- 1Institue of Research for Technology Development, Engineering Technology Department, University of Kentucky, Lexington, KY, United States
- 2Rocky Mountain Research Station, USDA Forest Service, Missoula, MT, United States
- 3Department of Energy and Materials, Kindai University, Higashi-osaka, Japan
- 4Department of Mechanical Engineering, Chubu University, Kasugai, Japan
- 5Department of Global Fire Science and Technology, Tokyo University of Science, Noda, Japan
- 6Department of Mechanical Engineering, Toyohashi University of Technology, Toyohashi, Japan
- 7Sekimoto SE Engineering, Sendai, Japan
- 8Department of Mechanical and Aerospace Engineering, University of California, San Diego, San Diego, CA, United States
The authors are a team of fire whirl researchers who have been actively studying whirls and large-scale wildland fires by directly observing them through fire-fighting efforts and applying theory, scale modeling, and numerical simulations in fire research. This multidisciplinary research-background team previously conducted scale model experiments to reconstruct hazardous large-scale fires in the laboratory, then conducted numerical simulations and developed fundamental theories to translate these findings into a basic understanding of combustion science and fluid dynamics. This article, a mix of reviews of the state of art experiments, theories, numerical modeling and artificial intelligence, and two case studies, is intended to address some safety concerns and raise awareness of large-scale fire whirls and forest fires with knowledge of thermodynamics, chemical kinetics, fluid dynamics, design, and practical fire-fighting experience, offering gaps that should be filled and future research to be conducted in each field, and crucial new observations and insights on large-scale fire incidents. We believe, this timely topic is of interest not only to fire research community but also to general readers, as the frequency and intensity of large-scale forest fires and fire whirls have increased, possibly due to the continuing global warming trend and human-induced changes in fuels. Each section and case study was written by one or two individual researchers based on their field of expertise which allows them to critically review progress made in their section of large-scale fire-whirls and forest-fires. Crucial observations and insights on the historical Great-Kanto-Earthquake-generated Hifukusho-Ato Fire-whirl (HAFW) and the slow rotations observed during recent forest firefighting efforts are presented. The first case study occurred in downtown Tokyo on 1 September 1923, as a result of the Great-Kanto-Earthquake, which claimed over 38,000 deaths within 15 min. The second case study discusses large-scale slow rotations observed during recent forest fires, which might had been responsible for the injuries and deaths of experienced firefighters.
Introduction
Although it is commonly stated that climate change is causing summers to become abnormally hot, what is much worse is that climate change promotes the occurrence of outdoor fires. Moreover, it is widely recognized that once a large-scale forest fire grows substantially, it becomes extremely difficult to control, with complete suppression typically requiring days or weeks. Improved fire prevention and mitigation strategies, therefore, are sorely needed. Outdoor fires occur in the world every day, many of them causing severe damage (NASA-FIRMS, 2022). When they arise near urban areas, they often inflict extensive property loss (e.g., Cohen 2000). Even those far from urban areas may raise serious issues; for example, large amounts of haze may be created and spread to neighboring cities or countries, creating health problems (Emmanuel 2000). Nearly 10% of greenhouse gas carbon dioxide emissions are attributable to wildland fires (see Global Fire Emissions Database GFED, 2022a; GFED, 2022b), exacerbating global warming. Carbon capture and storage by forest foliage promotes the oft-stated objective of reaching carbon-neutral conditions, but burning forests remove this beneficial function. Overall, promises of a hazard-free and healthy life without accelerated global warming would benefit significantly from improved abilities to control large-scale fires. This is a worldwide issue that should demand collaboration among all countries.
Fire whirls (herein also called “fire tornados”) often have been observed in large forest fires. They have been known to produce severe damage, sometimes catastrophic (Figure 1A). They also have been reported to appear in post-earthquake large-scale fires (e.g., Terada 1925). Tall, energetic fires associated with strongly spiraling upward-moving wind can promote the entrainment of a sufficient amount of air into the combustion zone, leading to appreciable fire growth. As the fire intensity increases, the entrained air inflow rate increases, accelerating the burning in the combustion zone, and the burning area expands accordingly. This positive feedback cycle helps to generate larger fire whirls. Worse, a fire whirl often does not remain at a fixed location but rather moves around in the vicinity of the fire. With such unexpected motion, evacuation becomes extremely difficult and may result in panic and isolation from outside help, leading to unforeseen tragedies. Fire whirls can also carry large embers that help accelerate the fire front. In addition to whirls containing the fire, other swirling flows (vortices) may appear nearby and convey hot burnt-gas products of the fire whirl throughout the thermally damaged zone. These vortices should be recognized as severe dangers because they may travel some distance away from the fire whirl yet not far enough away for people to escape. Obtaining a better understanding of the occurrence and movement of these “whirls without flaming” is a critical objective to consider in proposing strategies to mitigate their effects in such large fires, particularly strategies for determining how best to evacuate residents while maintaining sufficient safety margins. Relevant to the importance of such work is the observation that the 2002 Missionary Ridge Fire in Colorado caused tornado-like wind damage in an area that did not burn.
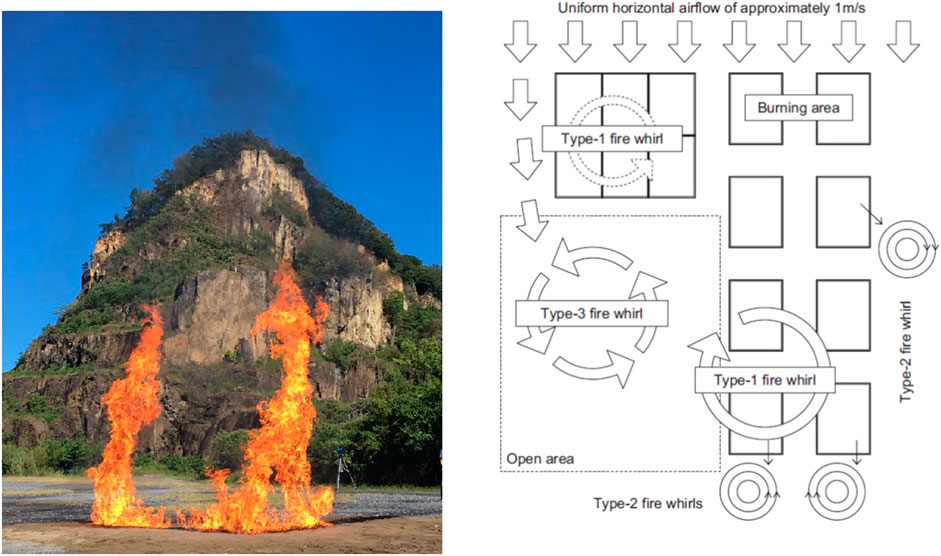
FIGURE 1. Figure 1A Fire whirls formed over an L-shaped line fire in an open-field experiment (Kuwana et al., 2022) Figure 1B (right) Type-I, II, III fire whirls (Kuwana et al., 2007).
As in all physical phenomena, activities in three different categories are capable of helping to increase understanding of large-scale and local intense rotational processes in fires. One of these categories is experiments, including field observations and scale modeling. It is essential for scientific progress. Theoretical analysis is a second, equally important category. The development of a comprehensible theory, which involves simplifications that lead to the identification of relevant dimensionless parameters that can facilitate scaling, necessarily requires neglecting certain phenomena that are known to be present. This leads to different theories that may appear contradictory but are complementary instead, for example, to different portions of fire whirls, thereby being applicable in different regions of space. The third category that is most recent and that now has become equally important is the broad area of computation, interpreted here to encompass not only finite-difference solutions of what are presumed to be the proper describing conservation equations by direct numerical simulation (DNS) or large-eddy simulation (LES) but also other computer-aided investigations, such as artificial intelligence (AI) and neural networks. Approaches in this last category are evolving rapidly, and the ultimate extent of their contributions cannot yet be evaluated well. However, they may revolutionize our thinking (hopefully for the better, not the worst).
To date, various research groups have pursued studies of fire whirls with unique approaches. Most of them have utilized scale-model concepts to avoid working with the actual full-scale fire (called the prototype) because it is too expensive, along with the difficulty of designing and running proper full-scale experiments. Scaling laws have been proposed through theoretical considerations, and various scale-model experiments have been attempted. Emmons, a pioneer in this subject, used a rotating mesh hanging from the ceiling to produce the swirl (Emmons and Ying 1967). Soma introduced a scaling approach to investigate fire-whirl occurrence conditions and study fire-whirl structures in laboratory-scale experiments and field tests (Soma and Saito 1991). More research has followed, including studies of various types of vortex-fire interactions, such as fire-driven vortex structures. There also have been investigations of geometrical effects on the generation and growth of fire whirls (Kuwana et al., 2007; Kuwana et al. 2008; Kuwana et al. 2015). With the development of high-performance computing technology, numerical modeling has recently progressed (Battaglia et al., 2000a; Zhou and Wu, 2007; Li et al., 2019); these approaches aid in grasping hard-to-visualize aspects of fires. Some success also has been achieved in categorizing types of fire whirls. A simple categorization was proposed by Kuwana et al. (2008), namely Type-I, Type-II, and Type-III, respectively (Figure 1B). While Type-I and Type-II fire whirls have received appreciable attention, Type-III fire whirls have not been studied as much (e.g., Kuwana et al., 2007; Kuwana et al., 2008; Ju et al., 2022).
This work is devoted to summarizing progress in fire-whirl research, along with describing reported fires. First, we shall introduce essential observations made during the fire-fighting of large-scale fires, after which critical studies of fire whirls utilizing scale-model and experimental approaches are presented to illuminate what is missing and future directions. State-of-art fire whirl theory and numerical modeling are then discussed, along with the relatively new technique using artificial intelligence (AI). The latter is considered one of the potential directions for future investigations into the prediction of fire spread by fire whirls. Therefore, its viability in providing a physical understanding of large fires is briefly examined. Lastly, we survey some actual fire-whirl incidents, where representative case studies are addressed, including tornado formation, which is lacking in most fire-whirl discussions. The mechanism of global warming in connection with large-scale fires is also discussed to clarify the social impact of mitigating large-scale outdoor fires.
Studies on large-scale fire whirls and forest fire
Observations made during fire-fighting and large-scale control-burns
Reports of large fire whirls on wildland fires seem to be increasing in recent years, no doubt in part due to more prolific observation technologies such as cell phone cameras, infrared cameras, and weather radar combined with easier dissemination of these observations using the Internet. But it also seems likely that the number of large fire whirls is increasing as burned areas have increased in many locations, specifically areas with heavier shrub and forest fuel types (Canadell et al., 2021; Zheng et al., 2021). These fuels release energy at a higher rate and are expected to be more conducive to large fire whirl formations since the greater buoyancy leads to stronger vortex stretching.
Indeed, some of the largest and most intense wildland fire-generated whirls have been reported recently, including some of tornado strength. A 2003 fire whirl in Australia produced at least EF2 wind damage, including picking up a 2-tonne police car and dropping it into a stormwater drain. It left a destruction path 20 km long. However, some segments along the path showed no wind damage. Fromm et al. (2006) and McRae et al. (2013) argue that this indicates that the whirl lifted off the ground at times, which was apparently a commonly thought behavior of regular tornadoes (but not a characteristic of fire whirls according to these authors). This apparent lifting observation and the fact that the damage path was long and wind damage occurred outside the burned area led the writers to categorize this event as a fire tornado–which they claim is a different type of event than a fire whirl. However, the United States National Oceanic and Atmospheric Administration (NOAA) states that breaks in tornado damage paths are now thought to usually be caused by factors other than the vortex lifting off the ground, such as a weakening of the tornado below the damage threshold, and then re-intensification (Edwards 2021). It is possible that this caused the breaks in the damage path of the 2003 Australian whirl, and it did not lift off the ground. Helmholtz’s theorems for vortex filaments in inviscid fluids potentially corroborate this idea (Hemholtz 1858), indicating that vortex lines and tubes can only form a closed loop, extend to infinity, or start/end at solid boundaries. Differentiating fire whirls from fire tornadoes in this way may be incorrect, and there does not seem to be a scientific consensus that this factor should be used for differentiation.
