- 1Hainan Key Laboratory of Marine Geological Resources and Environment, Haikou, China
- 2Shanghai Urban Construction Design and Research Institute (Group) Co., Ltd., Shanghai, China
- 3College of Harbour, Coastal and Offshore Engineering, Hohai University, Nanjing, China
- 4General Institute of Water Resources and Hydropower Planning and Design, Ministry of Water Resources of China, Beijing, China
- 5Marine Geological Survey Institute of Hainan Province, Haikou, China
- 6State Key Laboratory of Coastal and Offshore Engineering, Dalian University of Technology, Dalian, China
Coastal zones are crucial for protecting land from marine disasters, but they are increasingly threatened by erosion caused by storms and rising sea levels. Urban coastal resilience engineering is a multidisciplinary practice that seeks to enhance disaster prevention capabilities and resilience along urban coastlines. This process requires a comprehensive assessment based on the current conditions and regional characteristics, followed by tailored planning and design strategies. Previous research has mainly focused on individual coastline types, utilizing observations or numerical models for analysis. However, it often lacks a comprehensive approach that integrates planning, design, and assessment. This paper proposes a project life cycle management method for resilient coastal zone engineering, including design and construction within a layout planning framework. The proposed scheme incorporates small-scale numerical simulations for the evaluation and employs high-precision remote sensing data collected over different construction periods to assess the coastline’s transformations during the process of construction. Additionally, a hierarchical evaluation index system was established using the analytic hierarchy process to assess project outcomes after completion. The Haidian River-Haikou Bay Coastal Resilience Project serves as a case study and this study thoroughly evaluates the project’s impact on the vitality and resilience of the coastal zones. This research provides valuable insights and practical guidance for future coastal resilience restoration efforts in diverse urban contexts.
1 Introduction
Urban coastlines span over 400,000km globally and support critical infrastructures, ecosystems, and approximately 40% of the world’s population (Adger et al., 2005; Martínez et al., 2007). Over 65% of cities with populations exceeding 2.5 million are located in estuaries within coastal zones. In China, the combined mainland and island coastline extends approximately 32,000 km, with more than 50% of the large cities, 40% of its small and medium-sized cities, and 42% of the population. These areas contribute 60% of the nation’s GDP (China’s National Bureau of Statistics, 2023a, 2023b; Wang and Zhang, 2024). However, the growing coastal population (Navas et al., 2012; Neumann et al., 2015) and the intensified activities involving built infrastructure and sea defenses—whether man-made or nature-made—have highlighted the need to understand how these systems respond to both slow-onset impacts such as sea-level rise, and episodic and immediate impacts such as storms. Detailed studies on these systems are essential to understand their behavior at scales relevant to societal needs (Malvarez et al., 2021).
Resilience refers to a system’s capacity to withstand any disturbances and return to its original state. Canadian ecologist C. S. Holling introduced the concept of resilience in ecological systems to better understand the persistence of natural systems increasingly affected by human activities over recent centuries (Holling et al., 2004). Applying the concept of resilience to urban areas provides insights into strengthening cities against perturbations, minimizing rebuilding costs and reducing the impact on its citizens. Resilience is greatly affected by the city’s governance and infrastructure (Prasad et al., 2009). Recent initiatives, such as the Sustainable Development Goals (Goal 11) and the Sendai Framework for Disaster Risk Reduction 2015–2030 have prioritized the importance of resilience and disaster risk reduction (Kumar and Bindu, 2022).
Urban shorelines are broadly divided into natural shorelines (sandy, silt, and bedrock) and artificial shorelines (structures including seawalls, dikes, and retaining walls). Shoreline protection plays a vital role in preventing coastal zone retreat and maintaining the shoreline’s width to enhance resilience efficiency. Engineering methods that utilize nearshore hydrodynamic and sediment movement have been employed to improve the safety of urban coastal zones. Koster (2006) detailed the wave-dissipating function of artificially submerged sandbars and their role in replenishing berm sand through continuous sediment transport. Li et al. (2024) analyzed grain size distribution and proposed an exponential function for the equilibrium configurations of sandy–muddy transitional beach berms, incorporating factors such as boundary conditions, tidal ranges, and wave heights. Additionally, Li et al. (2022) observed that wave dynamics in intertidal zones of dissipative beach berms are significantly influenced by tidal levels with breaking dissipation peaking at the intertidal sandbar before diminishing further onshore Li has also found the reef-fronted beach show resilience to erosion induced by offshore sediment transport in physical experiments on the cross-shore profile (Li et al., 2024). Bayle et al. (2023) implemented a layered cobble beach design, with particle sizes decreasing from top to bottom. Field observation over 9 d revealed that tidal variations caused cobble particles to migrate offshore and accumulate at the toe of the slope, while erosion occurred in the underlying sand layer. However, the sand-cobble mixture layer effectively prevented further loss of the sand. Blenkinsopp et al. (2011) demonstrated that the net sediment flux of cobble beaches is approximately 1/63 of that of sandy beaches, as cobbles are less easily mobilized than sand. Feagin et al. (2023) highlighted the role of vegetation in increasing sand dune saturation, which can lead to greater sediment collapse and erosion at the edge of vegetated zones compared to non-vegetated areas. Therefore, the effectiveness of beach vegetation in protecting against sediment erosion remains uncertain. Current research provides inconsistent findings on whether vegetation promotes or inhibits sediment suspension, and under what conditions it exerts such effects. There is an urgent need for more biologically inspired and effective ways to repair coastal zones damaged by extreme events such as storm waves and surges. Resilience engineering extends beyond recovery efforts to integrate landscape design, economic considerations, and public appeal. The interdisciplinary nature of coastal resilience engineering involves the complex integration of coastal hydrodynamics, embankment construction, nearshore ecological restoration, and landscape diversity. However, policy needs to be explored in planning, design, engineering and operation management (Zhang and Jin, 2023) there remains a significant research gap in addressing the entire process—encompassing planning, design implementation, and management. Most previous studies have focused on isolated aspects of the process, resulting in limited understanding and inadequate assessment of the effectiveness of coastal resilience restoration projects.
This paper examines a representative case of coastal resilience restoration engineering in a marine city, incorporating multiple factors from the early planning stages. It evaluates and analyzes the benefits to the coast following project completion through a combination of analytic hierarchy process (AHP) methodology and remote-sensing imagery to evaluate and monitor coastal changes. The findings emphasize the impact of the Haidian River-Haikou Bay Coastal Resilience Project (HHCRP) on surrounding development, providing valuable insights for future coastal resilience and restoration initiatives.
2 Study area
2.1 Coastline status
The HHCRP is located in the northern part of Haikou City, covering an alongshore distance of over 19.6 km from east to west and a planning area of 3.84 km2 (Figure 1). The project area is characterized by distinct coastal features including tidal currents, offshore waves, and human activities. During high tide, currents flow from west to east with a maximum velocity of 22.5 cm/s, while during low tide, the flow is revised from east to west, reaching a maximum velocity of 44.8 cm/s. The western section features a semi-circular bay opening to the north, facing the Leizhou Peninsula in Guangdong Province (Figure 1H), and is influenced by offshore waves. Known as the “golden coastline”, this area serves as an area of urban development and a tourist hub attracting significant attention. However, unsustainable private sector developments, including real estate projects, have heightened vulnerability in the region. The coastline comprises three primary features: (1) Tidal channel embankment along with the Haidian River. The retaining walls, in use for over 30 years, provide structural support but exhibit signs of damage and hollowing. (2) Seawall in Haikou Bay. The eastern coastline of Haikou Bay consists of artificial structures, including retaining walls and dams with embankment elevations between 3.0 and 3.2 m. These elevations exceed the mean water level but fall below extreme high tide levels. Although the seawalls have served for decades, they are now significantly damaged. (3) Sediment barrier in shallow water. The western coast of Haikou Bay features a semi-circular bay open to the north with water depths ranging from 2.0 to 6.0 m. Sand barriers emerge during low tides and are submerged at high water levels (Figures 1A–G). Zhou et al. (2023) observed that offshore sandbars and ridges can mitigate wave energy, providing beach protection. However, these features can also increase the risks of beach mudding and blackening in Haikou Bay.
