- 1Departments of Chemistry and Biology, Laboratory for Molecular Photonics, University of Miami, Coral Gables, FL, United States
- 2Nano Carbon Materials, Istituto Italiano di Tecnologia, Genoa, Italy
- 3Department of Chemistry and Industrial Chemistry, University of Genoa, Genoa, Italy
- 4School of Chemical Sciences, Dublin City University, Glasnevin, Ireland
A fluorescent chromophore and a pH-sensitive heterocycle were integrated within a single covalent skeleton to generate four molecular switches with ratiometric fluorescence response. Upon acidification, the pH-sensitive heterocycle opens to shift bathochromically the absorption and emission bands of the fluorescent chromophore. As a result, an equilibrium between two species with resolved fluorescence is established with fast kinetics in aqueous environments. The relative amounts of the two interconverting forms and their relative emission intensities change with pH, providing the opportunity to probe this parameter ratiometrically with fluorescence measurements. Specifically, the resolved emissions of the two species can be collected in separate detection channels of the same microscope to map their ratio across a labeled sample and reconstruct its pH distribution ratiometrically with spatial resolution at the micrometer level. Additionally, the sensitivity of these molecular switches varies with the nature of the heterocyclic ring and with its substituents, allowing the possibility of regulating their response to a given pH range of interest with the aid of chemical synthesis. Thus, a family of valuable fluorescent probes for ratiometric pH sensing in a diversity of samples can emerge from the unique combination of structural and photophysical properties designed into our innovative molecular switches.
Introduction
The quantitative determination of pH in the intracellular space can provide invaluable information on the many processes responsible for regulating cell functions (Weisz, 2003; Boron 2004; Casey et al., 2010). However, the microscaled dimensions of cells in conjunction with the need to ensure minimal structural perturbations restrict the number of analytical methods compatible with these measurements significantly (Loiselle and Casey, 2010; Demuth et al., 2016). In this context, the noninvasive character, micrometer resolution, fast response, and inherent sensitivity of fluorescence measurements (Lakowicz, 2006) and optical microscopy (Murphy, 2001) are particularly convenient. Indeed, membrane-permeable dyes with pH-sensitive emission can be exploited to probe pH in the cytosol and in specific organelles of live cells (Han and Burgess, 2010; Wang et al., 2010; Li et al., 2014; Ni and Wu, 2014; Hou et al., 2017; Specht et al., 2017). Generally, the fluorescence quantum yield of the sensitive molecular probe is designed to change with pH; therefore, the emission intensity measured in a given intracellular region of interest can be correlated quantitatively with pH. Nonetheless, concentration and optical effects can also influence the magnitude of the detected emission intensity, complicating the quantitative determination of pH. These limitations can be overcome with ratiometric measurements, if the sensitive dye is engineered to produce dual emission in resolved spectral windows (Huang et al., 2018; Bigdeli et al., 2019). Under these conditions, the intensity of one emission can be measured relative to the other in order to eliminate any concentration or optical artifact. In addition to the inherent challenge in designing molecular constructs with dual emission, a further complication in the development of such ratiometric probes arises from the need to adjust their sensitivity to a relatively wide range of pH values. In fact, the pH in the intracellular environment can vary from ca. 4 in the acidic lysosomal compartments to as much as ca. 8 in the basic mitochondria (Weisz, 2003; Boron 2004; Casey et al., 2010). Thus, modular structural designs that can easily be modified with the aid of chemical synthesis to ensure ratiometric fluorescence response and tunable pH sensitivity would be especially valuable.
Our laboratories identified a mechanism to activate the fluorescence of a coumarin fluorophore in response to pH (Deniz et al., 2012; Swaminathan et al., 2012). It is based on the opening of a 2H,4H-benzo [1,3]oxazine (oxazine) ring upon acidification with the concomitant bathochromic shift in the coumarin absorption. Selective excitation of the protonated species then results in intense fluorescence at ca. 660 nm. Furthermore, the covalent connection of such a pH-activatable probe to folate ligands allows the activation of fluorescence in acidic endosomal compartments of cancer cells and their discrimination from normal cells (Tang et al., 2017). However, a significant increase in emission intensity is observed only at pH values lower than ca. 5. The need to tune the pH sensitivity of this molecular probe and impose ratiometric response on it suggested the possibility of exploring structural modifications of the original oxazine heterocycle and extending these operating principles to 2H,3H,4H,5H-[1,3]oxazole (oxazolidine) rings, which are also known to open in response to acidification (Szalóki et al., 2015; Fujioka et al., 2020). This article reports a detailed spectroscopic analysis of four members of this family of molecular switches together with representative imaging experiments in model preparations.
Materials and Methods
General Procedures
Chemicals were purchased from commercial sources and used as received. H2O (18.2 MΩ cm) was purified with a Barnstead International Nanopure Diamond Analytical system. Compounds 1, 3, 5–9, and 11 (Supplementary Figures S1,S2) were prepared according to literature procedures (Wu et al., 2007; Tomasulo et al., 2008; Deniz et al., 2010; Zhang et al., 2015; Mazza et al., 2019; Wang et al., 2020). Electrospray ionization mass spectra (ESIMS) were recorded with a Bruker micrOTOF-Q spectrometer. Nuclear magnetic resonance (NMR) spectra were recorded with a Bruker Avance 400 spectrometer. Absorption spectra were recorded in aerated quartz cells (path length = 1.0 cm) with a Varian Cary 100 Bio spectrometer, equipped with an Agilent Technology Cary Dual Cell Peltier accessory. Emission spectra were recorded in aerated quartz cells (path length = 1.0 cm) with a Varian Cary Eclipse spectrometer, equipped with a Varian Cary Single Cell Peltier accessory. Fluorescence quantum yields were determined against 9,10-diphenylanthracene (2Cl and 4Cl) and cresyl violet (2OpH and 4OpH), following a literature protocol (Würth et al., 2013). Fluorescence images were recorded with a Leica SP5 laser-scanning confocal microscope. Dynamic light scattering (DLS) measurements were performed with a Malvern Zen1600 apparatus.