Cunningham and Reeder (2009) used numerical simulation to investigate the 2003 Australian whirl. They compared simulations with and without fire-generated moisture and found that the moisture released by the fire caused significant increases in the plume height. They reasoned that these moisture dynamics would also play an essential role in the tornado genesis. The idea seems reasonable, as the increased buoyancy would increase vortex stretching. Others have also found that the rotational speed of a whirl increases as its height increases (Snegirev et al., 2004; Grishin et al., 2005; Grishin, 2006). Unfortunately, past fire-whirl research using scaling approaches has not included this moisture effect.
Another fire whirl of tornadic strength happened in 2018 in California in the Carr Fire (Lareau et al., 2018; Forthofer 2019). This whirl caused wind damage indicating EF-3 strength, reached 5,200 m in height, and possibly was the strongest tornado ever recorded in California (Cappucci, 2021). One fire-fighter died of traumatic injuries when the strong winds overturned his vehicle, rolling it multiple times. During the 1979 Mt. Nuki fire in Japan, several experienced fire-fighters were trapped by a moving fire whirl because it suddenly changed direction from a wind-driven downhill direction to the uphill direction, which was also rectangular to the wind-driven direction. Emori and Saito (1982) later reconstructed this moving type fire whirl in the 1/2500 scale model wind tunnel experiment where the heated nichrome lines simulated the actual temporal fire lines. Lareau et al. (2018) studied weather radar data of the Carr Fire event. They concluded that the rapid vertical growth of the fire plume when the fire whirl formed was linked to the release of moist instability in the pyrocumulonimbus. This likely enhanced the rotation near the surface, causing tornado-strength winds. They discuss the nomenclature and suggest two terms: tornado-strength fire-generated vortex and pyrotornado. The former has vortex stretching dominated by sensible heat-induced updrafts, and the latter also includes a significant stretching contribution from pyrocumulonimbus latent heat release.
Just over 1 year later, another firefighter was killed by a fire whirl of tornadic strength in Australia (Guardian, 2020; Clifford, 2021; Dye, 2021). He also died when the vehicle he was in flipped over. It landed on its top, and the firefighter died of traumatic asphyxiation when he was pinned underneath the fire truck. And less than a year after that, on 15 August 2020, the United States National Weather Service sent out the first-ever fire tornado warning after meteorologists observed tornado signatures in radar data over the Loyalton Fire (Gabbert, 2020). The warning activated emergency alert systems and was broadcast on phones, television, and radio. This may have set a precedent for future warnings and is likely a phenomenon that weather forecasters will watch more closely now.
A multi-vortex fire whirl was observed using an infrared camera in 2019 (Gabbert, 2019). This is yet one more similarity between large fire whirls and regular tornadoes. In the early 1970 s, the famous tornado researcher Ted Fujita discovered that tornadoes could contain smaller vortices orbiting around the main vortex (Fujita 1981). These are now called “suction vortices” and produce some of the worst tornado damage. It is unclear if the video of this fire whirl shows the equivalent of suction vortices or simply two independent fire whirls. Still, the similarity of the two phenomena points to the possibility that suction vortices could occur in a fire whirl.
Another interesting fire whirl phenomenon recently filmed was the apparent vortex breakdown at the top of a fire whirl (Tangey, 2012). In the video (at a time of 1:07–1:20), the tip of the fire whirl is seen to split, indicating some dramatic change in the behavior of the upward-rotating fluid. This appears to be the well-studied vortex phenomenon called vortex breakdown (Lucca-Negro and O’Doherty, 2001), which is theorized to be a flow phenomenon in swirling flows related to possibly a wave-like behavior, flow separation/stagnation behavior, or a kind of hydrodynamic instability. Vortex breakdown has been studied in combustion systems (such as gas turbine engines, diesel engines, and industrial burners) and in leading-edge vortices shed from delta wings. Vortex breakdown has not been examined in the wildland fire context, although it has been considered in connection with the so-called “blue whirl,” to be discussed later.
Field and laboratory experiments and scale modeling
Since the actual fire whirls (the prototype) are too large to control in the laboratory, researchers have designed reduced-size models (“scale-models”) to conduct experiments. The scale models employed in general are of two kinds (Soma and Saito 1991): the “basic model” designed to understand fundamental mechanisms and the “similarity model” to simulate prototype phenomena to develop scaling laws.
Understanding the similarity model is important in establishing an ultimate configuration for the fire whirl experiment, which can reconstruct the prototype fire whirls on the scale of a laboratory or a test field. Figure 2 illustrates and categorizes the types of configurations for fire-whirls experiments. One of the approaches to designing the configuration of the scale-model experiment is to build it based on the observation of the prototype fire whirls. Figure 2A, the scale-model version of Figure 1B, shows the configuration of scale-model experiments of wind-generated fire whirls that occurred in the Hifukusho-ato area shortly after the Great Kanto Earthquake in Tokyo in 1923. The gray area shows an L-shaped pool fire area, and light gray spots with swirling arrows show the places of fire-whirl generation (Kuwana et al., 2007; Kuwana et al. 2008; Kuwana et al. 2015). The pool-fire area plays the part of a hot boundary to restrict the lateral stream, resulting in a swirl of flow, which generates Type-2 and Type-3 fire whirls. Figure 2A’ shows that, for Type-3, solid walls can replace the pool fires. The asymmetric configuration of the pool fires in Figure 2A also can initiate a Type-1 fire whirl, as indicated. A scaling law obtained by Kuwana et al. (2007), Kuwana et al. (2008) involves the critical lateral flow velocity
where
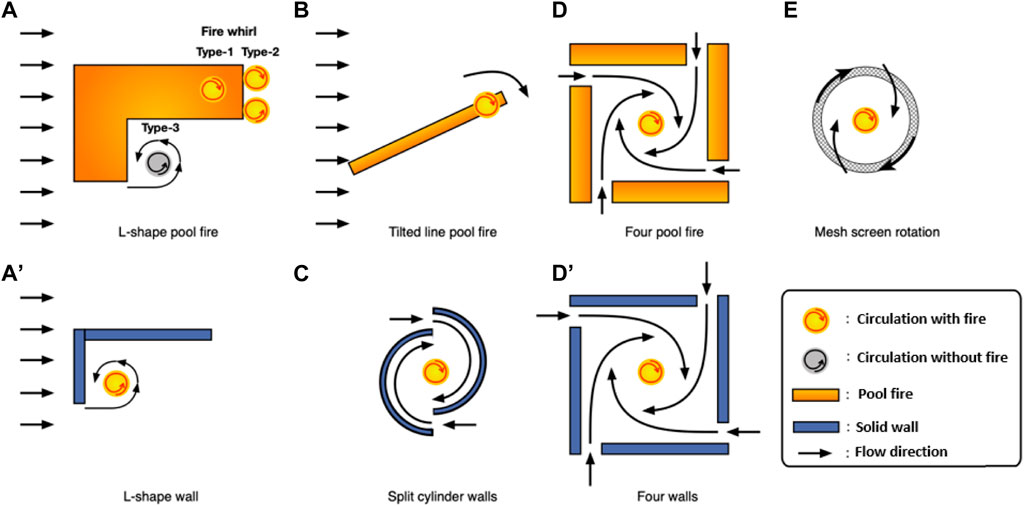
FIGURE 2. Seven different ways, as shown in (A), (A’), (B), (C), (D), (D’), and (E), to generate fire whirls. Type-3 fire whirl shown in (A) with a gray disc does not carry fire, while all other fire whirls with red circulation over a yellow disc carry fire.
Figure 2B is another example of an asymmetric configuration of a pool fire that can initiate a fire whirl; this is a scale model of a prototype fire whirl that occurred in Brazil in August 2010 (Zhou et al., 2016; Kuwana et al., 2020). The generation of a fire whirl by a tilted-line pool fire may be the simplest generation mode. Figure 2D shows a somewhat complicated configuration of fire-whirl generation employing four pool fires, where a swirling flow is generated by entrainment air into the buoyant upward flow (Zhou and Wu, 2007). The four-pool-fire configuration can be interpreted as an overlay of four sets of L-shape pool fires working as flow restrictions to generate a swirl flow.
While those configurations of pool fires are approaches to generating a fire whirl in the scale-model experiment, they also indicate where the fire whirl can occur in mass or forest fires. Although the pool fire works as a hot boundary to restrict a flow stream, solid walls also work as a boundary that can swirl the flow stream. Similar to the type-3 fire whirl shown in Figure 1A, the L-shape wall can generate a fire whirl, as shown in Figure 2A’ (Sasaki et al., 2018); this implies the risk that a prototype fire whirl can be generated behind an L-shape building complex. They found that fast inflow near the floor was responsible for an intense fire whirl formation, thus suggesting an effective way to mitigate fire whirl by blocking the near-floor flow using an obstacle.
Figure 2C illustrates one of the simple configurations that can generate fire whirls because this setup requires only a cylinder split in half, with each half displaced around a pool fire to form slits through which air is entrained with rotating components of velocity (Hassan et al., 2005; Lei et al., 2012; Xiao et al., 2016). This type of configuration does not need lateral wind because a spinning flow is generated by entrainment due to buoyant upward flow. The configuration in Figure 2D’, related to Figure 2D in the same way that Figure 2A’ is related to Figure 2A, also does not need lateral wind; it requires only four walls with axis-symmetrically-placed four slits (Satoh and Yang, 1996; Lei et al., 2012).
As previously indicated, Emmons and Ying (1967) investigated fire whirls generated by a cylindrical mesh screen rotating around a pool fire, as shown in Figure 2E. Their study is known as one of the pioneering studies of relatively stable fire whirls. The unique merit of this configuration is the capability of controlling the circulation
Through all past efforts, the fundamental structure and character of fire whirls have been understood very well. Nevertheless, some behavior observed in large-scale wildland fires cannot be identified in the lab-scale experiment (ex., Multi-vortex fire whirl). It is noted that all studies made so far were to generate swirling flow externally or naturally assisted (or triggered) by buoyancy, and no attempt was made to include the high-altitude atmospheric effects. This is still a missing piece of work for the lab-scale fire whirl study.
Development of fundamental theory: Fire-whirl theories
Tohidi et al. (2018) classified fire whirls into open and enclosed configurations and provided fluid dynamical insights on the whirls and their formation conditions. They extended three different types of fire whirls originally proposed by Soma and Saito (1991) with some additional statements about the Hifukusho-Ato Fire-whirl (HAFW), one of the case studies which we elaborate historical and physical characteristics of in detail later in this article.
Most fire-whirl theories concern axisymmetric configurations, attempting to predict 1) swirling-flow structures induced by buoyancy in a background circulation and 2) the dependence of flame length on flow parameters. The following summarizes representative fire whirl theories. Emmons and Ying (1967) analyzed the plume structure above the fuel pool. Their integral model predicted the axial velocity and the mean buoyancy within the plume and the plume radius. Their model parameters, such as the mixing coefficient, must be determined by fitting experimental data. Based on the measured plume radius, they deduced that the mixing coefficient rapidly increases with axial height. They then state that a vortex breakdown may have caused high turbulence and could account for the high mixing coefficient. However, the visible flame showed no evidence of any changes in height other than the gradual burn-out of fuel.