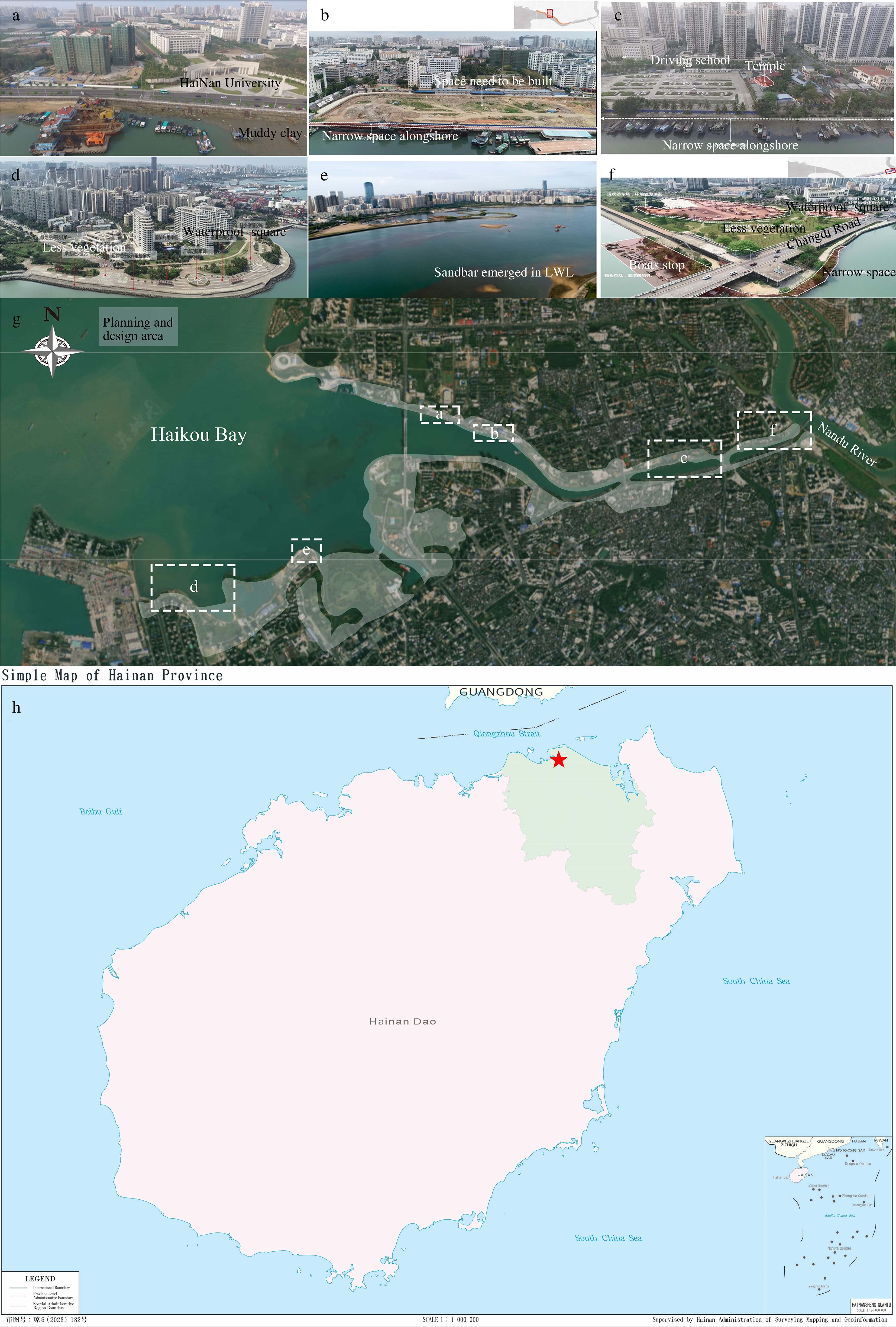
Figure 1. (A–F) are photographs before the project corresponding to the dotted boxes and numbers shown in the satellite plan; (G) is the implementation area of HHCRP located in Haikou City; (H) is the study area in the northern part of Hainan island in south China.
2.2 Hydrodynamics
Storms cause significant marine hazards, producing extremely high waves and storm surges. Between 1975 and 2015, Haikou City experienced an average of over 81 tropical cyclones, with approximately two cyclones making landfall annually. Flooding events typically coincided with rainstorms occurring from May to November. Storm surges, particularly severe when storms approach from the north and east, have occurred over 164 times in the past 55 years, with 42 surges exceeding 1 meter and the remainder over 0.3 m.
In Haikou, the high water level (HWL), mean water level (MWL), and low water level (LWL) are 2.93 m, 0.61 m, and −0.49 m, respectively, relative to the local datum levels. The HWL and the LWL are the mean high-water and low-water spring tide levels, respectively. Wave conditions in the region are dominated by wind and swell waves, predominantly from the north (N-dir), northeast (54%), and north-northeast (43%). The largest waves typically originate from N-dir with heights exceeding 3.0 m, and wave periods of approximately 6.0 s. High-energy storm waves, primarily caused by tropical cyclones, are most frequent between September and October. In contrast, wave activity is relatively subdued from October to June. In Haikou Bay, tropical cyclones generally produce maximum wave heights below 1 m.
2.3 HHCRP scheme
The local government initiated a plan to transform the coastline into an “attractive shoreline open space” accessible to the public. The proposal includes a “three-way continuous traffic system” incorporating walking, running, and cycling paths, alongside communal facilities such as large squares, rest areas, and small recreation spaces to enhance public services. The project aims to infuse vitality into the urban coastline to attract investment, improve the “slow-traffic system” for greater citizen comfort, and ensure the coastline meets safety requirements for flood control while expanding waterfront wetlands. By creating an open and resilient space, the urban coastline’s disaster-prevention capabilities and overall resilience will be significantly improved.
The HHCRP spans more than 19.6 km along the coastline, covering an area of 3.84 km2. The project begins at the eastern Nandu River and extends to western Dujuan Road. The main components of the construction include a slow-traffic system, embankment upgrading and reconstruction, resilience space creation, landscape environment transformation, and communal service facilities. The planning and design process adheres to the following principles: (1) Enhancing functional diversity: Integrate urban scenery, cultural promotion, rest areas, and recreational activities. The concept emphasizes creating public green spaces with ecologically friendly and attractive outdoor activities, including walking, running, and cycling. (2) Restoring coastal ecology: Focus on ecological system restoration by planting diverse flowers, shrubs, and trees. Establish new spaces that enhance coastal resilience and promote biodiversity. (3) Strengthening embankments: Incorporate landscape elements into structural restorations to create attractive urban shorelines. (4) Applying engineering economics: Develop cost-efficient plans and designs for terrain, garden pathways, and flooring to minimize expenses. Implement a scientifically designed operational mechanism for effective management and execution. (5) Sustainable design: Prioritize the use of natural renewable energy, reduce non-renewable resource consumption, and preserve existing trees to support resource regeneration.
We constructed a platform extending from the embankment into the water, creating open spaces for visitors to relax and enjoy the views. The platform’s elevation gradually increases inland, with the highest point meeting flood protection standards. This design effectively forms a secondary, invisible retaining wall, enhancing defense against storm surge erosion in urban areas. The old retaining wall serves as the primary barrier against extreme winds and waves, complemented by resilient spaces and green vegetation. This multi-layered approach strengthens the coastal zone’s resilience, mitigating destructive effects on urban areas. To improve the visitor experience, 16 resting stations were strategically placed along the shore. Each station features unique amenities such as shaded areas, restrooms, drinking water facilities, and spaces for relaxation, enhancing public service functions within resilient spaces (Figure 2).
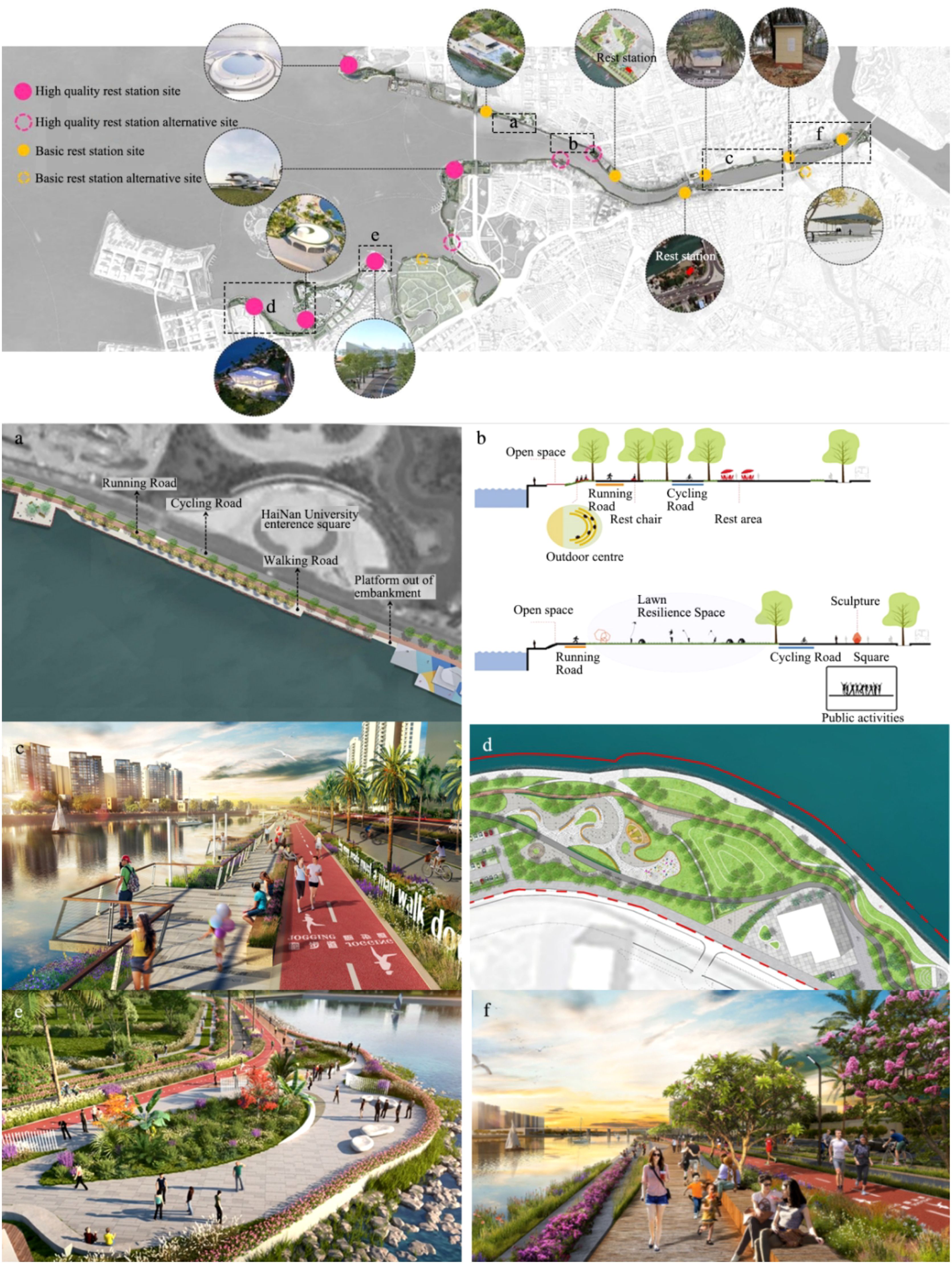
Figure 2. HHCRP planning sketch. (A–F) shows a planning sketch in each part of the study area corresponding to the dotted boxes and numbers shown in the satellite plan.
