion in Oral Cancer. Clin Cancer Res. (2022) 28:3557–72. doi: 10.1158/1078-0432.CCR-21-4543
131. Schmitd, LB, Perez-Pacheco, C, and D'Silva, NJ. Nerve density in cancer: less is better. FASEB Bioadv. (2021) 3:773–86. doi: 10.1096/fba.2021-00046
132. Perez-Pacheco, C, Schmitd, LB, Furgal, A, Bellile, EL, Liu, M, Fattah, A, et al. Increased nerve density adversely affects outcome in Oral Cancer. Clin Cancer Res. (2023) 29:2501–12. doi: 10.1158/1078-0432.CCR-22-3496
133. Balood, M, Ahmadi, M, Eichwald, T, Ahmadi, A, Majdoubi, A, Roversi, K, et al. Nociceptor neurons affect cancer immunosurveillance. Nature. (2022) 611:405–12. doi: 10.1038/s41586-022-05374-w
134. Schmitd, LB, Beesley, LJ, Russo, N, Bellile, EL, Inglehart, RC, Liu, M, et al. Redefining Perineural invasion: integration of biology with clinical outcome. Neoplasia. (2018) 20:657–67. doi: 10.1016/j.neo.2018.04.005
135. Schmitd, LB, Scanlon, CS, and D'Silva, NJ. Perineural invasion in head and Neck Cancer. J Dent Res. (2018) 97:742–50. doi: 10.1177/0022034518756297
136. Deborde, S, Gusain, L, Powers, A, Marcadis, A, Yu, Y, Chen, CH, et al. Reprogrammed Schwann cells organize into dynamic tracks that promote pancreatic Cancer invasion. Cancer Discov. (2022) 12:2454–73. doi: 10.1158/2159-8290.CD-21-1690
137. Deborde, S, Omelchenko, T, Lyubchik, A, Zhou, Y, He, S, McNamara, WF, et al. Schwann cells induce cancer cell dispersion and invasion. J Clin Invest. (2016) 126:1538–54. doi: 10.1172/JCI82658
138. Deborde, S, and Wong, RJ. The role of Schwann cells in Cancer. Adv Biol (Weinh). (2022) 6:e2200089. doi: 10.1002/adbi.202200089
139. Salvo, E, Campana, WM, Scheff, NN, Nguyen, TH, Jeong, SH, Wall, I, et al. Peripheral nerve injury and sensitization underlie pain associated with oral cancer perineural invasion. Pain. (2020) 161:2592–602. doi: 10.1097/j.pain.0000000000001986
140. Ye, J, Guo, W, Wang, C, Egelston, CA, D'Apuzzo, M, Shankar, G, et al. Peritumoral immune-suppressive mechanisms impede Intratumoral lymphocyte infiltration into colorectal Cancer liver versus lung metastases. Cancer Res Commun. (2023) 3:2082–95. doi: 10.1158/2767-9764.CRC-23-0212
141. Ye, Y, Salvo, E, Romero-Reyes, M, Akerman, S, Shimizu, E, Kobayashi, Y, et al. Glia and orofacial pain: Progress and future directions. Int J Mol Sci. (2021) 22:5345. doi: 10.3390/ijms22105345
142. Baruch, EN, Nagarajan, P, Gleber-Netto, FO, Rao, X, Xie, T, Akhter, S, et al. Inflammation induced by tumor-associated nerves promotes resistance to anti-PD-1 therapy in cancer patients and is targetable by interleukin-6 blockade. Res Sq. (2023):rs.3.rs-3161761. (preprint) doi: 10.21203/rs.3.rs-3161761/v1
143. McIlvried, LA, Atherton, MA, Horan, NL, Goch, TN, and Scheff, NN. Sensory neurotransmitter calcitonin gene-related peptide modulates tumor growth and lymphocyte infiltration in Oral squamous cell carcinoma. Adv Biol (Weinh). (2022) 6:e2200019. doi: 10.1002/adbi.202200019
144. Tao, ZY, Wang, L, Zhu, WY, Zhang, G, and Su, YX. Lingual denervation improves the efficacy of anti-PD-1 immunotherapy in Oral squamous cell carcinomas by downregulating TGFbeta signaling. Cancer Res Commun. (2024) 4:418–30. doi: 10.1158/2767-9764.CRC-23-0192
145. Darragh, LB, Nguyen, A, Pham, TT, Idlett-Ali, S, Knitz, MW, Gadwa, J, et al. Sensory nerve release of CGRP increases tumor growth in HNSCC by suppressing TILs. Med. (2024) 5:254–270.e8. doi: 10.1016/j.medj.2024.02.002