Byram and Martin (1970) discussed that buoyancy is equivalent to a horizontal plane surface at the upper end of the vortex, z = ze (Figure 3). They then assumed a simple combined vortex structure and derived equations for the pressure and velocity distributions. The core radius predicted by their model has a similar axial distribution to the plume radius measured by Emmons and Ying (1967). Note that in the Byram-Martin model, the horizontal-plane height acts as a model parameter to be determined by comparison with experimental data. Some estimates were made for velocities of full-scale fire whirls.
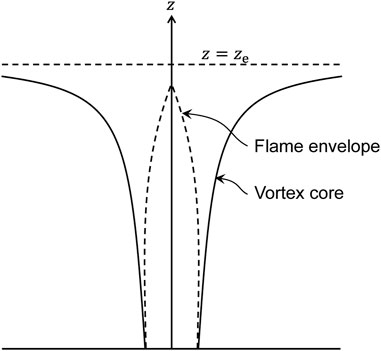
FIGURE 3. Flame and vortex structures proposed by Byram and Martin (1970).
Mullen and Maxworthy (1977) discussed the existence of vortex breakdown and the resulting vortex structure. Vortex breakdown creates a free stagnation point above which a two-cell vortex is formed, resulting in a hemispherical region of almost stationary fluid headed by the free stagnation point. Tip opening is often observed in wildland fire whirls, such as the one shown in Figure 2B of Williams (2022). The flame tip has a hemispherical shape heading downward, indicating the occurrence of vortex breakdown there. The point
Battaglia et al. (2000b) considered the inviscid-flow interaction between externally-imposed circulation and buoyancy. An equation for the stream function was derived and numerically solved. The model is simple but able to reproduce many experimental features. For example, the model predicted that the plume was radially constricted while stretching vertically. The model does not consider combustion reactions, so it cannot predict the flame shape or length.
Chuah and coworkers (Chuah and Kushida, 2007; Chuah et al., 2009; Kuwana et al., 2011) solved the equation for the mixture fraction under given flow fields such as the Burgers vortex. This way, they could predict the flame shape. They showed that the flame length mainly depends on the burning rate for laminar fire whirls. The significant increase in flame length observed in experiments is primarily because of the increase in the burning rate caused by changes in the flame-base structure. Klimenko and Williams, (2013) adopted a similar approach to analyze the influence of strong rotation on flame length. See Kuwana et al. (2022) for a review article on the mixture-fraction model with given flow fields.
Lei and coworkers (Lei et al., 2012; Lei et al., 2015; Lei et al., 2017) extended the integral model of Emmons and Ying (1967) to derive functional dependences of the quantities of interest, such as the flame height. They showed that their experimental data could be fitted with the derived equations, showing that integral models are powerful tools for analyzing and interpreting experimental data.
Coenen et al. (2019) considered swirling flows induced by plumes and sought a self-similar solution of the circulation
Williams (2022) recently proposed an integral zone model to predict the dependence of flame height on flow parameters. The model predicts that
Numerical modeling and simulation
Numerical simulation (CFD) is widely used in the study of fire whirls. CFD can reproduce phenomena in an ideal environment with no disturbances and handle prototype scale phenomena. Hence, it is suitable to perform parameter studies on the behavior of fire whirls under various conditions. Zhou and Wu (2007) reproduced the fire whirls spontaneously generated on the fuel pools of various arrangements using CFD. They examined the changes in rotational speed and flame height with respect to the different arrangements and considered the mechanism and condition for the fire whirl.
Since fire whirl is a three-dimensional transient flow phenomenon, detailed measurement is difficult, even in laboratory experiments. In order to obtain detailed information on the internal structure of a fire whirl, experiments and CFD are often used in combination.
Typical experimental equipment used in combination with CFD are a configuration in which the fuel source is placed in the center of a cylindrical rotating screen (Emmons and Ying 1967) (see Figure 2E), a square enclosure with axisymmetric vertical corner gaps (Satoh and Yang 1997) (see Figure 2D’ in Chapter 2), and a configuration in which a cylinder split in half, with each half displaced around a pool fire was adopted (Hassan et al., 2005) (see Figure 2C in Chapter 2).
Satoh and Yang (1997) conducted experiments and CFD analyses on a whirling flame established in a square enclosure with symmetrical vertical corner gaps and mainly investigated the effect of the gap size on the structure of the whirling flame. No combustion model was adopted in this calculation, and the modeling was performed by setting the heat generation rate distribution in the calculation space. Nevertheless, the temporal and spatial changes in the flow structure and mass flow rate during the growth process of the whirling flame were reasonably reproduced qualitatively.
Battaglia et al. (2000a) performed CFD analyses on Emmons and Ying’s experimental equipment to investigate the flame structure change due to rotational flow intensity. The combustion reaction model was employed in this calculation, but the fuel supply rate was fixed. Therefore, the effect of the increase in fuel evaporation rate due to the heat flux to the fuel, which is a characteristic of an actual fire, was not considered, causing disagreement in the numerically predicted flame length with the experimental measurement.
Kuwana et al. (2011) conducted laboratory experiments and CFD analyses on the equipment, similar to Hassan et al. (2005). This CFD analysis adopted a model that varies the fuel supply rate according to the amount of heat flux to the fuel pan. As a result, the shape of the flame base changed due to the swirling flow, increasing the heat flux to the fuel and the flame length. Furthermore, based on the detailed data of the flame structure obtained by the CFD analyses, a theoretical model of flame that can quantitatively predict the flame length and combustion velocity was established.
CFD analysis is also used to verify the flame model obtained by theoretical analysis. Li et al. (2019) conducted CFD analyses on flames with circulation flow under various Ekman and Grashof number conditions. Based on the result, they developed the flame base structure model, composed of three regions, and established a theoretical model of the flame considering thermal feedback to the fuel pool. CFD analyses were used to verify the combustion velocity and flame height predicted by this theoretical model.
Sasaki et al. (2018) examined the fire whirl formed behind an L-shaped wall about the variation in flame height and flow structure by experimental observation and CFD analyses (see Figure 2A’ in Chapter 2). Scale modeling was performed, and the scaling law was verified by CFD by keeping both the Froude and the Reynolds number constant but by changing the gravity constant.
In recent years, a form of whirling flame called ‘blue whirl’ has been observed in laboratory experiments on fire whirl (Xiao et al.2016), whose theoretical background was explained in the section on fire whirl theories. Chung et al. (2020) visualized in detail the structure of a blue whirl by CFD analysis, clarifying that the blue whirl is formed by vortex breakdown, and its flame structure is composed of rich and lean premixed flames, diffusion flame, and triple-flame.
Most of the past numerical work was directed toward understanding what happens in the corresponding (lab-scale) experiments and rarely attempted to perform “numerical experiments” to find out what might happen under extreme conditions (far from adopted experimental conditions, hence, no data available to validate). Because the complete dataset for large-scale fire whirls is rarely expected, a numerical approach is valuable to investigate (predict) the mechanism of large-scale fire whirls.
Hypotheses-free artificial intelligence to multi-variable problems: Inductive approach to study forest fire spreading
Studying the mechanism of wildfire spreading through live fuels is a challenging task because it includes many different parameters. Some parameters are very difficult to control, and some mechanisms are unknown to us. Because of this high degree of complexity, using an induction approach and the conventional scientific deductive approach may be helpful (Saito and Williams, 2014).
Figure 4 shows a general diagram that explains specific paths for both hypotheses-free inductive and traditional hypotheses-driven deductive scientific methods (Saito, 2022). Note that the third method, a largely unexplored transcendent method to raise human awareness on nature and the world around us which differs from the traditional scientific human understanding, exists (Suzuki 1973; Mefawar, 1984; Saito 1995; Nisbett 2003) to compensate for the limitations of science. The first approach represented by artificial intelligence may become a practically helpful tool when the above-explained complexity is evident. After successful correlations are established among observations and experimental laboratory data by appropriate statistical analysis, an artificial neural network or simple machine learning may be applied to predict fire-spreading behavior. An Artificial Neural Network (ANN) is considered a powerful tool for estimating the impact of several descriptive aspects on a predicted variable. With the use of ANN, interaction among variables can be simulated for linear and non-linear relations, helping us to advance our understanding of the role of each variable. The ANN would also apprehend the nature of the interactions among the predictors and the predicated variables, leading to a significant improvement in the model prediction.
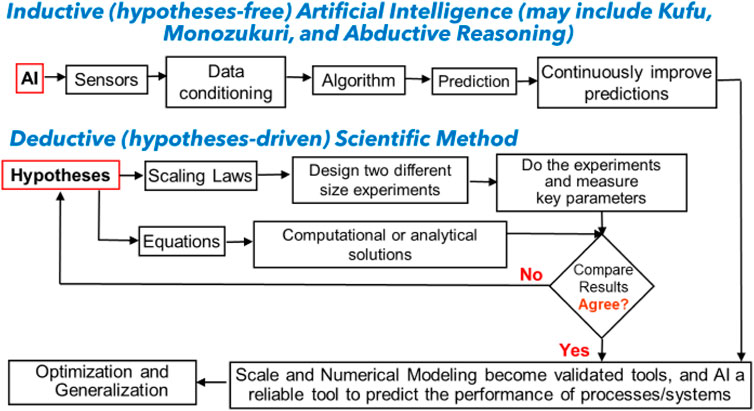
FIGURE 4. The deductive hypotheses-driven scale and numerical modeling, and inductive hypotheses-free artificial intelligence (Saito, 2022).
At a conceptual level, ANN is a massively parallel system of equations. These equations, called neurons, use information from other neurons to make a new observation in the network. Machine learning and ANN have recently been used to predict numerous physical problems. For instance, many researchers have used machine learning to predict the total burned area by fires. Safi and Bouroumi (2013) utilized the data from the Montesinho natural park in Portugal (since this region is one of the most concerned with forest fires) in an ANN model for prediction. Castelli et al. (2015) also used an artificial intelligence approach to predict the burned area using 517 forest fire events database between 2000 and 2003. With the increase in computing power, the use of AI in fire research is becoming more prominent, specifically relevant when scaling and numerical modeling face limitations in handling complex multi-parameter problems, as mentioned earlier. Numerous other studies used artificial intelligence to study, detect, and prevent the risk of uncontrolled destructive fires. Analyzing the spreading rate of wildfires and accurately predicting it is an effective way to avoid unplanned fires. For example, Wu et al. (2022) used an ANN model for wildfire propagation prediction based on weather climate change, topography, combustible factors, and ground cover types. They concluded that the ANN model could accurately predict the behavior of wildfires.
Artificial Intelligence was also used in developing evacuation simulations for wildfires (Zhao et al., 2021). Different AI models are available for fire prediction; therefore, some studies tested several models, such as R-CCNN and SSD, to examine the best performance for fire prediction (Alexandrov et al., 2019). The latter study tested the following models, YOLO, R-CNN, and SSD, for fire prediction, and they concluded that the YOLO and R-CNN models could accurately predict fires. YOLO outperformed the other models being the fastest and most accurate, while the SSD model performed the poorest. A comprehensive review by Jain et al. (2020) on different AI techniques helps us to predict the behavior of wildfires. In another aspect, AI and machine learning can reconstruct quantities unmeasured during experiments using the measured ones. Sitte and Doan (2022) used hidden fluid mechanics, a physics-informed machine learning technique, to reconstruct unmeasured quantities from pool fire experiments. In short, AI can extensively support fire prediction and evacuation planning alongside numerical and scale modeling for estimating unmeasured quantities that are not accessible through other means.