The platform elevations vary in different parts to meet flood and storm surge safety requirements. The platform outside the embankment extends the open spaces using high piles and multi-ribbed beam structures (Figures 3A–C). In areas where the embankment retaining walls were damaged or collapsed, an in-situ reinforcement method was used for repairs. Hollow sections were filled using shotcrete as an adhesive, saving significant time, and reducing environmental disruption. This approach successfully addressed challenges posed by floods and tidal currents.
The construction of flood and tide control engineering systems was based on population density, economic development, and flood disaster risk levels. The retaining walls in the existing seawalls were modified with ecological considerations in mind. The ecological restoration method integrates the “sponge” concept—absorbent and erosion-resistant designs. This includes rock slopes with a ratio of 1:2.0, using 250–350 kg rocks in front of embankments to buffer storm waves. Revetments and platforms with toe stones were added for additional stability. The first-level platform, 5–8 m wide, includes sand or planting troughs filled with soil for mangrove growth. The second-level platform, more than 2.0 m wide, is positioned with its top 0.5 m below the MWL (Figures 3D–F).
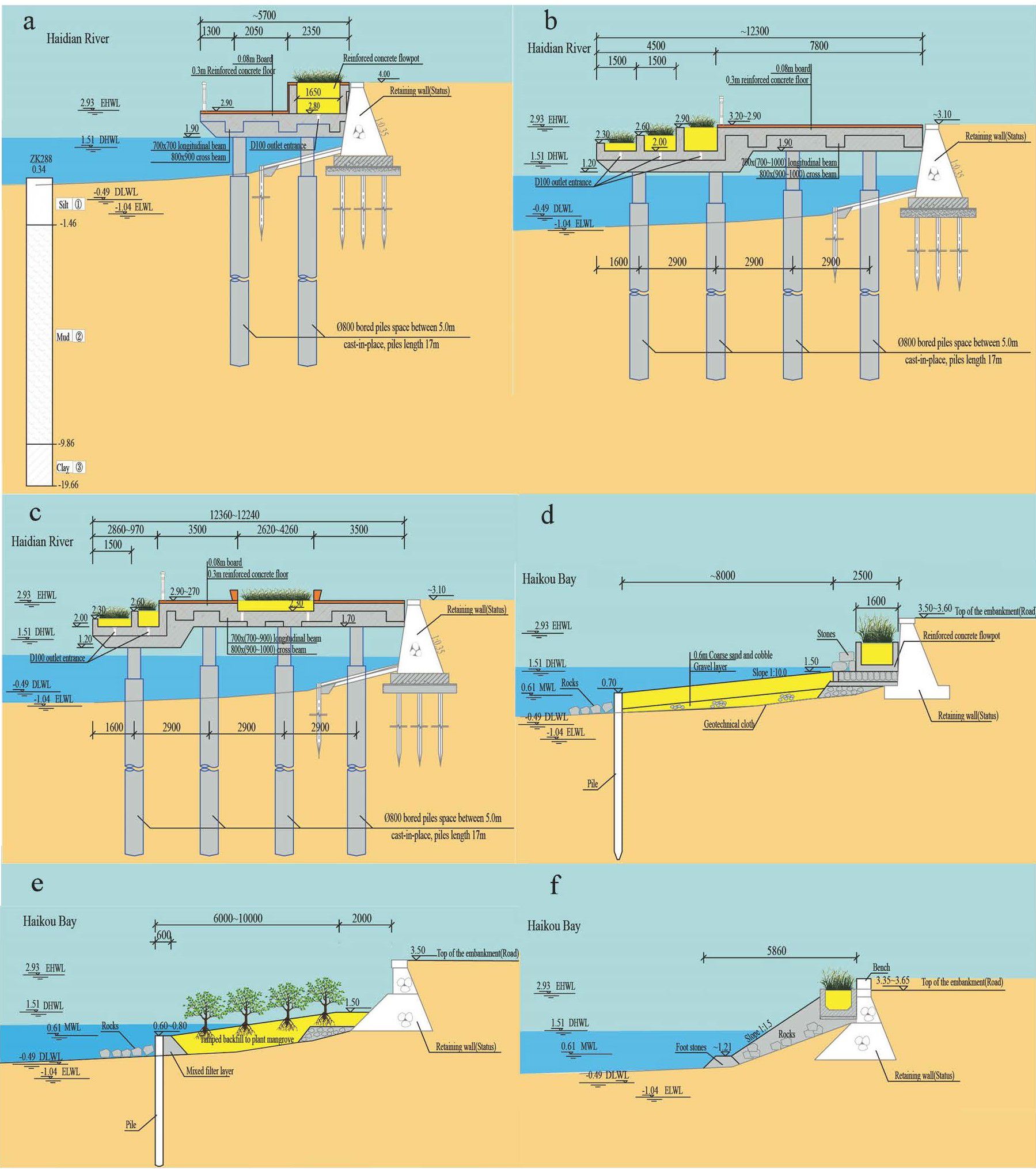
Figure 3. Design profile in the HHCRP. (A–C) is the platform structure out of the embankment in the Haidian River; (D–F) is the nearshore seawall biological reinforcement profile facing the open sea in Haikou Bay.
The project emphasizes ecological restoration, fostering a natural balance while meeting urban community needs and improving urban coastal resilience.
3 Methods
3.1 Numerical models setup
A coupled XBeach and ANSYS model was utilized to evaluate the hydrodynamic and structural interactions within the Haidian tidal channel. The analysis focused on evaluating the deformation and structural responses of the platform constructed as part of the resilience engineering project under various hydrodynamic conditions. Bathymetry measurement was conducted in December 2020 (Figure 4A). The dynamic simulation used the Surfbeat module in XBeach, with resolutions from 0.2 to 4 m. Wall boundaries were applied to the embankment location, and sediment transport was modeled using the van Thiel–van Rijn equation (Elsayed and Oumeraci, 2017).
The XBeach model was validated against observed tidal levels from a tidal station (Figure 4A). While the model effectively captured tidal levels and current transport patterns, the simulated crest values were slightly lower than the measured data. This discrepancy may stem from the tidal station’s location in a narrow section of the tidal channel, near the downstream southern bank of the Heping Bridge, where depth measurement inaccuracies could introduce some bias. Despite this, the influence of wave transport from the open sea into the tidal channel diminishes gradually upstream owing to the terrain’s features. Consequently, this reduction in wave energy ensures that the discrepancy does not significantly impact the qualitative analysis of the overall dynamic characteristics of the Haidian River (Figure 4B).
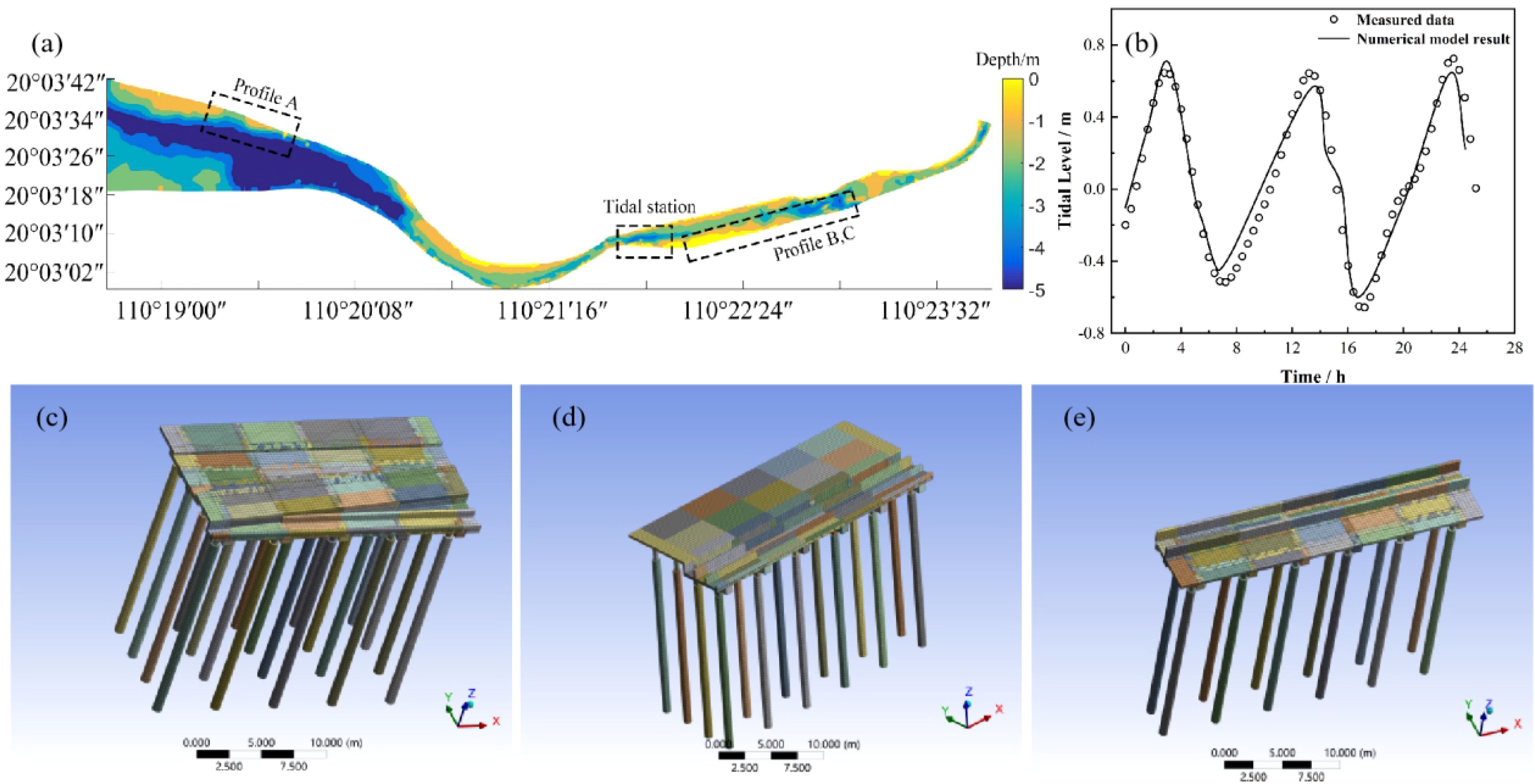
Figure 4. Water depth and model grid. (A)Water depth in Haidian River; (B) is model validation in the tidal station in a dotted box in (A); (C–E) Profiles A, B, and C in the dotted boxes shown in (A).