Synthesis of 2
Trifluoroacetic acid (TFA, 160 μL, 2.09 mmol) was added dropwise to a solution of 6 (100 mg, 0.32 mmol) and 7 (79 mg, 0.32 mmol) in EtOH (10 ml). The mixture was heated under reflux for 24 h. After cooling down to ambient temperature, the solvent was distilled under reduced pressure and the residue was dissolved in CH2Cl2 (50 ml) and washed with NaHCO3 (5% w/v, 20 ml) and H2O (20 ml). The organic phase was dried over anhydrous Na2SO4 and filtered and the solvent was distilled under reduced pressure. The residue was purified by column chromatography [SiO2, CH2Cl2/MeOH (98:2, v/v)] to give 2 (9%, 15 mg) as a green solid. ESIMS: m/z = 538.2359 [M + H]+ (m/z calcd. for C32H32N3O5 = 538.2342); 1H NMR (400 MHz, CD3CN): δ (ppm) = 7.81 (s, 1H), 7.64–7.66 (d, 8 Hz, 1H), 7.28–7.35 (m, 2H), 7.17–7.19 (d, 5 Hz, 2H), 6.98–7.09 (m, 2H), 6.82–6.89 (m, 1H), 6.77–6.79 (m, 1H), 6.67–6.72 (m, 2H), 6.51 (s, 1H), 5.02 (d, 19Hz, 1H), 4.78 (d, 19Hz, 1H), 3.41–3.46 (m 4H), 1.52 (s, 3H), 1.16–1.19 (m, 9H); 13C NMR (100 MHz, CD3CN): δ (ppm) = 183.5, 174.3, 137.5, 131.6, 130.5, 129.4, 128.1, 127.5, 126.5, 125.0, 123.1, 122.4, 122.2, 120.4, 109.3, 108.9, 100.1, 80.1, 55.0, 44.6, 38.7, 25.2, 17.8, 11.9.
Synthesis of 4
Palladium(II) acetate (18 mg, 0.07 mmol) was added to a solution of 10 (40 mg, 0.07 mmol) and 11 (20 mg, 0.14 mmol) in Et3N (20 ml). The mixture was heated under reflux and Ar for 24 h. After cooling down to ambient temperature, the reaction mixture was diluted with CH2Cl2 (20 ml) and washed with H2O (2 × 20 ml). The organic phase was dried over anhydrous Na2SO4 and filtered and the solvent was distilled under reduced pressure. The residue was purified by column chromatography [SiO2, CH2Cl2/MeOH (93:7, v/v) and Et3N (1% v/v)] to give 4 (25%, 10 mg) as a green solid. ESIMS: m/z = 563.2888 [M + H]+ (m/z calcd. for C36H39N2O4 = 563.2910); 1H NMR (400 MHz, CDCl3): δ (ppm) = 7.60 (s, 1H), 7.44 (d, 8 Hz, 2H), 7.24–7.31 (m, 4H), 6.96 (s, 1H), 6.87–6.92 (m, 2H), 6.75–6.81 (m, 2H), 6.65–6.72 (m, 1H), 6.59 (d, Hz, 1H), 6.51 (s, 1H), 4.30 (m, 1H), 3.81–3.85 (m, 4H), 3.66–3.67 (m, 2H), 3.41–3.49 (m, 4H), 1.49 (s, 3H), 1.25–1.23 (t, 6H), 1.19 (s, 3H); 13C NMR (100 MHz, CDCl3): δ (ppm) = 161.1, 158.8, 155.8, 150.6, 150.5, 140.4, 139.5, 131.6, 130.8, 128.8, 127.8, 127.3, 127.1, 126.8, 126.5, 125.5, 120.2, 117.1, 114.2, 112.1, 110.5, 109.1, 108.9, 97.2, 63.5, 55.3, 50.1, 47.9, 44.8, 28.6, 20.4, 12.5.
Synthesis of 9
2-Bromoethanol (298 μL, 4.21 mmol) was added dropwise to a solution of 8 (1 g, 3.51 mmol) in MeCN (10 ml). The mixture was heated under reflux and Ar for 24 h. After cooling down to ambient temperature, the solvent was distilled under reduced pressure and the residue was dissolved in hexane (10 ml), sonicated for 30 min, and filtered. The residue was dissolved in CH2Cl2 (50 ml) and stored in a fridge for 2 h. The resulting precipitate was filtered off, washed with CH2Cl2 (20 ml), and dried to give 9 (63%, 910 mg) as a pink solid. ESIMS: m/z = 330.0349 [M–Br]+ (m/z calcd. for C13H17INO = 330.0355); 1H NMR (400 MHz, CD3CN): δ (ppm) = 8.13 (s, 1H), 7.99 (d, 8 Hz, 1H), 7.59 (d, 8 Hz, 1H), 4.52 (t, 5 Hz, 2H), 3.99 (t, 5 Hz, 2H), 2.79 (s, 3H), 1.58 (s, 6H).