However, there is a clear difference between the hypotheses-driven scientific method and the hypotheses-free AI. The following Einstein quote clarifies the role of science in human understanding, i.e., “the grand aim of all science is to cover the greatest number of empirical facts by logical deduction from the smallest number of hypotheses or axioms.” Contrary, artificial intelligence does not offer hypotheses, which we humans use as the basic element of human understanding. The hypotheses-free algorisms can be built and trained based on statistical data to predict future events. Still, AI’s contribution to traditional human knowledge based on hypotheses-based scientific methods may be questionable. Still, it may provide us with a new opportunity to expand the regime of human learning with this new inductive tool. For example, combining AI and scientific methods (scale and numerical modeling) is suggested to enhance human understanding. AI may develop a successful algorithm to predict fire spreading rate and direction. Then, another algorithm may be able to track down to find hypotheses to support empirical correlations that the AI algorithm uses. These ideas need to be further tested for practicality and human understanding and reasoning perspectives.
Case studies on large-scale fire whirls and forest fire
The type-3 (hifukusho-ato) fire whirls and the froude number scaling law
Scale modeling large-scale disasters: fire whirls, tornados, hurricanes, and the crash of a jet-liner into Mt. Fuji.
The great kanto earthquake and fire-whirl tragedy
Background
Japan suffered the Great Kanto Earthquake (GKE), magnitude 7.9, which directly hit downtown Tokyo on 1 September 1923. Mass fires broke out in the aftermath of this GKE due to its timing, 11: 57 in the morning, a time at which many residents were cooking and preparing lunch with popular wood-burning stoves, and many Japanese houses in that era were built of wood and paper products. The three elements of the fire triangle: fuel, oxygen, and temperature, were easily met under the fine September weather conditions that existed at that time, with a south wind of 4–5 m/s spreading fire in the residential areas of downtown Tokyo. To escape the fire threat, a large crowd of approximately 40,000 people had gathered in the open, flat Hifukusho-Ato area of about 70,000 m2, where no structures or trees were believed to exist. The Hifukusho-Ato-Fire-Whirl (HAFW) tragedy struck an unexpected historical disaster. The historical committee report (Terada, 1925) described that several fire whirls swept through that area, carrying flames and burning debris, resulting in an estimated 38,000 deaths within 15 min. Soma (1975) and Kuwana et al. (2007) and Kuwana et al. (2008) each designed different size reduced-scale model experiments in the laboratory and conducted wind tunnel fire whirl experiments. In addition, Soma and Saito (1991) designed a 1/100 scale open field fire whirl experiment which will be detailed in the section of HAFW. All they observed was the occurrence of large circulations which did not carry fire.
The committee report (Terada, 1925), which detailed this tragic accident, offered as a likely cause the occurrence of tornados triggered by a weather front passing by with substantial cumulus clouds observed on that date. According to Terada (1925), gigantic cumulonimbus appeared in the sky over Tokyo right after GKE, coinciding with the time of the HAFW occurrence. Later Soma (1975) elaborated on the nature of these gigantic cumulonimbus clouds, which mass fires can create. Jones and Miller (2016) reported that California wildfires became so hot that they produced mushroom-like clouds known as pyro-cumulus clouds. However, these two different clouds, the one shown in the report (Terada, 1925) and the other by CNN news, showed a strong resemblance in shape. This indicates that the initial thought weather-front-created unusual shape of HAFW cumulus clouds (Terada 1925) may well be pyro-cumulus clouds created by the GKE-created mass fires, different from the weather-front-created ones.
The HAFW section explains that our past scale model type-3 fire whirl experiments of different scales, 1/100 (Soma and Saito 1991), 1/1000 (Kuwana et al., 2007; Kuwana et al., 2008), and smaller scales, all showed type-3 fire whirls to be a large slow circulation relative to the fire area without carrying fire (see Figure 1B; Figure 2; Figure 6; and Figure 7). Therefore, asking whether the type-3 fire whirl alone could incinerate 38,000 people within 15 min is reasonable. This recently raised question may suggest two potential factors, downburst from pyro-cumulus clouds, which possibly fed fire into type-3 fire whirl, and the unknown high energy content fuels at the tragedy site ignited when type-3 fire whirl occurred, providing tremendous energy into type-3 fire whirl. The nature of the downburst will be further elaborated concerning wildland fires and fire-generated weather. In contrast, the second factor, which can transform type-3 fire whirl into type-1 fire whirl, will require a thorough review of the historical documents for future study.
The pyro-cumulus clouds tower above the ash, and smoke from raging wildfires is often seen for miles. They are also known to cause a downburst, a strong downward wind driven by the gravity force acting on water droplets formed at the upper layer of the clouds. It is unclear, at this point, whether the formation of a downburst nearby Hifukusho-Ato occurred. If yes, what part did it play? Figure 1 from Kuwana et al. (2007) depicts a large, strong circulation arising from the Sumida River primarily bent over to the north, thereby essentially differs from vertically oriented slow circulations without fire, observed from different scale model experiments (Soma and Saito 1991; Kuwana et al., 2007; Kuwana et al., 2008). This discrepancy may indicate a possibility that weather involvement plays some role in creating this Figure 1 fire whirl. Quantifying the frequency and size of those cloud formations, sometimes called flammagenitus clouds, pyrocumulus clouds, or fire clouds (which are also discussed in the following case study section 2), could be a new effective method to estimate fire severity trends and how fires affect climate change, as mentioned in the later global warming section.
Although the accidental occurrence of tornados turned out not to be the cause, as explained by Soma in what follows, the idea of similarity between tornados and fire whirls remains a topic worth studying, this similarity having been little explored yet. This relativistic study to identify common mechanisms between fire whirls and tornados and notable differences may help enhance our understanding of nature’s mechanisms (Emori et al., 2000). Another large-scale tropical circulation phenomenon, hurricanes, whose circulation diameter is much larger than tornados but share some common governing mechanisms with tornados (Fujita, 1971), will be added based on this relativistic study merit in the following section since they are known to cause severe damage to the environment and structures.
Fire whirls, tornados, and hurricanes
One of the common mechanisms for all three of these phenomena is the interaction between buoyancy-driven upward currents and the air circulation that promotes rotation. For fire whirls, the upward buoyancy-driven flow is created by the fire, which interacts with a relatively mild wind. At the same time, for tornados, it is provided by the heated ground surface interacting with the Coriolis force, which provides a rotating motion that can create a supercell structure or lead to smaller suction vortices rising upwardly, both driven by a pressure difference (Fujita 1981). They are called mesocyclones, whose horizontal characteristic length extends between 40 cm and 40 m. At the same time, tornados are mesocyclones with their horizontal characteristic length extending between 40 m and 4000 m, where the Coriolis force becomes influential.
For type-3 fire whirls, the Coriolis force is typically irrelevant because the diameter of the fire whirl is relatively small (less than 50 m) compared with the horizontal characteristic length of tornados (Morton, 1970). However, certain type-1 fire whirls in the categorization of Soma (Soma and Saito 1991), such as the Hamburg fire whirl (estimated diameter 2.4–3.0 km and height 5.0 km) as well as a large forest fire whirl created during a prescribed fire to reduce debris left by a timber harvest (Albini 1982) whose diameter appeared to be much larger than 50 m, may be large enough for Coriolis effects to come into play.
This 50-m-diameter criterion is used as a characteristic critical diameter to roughly estimate the influence of the Coriolis force, below which there is little to no effect of the Coriolis force and above which the Coriolis force may become influential. This means that tornados and large-scale type-1 fire whirls may be affected by the Earth’s rotation, while most type-3 fire whirls are not. Noted that fire whirls greater than 50 m in diameter have been observed to rotate in an anticyclonic direction, suggesting that other factors may be stronger than the Coriolis force in some cases (see, for example, the fire whirl reported by Moore et al. (2008)).
The same criterion can be applied to hurricanes and typhoons (Fujita 1971; Fujita et al., 1972), whose circulation diameters normally exceed those of tornados; therefore, they are even more strongly affected by the Coriolis force than tornados, with the result that hurricanes in the northern hemisphere rotate counterclockwise, while those in the southern hemisphere rotate clockwise. For Coriolis-force-driven tornados and type-1 fire whirls, the Rossby number, defined as the ratio of the centrifugal force associated with the intensity of circulation to the Coriolis force, has been used to establish similarity between different-scale phenomena (Emori et al., 2000).
Downbursts, or microbursts, are other unique phenomena associated with supercell-driven tornados (Fujita 1981). They generate very fast downward currents driven by gravity acting on condensed water droplets created by the cooling that occurs as the buoyancy-driven hot convective currents move upward and reach a certain height at which the atmospheric temperature is sufficiently cooler than that near the surface. Fire whirls are not known to create supercells or downbursts. Pushed by the weather front, the mother tornado and smaller suction vortices created around it move together, whence the pressure force provides the drive to move them. Interestingly, some fire whirls also move. Williams and coworkers (Kuwana et al., 2020) suggested a flow-separation and stagnation analogy to explain the mechanism by which fire whirls move along line fires. These comments summarize the characteristics of fire whirls, tornados, and hurricanes that must be considered when considering their similarity and scaling.
Soma (1975) reviewed the historical record of the GKE and proposed a new mechanism, the interaction of mass fires with a relatively low-speed wind. His wind-tunnel fire-experiments qualitatively reconstructed HAFW, the type-3 fire whirl in his fire-whirl categorization, validating his proposed mechanism. Before the HAFW section, let’s first review his investigation of a jet-liner crash accident into Mt. Fuji with wind tunnel scale model experiments which helped the HAFW experiments.
Scale modeling a jet-liner crash into Mt. Fuji to seek similarity in turbulence
Soma (1988) previously carried out scale model experiments to investigate the 1967 British jet-liner crash into Mt. Fuji, which killed all 167 passengers. The cause was mainly due to his continuing effort to obtain a total of 25 trials, spanning more than 4 years, to measure the flow patterns behind Mt. Fuji created by strong winds, approximately the wind recorded at the time of the airliner crash, i.e., over 40 m/s. A gravity-neutral balloon that emitted a periodical radio signal was released several times from the 3,333 m summit under these strong wind conditions. The signal was measured at the base of Mt. Fuji to obtain the trajectory of the balloon, which followed turbulent motion at lee-wind locations. With this prototype data, Soma and his investigation team designed a 1/25,000 scale model of Mt. Fuji and conducted open-loop low-speed wind-tunnel experiments and obtained remarkably similar wind patterns based on the Fr and the St number scaling laws, whose results are available (Saito and Willaims 2014; Soma et al., 2022). Figure 5 shows the Strouhal-number/Froude-number correlation, which supports the scientific background of Soma’s scale-modeling investigation, where the steady and fluctuating velocity components and the variation of the air density with atmospheric altitude were considered for scaling. The force balance between these velocities forms the Strouhal number. The Froud number is formed by the ratio between the inertial force of the steady airflow and the gravity force acting on the air. This St/Fr correlation was also applied to successfully scale model fire spreading through forest fuel beds (Finney et al., 2015), as shown in Figure 5.