Three characteristic profiles were selected for the ANASYS model setup, with a longitudinal calculation segment length set to 15 m. The upper load configuration included the structural self-weight, a 5 kPa load for people load, and a 16 kPa for planting load. Profile A is located at the entrance of Hainan University on the northern bank of the Haidian River. It faces a wide water area which influenced by tidal currents from the western side of the river mouth. The upper structure of the platform is an integral beam-slab design, featuring regular structural elements and planting troughs measuring 1.65m. Profiles B and C are located along Changdi Road on the south bank of the channel. They share the same integral beam-slab structure as Profile A (Figures 4C–E). All three profiles include flowers and trees. The model outputs included shear forces, and bending moments in the beam system, axial forces in the pile foundation, and pile displacements at the pile head. Structural analysis was conducted using the STATIC STRUCTURE module in ANSYS Workbench. To enhance computational efficiency and accuracy, the beam system and piles were simplified into one-dimensional beam models. The platform panel was simplified into a two-dimensional shell model using three-dimensional eight-node finite-strain shell elements. The connection between the beam system and piles was assumed to be rigid. The high-pile platform operates with a defined load transfer path: the platform panel transfers loads to the longitudinal beams. These forces are then transmitted to the transverse beams. Loads are ultimately passed to the piles and, subsequently, to the soil foundation. This approach ensures the model accurately captures internal forces, such as shear forces, bending moments in the beam system, axial forces, and displacements at the pile head for all three profiles (A, B, and C). XBeach provided hydrodynamic outputs such as water levels, wave heights, and flow velocities at specified points, which were used as input parameters in ANSYS. These hydrodynamic conditions determined the stress distribution on the structure. When water levels are below the panel, the structure experiences horizontal loads due to waves and currents acting on the piles. When water levels are higher than that of the panel height, the structure undergoes upward deformation due to buoyancy forces.
3.2 Remote-sensing analysis
High-resolution remote-sensing analysis is an important method for assessing urban coastal development. In this study, we utilized WorldView-2 satellite data with 1.8 m resolution multispectral images to monitor changes in the coastal area during project implementation. This approach provided valuable insights into the surrounding area’s transformation, both positive and negative. Although the analysis did not specifically capture human assemblies, the findings clearly indicate that the project has significantly increased coastal green spaces and expanded open areas. These changes have enhanced the coastal area’s resilience and spatial capacity, achieved through unified planning and design.
3.3 Public involvement
Qualitative interviews were carried out to gather insights from local communities, who are key stakeholders directly impacted by and familiar with the coastal environment and its dynamics. The study included over 120 participants from the local community, selected based on defined criteria, such as residency in the area during the engineering period (2018–2023). The interviews focused on the following aspects:
1. Space size: Participants were asked how the project impacted their lives post-completion, including changes in space width, transportation convenience, and economic attractions.
2. Biological factors: User satisfaction with the landscape environment was assessed, including comfort levels and areas for improvement. Topics included animal and plant landscapes, local microenvironments, water features, and quality.
3. Disaster prevention and mitigation: Feedback was gathered on whether the project improved conditions related to water accumulation and road destruction outside the engineering range.
4. User behaviors: Participants described activities they engaged in, such as walking, running, cycling, family outings, parties, and group activities.
5. Public facilities: Satisfaction with the availability and quality of public spaces and facilities was evaluated.
A detailed stakeholder analysis was carried out to develop efficient coastal management strategies. Effective erosion protection requires proposals that can address the diverse concerns of societal sectors to achieve impactful results (Dasgupta and Serageldin, 2000). Stakeholder groups were identified based on attributes, interrelationships, and interests related to the coastal management issues (Buanes et al., 2004; Mushove and Vogel, 2005). Particular attention was given to stakeholders with power and legitimacy, such as government officials and influential decision-makers (Mitchell et al., 1997). All of these criteria were analyzed to achieve better solutions, despite occasional conflicting inputs from certain stakeholders (Saengsupavanich et al., 2009), with the aim of resolving the issues at hand. Following stakeholder consultation, plans for breakwaters and beach nourishment in the critical erosion areas were proposed. These solutions addressed the needs of all relevant stakeholders, ensuring the integration of diverse viewpoints into a cohesive coastal management strategy.
The performance evaluation method is a crucial approach for quantifying coastal resilience. In this study, we employed the AHP, a decision-making methodology proposed by Saaty (1980), to evaluate the performance of coastal space restoration. AHP integrates qualitative and quantitative analyses, systematizing the decision-making process by converting qualitative judgments into quantitative measures. The method conceptualizes the problem as a system, identifies relationships among factors, organizes them into hierarchical levels, and constructs a hierarchical model. Using the AHP 1–9 scale, a survey was conducted to evaluate the studied objectives. A judgment matrix was then derived through pairwise comparisons, and its eigenvector was calculated to represent the relative weights of the elements. The eigenvector represents the relative weights of each element concerning higher-level elements, converting expert qualitative assessments into numerical values. This process ultimately derives the combined weights of evaluation indicators for achieving the overall goal. The advantages of AHP include its ability to address complex issues that are difficult to quantify, combine qualitative and quantitative analysis, involve decision-makers directly in the process, and maintain consistency. AHP is a simple decision-making method for complex, ambiguous issues, particularly useful when full quantification is challenging. It is a convenient and flexible multi-criteria decision-making method.
For all the target layers, we focused on one element: the feasibility of the HHCRP. At the criteria level, we identified five elements, covering coastal space factors, ecological factors, disaster prevention and mitigation, activity behaviors, and public facility needs. At the indicator level, we established 20 elements. By combining qualitative and quantitative analyses, we created an evaluation system to comprehensively assess the operational status of the HHCRP (Table 1).
4 Results
4.1 Dynamic-structure interaction simulation
The Haidian River is a tidal channel gradually narrowing from west to east, with depths gradually becoming shallower from the western river mouth to the eastern end. This variation induces non-linear waves and current transport in shallow waters. At Profile A, the wave height is less than 0.2m and flow velocities exceed 0.5m/s. However, the flow velocities drop below 0.1m/s in profiles B and C, owing to the wall boundary on both sides. The wave-current interaction predominantly influences flow velocities within the tidal channel. Figures 5B, C shows the cross-shore flow velocity variations, indicating a negative correlation with water depth. Flow velocities are higher in the shallow nearshore areas and sandbars, while they decrease in the deeper main channel.
