- 1College of Life and Environmental Sciences, Wenzhou University, Wenzhou, Zhejiang, China
- 2National and Local Joint Engineering Research Center of Ecological Treatment Technology for Urban Water Pollution, Wenzhou University, Wenzhou, China
Cadmium (Cd) is a toxic heavy metal that, when present as a pollutant in the marine environment, is readily accumulated by marine bivalves, causing oxidative stress and tissue damage. This study explored whether short-term depuration could reverse Cd2+-induced toxicity in the ovary of the clam Meretrix meretrix. Clams exposed to 3 mg·L-1 Cd2+ for three days showed increased accumulated Cd2+ in their ovaries with obvious tissue damage as shown by loose structure and some apoptotic cells compared with non-exposed clams. Increased oxidative stress in the ovarian tissue was also obvious, as revealed by increased levels of oxidative indicators such as reactive oxygen species (ROS), malondialdehyde (MDA), DNA-protein crosslinking (DPC), and protein carbonylation (PCO) and increased expression levels of genes related to oxidative stress and apoptosis, which included the Bax, Bcl-2, caspase-3, HO-1, Hsp70, NQO1, Nrf2, and MT genes. When the clams were exposed to Cd2+ for three more days, the accumulated Cd2+ level in the ovary increased to more than 10-fold the level in the control clams, accompanied by more severe damage and cell death as well as oxidative stress. However, when the initial three-day Cd2+ exposure was followed by three days of depuration in Cd2+-free seawater, the Cd2+ level in the ovary was reduced by as much as 20%, accompanied by some recovery of tissue damage and reduced oxidative stress, suggesting that short-term depuration may mitigate Cd2+-induced toxicity in M. meretrix, allowing the clams to recover and potentially reducing the risk of Cd2+ exposure from consuming contaminated clams.
Highlights
● Cadmium (Cd) is a toxic metal found as a pollutant in the marine environment.
● Cd-induced oxidative damage in Meretrix meretrix ovary was assessed after exposure.
● Cd exposure led to increased oxidative stress and tissue damage in the ovary.
● Short-term depuration reduced the extent of oxidative stress and tissue damage.
● Cd-induced oxidative damage could be partly reversed by depuration.
1 Introduction
Pollution of the marine environment caused by toxic heavy metals has become a significant factor in the continuous deterioration of the environment, especially in the water that lies near the coastal region with heavy industrial activities (Luo et al., 2021; Lakshmanna et al., 2022; Patchaiyappan et al., 2023). These metals can accumulate in the tissues of marine animals, causing damage to cellular components such as cell membranes and nuclei, mitochondria, enzymes, and proteins, eventually leading to oxidative stress and cell death (Bartlett et al., 2020; Chen et al., 2020; Gao et al., 2021a, 2021b; Bai et al., 2022). Thus, rising levels of toxic heavy metals in the marine environment can become a perpetuating abiotic factor that affects the well-being of marine animals as well as human health through the food chain.
Marine animals, in particular shellfish such as clams, oysters, and mussels, which collectively make up the bivalves, are especially vulnerable to the effects of toxic heavy metals because of their filter-feeding lifestyle. Bivalves are of particular importance to the research on the toxic effects caused by heavy metals on marine organisms because, in addition to their filter-feeding lifestyle, they are basically sedentary, and they are widely distributed in the water ecosystem. Bivalves are also consumed by humans on a large scale. Toxic heavy metals that are found in the marine environment as pollutants include zinc, lead, cadmium, copper, selenium, and mercury, and their levels have risen substantially in the past few decades (Bandara and Manage, 2023). Among these, cadmium (Cd) has received much attention as a toxic metal of significance with respect to its toxicological effects in marine bivalves (Zhan et al., 2023).