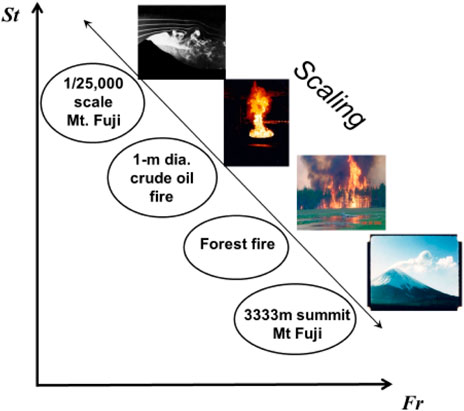
FIGURE 5. The Strouhal number - the Froude number scaling correlation to connect phenomena separated by 105 orders of magnitude in the characteristic length.
Interestingly, Warhaft (2022) introduced a new approach to studying a centuries-old tough fluid mechanics problem, turbulence, from artists’ perspective to overcome the scientific impasse that resisted progress in the current study of turbulence. Soma’s 1/25,000 scale-model flow-visualization experiments were indeed intended to use the relativistic scale modeling perspective to reveal the complex full-scale turbulent-flow pattern behind the Mt. Fuji.
Hifukusho-ato fire-whirl
The HAFW tragedy investigation required a careful review of the available historical weather record at the accident site to establish reliable data on the formation of cumulus (or pyro-cumulus) clouds, wind speed, and wind direction at the accident site (Soma, 1975). The latter is particularly important to determine the formation of fire whirls; the south wind of 4–5 m/s was established. Soma (1975) estimated the average heat generation rate from the fire area, assuming typical fires in Japanese houses and urban cities, designed a conceptual 1/2500 HAFW scale model, and conducted wind tunnel fire experiments using the same low-speed wind-tunnel used earlier for the jet-liner crash investigation. The wind speed for the 1/2500 scale model was adjusted by trial and error since the scaling laws were unknown then. Later, a 1/100 scale open field experiments were designed at a landfill off Tokyo Bay, in which the Fr number scaling on the wind speed and the burning area heat release rate were successfully applied (Soma and Saito 1991).
The Fr number scaling restricts the horizontal wind speed to be proportional to (the characteristic length)1/2 and the total heat generation rate being proportion to (the characteristic length)5/2. In addition, the geometrical similarity of (the flame height)/(the fire length) was satisfied by an equidistant distribution of sixty-nine 80 cm-diameter shallow methanol pan fires. Each pan separated to create an open space through which fresh air was entrained and circulated to bring the flame height down. This was the direct application of the scaling technique to realize the surface to volume scaling (Emori et al., 2000). Note that if a continuous burning area had been used as a result of the simple geometrical scaling of the full-scale fire, then the (the flame height)/(the fire length) similarity would not be satisfied and would result in a much higher flame height for the model. Figure 6 shows a schematic diagram of this 1/100-scale-model outdoor fire-whirl experiment.
The outdoor HAFW experiment on 23 October 1979, which relied on the natural wind condition, fortunately, satisfied the Fr-number-scaled wind velocity and generated the type-3 fire whirl, as detailed by Soma and Saito (1991). To further investigate the applicability of the Froude-number scaling to the type-3 fire whirl, Kuwana et al. (2007) designed an L-shaped 1/1,000 scale model using distributed small heptane pool fires under a low-speed horizontal wind available in the Japanese Building-Research-Institute’s open-loop wind tunnel. It was aimed at further verification of the Froude-number scaling law and the occurrence of type-3 fire whirl (Figure 1B), similar in shape to the one that appeared in the 1/100 scale model with speed scaled down by the Froude number (Figure 7).
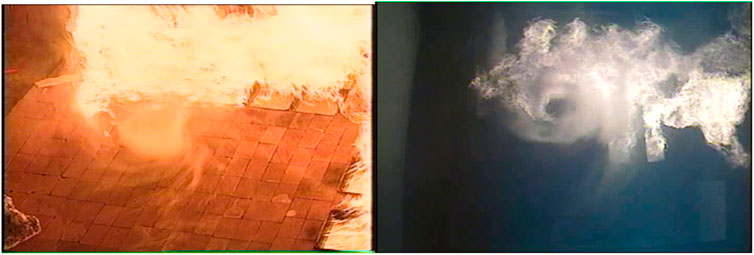
FIGURE 7. A photograph of a type 3 fire whirl is visualized by rising water vapor from the floor. Left: side view and right: top view. For a schematic of the 1/1,000 scale model HAFW experiment, see Figure 1.
Based on the success in the validation of the Froude-number scaling at 1/100 and 1/1,000 scales in model experiments, Kuwana et al. (2007) and Kuwana et al. (2008) advanced the idea that the burning area may be regarded as an obstruction to estimate its near-wake flow structure; thus, the asymmetry of the L-shaped mass fire favors the formation of type-3 fire whirls. With this idea, the similarity between the asymmetric cold-jet experiments in a crossflow by Wu et al. (1988) and our type-3 fire-whirl experiments Kuwana et al. (2007) and Kuwana et al. (2008) became available. Specific empirical correlations (the critical lateral wind velocity, Uc) ≈ (the upward buoyant velocity at the flame tips)/3 and (the fire plume height)/(the horizontal length scale of the obstruction, L) ∼ Fr1/4 were used to obtain the final form of the equation, Uc ∼ L3/8m1/4, where m = (the mass of fuel consumed per unit ground area per unit time).
The role of large-scale slow rotations in forest fire spreading and firefighting
Several wildland firefighter accidents and near misses over the last few years have made it clear that even relatively slow but large-scale rotations can cause dangerous and unexpected fire behavior. And it has become apparent that there is no well-defined lower limit to the rotational speed required for an event to be termed a fire whirl. Instead, it is more of a continuum from no rotation to the fastest spinning whirls. These slower rotations on wildland fires, slower than would typically be called a fire whirl, have not received much investigation. They seem especially significant for firefighters if they are of large scale (hundreds to thousands of meters in diameter) and correspondingly long-time scales (several minutes to hours). These large and long-duration rotations can significantly affect fire behavior, sometimes unexpectedly. Impacts include a local increase in windspeed and change in direction compared to the ambient winds and the potential for increased lofting of embers resulting in spot fires. These large rotations’ origins and influencing factors are similar to fire whirls. Some of these events could even be considered weak fire whirls.
The Route Fire in 2021 in California was such an event (Los Angeles County Fire Department and US Forest Service, 2021). The fire ignited and spread rapidly in complex mountainous terrain in brush fuels under a moderate ambient wind. A large-scale rotation (hundreds of meters in diameter) formed on the downwind side of the fire (Figure 8). The near-surface winds from this rotation caused the fire to spread forward in a curving, hooking motion around a group of unsuspecting firefighters. Terrain obscured the firefighters’ view of the fire moving around them, which cut them off from their planned escape. Luckily, the firefighters on foot could crowd into the safety of nearby vehicles and drive out through the flames (US Forest Service, 2021). Some of these firefighters received minor burns, but this was nearly a mass casualty event.
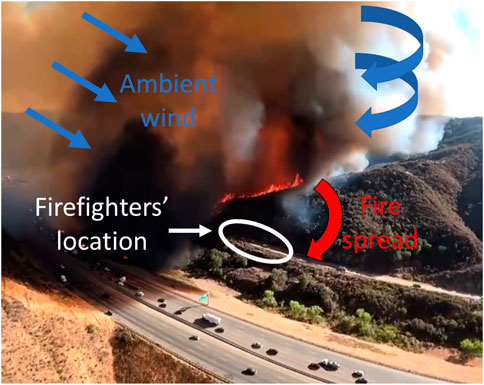
FIGURE 8. The ambient wind combined with a strong thermal plume on the Route Fire to create a large, slowly rotating vortex. The resulting near-surface wind flow caused the fire to spread in a hooking motion, trapping firefighters. Video of the event can be seen at: https://www.youtube.com/watch?v=d4lus6ePx8U.
A year earlier, a firefighter was entrapped in the El Dorado Fire in California and died in another incident where a significant, slow rotation caused unexpected fire behavior (US Forest Service, 2022). That fire also occurred in complex, mountainous terrain with a moderate ambient wind. The landscape, wind, and strong thermal plume generated from a burn-out operation resulted in a pair of large, slow rotations on the lee side of the plume (Figure 9). These were the classic counterrotating pair. Investigators identified this rotation as likely causing the observed locally strong winds and multiple spot fires that entrapped and killed the firefighter.
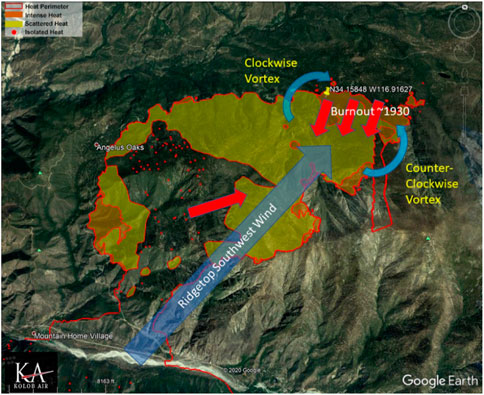
FIGURE 9. Wind, terrain, and a strong thermal plume caused by firefighter burn-out operations on the El dorado fire resulted in a pair of large-scale counterrotating vortices near the downwind side of the fire. Strong, vortex-induced winds in this area increased fire behavior, creating many spot fires. This led to the entrapment and death of one firefighter.
These two incidents underscore the need for further investigation and understanding of these large slow rotations related to wildland fire behavior. Although there has been a significant amount of research into the behavior of thermal plumes in a crossflow ambient wind, application to wildland fire is limited. Many of these studies found that two counterrotating vortices form near the lee side of the plume (Church et al., 1980; Smith et al., 1986; Cunningham et al., 2005). Forthofer and Goodrick (2011) reviewed some of these studies. Observations have also suggested that whirls can be shed from the lee side of a plume, potentially dragging a wildland fire with the whirl and increasing the spread rate.
Identifying conditions conducive to these slow large-scale vortices could help firefighters better anticipate erratic fire behavior. For instance, Finney (Finney et al., 2015) provided a Strouhal-Froude (St-Fr) correlation to scale fires from as small as wind tunnel experiments to as large as the prescribed crown fire that took place in Alaska. However, at very large scales, the applicability of this correlation might suffer because the Coriolis force starts influencing the circulation direction and intensity. As a result, the Rossby number, Ro = (centrifugal force)/(Coriolis force), should also be accounted for at those scales. As such, it will be of interest for future researchers to calculate the Ro-St-Fr numbers for the actual cases of large-scale fires (where slow rotations described in this section and fire whirls are prominent) to enable the performance of dynamically similar small-scale experiments. Therefore, testing the proposed Ro-St-Fr domination in extreme-fire cases.
The effect of global warming on large-scale natural disasters
The relationship between wildfires and climate conditions has been known for decades (Chandler et al., 1983); there is a high fire season during hot summers when the temperatures are high, and the humidity and fuel moisture content is low. Since global warming has resulted in a worldwide increase in the average temperatures (Houghton et al., 1996), it is expected that many studies aimed to investigate the impact global warming had on wildfire severity since the early 1990 s (Flannigan and van Wagner, 1991; Balling et al., 1992; Davis and Michaelsen, 1995). In a later article published in 1998, Josep et al. (Piñol et al., 1998) calculated two wildfire hazard indices (for the years between 1941–1994) based on weather data obtained daily for a locality in northeastern Spain. The goal was to investigate the correlation between the increasing wildfire severity and climate change. They found that the mean value for both indices has increased, with a notable increase in the high-risk days in which the hazard index is very high, resulting from the increase in mean daily maximum temperatures and the decrease in the relative humidity. The increase in wildfire severity in terms of their occurrence frequency and the area burnt has also increased and was highly correlated to both fire hazard indices. Although there are other non-climate-related causes contributing to this increase in wildfire severity, Josep found out that most of the fire impacts took place during extreme drought periods rather than in average dryer weather, which means fire incidence can be more prominently correlated to the day of very high-value-indices rather than the higher mean. They have also indicated a stronger correlation between the weather condition and the number of fires rather than the burnt area. In summary, they concluded a positive correlation between the worsening climate change and the increasing fire occurrence.