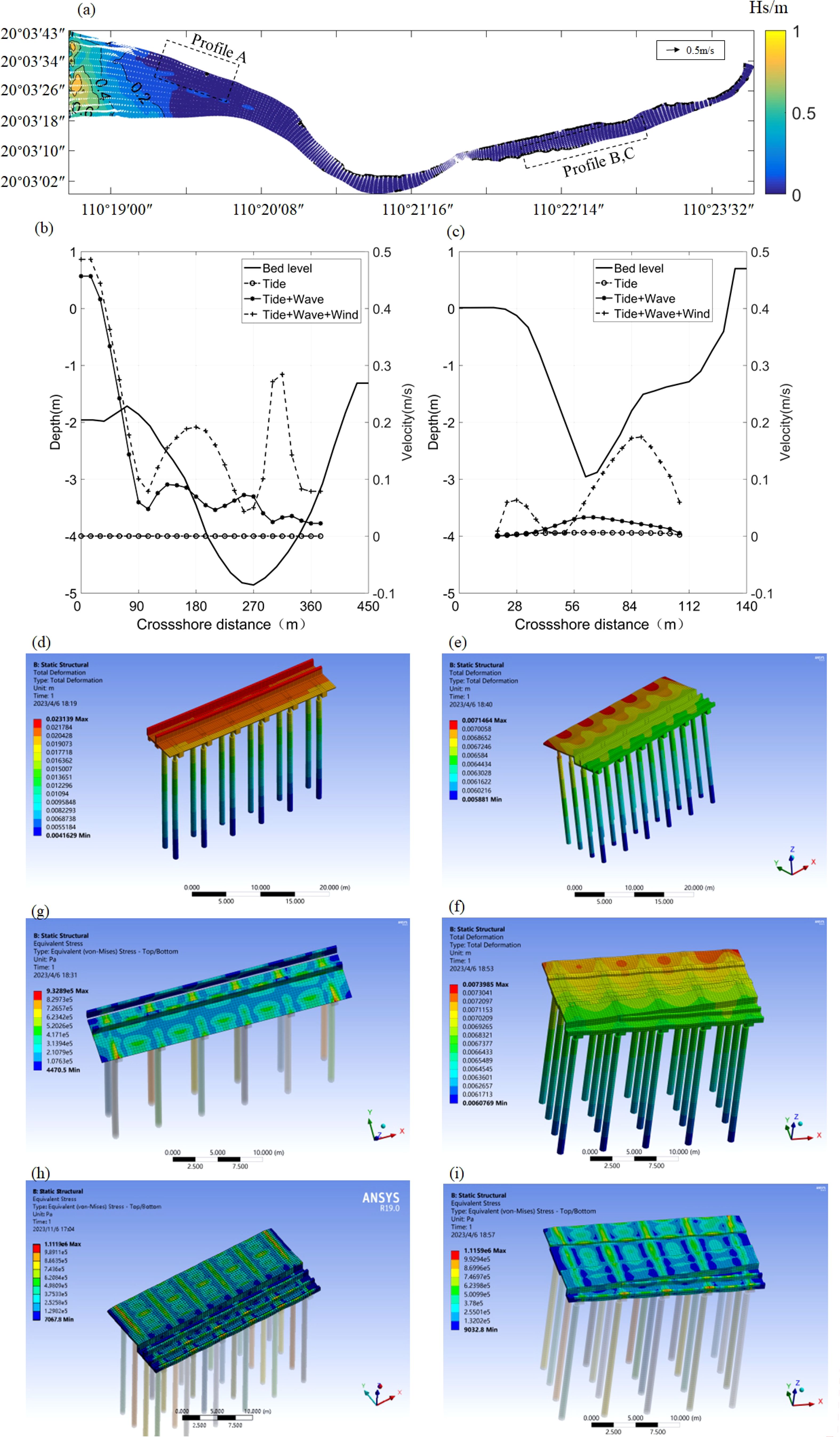
Figure 5. Cloud map of the total deformation and equivalent stress in each profile. (A) is wave height and velocity distribution in spring tide; (B, C) is the depth and cross-shore velocity distributions in the areas of profiles A, B, and C; (D–F) is deformation cloud map under structural action in profiles A, B, and C; (G–I) is stress cloud map under structural action in profiles A, B and C.
Outputs from XBeach—wave height, flow velocity, and water level—were used to drive the ANSYS model for the deformation and stress analysis. Profile A exhibited vertical displacement of approximately 20 mm, attributed to larger planting loads and fewer supporting piles. Profiles B and C showed vertical displacement of approximately 7 mm, due to more evenly distributed loads and additional support from piles. The displacement at the head of the pile is smaller in Profiles B and C but larger between the piles, mirroring the bending moment distribution seen in simply supported beams. The maximum moment in the span caused greater displacement in the panel than at the head of the pile. On the water-facing side, displacement is larger than on the land-facing side. In Profile C, uneven planting loads resulted in non-uniform displacement across the panel. Stress in the pile cap beams for all profiles was concentrated but remained below 100 kPa, posing minimal risk to structural settlement or stability. The bending moments, shear forces, axial forces, and displacements (Table 2) for each profile were all within permissible limits indicating that the structural design of the viaduct is uniformly stable. Deformations across all profiles were coordinated, trending towards long-term stability under sustained and stable upper loads.
During LWL, wave forces act directly on the cluster of piles. Profile A, with its wider water area, experiences more significant forces compared to Profiles B and C. However, under post-storm wave conditions, dynamic forces are relatively low, and the current-induced responses around the piles are minimal. When the water level exceeds the pile top elevation, wave uplift forces act on the panel, affecting the overall structural loading. Due to river bank restrictions, incoming waves cannot easily propagate into the narrow channel. Therefore, only Profile A located at the river mouth, is significantly affected by wave uplift forces. In shallow water, waves deform nearshore and exert positive pressure on onshore structures. The platform structure is independent of the pile foundations and unconnected to the existing revetment. When the HWL is designed at 1.51 m, with significant bottom clearance, wave peaks do not contact the panel, and wave uplift forces cannot be calculated. However, when water levels exceed the panel’s bottom, wave uplift forces must be accounted for. The maximum wave uplift force per unit length along the bottom of the panel was 18.08 kN/m, with a uniform pressure of 3.23 kPa. At the extreme HWL of 2.93 m, the bridge structure becomes submerged, requiring consideration of static buoyancy forces. When submerged at a water level of 2.93 m, static pressure on the underside of the panel at -0.43 m was computed to be 4.3 kPa (Figure 5).
4.2 Observation in the construction process
We monitored changes in coastal open spaces before and after the project’s implementation using high-resolution satellite remote-sensing images. In 2018, the Haidian River had a narrow, resilient space along city roads, with non-continuous waterfront walkways. Vacant plots, some occupied by buildings, disrupted the functionality of the space (Figure 6A).Many revetments were old and damaged, with boats docked along the coast detracting from the aesthetic appeal and hindering resilient space effectiveness. In Haikou Bay, existing waterfront walkways were poorly connected. Retaining walls appeared worn, and several roads lacked greenery, with damaged embankments in need of repair. In 2020, the width of resilient spaces increased with the construction of continuous waterfront walkways. Permeable bricks and materials were introduced to enhance water absorption. Wastelands and private buildings in the original waterfront spaces were demolished, creating opportunities for green belts and rest stations, which attracted more tourist stops. The construction revitalized the local economy by encouraging the establishment of commercial venues, including restaurants and bar terraces, turning them into popular leisure destinations. In Haikou Bay, the existing seawall underwent an ecological transformation with reinforced dike bases using stones. Concrete boxes were added to embankments to support new landscape plants, improving the shoreline’s visual appeal. The combination of landscape design and red permeable walkways enhanced the area’s attractiveness for residents and tourists alike. Small scenic areas on green land further boosted tourism and facilitated the growth of small-scale commodity economies. In 2023, the red lines in the images (Figure 6B) represent the now-continuous waterfront walkways along the shoreline. Significant increases in coastal green coverage were achieved, making the shoreline more visually appealing and functional. The project successfully enhanced the attractiveness of the waterfront, increased visitor numbers, and improved the area’s overall quality and image.
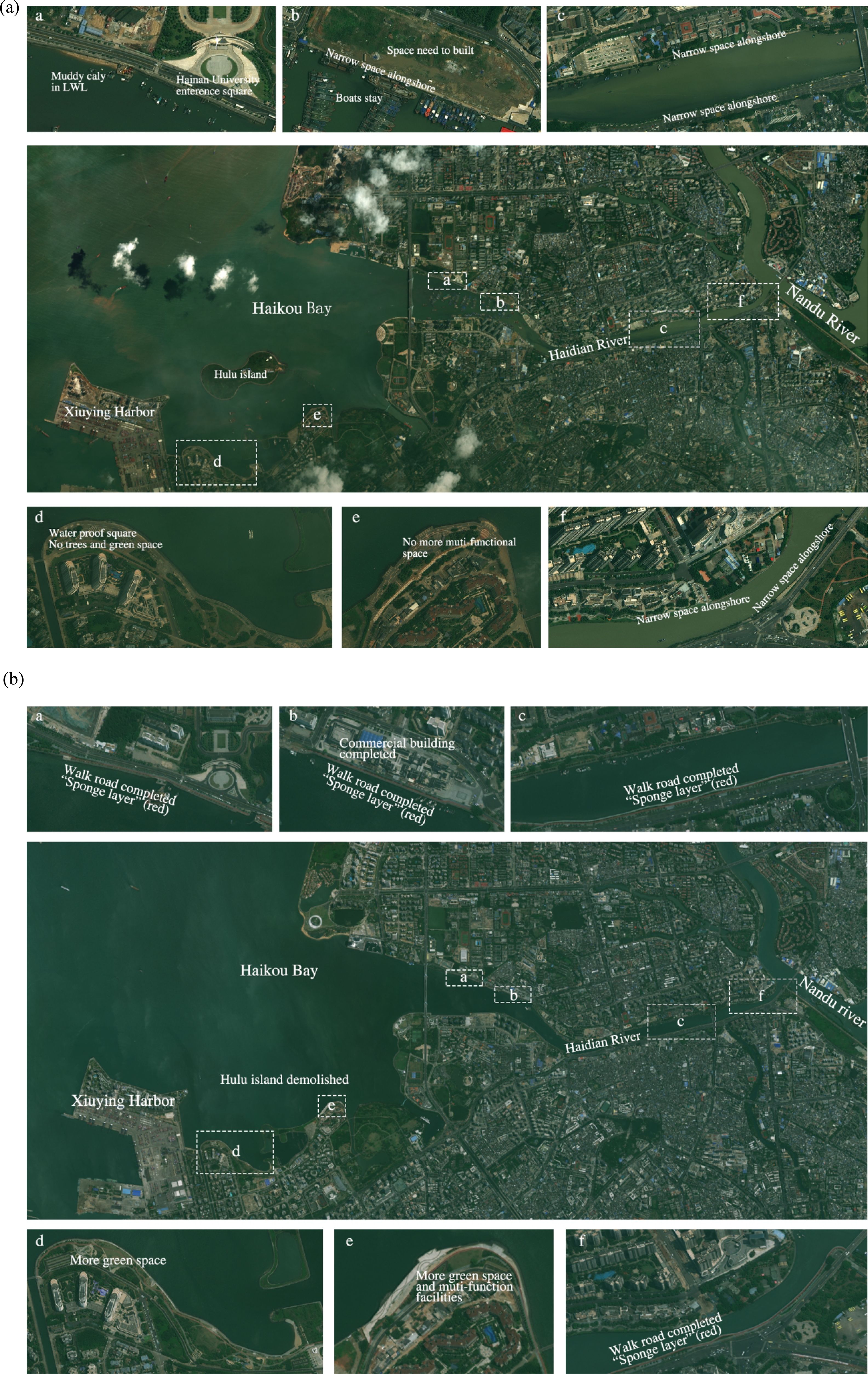
Figure 6. Remote sensing satellite imagery. (A) Before the HHCRP starts in 2018; (B) After the HHCRP was completed in 2023. Each subpanel shows larger view in (A) and (B) which marked in dotted box with its order.