146. Chen, S, Chen, Q, Zhang, X, Shen, Y, Shi, X, Dai, X, et al. Schwann cell-derived amphiregulin enhances nerve regeneration via supporting the proliferation and migration of Schwann cells and the elongation of axons. J Neurochem. (2023) 166:678–91. doi: 10.1111/jnc.15916
147. Tung, SL, Huang, WC, Hsu, FC, Yang, ZP, Jang, TH, Chang, JW, et al. miRNA-34c-5p inhibits amphiregulin-induced ovarian cancer stemness and drug resistance via downregulation of the AREG-EGFR-ERK pathway. Oncogenesis. (2017) 6:e326. doi: 10.1038/oncsis.2017.25
148. Zaiss, DMW, Gause, WC, Osborne, LC, and Artis, D. Emerging functions of amphiregulin in orchestrating immunity, inflammation, and tissue repair. Immunity. (2015) 42:216–26. doi: 10.1016/j.immuni.2015.01.020
149. Tang, D, Zhang, J, Yuan, Z, Gao, J, Wang, S, Ye, N, et al. Pancreatic satellite cells derived galectin-1 increase the progression and less survival of pancreatic ductal adenocarcinoma. PLoS One. (2014) 9:e90476. doi: 10.1371/journal.pone.0090476
150. Chawla, S, Warren, TA, Wockner, LF, Lambie, DLJ, Brown, IS, Martin, TPC, et al. Galectin-1 is associated with poor prognosis in patients with cutaneous head and neck cancer with perineural spread. Cancer Immunol Immunother. (2016) 65:213–22. doi: 10.1007/s00262-015-1788-z
151. Sundblad, V, Morosi, LG, Geffner, JR, and Rabinovich, GA. Galectin-1: a Jack-of-all-trades in the resolution of acute and chronic inflammation. J Immunol. (2017) 199:3721–30. doi: 10.4049/jimmunol.1701172
152. Huang, T, Fan, Q, Wang, Y, Cui, Y, Wang, Z, Yang, L, et al. Schwann cell-derived CCL2 promotes the Perineural invasion of cervical Cancer. Front Oncol. (2020) 10:19. doi: 10.3389/fonc.2020.00019
153. Ji, RR, Chamessian, A, and Zhang, YQ. Pain regulation by non-neuronal cells and inflammation. Science. (2016) 354:572–7. doi: 10.1126/science.aaf8924
154. Zhou, Y, Lu, Y, Fang, X, Zhang, J, Li, J, Li, S, et al. An astrocyte regenerative response from vimentin-containing cells in the spinal cord of amyotrophic lateral sclerosis's disease-like transgenic (G93A SOD1) mice. Neurodegener Dis. (2015) 15:1–12. doi: 10.1159/000369466
155. Byers, MR, Maeda, T, Brown, AM, and Westenbroek, RE. GFAP immunoreactivity and transcription in trigeminal and dental tissues of rats and transgenic GFP/GFAP mice. Microsc Res Tech. (2004) 65:295–307. doi: 10.1002/jemt.20130
156. Haggard, P, and de Boer, L. Oral somatosensory awareness. Neurosci Biobehav Rev. (2014) 47:469–84. doi: 10.1016/j.neubiorev.2014.09.015
Keywords: neuroimmune, chronic, HIV, Candida , Treg, perineural invasion, PNI, immune suppression
Citation: D’Silva NJ and Pandiyan P (2024) Neuroimmune cell interactions and chronic infections in oral cancers. Front. Med. 11:1432398. doi: 10.3389/fmed.2024.1432398
Edited by:
Ibrahim M. Sayed, Assiut University, EgyptReviewed by:
Gellan Ahmed, Assiut University, EgyptCopyright © 2024 D’Silva and Pandiyan. This is an open-access article distributed under the terms of the Creative Commons Attribution License (CC BY). The use, distribution or reproduction in other forums is permitted, provided the original author(s) and the copyright owner(s) are credited and that the original publication in this journal is cited, in accordance with accepted academic practice. No use, distribution or reproduction is permitted which does not comply with these terms.
*Correspondence: Pushpa Pandiyan, cHhwMjI2QGNhc2UuZWR1