Synthesis of 10
TFA (209 μL, 2.65 mmol) was added dropwise to a solution of 7 (100 mg, 0.41 mmol) and 9 (167 mg, 0.41 mmol) in EtOH (8 ml). The mixture was heated under reflux for 24 h. After cooling down to ambient temperature, the solvent was distilled under reduced pressure and the residue was dissolved in CH2Cl2 (5 ml). The addition of Et2O (200 ml) and refrigeration for 12 h resulted in the formation of a precipitate. After filtration, the solid residue was dissolved in aqueous NaHCO3 (5% w/v, 20 ml) and stirred for 1 h at ambient temperature. The aqueous mixture was extracted with EtOAc (3 × 40 ml). The organic phase was dried over anhydrous Na2SO4 and filtered and the solvent was distilled off under reduced pressure to give 10 as a green solid (22%, 50 mg). ESIMS: m/z = 557.1293 [M + H]+(m/z calcd. for C27H30IN2O3 = 557.1301); 1H NMR (400 MHz, CDCl3): δ (ppm) = 7.59 (s, 1H), 7.45 (d, 8 Hz, 1H), 7.34 (s, 1H), 7.25–7.30 (m, 1H), 6.79 (d, 8 Hz, 1H), 6.64 (d, 17 Hz, 1H), 6.56–6.63 (m, 2H), 6.51 (s, 1H), 4.13 (q, 7 Hz, 1H), 3.76–3.82 (m, 1H), 3.54–3.67 (m, 2H), 3.41–3.46 (m, 4H), 1.42 (s, 3H), 1.27–1.21 (m, 6H), 1.17 (s, 3H).
Preparation of Doped Nanoparticles and Beads
CH2Cl2 solutions of 1–4 (0.4–3.1 mM, 10 µL) were individually mixed with a CH2Cl2 solution of Pluronic 123 (2.5 mg ml−1, 600 µL) and stirred for 15 min at ambient temperature. The solvent was distilled off under reduced pressure. The residue was dissolved in H2O (1 ml) for spectroscopic measurements or in an aqueous solution of sodium alginate (4% w/v, 2 ml) for imaging experiments. DLS measurements revealed the hydrodynamic diameter of the doped polymer nanoparticles to be ca. 80 nm. Droplets of the resulting mixture of sodium alginate and polymer nanoparticles were added to an aqueous solution of CaCl2 (0.24 M, 750 µL) with a syringe through a 25-gauge needle. Beads with diameters of a few millimeters formed upon contact of the droplets with the CaCl2 solution. After 2 min, individual beads were suspended in H2O (500 µL) at different pH, ranging from 7.4 to 4.0, and then transferred to a glass Petri dish.
Results
Compounds 1Cl and 2Cl incorporate an oxazine heterocycle and differ in the position of a nitro substituent (Figure 1). Compounds 3Cl and 4Cl have an oxazolidine heterocycle in place of the oxazine ring and differ in the substituent on 2H,3H-indole (indole) heterocycle. Compounds 1Cl and 3Cl were prepared according to literature protocols (Deniz et al., 2010; Mazza et al., 2019). Compounds 2Cl and 4Cl were synthesized in one and three steps, respectively (Supplementary Figures S1,S2), and their structural identities were confirmed by high-resolution ESIMS and 1H NMR spectroscopy.
The absorption spectra (Figures 2, 3) of acetonitrile solutions of 1Cl–4Cl show an intense band at 399–412 nm (ClλAb in Table 1) associated with the coumarin chromophore in all instances. Upon addition of increasing amounts of trifluoroacetic acid (TFA), this absorption decreases in intensity with the concomitant appearance and growth of a new band at 576–594 nm (OpHλAb in Table 1). This additional absorption resembles that of model compound 5 (Supplementary Figure S3) and is indicative of the opening and protonation of the oxazine or oxazolidine heterocycle to form 1OpH–4OpH. Indeed, this structural transformation converts the carbon atom at the junction of the indole and either the oxazine or the oxazolidine heterocycle from sp3 to sp2 and brings the coumarin component in conjugation with the resulting 3H-indolium cation. As a result of the extended electronic delocalization, the main absorption of the coumarin chromophore shifts bathochromically, in agreement with literature on related compounds (Deniz et al., 2012; Swaminathan et al., 2012; Tang et al., 2017).