4.3 Coastal communities involved after the project was completed
An inclusive coastal management system is important to incorporate the views, interests, and suggestions of all stakeholders to achieve a sustainable balance between urban development and the coastal environmental conservation. Environmental aspects, such as physical conditions, must be thoroughly studied first before proposing solutions to address erosion issues (Lucrezi et al., 2016; Saengsupavanich, 2012). These solutions should prioritize cost-efficiency and be implemented within optimized timeframes (Rangel-Buitrago et al., 2017). We distributed and collected 120 questionnaires from long-term residents of the area, spanning various professions and age groups. These individuals shared a deep familiarity with the region and its developments, having monitored the project from construction to completion. A consistency test was conducted on the statistical data obtained to ensure reliability and credibility. Table 3 lists the weights calculated for each element at the criterion and indicator levels. The weight coefficient for disaster prevention and mitigation was 0.417, the highest among all factors, followed by the coastal ecological factors at 0.263. This reflects aspects such as vegetation coverage, flowers, vegetation, and water environment quality, all of which directly improve the visual appeal of the coastal area. The focus on behavioral patterns and public facilities is relatively low. It is clear that the public is most concerned with the functional needs of the coastal resilience project, with less attention given to its usage needs. We refined the evaluation of the five aspects at the criterion level by constructing 20 indicators at the indicator level. Except for public facilities, there is a clearly noticeable factor of public concern under each criterion level. For example, wide space (0.54) and vegetation coverage (0.478) in biological factors, improved resilience function (0.54) in disaster prevention and mitigation factors, and fitness activities (0.467) in behavioral patterns. In public facilities, except for recreational facilities and accessibility (0.381), the weights of the other factors were similar. Among the 20 total indicators, the public was most concerned about the resilience function of urban coastal space (C9).
Of the involved public, 37% were most concerned about the disaster prevention and mitigation capabilities within the functional aspect of coastal resilience. This concern reflects the public’s interest in how the project improves Haikou City’s disaster prevention and mitigation capabilities, especially given the city’s annual storm-induced waterlogging. In total, 27% and 33% of the public focused on the ecological and spatial aspects, respectively. Less than 10% of the public considered public facilities and behavioral patterns the most important aspects of the coastal zone. We also analyzed the most important indicator-level factors for each criterion. Regarding space size, 57% of the public were most concerned with space width and area (C1) because it facilitated their activities, followed by traffic convenience (C2) and economic attractiveness (C3), at 39% and 12%, respectively. In biological factors, 64% were most concerned with green space coverage (C4), considering it a direct indicator of waterfront landscape quality, followed by 28% focusing on water quality improvement (C7), and 10% and 5% concerned with plant and animal species (C5) and local small environments (C6), respectively. In disaster prevention and mitigation, 98% of the public were most concerned with the resilience space’s defense capability against extreme disasters (C8) and waterfront safety (C9). In behavioral factors, 70% of the public focused on the connectivity of the roads along the shore (C11), followed by coastal consumption (C13) at 22%. In terms of public facilities, 62% were most concerned with whether recreational facilities were complete (C15). The remaining indicators had similar proportions.
We also had participants score each element of the indicator layer, using quantitative methods to process qualitative indicators so that they could be compared and analyzed under a unified standard. The scores were divided into five levels (1–10 scale), with qualitative evaluation indicators rated based on people’s most intuitive perceptions (Figure 7). Approximately 50% of the public involved were very satisfied with the 20 indicators we proposed (scores of 9–10), believing that the HHCRP had greatly improved the coastal environment and provided recreational areas for citizens. They had a positive attitude towards the HHCRP. Approximately 30% of respondents were relatively satisfied (scores of 7–8), noting some shortcomings in the HHCRP. Approximately 30% rated it below 6, considering it average or in need of improvement. The effect of “the three ways unblocked” received the highest rating, with an average score of 8.33, while the variety of plant and animal species received the lowest rating, with an average score of 7.77. Overall, the average scores for all indices were above seven, indicating relative satisfaction among the public. Respondents believed that the HHCRP had stimulated some commercial and waterfront consumption, but that this was not the primary function of the waterfront. Commercial establishments cannot be continuously distributed along the entire waterfront; restaurants or bars are typically located at key points. Respondents also felt that reception services, tour guide facilities, and sanitation arrangements needed further improvement.
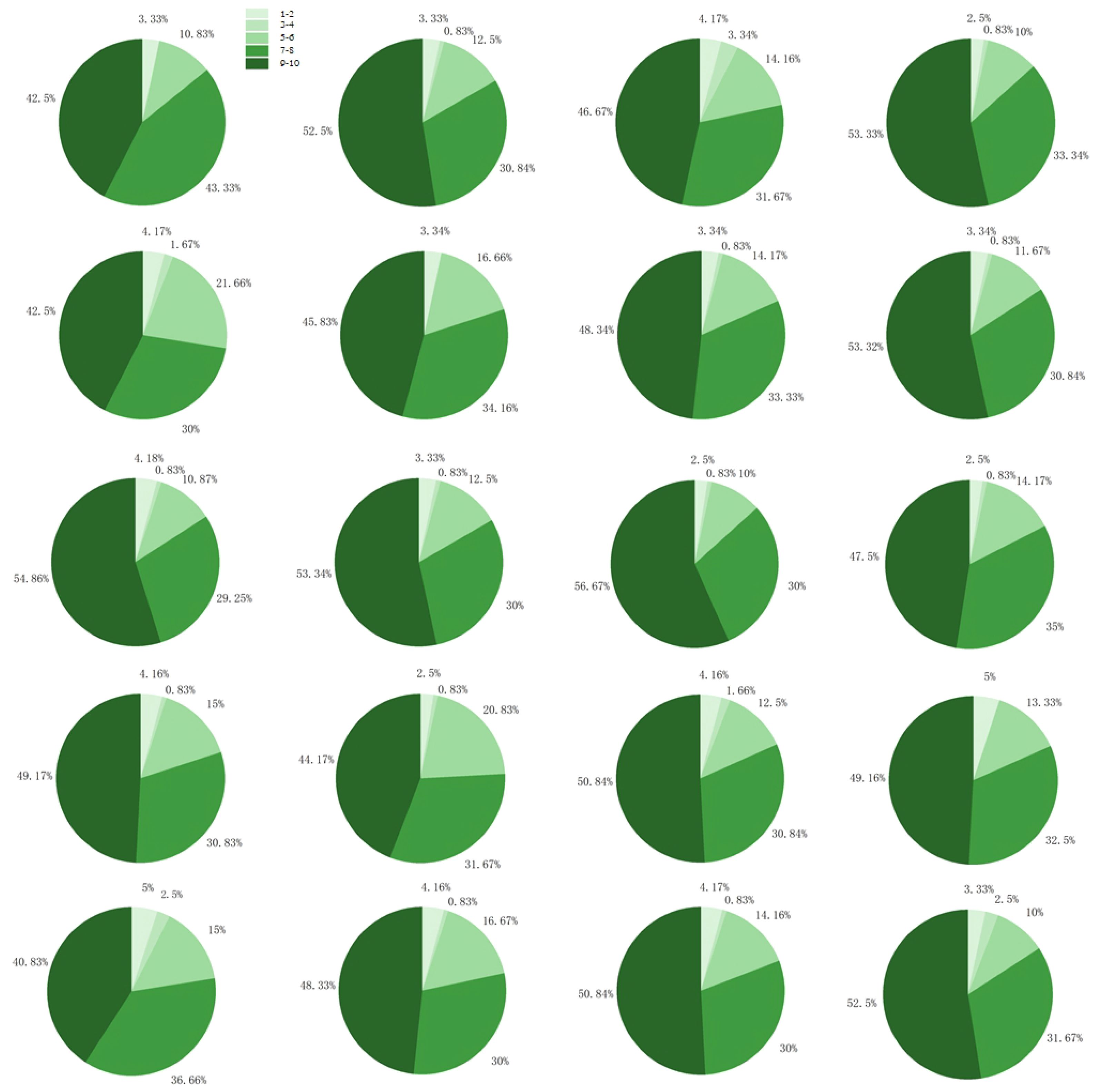
Figure 7. Score statistics from questionnaires. A score of 9-10 means very satisfied, 7-8 means relatively satisfied, 5-6 means average, 3-4 means relatively unsatisfied, and 1-2 very unsatisfied.