Despite that, the effect of global warming appeared settled since Flannigan et al. (1998) presented a controversial discussion and simulations which indicated a decrease in the fire frequency with global warming in certain regions. For instance, they mentioned the decrease in fire frequency in Canada since 1890, although there was a 1.7 Celsius/century temperature increase in that region. These results contradict the general notion that global warming is causing an increase in fire frequency. Such a complication is expected since there are many factors that can affect the fire severity, such as human activities, fire suppression, slash and burn activity, etc. Therefore, to investigate further, Flannigan calculated the Fire Weather Index (FWI), representing the fire spreading intensity based on weather temperature, relative humidity, precipitation, and wind speed. Their results showed that the FWI would be higher (more severe fire) if only the temperature were to increase, keeping all the other variables constant. However, they realized that in some regions, the expected precipitation increased with an increase in atmospheric CO2, leading to a decrease in the FWI, outweighing any increase due to higher temperatures. They also speculated an increase in the relative humidity with warmer weather in some regions, which decreased the FWI values. Eventually, they concluded that the changing fire frequency is tied to the drought frequency rather than the global temperature changes.
In 2006, Westerling et al. (2006) published an article in science to address the earlier warming spring effect on fire activity in western United States forests. They concluded that the increase in global temperature is causing an increase in the duration of the fire season. This resulted in a dramatic increase in the fire frequency and area burnt in recent years compared to a couple of decades preceding 1986. Running (2006) also wrote in the same year, discussing the effect of the rising temperatures on the early snow melting in the western United States. This arid region which 75% of the streamflow comes from snow, is protected by the latter from being a high fire hazard. Once the snow melts, the forest quickly becomes prone to fires due to low humidity and dry, windy weather conditions. Therefore, the global warming effect cannot only be restricted to the resulting increase in temperatures but also involves a compounded effect due to its impact on snowmelt, the duration of the fire season, and the expansion of areas susceptible to extreme fires. This is an important fact, particularly given that the common wildfire statistic in 2006 estimates that 5% of all fires account for 95% of the area burned (Running 2006). To further complicate the problem, wildfires contribute to the global increase in atmospheric CO2, and Westerling et al. (2006) suggested that this will cause a feed-forward loop that will accelerate the crisis. This is seen in the formation of pyrocumulus clouds in the cases of extreme wildfires mentioned in the previous sections, where an increase in the formation frequency and the size of those clouds could dramatically affect climate change trends. Shi et al. (2021) also suggested that global warming is likely to impact fire severity in NE Australia, notably with the deglaciation taking place.
Recently, collective research has shown that the global warming effect on fuel moisture content is the main path through which global warming influences fire severity (Diffenbaugh et al., 2021). In this commentary, the authors refer to the Zhuang et al. (2021) analysis of the warming effect on the vapor pressure deficit (VPD) historical trends. The VPD represents how far below saturated conditions is the atmospheric pressure and is a key element of the wildland fuels’ dry-out rate (Potter 2012). Zhuang analyzed the VPD at the start of the largest fires in California history and indicated that the VPD was indeed the most extremely affected by the warming. They estimated a 1/3 to 1/2 global warming contribution to atmospheric dryness conditions in those fires.
Balch et al. (2022) also noted that global warming is weakening the night-time barrier to fire. This is because, during the night, the lower temperatures and lower VPDs previously acted as a fire retardant. However, in recent years, the hotter and dryer nights led to increased fire intensity during the night-time. Balch concluded that the global-warming-promoted night-time warming is expected to accelerate more severe and intense fires. Recently, in July 2022, an outburst of fires in Spain following a heat wave that struck Europe took the life of two people (Edme and Leicester, 2022). According to this article, thousands of people were forced to evacuate due to more than 30 wildfires around the country, and about 136 km2 of forests were blackened. Meanwhile, following the same heat wave in France, more than 31,000 people were evacuated in less than a week following the fires onset on July 12. In the words of the chief of the regional fire service, “The fire is literally exploding,” where the prime minister of Spain commented: “Climate change kills.”
In conclusion, most of the controversial attitudes towards the global warming effect on wildfires appear to come from restricting the linkage of global warming to an increase in atmospheric temperature only. Many factors, including temperature, relative humidity, and fuel aridity, determine wildfire severity. The effect of global warming on the fire severity should include at least the following elements: its impact on this region’s atmospheric temperature, fuel moisture content, VPD values, snowmelt, precipitation, and streamflow, among others. Overall, global warming appears to be inducing an increased fire intensity in many regions, as well as causing an increase in the areas vulnerable to extreme fires. Finally, although many studies have looked at the linkage of satellite and weather data to correlate global warming with fire severity, it would be of high interest that future studies focus on creating laboratory or field-scaled global-warming-simulating experiments to quantify the effect of various parameters linked with fire severity at those scales. This will help directly evaluate and correlate the impact of global warming at smaller scales, which can be scaled up to a global level, creating a sense of understanding and urgency within the general public to start treating climate change as a serious issue.
Conclusion
This article aims to provide a summary of the fire-whirl research progress and offers crucial observations and insights on critical large-scale fire incidents. Recent observations have shown the significance of slowly rotating motions on overall fire behavior, the possibility of multi-vortex whirls, and vortex breakdown. Also, these observations and related communication provide a vital link between researchers and firefighters, which is necessary to facilitate future advancements and improved safety. Researchers should continue to seek out observations of full-scale fire rotations as discoveries and understanding are still occurring.
The types of configurations for fire-whirls experiments were illustrated and categorized in this work’s experimental and scale modeling section. Some of them are scale models of the prototype fire whirl that occurred naturally in the field, which also implies the conditions for fire whirl-related risks. Some of them can generate relatively stable fire whirl, which is appropriate as a “basic model” to understand fundamental mechanisms. Although the fundamental structure and character of fire whirls have been understood reasonably well through past experiments, some behaviors observed in large-scale wildland fires, such as multi-vortex fire whirls, may require creative thinking to reconstruct them in the laboratory. No attempt was made to include the high-altitude atmospheric effects, which are still missing in the laboratory-scale fire-whirl study. The theoretical section of this article summarizes representative fire-whirl theories that concern axisymmetric configurations. The theories primarily aim to predict the swirling-flow structures induced by buoyancy in a background circulation and the dependence of flame length on flow parameters. Some theories adopt the integral approach and introduce model parameters whose values can be determined by fitting experimental data, while others directly solve partial differential equations to obtain distributions. The occurrence of vortex breakdown and its consequence are briefly mentioned.
The numerical simulation section explains typical CFD models of fire whirls. It emphasizes benefits of modeling since large-scale fire whirls involve a transient turbulent three-dimensional flow with rapid temporal and flow fluctuations, which are difficult to analyze by theory and measure by laboratory and field experiments. Most of the past numerical studies focused on understanding the corresponding laboratory-scale investigations. There are few comprehensive numerical experiments to calculate the behavior of large-scale fire whirls under extreme conditions such as HAFW. Because the reliable dataset for large-scale fire whirls is rarely available, a numerical approach would help investigate and predict the mechanism of large-scale fire whirls. Artificial intelligence (AI), which recently made rapid progress, can extensively support fire prediction and evacuation planning alongside scale and numerical modeling since AI can estimate unmeasured quantities that are not accessible through other means. This is another opportunity for future researchers interested in building a more complex model of the real large-scale fire whirls.
The first case study investigated after the aforementioned sections included fire whirls, tornados, hurricanes, and airplane crash accidents. The detailed explanations and discussions were focused on two historical studies: The Great-Kanto-Earthquake-generated Hifukusho-Ato fire whirls in 1923 and the British airliner crash into Mt. Fuji in 1967. The HAFW study revealed some new aspects of historical documents, the role of pyro-cumulus clouds and microbursts on mass fires and fire whirls, which were not specifically addressed by any of the past researchers who sought the root cause of the HAFW disaster. The low-speed wind-tunnel turbulent-flow experiments over the 1/25,000 scale model of Mt Fuji reveled large circulations behind Mt. Fuji which can only appear under strong wind conditions, these circulations being strong enough to crash the jet-liner into the ground. There is an overlap in interest and relevance between HAFW and Mt. Fuji scale model experiments since they share the Fr number scaling law and the scale model experimental technique to reconstruct large full-scale phenomena in small laboratory experiments.
The second case study on several accidents over the last few years has proven that slower but large-scale rotations can also be dangerous and cause unexpected fire behaviors. Although a Strouhal-Froude correlation to scale fires of different sizes exists in literature, its applicability weakens at vast scales. This is a result of the Coriolis forces being influential at those scales. Therefore, the Rossby number should be considered to enable dynamic similarity and laboratory/field testing to investigate rotating mechanisms at various scales. Finally, mitigating large-scale fire incidents is essential not only for saving lives and property but from an environmental aspect as well. This is because wildfires input a large amount of atmospheric CO2 into the atmosphere, accelerating the global warming crisis, which in turn is increasing the severity and forest vulnerability to extreme fires. This feed-forward loop is causing an exacerbation of both wildfire and global warming catastrophes. As such, it would be of interest in future work to focus on creating scale model experiments of global warming at a field scale to assess the direct impacts of global warming on fires and vice versa, helping our understanding, predicting, and hopefully mitigating any future crisis. More importantly, these experiments can draw the public’s attention to start considering global warming as a serious and direct threat to their safety.
Author contributions
This article was written collaboratively. The following individuals are primarily responsible for each section; Introduction, YN and FW; Observations made during fire-fighting and large-scale control-burns, JF; Field and laboratory experiments and scale modeling, TH; Development of fundamental theory: Fire-whirl theories, KK; Numerical modeling and simulation, MF; Hypotheses-free artificial intelligence to multi-variable problems, AD, KS, and NA; The Type-3 (Hifukusho-Ato) fire whirls and the Froude number scaling law, KS and KSe; The role of large-scale slow rotations in forest fire spreading and fire-fighting, JF; The effect of global warming on large-scale natural disasters, AD; Abstract, conclusions, and discussion, all authors, Article and team coordination, AD.
Acknowledgments
Sponsorship from the Institute of Research for Technology Development (IR4TD) at the University of Kentucky made this international collaboration on large-scale fire disaster studies possible.
Conflict of interest
The authors declare that the research was conducted in the absence of any commercial or financial relationships that could be construed as a potential conflict of interest.
Publisher’s note
All claims expressed in this article are solely those of the authors and do not necessarily represent those of their affiliated organizations, or those of the publisher, the editors and the reviewers. Any product that may be evaluated in this article, or claim that may be made by its manufacturer, is not guaranteed or endorsed by the publisher.
References
Alexandrov, D., Pertseva, E., Berman, I., Pantiukhin, I., and Kapitonov, A. (2019). “Analysis of machine learning methods for wildfire security monitoring with an unmanned aerial vehicle,” in 2019 24th conference of open innovations association (FRUCT), Moscow, Russia, 08-12 April 2019, 3–9. IEEE.
Balch, J. K., Abatzoglou, J. T., Joseph, M. B., Koontz, M. J., Mahood, A. L., McGlinchy, J., et al. (2022). Warming weakens the night-time barrier to global fire. Nature 602 (7897), 442–448.