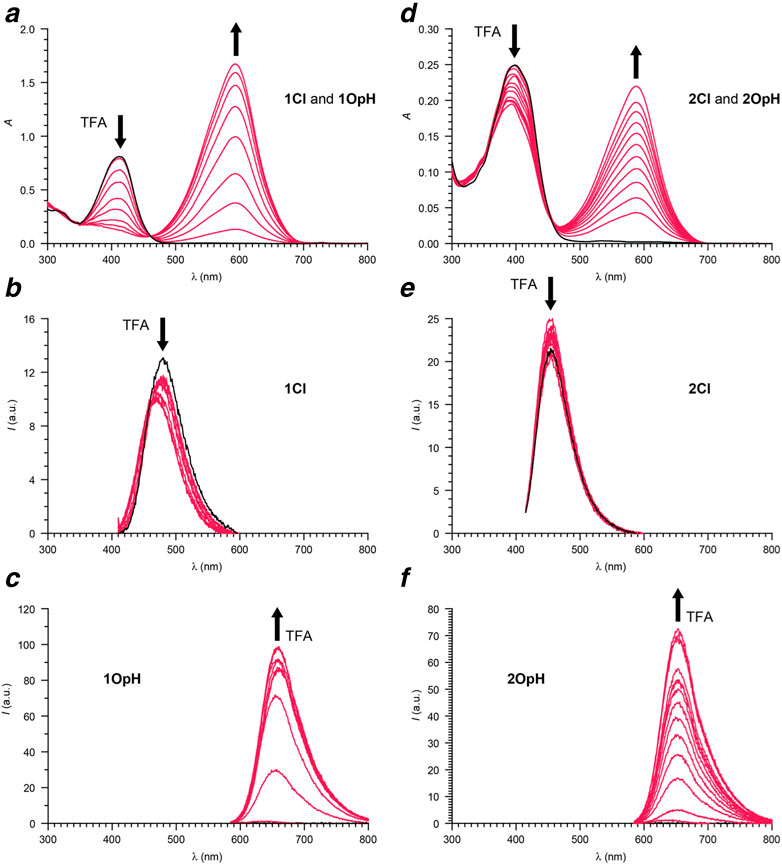
FIGURE 2. Absorption (a) and emission (b, λEx = 405 nm; c, λEx = 575 nm) spectra of a MeCN solution of 1 (22 µM) recorded before (black) and after (red) the addition of increasing amounts of TFA (0.1–1.8 eq.). Absorption (d) and emission (e, λEx = 405 nm; f, λEx = 575 nm) spectra of a MeCN solution of 2 (7 µM) recorded before (black) and after (red) the addition of increasing amounts of TFA (0.1–1.8 eq.).
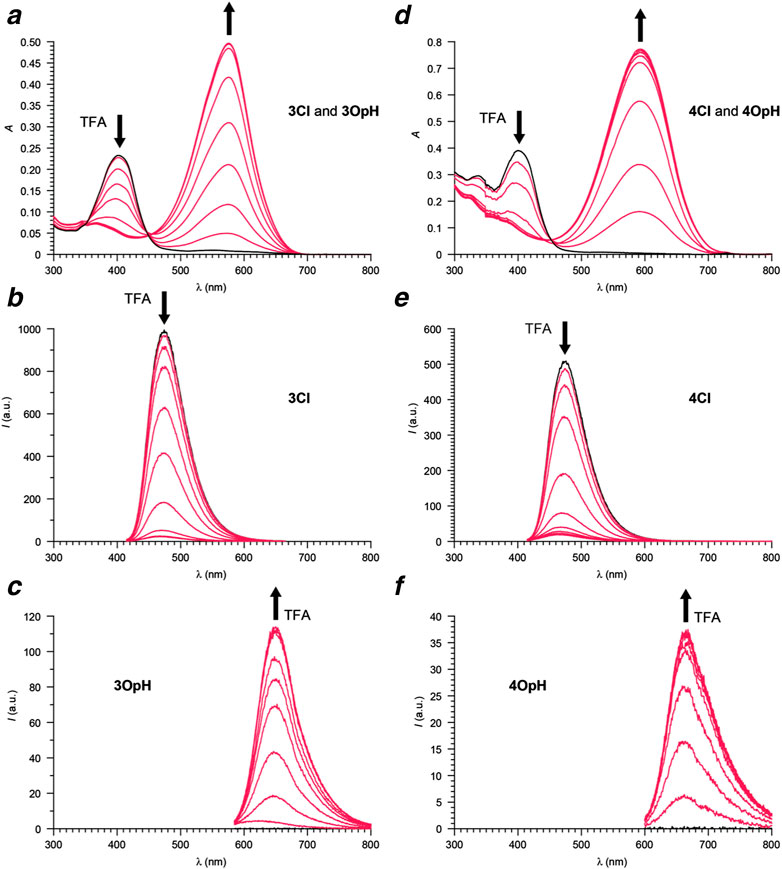
FIGURE 3. Absorption (a) and emission (b, λEx = 405 nm; c, λEx = 575 nm) spectra of a MeCN solution of 3 (6 µM) recorded before (black) and after (red) the addition of increasing amounts of TFA (0.1–1.1 eq.). Absorption (d) and emission (e, λEx = 405 nm; f, λEx = 575 nm) spectra of a MeCN solution of 4 (11 µM) recorded before (black) and after (red) the addition of increasing amounts of TFA (0.1–1.1 eq.).
The emission spectra (Figures 2, 3) of acetonitrile solutions of 1Cl–4Cl reveal an intense band at 454–480 nm (ClλEm in Table 1) for the coumarin chromophore in all instances. However, the fluorescence quantum yields (Clϕ in Table 1) of the oxazine derivatives are significantly lower than those of the oxazolidine counterparts. Upon acidification, this emission band decreases in intensity with the concomitant appearance and growth of a new band at 647–666 nm (OpHλEm in Table 1) for 1OpH–4OpH. Once again, the fluorescence quantum yields (OpHϕ in Table 1) of the oxazine derivatives are significantly lower than those of the oxazolidine counterparts. Presumably, electron transfer from the excited coumarin chromophore to the nitro group is responsible for suppressing the ability of the ring-closed and protonated ring-open forms of 1 and 2 to be emitted.