4.4 Whole process management
We developed a comprehensive process management system and successfully applied it to the HHCRP (Figure 8). This system integrates several key stages: status analysis, layout planning, design scheme, construction process tracking, and completion evaluation. Each stage focuses on different aspects, which are highly interrelated. In the status analysis phase, it is essential to understand the specific geographical characteristics of the study area and dynamic environmental parameters such as wave heights, tidal levels, currents, and wind conditions. In addition, water depth measurements, bedload investigations, and assessments of the current state of embankment structures are critical. The analysis should evaluate whether visibly intact structures are still viable or if damaged retaining walls require repair. During the layout planning stage, a tailored plan for the study area was developed within the broader context of regional planning. This plan had to address both basic infrastructural needs and functional improvements. A thorough analysis of existing problems was necessary to evaluate the potential impact of implementing the proposed plans in various regions. In the design scheme stage, we first conducted case studies of similar projects to identify recurring patterns and key considerations. From these studies, we derived critical design principles such as environmental sustainability, resilience, multifunctionality, and ensuring unobstructed transportation routes. These insights formed the basis for the project’s design concept, which subsequently informed spatial planning and layout. Urban coastal resilience engineering is a multidisciplinary, systems-oriented project that incorporates structural engineering, landscaping, architecture, bridge design, electrical systems, and other infrastructure. Once the design scheme was finalized, numerical models were employed to simulate the impact of construction on regional hydrodynamics and sediment transport. For construction process tracking, we utilized a variety of advanced monitoring techniques, such as high-precision remote sensing, unmanned aerial vehicles (UAVs), and field surveys. A strong emphasis was placed on process management to ensure that any emerging issues were addressed promptly and effectively. Finally, in the completion evaluation phase, we assessed the success of the project through a satisfaction survey based on AHP methodology. This provided a structured framework for evaluating the effectiveness of the resilience measures implemented. Continuous post-completion evaluations are crucial for monitoring the long-term benefits and impacts of the coastal resilience project.
5 Discussion: importance of elevation planning and design
A comprehensive consideration of elevation is critical for urban coastal zone planning (Gao et al., 2022). However, there is an upper threshold for ground elevations along river mouth coasts, which is closely related to factors such as soil funding, groundwater levels, and sea-level rise. Excessively raising ground elevations is not only uneconomical but also risks accelerating subsidence. Therefore, when engineering measures to enhance coastal resilience, costs and benefits must be balanced to ensure the safety of lives and property in coastal areas while maintaining post-construction terrain stability. Urban subsidence also necessitates consideration of levee elevation from another perspective. Ao (2024) predicted coastal urban land subsidence by 2120, indicating that over 22% of coastal cities in China may be lower than the relative sea levels, affecting more than 9% of the population. Coastal cities also face additional risks from sea-level rise, combined urban subsidence and sea-level rise can lead to exacerbated flooding, posing a serious threat to coastal populations. This further underscores the importance of ensuring flood protection elevations for frontline levees along the coast.
Analyzing the impact of extreme events, such as storm waves and surges, is crucial for evaluating the functional effectiveness of resilient engineering. Storm No.2118 “Kompasu” on 13 October 2021, reached a level 12 wind speed impact on the HHCRP area (Figure 9A). The wave height ranged from 3 to 5 m in the nearshore HHCRP. Storm surges of 0.5 to 1.2 m occurred along the HHCRP coastline from the 13th to 14th October, exceeding local alert tide levels. Owing to the lower top elevation of the first-level levee’s waterfront platform along the Haikou Bay coast (western part of the HHCRP), the waves climbed over the levee top during the high-water periods, severely damaging the green belts within the resilient space behind it, mainly causing land erosion and tree collapse (Figure 9B). However, the existence of resilient spaces prevented the further expansion of the affected areas. During the post-storm restoration period, reinforcement and repair works were carried out to strengthen the resilient spaces. The platform along the Haidian River tidal channel (eastern part of the HHCRP), due to its higher design elevation, remained unaffected by the HWL and wave overtopping, causing minimal damage to the terrestrial resilient space (Figure 9C). This illustrates the importance of elevation in the design of coastal resilient spaces, emphasizing the need to avoid setting elevations that are too low for the sake of proximity to water surfaces, especially in river mouth coastal areas where protective measures should be implemented to raise the elevation of first-level levees.
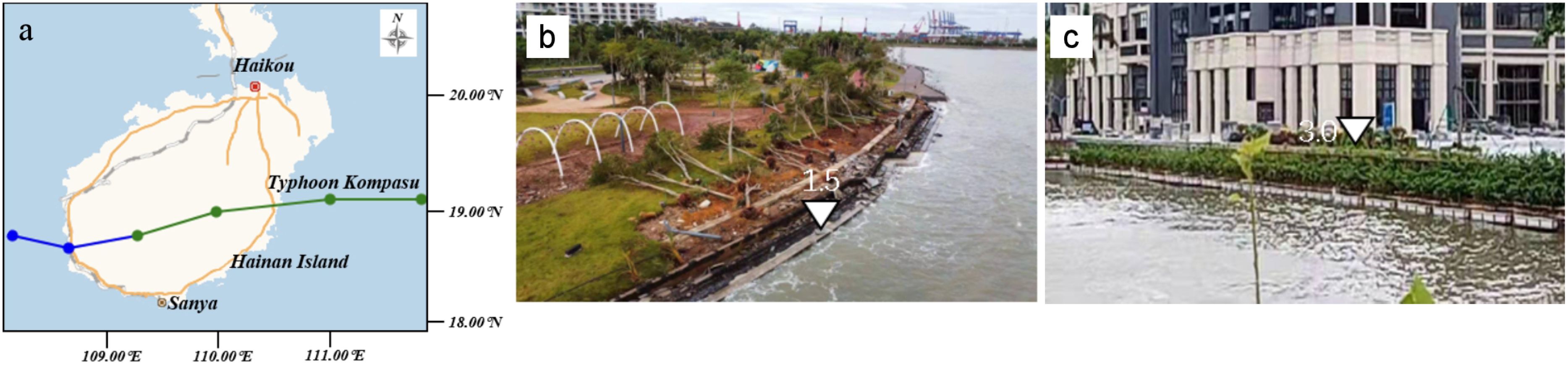
Figure 9. Resilience was effective during storm No.2118 “Kompasu”. (A) The typhoon path; (B) seawall facing Haikou Bay (western part of the HHCRP); (C) platform out of the embankment facing Haidian River (eastern part of the HHCRP).
6 Conclusion
The HHCRP was a coastal resilience project designed for urban coastlines. It involved the planning and design of a 19.6 km coastline with a 3.84 km² planning area, which extended narrow land areas through high-pile platforms. This improved the spatial, ecological, and disaster-reduction capabilities of the coastal area, revitalized the coastal landscape, and enhanced the resilience capacity of the coastal zone. Based on the HHCRP, an entire process management system was established. This article also discussed the role of elevation planning and design in coastal engineering. The main conclusion was that reasonable planning and design were essential prerequisites for coastal resilience projects.
1. A dynamic-structure interaction model was developed to simulate the displacement, bending moment, and shear force of the pile foundation under different water levels. It was shown that the design scheme was reasonable, with no local deformation or excessive forces, and the structure met the durability design requirements.
2. The implementation of the HHCRP successfully removed the buildings occupying the space, making the coastal area wider and unobstructed. The increased vegetation cover was visible in the green areas, and the red water-absorbing walkways improved the permeability of the waterfront space. The surrounding open space was also developed, with commercial buildings constructed owing to the coastal zone construction. Remote sensing confirmed that the completed project significantly improved the coastal disaster defense capabilities and landscape attractiveness.
3. Based on AHP, it was found that the public was most concerned about enhancing urban disaster-reduction capabilities, hoping that resilient spaces could mitigate inland damage and flooding from storms, which are significant issues for coastal cities such as Haikou.
4. A suitable evaluation system helped us to understand both the successes and areas for improvement, which can guide similar projects. The contributions to the entire process management system, including status analysis, layout planning, design scheme, construction process tracking, and completion evaluation, were integral to the coastal resilience engineering process in the HHCRP.
Coastal resilience projects address systemic problems. This study used the HHCRP as an example to provide a comprehensive planning and design evaluation method. However, these projects are complex and require extensive promotion and public education. Timely evaluations are essential, as policies drive project implementation, but public feedback shapes policy directions. Resident participation is key, and while various concerns were addressed, a comprehensive evaluation requires more than public feedback. Objective indices, such as per capita water usage, GDP energy consumption, and green coverage rates, are necessary for further evaluation. Other indices, including economic development levels or social development in the criteria layer, are also required. While collecting such data is challenging, a robust coastal resilience evaluation system must be established.
Data availability statement
The raw data supporting the conclusions of this article will be made available by the authors, without undue reservation.
Author contributions
YTZ: Conceptualization, Formal Analysis, Methodology, Software, Writing – original draft. CHJ: Writing – review & editing. YSJ: Writing – original draft, Data curation, Formal analysis. YZ: Investigation, Writing – review & editing. YJ: Software, Writing – original draft. XW: Writing – review & editing, Project administration. XF: Writing – review & editing, Supervision. WBF: Writing – review & editing, Supervision.
Funding
The author(s) declare financial support was received for the research, authorship, and/or publication of this article. This work was financially supported by funding from the Hainan Key Laboratory of Marine Geological Resources and Environment (22-HNHYDZZYHJKF028); funding from the Key Laboratory of Marine Ecological Conservation and Restoration, Ministry of Natural Resources/Fujian Provincial Key Laboratory of Marine Ecological Conservation and Restoration (EPR2023009); and funding from the Key Laboratory of Ministry of Education for Coastal Disaster and Protection, Hohai University (J202405).