Balling, R. C., Meyer, G. A., and Wells, S. G. (1992). Climate change in yellowstone national park: Is the drought-related risk of wildfires increasing? Clim. Change 22, 35–45. doi:10.1007/bf00143342
Battaglia, F., McGrattan, K. B., Rehm, R. G., and Baum, H. R. (2000a). Simulating fire whirls. Combust. Theory Model. 4, 123–138. doi:10.1088/1364-7830/4/2/303
Battaglia, F., Rehm, R. G., and Baum, H. R. (2000b). The fluid mechanics of fire whirls: An inviscid model. Phys. Fluids 12, 2859–2867. doi:10.1063/1.1308510
Byram, G. M., and Martin, R. E. (1970). The modeling of fire whirlwinds. For. Sci. 16, 386–399. doi:10.1093/forestscience/16.4.386
Canadell, J. G., Meyer, C. P., Cook, G. D., Dowdy, A., Briggs, P. R., Knauer, J., et al. (2021). Multi-decadal increase of forest burned area in Australia is linked to climate change. Nat. Commun. 12, 6921. doi:10.1038/s41467-021-27225-4
Cappucci, M. (2021). Two rare tornadoes struck northern California on Monday. The Washington Post. Available at: https://www.washingtonpost.com/weather/2021/01/05/northern-california-tornadoes/(Accessed January 5, 2021).
Castelli, M., Vanneschi, L., and Popovič, A. (2015). Predicting burned areas of forest fires: An artificial intelligence approach. Fire Ecol. 11 (1), 106–118. doi:10.4996/fireecology.1101106
Chandler, C., Cheney, P., Thomas, P., Trabaud, L., and Williams, D. (1983). Fire in forestry, vol I. Forest fire behaviour and effects. New York: John Wiley, 450.
Chuah, K. H., and Kushida, G. (2007). The prediction of flame heights and flame shapes of small fire whirls. Proc. Combust. Inst. 31, 2599–2606. doi:10.1016/j.proci.2006.07.109
Chuah, K. H., Kuwana, K., and Saito, K. (2009). Modeling a fire whirl generated over a 5-cm-diameter methanol pool fire. Combust. Flame 156, 1828–1833. doi:10.1016/j.combustflame.2009.06.010
Chung, J. D., Zhang, X., Kaplan, C. R., and Oran, E. S. (2020). The structure of the blue whirl revealed. Sci. Adv. 6, eaba0827. doi:10.1126/sciadv.aba0827
Church, C., Snow, J., and Dessens, J. (1980). Intense atmospheric vortices associated with a 1000 MW fire. Bull. Am. Meteorological Soc. 61, 682–694. doi:10.1175/1520-0477(1980)061<0682:iavawa>2.0.co;2
Clifford, J. (2021). Bushfires inquest hears of last moments of firefighter Samuel McPaul in fire tornado. ABC News. Available at: https://www.abc.net.au/news/2021-09-15/inquest-of-samuel-mcpaul-terrifying-last-moments/100462930 (Accessed September 5, 2021).
Coenen, W., Rajamanickam, P., Weiss, A. D., Sánchez, A. L., and Williams, F. A. (2019). Swirling flow induced by jets and plumes. Acta Mechanica 230, 2221–2231. doi:10.1007/s00707-019-02382-2
Cohen, J. D. (2000). Preventing disaster – home ignitability in the wildland-urban interface. J. For. 98 (3), 15–21.
Cunningham, P., and Reeder, M. J. (2009). Severe convective storms initiated by intense wildfires: Numerical simulations of pyro-convection and pyro-tornadogenesis. Geophys Res. Lett. 36, L12812. doi:10.1029/2009gl039262
Cunningham, P., Goodrick, S. L., Hussaini, M. Y., and Linn, R. R. (2005). Coherent vortical structures in numerical simulations of buoyant plumes from wildland fires. Int. J. Wildland Fire 14 (1), 61–75. doi:10.1071/wf04044
Davis, F. W., and Michaelsen, J. (1995). “Sensitivity of fire regime in chaparral ecosystems to climate change,” in Global change and mediterranean-type ecosystems. Editors J. M. Moreno, and W. C. Oechel (New York: Springer-Verlag), 435–456.
Diffenbaugh, N. S., Konings, A. G., and Field, C. B. (2021). Atmospheric variability contributes to increasing wildfire weather but not as much as global warming. Proc. Natl. Acad. Sci. 118 (46), e2117876118. doi:10.1073/pnas.2117876118
Dye, J. (2021). Fire tornado that killed firefighter during Black Summer sparked by lightning, inquiry hears. The Sydney Morning Herald. Available at: https://www.smh.com.au/national/nsw/fire-tornado-that-killed-firefighter-during-black-summer-sparked-by-lightning-inquiry-hears-20210914-p58rjl.html (Accessed September 14, 2021).
Edme, B., and Leicester, J. (2022). Europe broils in heat wave that fuels fires in France, Spain. Available at: https://apnews.com/article/wildfires-france-fires-heat-waves-b7c3ecaba66d9851ba0381e68d207784 (Accessed July 21, 2022).
Edwards, R. (2021). The online tornado FAQ”. Storm prediction center. Available at: https://www.spc.noaa.gov/faq/tornado/(Accessed June 15, 2021).
Emmanuel, S. C. (2000). Impact to lung health of haze from forest fires: The Singapore experience. Respirology 5 (2), 175–182. doi:10.1046/j.1440-1843.2000.00247.x
Emmons, H. W., and Ying, S. J. (1967). The fire whirl. Proc. Combust. Inst. 11, 475–488. doi:10.1016/s0082-0784(67)80172-3
Emori, R. I., and Saito, K. (1982). Model experiment of hazardous forest fire whirl. Fire Technol. 18, 319–327. doi:10.1007/bf02473115
Emori, R. I., Saito, K., and Sekimoto, K. (2000). Scale models in engineering (mokei jikken no riron to ouyou). Third edition. Gihodo, Tokyo: Elsevier. in Japanese.
Finney, M. A., Cohen, J. D., Forthofer, J. M., McAllister, S. S., Gollner, M. J., Gorham, D. J., et al. (2015). Role of buoyant flame dynamics in wildfire spread. Proc Natl. Acad. Sci. 112 (32), 9833–9838. doi:10.1073/pnas.1504498112
Flannigan, M. D., Bergeron, Y., Engelmark, O., and Wotton, B. M. (1998). Future wildfire in circumboreal forests in relation to global warming. J. Veg. Sci. 9 (4), 469–476. doi:10.2307/3237261
Flannigan, M. D., and Van Wagner, C. E. (1991). Climate change and wildfire in Canada. Can. J. For. Res. 21, 66–72. doi:10.1139/x91-010
Forthofer, J. M., and Goodrick, S. L. (2011). Review of vortices in wildland fire. J. Combust. 2011, 1–14. doi:10.1155/2011/984363
Fromm, M., Tupper, A., Rosenfeld, D., Servranckx, R., and McRae, R. (2006). Violent pyro-convective storm devastates Australia’s capital and pollutes the stratosphere. Geophys Res. Lett. 33, L05815. doi:10.1029/2005gl025161
Fujita, T. T., Watanabe, K., Tsuchiya, K., and Shimada, M. (1972). Typhoon-associated tornadoes in Japan and new evidence of suction vortices in a tornado near tokyo(1). (Kishou Shushi) 50, 431–453. doi:10.2151/jmsj1965.50.5_431
Fujita, T. T. (1971). Proposed characterization of tornadoes and hurricanes by area and intensity. Washington, D.C., United States: NASA. NASA-CR-1255445.
Fujita, T. T. (1981). Tornadoes and downbursts in the context of generalized planetary scales. J. Atmos. Sci. 38, 1511–1534. doi:10.1175/1520-0469(1981)038<1511:taditc>2.0.co;2
Gabbert, Bill. (2019). Fire whirl filmed with infrared camera. Wildfire Today. Available at: https://wildfiretoday.com/2019/04/03/fire-whirl-filmed-with-infrared-camera/(Accessed April 3, 2019).
Gabbert, Bill. (2020). NWS issued fire tornado warning for Loyalton Fire northwest of Reno Saturday. Wildfire Today. Available at: https://wildfiretoday.com/2020/08/16/nws-issued-fire-tornado-warning-for-loyalton-fire-northwest-of-reno-saturday/(Accessed August 16, 2020).
GFED (2022a). Global fire emissions database (GFED). Available at: http://www.globalfiredata.org/.
GFED (2022b). Global fire emissions database. Referred from GFED. Available at: https://www.globalfiredata.org/index.html.
Grishin, A. M., Golovanov, A. N., Kolesnikov, A. A., Strokatov, A. A., and Tsvyk, R. S. (2006). Experimental study of thermal and fire tornados. In Doklady Physics 50 (2), 66–68. Nauka/Interperiodica.
Guardian (2020). Volunteer firefighter Samuel McPaul died when ‘fire tornado’ overturned 10-tonne truck. Guardian Staff Reporter, the Guardian. Available at: https://www.theguardian.com/australia-news/2019/dec/31/volunteer-firefighter-samuel-mcpaul-died-when-fire-tornado-overturned-10-tonne-truck.
Hartl, K. A., and Smits, A. J. (2021). The effect of offset on burner fire whirls. Combust. Sci. Technol. 2021, 1–24. doi:10.1080/00102202.2021.2019230
Hartl, K. A., and Smits, A. J. (2022). The interaction of double burner fire whirls. Combustion and Flame. Combust. Flame 235, 111679. doi:10.1016/j.combustflame.2021.111679
Hassan, M. I., Kuwana, K., Saito, K., and Wang, F. (2005). Flow structure of a fixed-frame type fire whirl. Fire Saf. Sci. 8, 951–962.
Helmholtz, H. (1858). über Integrale der hydrodynamischen Gleichungen, welche den Wirbelbewegungen Entsprechen. J.f″ur die reine und angewandte Math. 55, 25–55. doi:10.1515/crll.1858.55.25
Houghton, J. T., Meira Filho, L. G., Callander, B. A., Harris, N., Kattenberg, A., and Maskell, K. (1996). Climate change 1995. The science of climate change, IPCC. Cambridge: Cambridge University Press, 572.
Jain, P., Coogan, S. C., Subramanian, S. G., Crowley, M., Taylor, S., and Flannigan, M. D. (2020). A review of machine learning applications in wildfire science and management. Environ. Rev. 28 (4), 478–505. doi:10.1139/er-2020-0019
Jones, J., and Miller, B. (2016). How wildfires create towering pyrocumulus clouds. CNN news. Available at: https://www.cnn.com/2016/05/06/weather/pyrocumulus-weather/index.html (Accessed July 25, 2016).
Ju, X., Shiino, K., Matsuoka, T., Yamazaki, T., and Nakamura, Y. (2022). Scale model experiments of fire whirls over the non-fuel zone around an L-shaped fire source. Combust. Flame 238, 111930. doi:10.1016/j.combustflame.2021.111930
Klimenko, A. Y., and Williams, F. A. (2013). On the flame length in fire-whirls with strong vorticity. Combust. Flame 160, 335–339. doi:10.1016/j.combustflame.2012.10.019
Kuwana, K., Matsue, K., Fukumoto, Y., Dobashi, R., and Saito, K. (2022) “Fire whirls: A Combustion Science Perspective”, Combustion Science and Technology 2022. doi:10.1080/00102202.2021.2019234
Kuwana, K., Morishita, S., Dobashi, R., Chuah, K. H., and Saito, K. (2011). The burning rate’s effect on the flame length of weak fire whirls. Proc. Combust. Inst. 33, 2425–2432. doi:10.1016/j.proci.2010.05.049
Kuwana, K., Saito, K., and Williams, F. A. (2020). Fire-whirl movement along line fires – a separated-flow rotating-cylinder analogy. Combust. Sci. Technol. 192, 1160–1172. doi:10.1080/00102202.2020.1712376
Kuwana, K., Sekimoto, K., and Saito, K. (2015). Fire and explosion - scale-model experiment of wind-generated fire whirls. Prog. Scale Model. 2, 167–173.