Compounds 1Cl–4Cl are relatively hydrophobic and essentially insoluble in aqueous environments. However, they readily dissolve in the presence of appropriate amounts (>1 mg mL−1) of Pluronic 123 and retain their photophysical properties as long as their concentration is maintained in the micromolar regime (<50 μM) to avoid aggregation. Indeed, this particular amphiphilic polymer is known to form micelles capable of capturing hydrophobic molecules in their interior and solubilizing them in aqueous environments (Pitto-Barry and Barry, 2015; Jarak et al., 2020). Consistently, DLS measurements on the resulting solutions indicate the formation of nanoparticles with an average hydrodynamic diameter of ca. 80 nm in all instances. Absorption spectra (Supplementary Figures S4,S5), recorded at different pH values, are consistent with the spectral changes observed in acetonitrile upon acidification (Figures 2,3). Specifically, the absorption band of the ring-closed form at 398–412 nm (ClλAb in Table 1) decreases and that of the protonated ring-open form at 565–608 nm (OpHλAb in Table 1) increases upon acidification in all instances. Analyses of the pH dependence of the absorbance at OpHλAb suggest that pKa is 5.15 for 1; 3.51 for 2; 5.97 for 3; 5.28 for 4. Comparison of the values estimated for 1 and 2 demonstrates that the relocation of the nitro group from the para- to the meta-position, relative to the oxygen atom, on the phenylene ring fused to the oxazine heterocycle hinders protonation and ring opening. Comparison of the values determined for 1 and 3 shows that the transition from an ozaxine to an oxazolidine ring has instead the opposite effect.
The pH dependence of the equilibrium between the ring-closed and protonated ring-open forms of 1 to 4, evident from the absorption spectra, translates into dual emission (Supplementary Figures S4,S5). Specifically, the emission band of one species at 465–478 nm (ClλEm in Table 1) decreases and that of the other at 647–671 nm (OpHλEm in Table 1) increases with acidification in all instances, consistently with the behavior observed in acetonitrile (Figures 2, 3). As a result, the ratio between the emission intensities of the ring-closed form (ICl) and that of the protonated ring-open species (IOpH) increase monotonically with a rise in pH. The corresponding plots (Figure 4) show that 1, 3, and 4 respond to pH predominantly between values of ca. 4 and ca. 8. The largest change in the magnitude of the ratio between the two emission intensities is observed for 4. Instead, 2 responds to pH predominantly between values of ca. 2 and ca. 5, demonstrating that the position of the nitro group on the benzoxazine heterocycle has a pronounced influence on the equilibrium between two interconverting species. These observations suggest that the nature and position of the substituents on the pH-sensitive benzoxazine ring can be exploited to tune the response of these molecular switches.
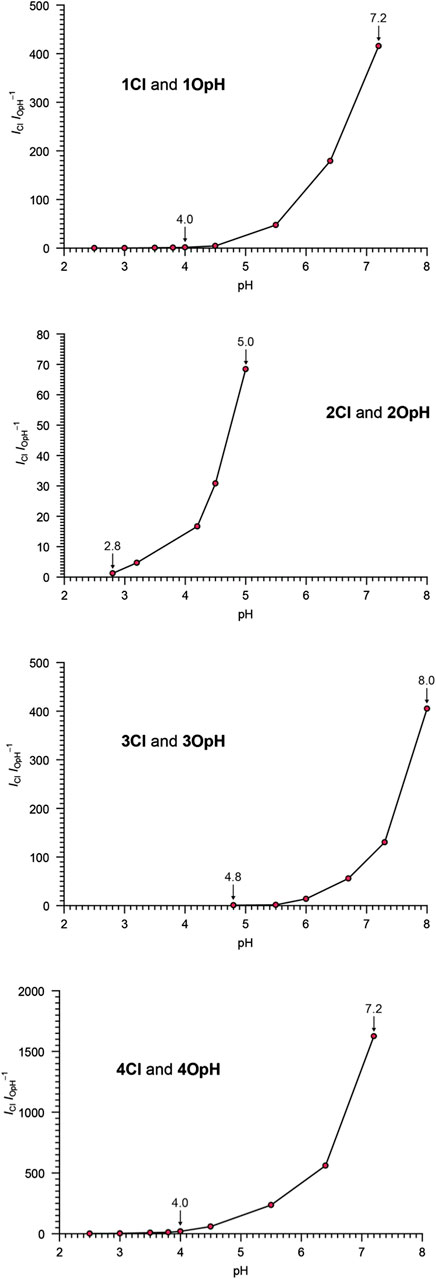
FIGURE 4. pH dependence of the ratio between the emission intensities of Cl and OpH (λEx = 405 or 575 nm) for aqueous solutions of 1–4 (2–8 µM) and Pluronic 123 (1.5 mg ml−1).