Conflict of interest
Authors XW and YTZ were employed by the company Shanghai Urban Construction Design and Research Institute Group Co., Ltd.
The remaining authors declare that the research was conducted in the absence of any commercial or financial relationships that could be construed as a potential conflict of interest.
Generative AI statement
The author(s) declare that no Generative AI was used in the creation of this manuscript.
Publisher’s note
All claims expressed in this article are solely those of the authors and do not necessarily represent those of their affiliated organizations, or those of the publisher, the editors and the reviewers. Any product that may be evaluated in this article, or claim that may be made by its manufacturer, is not guaranteed or endorsed by the publisher.
References
Adger W. N., Hughes T. P., Folke C., SR C., Rockstorm J. (2005). Social-ecological resilience to coastal disasters. Science 309, 1036–1039. doi: 10.1126/science.1112122
Ao Z., Hu X., Tao S., Hu X., Wang G., Li M., et al. (2024). A national-scale assessment of land subsidence in China’s major cities. Science 384, 301–306. doi: 10.1126/science.adl4366
Bayle P. M., Blenkinsopp C. E., Martins K., Kaminsky G., Weiner H. M., Cottrell D. (2023). Swash-by-swash morphology change on a dynamic cobble berm revetment: High-resolution cross-shore measurements. Coast. Eng. 104341. doi: 10.1016/j.coastaleng.2023.104341
Blenkinsopp C. E., Turner I. L., Masselink G., Russell P. E. (2011). Swash zone sediment fluxes: field observations. Coast. Eng. 58, 28–44. doi: 10.1016/j.coastaleng.2010.08.002
Buanes A., Jentoft S., Karlsen G. R., Maurstad A., Soreng S. (2004). In whose interest? An exploratory analysis of stakeholders in Norwegian coastal zone planning. Ocean Coast. Manage. 47, 207–223. doi: 10.1016/j.ocecoaman.2004.04.006
China’s National Bureau of Statistics (2023a). CHINA statistical yearbook (China’s National Bureau of Statistics). Available at: http://www.stats.gov.cn/sj/ndsj/ (Accessed October 16, 2023).
China’s National Bureau of Statistics (2023b). National data. Available online at: https://data.stats.gov.cn/easyquery.htm?cn-C01 (Accessed October 16, 2023).
Dasgupta P., Serageldin I. (2000). Social Capital: a Multifaceted Perspective (Washington: The world bank). Available online at: http://documents.worldbank.org/curated/en/422191468315292516/Social-capital-a-multifaceted-perspective.
Elsayed S. M., Oumeraci H. (2017). Effect of beach slope and grain-stabilization on coastal sediment transport: An attempt to overcome the erosion overestimation by XBeach. Coast. Eng. 121, 179–196. doi: 10.1016/j.coastaleng.2016.12.009
Feagin R. A., Innocenti R. A., Bond H., Wengrove M., Huff T., Lomonaco P., et al. (2023). Does vegetation accelerate coastal dune erosion during extreme events? Sci. Adv. 9, eadg7135. doi: 10.1126/sciadv.adg7135
Gao G., Beardall J., Jin P., Gao L., Xie J., Gao K. (2022). A review of existing and potential blue carbon contributions to climate change mitigation in the Anthropocene. J. Appl. Ecol. 59, 1686–1699. doi: 10.1111/1365-2664.14173
Holling C. S., et al. (2004). Regime shifts,resilience, and biodiversity in ecosystem management. Annual Review of Ecology, Evolution, and Systematics. 35, 557–81.
Kiran K. S., Bindu C. A. (2022). Resilience master plan as the pathway to actualize sustainable development goals–A case of Kozhikode, Kerala, India. Prog. Disaster Sci. 14, 100226. doi: 10.1016/j.pdisas.2022.100226
Koster L. (2006). Humplike nourishing of the shoreface. A study on more efficient nourishing of the shoreface. Available online at: https://resolver.tudelft.nl/uuid:e354c6da-99bb-4419-8419-31654896027f.
Li Y., Zhang C., Chen S., et al. (2024). Experimental Investigation on Cross-Shore Profile Evolution of Reef-Fronted Beach[J]. Coastal Engineering 2024, 104653.
Li Y., Zhang C., Song J., Chi S., Zhao S., Qi H., et al. (2022). Tide-modulated wave characteristics and breaking regimes in the intertidal zone of a dissipative beach. Ocean Eng. 266, 113055. doi: 10.1016/j.oceaneng.2022.113055
Li Y., Zhang C., Zhao S., Qi H., Cai F., Zheng J. (2024). Equilibrium configurations of sandy-muddy transitional beaches on South China coasts: Role of waves in formation of sand-mud transition boundary. Coast. Eng. 187, 104401. doi: 10.1016/j.coastaleng.2023.104401
Lucrezi S., Saayman M., van der Merwe P. (2016). An assessment tool for sandy beaches: A case study for integrating beach description, human dimension, and economic factors to identify priority management issues. Ocean Coast. Manage. 121, 1–22. doi: 10.1016/j.ocecoaman.2015.12.003
Malvarez G., Ferreira O., Navas F., Cooper J. A. G., Talavera L. (2021). Storm impacts on a coupled human-natural coastal system: Resilience of developed coasts. Sci. Total Environ. 768, 144987. doi: 10.1016/j.scitotenv.2021.144987
Martínez M. L., Intralawan A., Vázquez G., Pérez-Maqueo O., Sutton P., Landgrave R. (2007). The coasts of our world: Ecological, economic and social importance. Ecol. Economics 63, 254–272. doi: 10.1016/j.ecolecon.2006.10.022
Mitchell R. K., Agle B. R., Wood D. J. (1997). Toward a theory of stakeholder identification and salience: Defining the principle of who and what really counts. Acad. Manage. Rev. 22, 853–886. doi: 10.2307/259247
Mushove P., Vogel C. (2005). Heads or tails? Stakeholder analysis as a tool for conservation area management. Global Environ. Change 15, 184–198. doi: 10.1016/j.gloenvcha.2004.12.008
Navas F., Carrero R., Cáceres F. (2012). A new approach to Future Scenarios for the EU COASTANCE Project. Ministry Environ. Regional Government Andalusia Tech. Rep., 119.
Neumann B., Vafeidis A. T., Zimmermann J., Nicholls R. J. (2015). Future coastal population growth and exposure to sea-level rise and coastal flooding-a global assessment. PloS One 10, e0118571. doi: 10.1371/journal.pone.0118571
Prasad N., Ranghieri F., Shah F., Trohanis Z., Kessler E., Sinha R. (2009). Climate resilient cities: A primer on reducing vulnerabilities to disasters (Washington, D. C.: World Bank Publications). Available online at: http://documents.worldbank.org/curated/en/164671468026943315/Climate-resilient-cities-a-primer-on-reducing-vulnerabilities-to-disasters.
Rangel-Buitrago N., Williams A. T., Anfuso G. (2017). Hard protection structures as a principal coastal erosion management strategy along the Caribbean coast of Colombia. A chronicle of pitfalls. Ocean Coast. Manage. 156, 58–75. doi: 10.1016/j.ocecoaman.2017.04.006
Saaty T. (1980). The analytic hierarchy process : planning, priority setting, resource allocation Vol. 1 (Kobe, Japan), 69.
Saengsupavanich C., Chonwattana S., Naimsampao T. (2009). Coastal erosion through integrated management: A case of Southern Thailand. Ocean Coast. Manage. 52, 307–316. doi: 10.1016/j.ocecoaman.2009.03.005
Saengsupavanich C. (2012). Unwelcome environmental impact assessment for coastal protection along a 7-km shoreline in Southern Thailand. Ocean Coast. Manag. 61, 20–29. doi: 10.1016/j.ocecoaman.2012.02.008
Wang G., Zhang K. (2024). Turning peril into opportunity: Construction and validation of a resilience assessment system for China's coastal megacities. Sustain. Cities Soc. 112, 105606. doi: 10.1016/j.scs.2024.105606
Zhang J., Jin J. (2023). Discussion on several issues concerning the construction of national water network[J]. Water Resources Development Research 23, 1–7.
Keywords: coastal resilience, coastal engineering, management, planning, design, construction, assessment, marine structure
Citation: Zhou Y, Jiang C, Jiang Y, Zhu Y, Jin Y, Wang X, Feng X and Feng W (2025) A whole process resilience management practice in coastal engineering. Front. Mar. Sci. 11:1518249. doi: 10.3389/fmars.2024.1518249
Received: 28 October 2024; Accepted: 16 December 2024;
Published: 23 January 2025.
Edited by:
Juan Jose Munoz-Perez, University of Cádiz, SpainReviewed by:
Valeria Chávez, National Autonomous University of Mexico, MexicoDavide Pasquali, University of L’Aquila, Italy
Copyright © 2025 Zhou, Jiang, Jiang, Zhu, Jin, Wang, Feng and Feng. This is an open-access article distributed under the terms of the Creative Commons Attribution License (CC BY). The use, distribution or reproduction in other forums is permitted, provided the original author(s) and the copyright owner(s) are credited and that the original publication in this journal is cited, in accordance with accepted academic practice. No use, distribution or reproduction is permitted which does not comply with these terms.
*Correspondence: Yu Zhu, emh1eXVfaHl5QGhodS5lZHUuY24=