Cadmium (Cd) is highly toxic to animals (Genchi et al., 2020), and it is commonly found in aquatic environments as a result of runoff from industrial wastes (Qian et al., 2015; Lakshmanna et al., 2022; Yunus, 2020; Bandara and Manage, 2023). Once inside the body of an animal, Cd can interact with proteins and DNA to interfere with their proper functioning, resulting in the inhibition of enzyme activities and DNA repair (Candéias et al., 2010; Whiteside et al., 2010; Wu et al., 2011; Li et al., 2015). Cd also causes lipid peroxidation and induces the production of reactive oxygen species, all of which contribute to elevated oxidative stress levels (Géret et al., 2002; Escorcia and Chang, 2010; Chahouri et al., 2023). Research on the toxicological effects of Cd on marine bivalves has gathered momentum in the past few decades, and these effects have been demonstrated in different species and by different investigators. A thorough assessment of the results gathered from relevant past studies has been presented in a recent meta-analysis (Zhan et al., 2023). Recent studies employing proteomic, metabolomic, or transcriptomic approaches suggest that Cd may also exert its toxicity in bivalves via disruption of bivalve cytoskeleton structure, ion homeostasis, energy metabolism, apoptosis, and immune response (Liu et al., 2023; Zhou et al., 2023; Xu et al., 2024).
Among the bivalves in which the effect of Cd has been of particular concern is the clam Meretrix meretrix. In China, M. meretrix is an economically important bivalve that is cultivated for food in different coastal regions. The water in some of the sites where M. meretrix aquaculture farms are located is also under the threat of heavy metal pollution caused by nearby human activities (Yang et al., 2023). We and others have previously shown that Cd can induce oxidative stress in M. meretrix tissues such as the gill and ovary, and such an effect is manifested as increased levels of malate dehydrogenase and cytochrome oxidase activities as well as elevated activity levels of antioxidant enzymes, e.g., catalase and SOD, all of which are direct responses to increased levels of oxidative damage and energy allocation and consumption (Wang et al., 2021; Bai et al., 2022; Sun et al., 2024; Xu et al., 2024). In addition, Cd also induces mitochondria-mediated apoptosis of gill cells and oval cells (Wang et al., 2021; Bai et al., 2022). Similar findings also extend to the effects of Cd on other bivalves, such as Tegillarca granosa (Yang et al., 2023), Sinonovacula constricta (Chen et al., 2020), and Crassostrea gigas (Perića et al., 2020). If the metal accumulated in the animal tissues can be released through a change in the water environment, then this will alleviate the extent of the metal-induced toxicity in the exposed animals. The bioaccumulation of Cd in bivalves followed by its elimination during depuration has been carried out for a number of species, such as the clams Ruditapes decussatus and Ruditapes philippinarum (Freitas et al., 2012) and the freshwater mussels Anodonta woodiana (Jing et al., 2019). These studies have shown that Cd toxicity is partly reversible, but the elimination of the accumulated metal is rather limited despite a long period (up to several weeks) of depuration. The extent of toxicity mitigation contributed by depuration has not been examined in greater detail.
To investigate how short-term exposure to a high concentration of Cd2+ followed by short-term depuration might affect the toxicity of Cd2+ in M. meretrix, M. meretrix individuals were exposed to water containing a high concentration of Cd2+ (3 mg·L-1) for three days and then subjected to depuration for three days to determine the changes in the state of Cd2+-induced oxidative stress in the ovary. Various standard oxidative stress indicators were assessed to determine the extent of reversal of toxicity. In addition to these oxidative stress indicators, the expression of various genes related to oxidative stress and inflammation, or apoptosis, was also measured to provide further insight into the effect of depuration on detoxification at the gene level. The result showed that M. meretrix could eliminate some of the Cd2+ absorbed to produce substantial relief in the toxicity induced by Cd2+. The result also suggested that depuration might play a more important role in restoring the physiological status of the exposed clams rather than reducing the risk of Cd2+ ingestion linked to consumption of the clams raised in Cd2+-contaminated water.
2 Materials and methods
2.1 Clam collection and treatment
The clams (Meretrix meretrix) were purchased in July 2022 from Wengyang Aquafarm in Wenzhou, Zhejiang, China. The clams had an average shell length, shell width, and shell height of 47.04 ± 0.52 mm, 23.78 ± 0.32 mm, and 38.74 ± 0.44 mm, respectively, with an average weight of 30.35 ± 0.82 g. The clams were kept in 15‰ artificial seawater (pH 7.9) inside an aquarium (67 × 46 × 36.5 cm) at 22 ± 1°C for two days without food as an acclimation period. After acclimation, the clams were randomly divided into three groups: the control group and two Cd2+-treated groups, with each group consisting of three tanks with 40 clams per tank. For the control group, the clams were just kept in 15‰ artificial seawater only. As for the two Cd2+-treated groups, in one group, the clams were kept in 15‰ artificial seawater containing 3 mg·L-1 Cd2+ for up to 6 days (Cd-1 group), while in the second group, the clams were kept in 15‰ artificial seawater containing 3 mg·L-1 Cd2+ for 3 days, and then in just 15‰ artificial seawater for another three days (Cd-2 group). For each group, the water in the tank was replaced every day with fresh water without Cd2+ or with 3 mg·L-1 Cd2+ as required. The concentration of Cd2+ in the water of each tank and the ovaries of the clams were measured every 24 h during the treatment period. At the end of days 1, 3, and 6, the ovaries were extracted from the clams of each group and subjected to each of the experiments described below.