The resolved emission bands of the ring-closed and protonated ring-open forms of 1‐4 provide, in principle, the opportunity to probe pH ratiometrically with the acquisition of fluorescence images in separate detection channels of the same microscope. Alginate hydrogels are optimal biocompatible model systems (Seliktar 2012; Rosales and Anseth, 2016; Zhang and Khademhosseini, 2017) to explore these possibilities and allow the identification of appropriate imaging conditions to capture and differentiate the fluorescence of the two interconverting species. Specifically, alginate beads of millimeter dimensions can be doped with nanoparticles of Pluronic 123 containing 4, dispersed in water with a given pH, and imaged. The fluorescence of 4Cl can be collected between 450 and 600 nm (yellow channel in Figure 5) and that of 4OpH can be recorded between 600 and 770 nm (green channel in Figure 5). The resulting images show a fluorescence increase in the yellow channel (a–d in Figure 5) with a concomitant emission decrease in the green channel (e–h in Figure 5) as the pH rises from 4.0 to 7.4. Plots (i in Figure 5) of the emission intensities measured in the two channels further confirm that they are pH-dependent. In fact, the ratio (j in Figure 5) between them increases monotonically with pH, by analogy with the spectroscopic measurements (Figure 4), providing the opportunity to quantify this parameter ratiometrically. Additionally, storage of the sample at a fixed pH does not cause any change in the detected intensities (Supplementary Figure S6), demonstrating that the sensitive probes neither undergo hydrolytic degradation nor photobleaching under these experimental conditions.
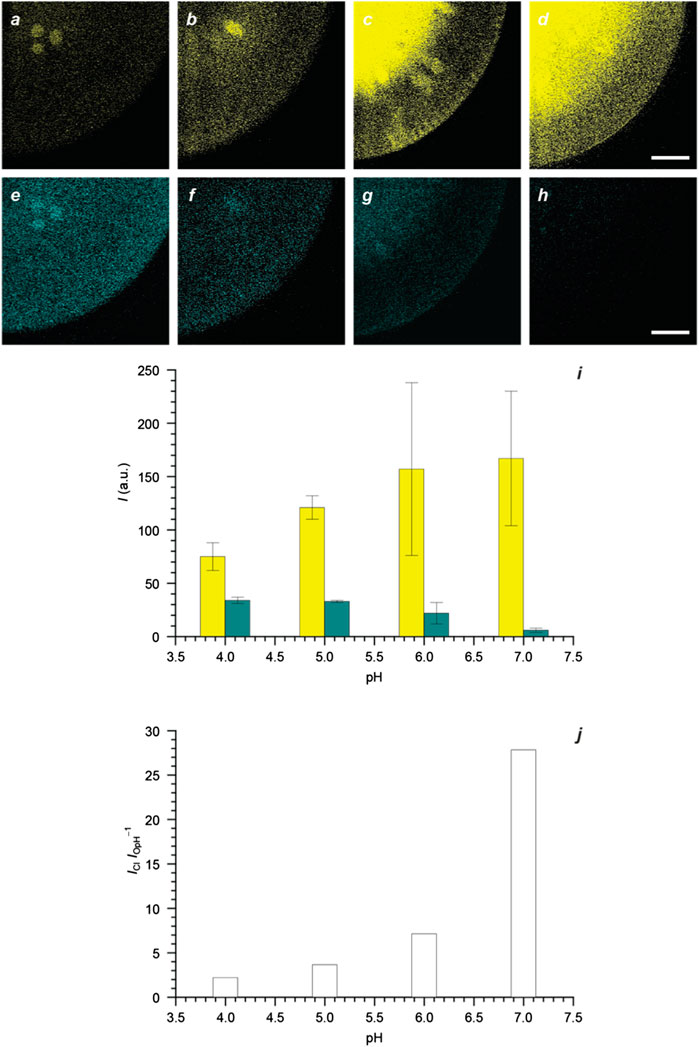
FIGURE 5. Fluorescence images (scale bar = 200 μm) of four alginate beads doped with Pluronic 123 nanoparticles (1.5 mg mL−1) containing 4 (5 μM) and maintained in H2O at pH of 4.0 (a and e), 5.0 (b and f), 6.0 (c and g), and 7.0 (d and h) together with the corresponding emission intensities (i), integrated across the field of view, and their ratio (j) [yellow channel: λEx = 405 nm, λEm = 450–600 nm; green channel: λEx = 561 nm, λEm = 600–770 nm].
Conclusion
The covalent integration of a coumarin chromophore and either an oxazine or an oxazolidine heterocycle within the same molecular skeleton provides the opportunity of assembling molecular switches with ratiometric fluorescence response to pH. The oxazine or oxazolidine heterocycle opens upon acidification to establish an equilibrium between a ring-closed and a protonated ring-open form. In the latter species only, the coumarin chromophore can extend electronic delocalization over an indolium auxochrome. As a result, the protonated ring-open form is absorbed and emitted at wavelengths that are significantly longer than those where the ring-closed species is absorbed and emitted. This behavior enables the selective excitation of the two interconverting forms at distinct wavelengths and the collection of their emissions in resolved spectral windows. Furthermore, the relative amounts of the two equilibrating species and, hence, their relative emission intensities change with pH. It follows that separate detection channels of the same microscope can be exploited to collect the fluorescence of the two components independently and reconstruct a ratiometric profile of the pH distribution of any sample of interest labeled with these compounds.