Kuwana, K., Sekimoto, K., Saito, K., Williams, F. A., Hayashi, Y., and Masuda, H. (2007). Can we predict the occurrence of extreme fire whirls? AIAA J. 45, 16–19. doi:10.2514/1.28533
Kuwana, K., Sekimoto, K., Saito, K., and Williams, F. A. (2008). Scaling fire whirls. Fire Saf. J. 43, 252–257. doi:10.1016/j.firesaf.2007.10.006
Lareau, N. P., Nauslar, N. J., and Abatzoglou, J. T. (2018). The Carr fire vortex: A case of pyrotornadogenesis? Geophys. Res. Lett. 45, 13107–13115. doi:10.1029/2018GL080667
Lei, J., Liu, N., Zhang, L., and Satoh, K. (2015). Temperature, velocity and air entrainment of fire whirl plume: A comprehensive experimental investigation. Combust. Flame 162, 745–758. doi:10.1016/j.combustflame.2014.08.017
Lei, J., Liu, N., and Tu, R. (2017). Flame height of turbulent fire whirls: A model study by concept of turbulence suppression. Proc. Combust. Inst. 36, 3131–3138. doi:10.1016/j.proci.2016.06.080
Lei, J., Liu, N., Zhang, L., Deng, Z., Akafuah, N. K., Li, T., et al. (2012). Burning rates of liquid fuels in fire whirls. Combust. Flame 159, 2104–2114. doi:10.1016/j.combustflame.2012.01.019
Li, S., Yao, Q., and Law, C. K. (2019). The bottom boundary-layer structure of fire whirls. Proc. Combust. Inst. 37, 4277–4284. doi:10.1016/j.proci.2018.05.009
Los Angeles County Fire Department and US Forest Service (2021). Route fire rapid lesson sharing. Wildland Fire Lessons Learned Center. Available at: https://www.wildfirelessons.net/orphans/viewincident?DocumentKey=990d787c-f248-45d2-b503-36a819105d1f (Accessed January 5, 2021).
Lucca-Negro, O., and O’Doherty, T. (2001). Vortex breakdown: A review. Prog. Energy Combust. Sci. 27, 431–481. doi:10.1016/s0360-1285(00)00022-8
McRae, R. H. D., Sharples, J. J., Wilkes, S. R., and Walker, A. (2013). An Australian pyro-tornadogenesis event. Nat. Hazards 65, 1801–1811. doi:10.1007/s11069-012-0443-7
Moore, T., Contreras, T., Murphy, K., Butler, B., Forthofer, J., Bailey, K., et al. (2008). Indians Fire accident prevention analysis. Vallejo, United States: USFS Pacific Southwest Region.
Mullen, J. B., and Maxworthy, T. (1977). A laboratory model of dust devil vortices. Dynamics of Atmospheres and Oceans 1 (3), 181–214. doi:10.1016/0377-0265(77)90006-9
NASA-FIRMS (2022). Fire information for resource management systems (FIRMS). NASA. Available at: https://firms.modaps.eosdis.nasa.gov/.
Piñol, J., Terradas, J., and Lloret, F. (1998). Climate warming, wildfire hazard, and wildfire occurrence in coastal eastern Spain. Clim. change 38 (3), 345–357. doi:10.1023/a:1005316632105
Potter, B. E. (2012). Atmospheric interactions with wildland fire behaviour–I. Basic surface interactions, vertical profiles and synoptic structures. Int. J. Wildland Fire 21 (7), 779–801. doi:10.1071/wf11128
Running, S. W. (2006). Is global warming causing more, larger wildfires? Science 313 (5789), 927–928. doi:10.1126/science.1130370
Safi, Y., and Bouroumi, A. (2013). Prediction of forest fires using artificial neural networks. Appl. Math. Sci. 7 (6), 271–286. doi:10.12988/ams.2013.13025
Saito, K. (1995). “Kufu: Foundations of employee empowerment and kaizen,” in Principles of continuous learning systems (New York: McGraw-Hill).
Saito, K. (2022). The opening lecture at the 9th international symposium on scale modeling. Napoli, Italy: ISSM9.
Saito, K., and Williams, F. A. (2014). Scale modeling in the age of highspeed computation. Prog. Scale Model. 2, 1–16. Springer. doi:10.1007/978-3-319-10308-2_1
Sasaki, T., Igari, M., and Kuwana, K. (2018). Fire whirls behind an L-shaped wall in a crossflow. Combust. Flame 197, 197–203. doi:10.1016/j.combustflame.2018.06.036
Satoh, K., and Yang, K. T. (1996). Proceedings of the ASME heat transfer division. New York, United States: ASME, 393–400.
Satoh, K., and Yang, K. T. (1997). Simulations of swirling fires controlled by channeled self-generated entrainment flows. Fire Saf. Sci. 5, 201–212. doi:10.3801/iafss.fss.5-201
Shi, G., Yan, H., Zhang, W., Dodson, J., Heijnis, H., and Burrows, M. (2021). Rapid warming has resulted in more wildfires in northeastern Australia. Sci. total Environ. 771, 144888. doi:10.1016/j.scitotenv.2020.144888
Sitte, M. P., and Doan, N. A. K. (2022). Velocity reconstruction in puffing pool fires with physics-informed neural networks. arXiv preprint arXiv:2208.03542.
Smith, M. C., Haines, D. A., and Main, W. A. (1986). Some characteristics of longitudinal vortices produced by line-source heating in a low-speed wind tunnel. Int. J. Heat Mass Transf. 29 (1), 59–68. doi:10.1016/0017-9310(86)90034-7
Snegirev, A., Mardsen, J. A., Fransis, J., and Makhviladse, G. M. (2004). Int. J. Heat. Mass Tranf. 47, 2523.
Soma, S. (1988). A study on large scale turbulences created by strong wind in the downstream section of Mt. Japan: Fuji, A special report to the Ministry of Science and Technology and Meteorology Research Institute of Japan. March, in Japanese.
Soma, S., Kuwana, K., Nakamura, Y., Darwish Ahmad, A., and Saito, K. (2022). Downwind turbulent flow-patters created by over-30m/s wind at the summit of Mt. Fuji, to be submitted to Progress in Scale Modeling.
Soma, S., and Saito, K. (1991). Reconstruction of fire whirls using scale models. Combust. Flame 86, 269–284. doi:10.1016/0010-2180(91)90107-m
Soma, S. (1975). Study on the fire-tornado at the former site of the Hifukusho (the army clothing depot). Jpn. J. Geol. 84, 12–25. in Japanese.
Tangey, Chris (2012). Fire tornad Australia HD -clearest ever capture in nature. Available at: https://www.youtube.com/watch?v=lsyvOYcWgcg (Accessed September 18, 2012).
Terada, T. (1925). Reports on whirls generated on September 1, 1923, report of the earthquake prevention committee, 275. in Japanese.
Tohidi, A., Gollner, M. J., and Xiao, H. (2018). Fire whirls. Annu. Rev. Fluid Mech. 50, 187–213. doi:10.1146/annurev-fluid-122316-045209
US Forest Service (2022). El Dorado incident – learning review narrative. Wildland fire lessons learned center. Available at: https://www.wildfirelessons.net/orphans/viewincident?DocumentKey=18cfb96c-caf4-43e1-81c3-0f8ab6349c1 (Accessed January 7, 2022).
US Forest Service (2021). Near miss on the Route fire. firefighters trapped [video]. YouTube. Available at: https://www.youtube.com/watch?v=d4lus6ePx8U (Accessed December 11, 2021).
Weiss, A. D., Rajamanickam, P., Coenen, W., Sánchez, A. L., and Williams, F. A. (2020). A model for the constant-density boundary layer surrounding fire whirls. J. Fluid Mech, 900, A22. doi:10.1017/jfm.2020.513
Westerling, A. L., Hidalgo, H. G., Cayan, D. R., and Swetnam, T. W. (2006). Warming and earlier spring increase western U.S. Forest wildfire activity. Science 313, 940–943. doi:10.1126/science.1128834
Williams, F. A. (2022). A simplified model for the intermediate structure of strong fire whirls, ” Progress in Scale Modeling. Int. J. 3, 03–0102.
Wu, J. M., Vakili, A. D., and Yu, F. M. (1988). Investigation of the interacting flow of nonsymmetric jets in crossflow. AIAA J. 26, 940–947. doi:10.2514/3.9994
Wu, Z., Wang, B., Li, M., Tian, Y., Quan, Y., and Liu, J. (2022). Simulation of forest fire spread based on artificial intelligence. Ecol. Indic. 136, 108653. doi:10.1016/j.ecolind.2022.108653
Xiao, H., Gollner, M. J., and Oran, E. S. (2016). From fire whirls to blue whirls and combustion with reduced pollution. Proc. Natl. Acad. Sci. 113 (34), 9457–9462. doi:10.1073/pnas.1605860113
Zhao, X., Lovreglio, R., Kuligowski, E., and Nilsson, D. (2021). Using artificial intelligence for safe and effective wildfire evacuations. Fire Technol. 57 (2), 483–485. doi:10.1007/s10694-020-00979-x
Zheng, B., Ciais, P., Chevallier, F., Chuvieco, E., Chen, Y., and Yang, H. (2021). Increasing forest fire emissions despite the decline in global burned area. Sci. Adv. 7, eabh2646. doi:10.1126/sciadv.abh2646
Zhou, K., Liu, N., and Yuan, X. (2016). Effect of wind on fire whirl over a line fire. Fire Technol. 52, 865–875. doi:10.1007/s10694-015-0507-9
Zhou, R., and Wu, Z.-N. (2007). Fire whirls due to surrounding flame sources and the influence of the rotation speed on the flame height. J. Fluid Mech. 583, 313–345. doi:10.1017/s0022112007006337
Keywords: large scale fires, global warming, fire whirls, large circulation, scale modeling
Citation: Darwish Ahmad A, Akafuah NK, Forthofer J, Fuchihata M, Hirasawa T, Kuwana K, Nakamura Y, Sekimoto K, Saito K and Williams FA (2023) Large-scale fire whirl and forest fire disasters: Awareness, implications, and the need for developing preventative methods. Front. Mech. Eng 9:1045542. doi: 10.3389/fmech.2023.1045542
Received: 15 September 2022; Accepted: 02 January 2023;
Published: 19 January 2023.
Edited by:
Fumiaki Takahashi, Case Western Reserve University, United StatesReviewed by:
Alexander Smits, Princeton University, United StatesMichael John Gollner, University of California, Berkeley, United States
Copyright © 2023 Darwish Ahmad, Akafuah, Forthofer, Fuchihata, Hirasawa, Kuwana, Nakamura, Sekimoto, Saito and Williams. This is an open-access article distributed under the terms of the Creative Commons Attribution License (CC BY). The use, distribution or reproduction in other forums is permitted, provided the original author(s) and the copyright owner(s) are credited and that the original publication in this journal is cited, in accordance with accepted academic practice. No use, distribution or reproduction is permitted which does not comply with these terms.
*Correspondence: Adnan Darwish Ahmad, YWRuYW5kYXJ3aXNoQHVreS5lZHU=