A significant advantage of this family of molecular switches over existing compounds with ratiometric fluorescence response to pH is their synthetic accessibility and versatility. Three synthetic steps from readily available precursors are sufficient to assemble the parent molecular scaffold of both oxazine and oxazolidine derivatives, offering the opportunity to prepare relatively large amounts of these compounds. Additional synthetic modifications permit the manipulation of the substituents on the indole heterocycle and on the phenylene ring fused to the oxazine ring. These structural manipulations can be exploited to adjust the pH response and, in principle, to introduce hydrophilic chains for aqueous solubility (Cusido et al., 2012) or reactive functional groups for the covalent connection of biomolecules (Cusido et al., 2016; Zhang et al., 2018) or synthetic polymers (Tang et al., 2017). In fact, the fascinating prospect of incorporating targeting agents to direct these molecular switches into specific intracellular components and allow local pH probing can definitely be envisaged. Thus, the combination of photophysical and structural properties that can be engineered into these molecular switches can lead to the realization of versatile fluorescent probes for the intracellular visualization of pH distributions at the microscale with ratiometric imaging schemes.
Data Availability Statement
The original contributions presented in the study are included in the article/Supplementary Material; further inquiries can be directed to the corresponding author.
Author Contributions
MA performed spectroscopic measurements. FC synthesized compounds. JB performed imaging experiments. SG supervised synthesis and contributed to manuscript production. FR designed the experiments, analyzed data, and wrote the manuscript.
Funding
The National Science Foundation (CHE-1505885) and the Istituto Italiano di Technologia (IIT) are acknowledged for financial support.
Conflict of Interest
The authors declare that the research was conducted in the absence of any commercial or financial relationships that could be construed as a potential conflict of interest.
Supplementary Material
The Supplementary Material for this article can be found online at: https://www.frontiersin.org/articles/10.3389/fmats.2021.630046/full#supplementary-material.
References
Bigdeli, A., Ghasemi, F., Abbasi-Moayed, S., Shahrajabian, M., Fahimi-Kashani, N., Jafarinejad, S., et al. (2019). Ratiometric fluorescent nanoprobes for visual detection: design principles and recent advances - a review. Anal. Chim. Acta. 1079, 30–58. doi:10.1016/j.aca.2019.06.035
Boron, W. F. (2004). Regulation of intracellular pH. Adv. Physiol. Educ. 28, 160–179. doi:10.1152/advan.00045.2004
Casey, J. R., Grinstein, S., and Orlowski, J. (2010). Sensors and regulators of intracellular pH. Nat. Rev. Mol. Cell Biol. 11, 50–61. doi:10.1038/nrm2820
Cusido, J., Battal, M., Deniz, E., Yildiz, I., Sortino, S., and Raymo, F. M. (2012). Fast fluorescence switching within hydrophilic supramolecular assemblies. Chemistry 18, 10399–10407. doi:10.1002/chem.201201184
Cusido, J., Ragab, S. S., Thapaliya, E. R., Swaminathan, S., Garcia-Amorós, J., Roberti, M. J., et al. (2016). A photochromic bioconjugate with photoactivatable fluorescence for superresolution imaging. J. Phys. Chem. C 120, 12860–12870. doi:10.1021/acs.jpcc.6b03135
Demuth, C., Varonier, J., Jossen, V., Eibl, R., and Eibl, D. (2016). Novel probes for pH and dissolved oxygen measurements in cultivations from millilitre to benchtop scale. Appl. Microbiol. Biotechnol. 100, 3853–3863. doi:10.1007/s00253-016-7412-0
Deniz, E., Sortino, S., and Raymo, F. M. (2010). Fluorescence switching with a photochromic auxochrome. J. Phys. Chem. Lett. 1, 3506–3509. doi:10.1021/jz101473w
Deniz, E., Tomasulo, M., Cusido, J., Yildiz, I., Petriella, M., Bossi, M. L., et al. (2012). Photoactivatable fluorophores for super-resolution imaging based on oxazine auxochromes. J. Phys. Chem. C 116, 6058–6068. doi:10.1021/jp211796p
Fujioka, H., Uno, S., Kamiya, M., Kojima, R., Johnsson, K., and Urano, Y. (2020). Activatable fluorescent probes for hydrolase enzymes based on coumarin-hemicyanine hybrid fluorophores with large Stokes shifts. Chem. Commun. 56, 5617–5620. doi:10.1039/d0cc00559b
Han, J., and Burgess, K. (2010). Fluorescent indicators for intracellular pH. Chem. Rev. 110, 2709–2728. doi:10.1021/cr900249z
Hou, J. T., Ren, W. X., Li, K., Seo, J., Sharma, A., Yu, X. Q., et al. (2017). Fluorescent bioimaging of pH: from design to applications. Chem. Soc. Rev. 46, 2076–2090. doi:10.1039/c6cs00719h
Huang, X., Song, J., Yung, B. C., Huang, X., Xiong, Y., and Chen, X. (2018). Ratiometric optical nanoprobes enable accurate molecular detection and imaging. Chem. Soc. Rev. 47, 2873–2920. doi:10.1039/c7cs00612h
Jarak, I., Varela, C. L., Tavares da Silva, E., E., T, Veiga, F., Figueiras, A., et al. (2020). Pluronic-based nanovehicles: recent advances in anticancer therapeutic applications. Eur. J. Med. Chem. 206, 112526. doi:10.1016/j.ejmech.2020.112526
Lakowicz, J. R. (2006). Principles of fluorescence spectroscopy. New York, NY, United States: Springer
Li, X., Gao, X., Shi, W., and Ma, H. (2014). Design strategies for water-soluble small molecular chromogenic and fluorogenic probes. Chem. Rev. 114, 590–659. doi:10.1021/cr300508p
Loiselle, F. B., and Casey, J. R. (2010). Measurement of intracellular pH. Methods Mol. Biol. 637, 311–331. doi:10.1007/978-1-60761-700-6_17
Mazza, M. M. A., Cardano, F., Cusido, J., Baker, J. D., Giordani, S., and Raymo, F. M. (2019). Ratiometric temperature sensing with fluorescent thermochromic switches. Chem. Commun. 55, 1112–1115. doi:10.1039/c8cc09482a
Murphy, D. B. (2001). Fundamentals of light microscopy and electronic imaging. New York, NY, United States: Wiley-Liss.
Ni, Y., and Wu, J. (2014). Far-red and near infrared BODIPY dyes: synthesis and applications for fluorescent pH probes and bio-imaging. Org. Biomol. Chem. 12, 3774. doi:10.1039/c3ob42554a
Pitto-Barry, A., and Barry, N. P. E. (2015). Pluronic block-copolymers in medicine: from chemical and biological versatility to rationalisation and clinical advances. Polym. Chem. 5, 3291. doi:10.1039/C4PY00039K
Rosales, A. M., and Anseth, K. S. (2016). The design of reversible hydrogels to capture extracellular matrix dynamics. Nat. Rev. Mater. 1, 15012. doi:10.1038/natrevmats.2015.12
Seliktar, D. (2012). Designing cell-compatible hydrogels for biomedical applications. Science 336, 1124. doi:10.1126/science.1214804
Specht, E. A., Braselmann, E., and Palmer, A. E. (2017). A critical and comparative review of fluorescent tools for live-cell imaging. Annu. Rev. Physiol. 79, 93. doi:10.1146/annurev-physiol-022516-034055
Swaminathan, S., Petriella, M., Deniz, E., Cusido, J., Baker, J. D., Bossi, M. L., et al. (2012). Fluorescence photoactivation by intermolecular proton transfer. J. Phys. Chem. 116, 9928. doi:10.1021/jp307787w
Szalóki, G., Alévêque, O., Pozzo, J.-L., Hadji, R., Levillain, E., and Sanguinet, L. (2015). Indolinooxazolidine: a versatile switchable unit. J. Phys. Chem. B 119, 307. doi:10.1021/jp511825f
Tang, S., Zhang, Y., Thapaliya, E. R., Brown, A. S., Wilson, J. N., and Raymo, F. M. (2017). Highlighting cancer cells with halochromic switches. ACS Sens. 2, 92. doi:10.1021/acssensors.6b00592
Tomasulo, M., Sortino, S., and Raymo, F. M. (2008). Bichromophoric photochromes based on the opening and closing of a single oxazine ring. J. Org. Chem. 73, 118. doi:10.1021/jo7017119
Wang, R., Yu, C., Yu, F., Chen, L., and Yu, C. (2010). Molecular fluorescent probes for monitoring pH changes in living cells. Trac. Trends Anal. Chem. 29, 1004. doi:10.1016/j.trac.2010.05.005
Wang, Y. C., Huang, Y. H., Tsai, H. C., Basha, R. S., and Chou, C. M. (2020). Palladium-catalyzed proaromatic C(alkenyl)-H olefination: synthesis of densely functionalized 1,3-dienes. Org. Lett. 22, 6765. doi:10.1021/acs.orglett.0c02241
Weisz, O. A. (2003). Organelle acidification and disease. Traffic 4, 57. doi:10.1034/j.1600-0854.2003.40201.x
Wu, J. S., Liu, W. M., Zhuang, X. Q., Wang, F., Wang, P. F., Tao, S. L., et al. (2007). Fluorescence turn on of coumarin derivatives by metal cations: a new signaling mechanism based on C=N isomerization. Org. Lett. 9, 33. doi:10.1021/ol062518z
Würth, C., Grabolle, M., Pauli, J., Spieles, M., and Resch-Genger, U. (2013). Relative and absolute determination of fluorescence quantum yields of transparent samples. Nat. Protoc. 8, 1535. doi:10.1038/nprot.2013.087
Zhang, Y., Swaminathan, S., Tang, S., Garcia-Amorós, J., Boulina, M., Captain, B., et al. (2015). Photoactivatable BODIPYs designed to monitor the dynamics of supramolecular nanocarriers. J. Am. Chem. Soc. 137, 4709. doi:10.1021/ja5125308
Zhang, Y. S., and Khademhosseini, A. (2017). Advances in engineering hydrogels. Science 356, eaaf3627. doi:10.1126/science.aaf3627
Keywords: coumarins, fluorescence imaging, molecular switches, oxazines, oxazolidines, pH sensors, ratiometric sensors
Citation: Mazza MMA, Cardano F, Baker JD, Giordani S and Raymo FM (2021) Switchable Coumarins for Ratiometric pH Sensing. Front. Mater. 8:630046. doi: 10.3389/fmats.2021.630046
Received: 16 November 2020; Accepted: 07 January 2021;
Published: 15 February 2021.
Edited by:
Xun Yu, New York Institute of Technology, United StatesCopyright © 2021 Mazza, Cardano, Baker, Giordani and Raymo. This is an open-access article distributed under the terms of the Creative Commons Attribution License (CC BY). The use, distribution or reproduction in other forums is permitted, provided the original author(s) and the copyright owner(s) are credited and that the original publication in this journal is cited, in accordance with accepted academic practice. No use, distribution or reproduction is permitted which does not comply with these terms.
*Correspondence: Françisco M. Raymo, ZnJheW1vQG1pYW1pLmVkdQ==