2.2 Measurement of Cd2+ concentration
Cadmium concentrations in the water and M. meretrix ovary were measured daily using an atomic absorption method as previously described (Chen et al., 2020). To measure the Cd level in the M. meretrix ovary, two clams were randomly taken from each tank after each day of exposure, and their ovaries were extracted and subjected to Cd content analysis. The accumulation and release of Cd2+ by M. meretrix ovary were also evaluated by calculating the bioaccumulation factor (BCF) and elimination rate (ER), respectively. The formulae used to calculate BCF and the elimination rate for Cd2+ were essentially as described in Jing et al. (2019) and are as follows:
where Cexposure and Ccontrol are the concentrations of Cd2+ in the ovary of the Cd2+-exposed group and control group, respectively, whereas Cwater is the concentration of Cd2+ in the water.
where Celimination refers to the concentration of Cd2+ in the ovary after 3 days elimination period, while Cexposureis the concentration of Cd2+ before elimination. The elimination rate has a negative value, and a smaller negative value indicates a higher elimination ability.
2.3 Histological and Tunel assays of ovary specimen
For histological analysis and Tunel assay, two clams were taken from each tank on days 1, 3, and 6, and their ovaries were extracted. One part of each ovary was used for morphological analysis, which was carried out via hematoxylin-eosin (H&E) staining performed according to the method described by Bai et al. (2022), while the other part was used for the Tunel assay. In brief, the ovaries were fixed in 4% (v/v) paraformaldehyde overnight, embedded in paraffin, and sectioned to yield 6-µm-thick slices. The slices were stained with hematoxylin-eosin (HE) and observed with a light microscope (Olympus BX51, Japan).
For the Tunel assay, the paraffin-fixed slices were dewaxed by immersing in xylene twice, with each lasting for 10 min. The slices were subsequently immersed in 100%, 95%, 90%, 80%, and 70% ethanol, with each immersion lasting for 2 min. The slices were then washed three times in PBS, with 5 min per wash, and then incubated with protease K working solution at 20-37°C for 15-30 min. The protease K working solution was prepared by adding 1 μL of 100 × protease K to 99 μL of PBS. Following incubation with protease K, the sample was rinsed in PBS three times, with each washing lasting for 5 min. A color reaction reagent was prepared by adding 1 part of a 5× equilibrium solution to 4 parts of deionized water, and 100 μL of this solution was added to each slice and incubated at room temperature for 30 min. The excess solution was then removed, and 50 μL of TdT (terminal deoxynucleotidyl transferase) enzyme working solution was added to each sample placed on a microscopic slide and then covered with a glass slide and incubated in the dark at 37°C for 60 min. The sample was then rinsed with PBS three times for 5 minutes each. The excess PBS was drained with filter paper, and DAPI was added dropwise, followed by incubation in the dark for 5 min to stain the sample. After that, the sample was rinsed four times in PBS, with each rinse lasting 5 min. The excess liquid was again drained with filter paper, and the film was sealed with a sealing solution containing an anti-fluorescence quencher. Finally, the sample was observed under a fluorescence microscope (Leica DFC7000L, Germany) with an excitation wavelength set at 450-500 nm and an emission wavelength set at 515-565 nm for the detection of green fluorescence.
2.4 Assays of oxidative stress indicators
To measure the levels of different oxidative stress indicators, three clams were randomly taken from each tank on days 1, 3, and 6, and their ovaries were extracted, and each ovary was homogenized in ice-cold physiological saline (weight to volume ratio of 1 g to 9 mL) inside a tissue grinder (SCIENTZ-48, China). The resulting homogenate was centrifuged at 12000 × g/4 °C for 10 min, and the supernatant was used to determine the levels of 8-hydroxy-2’-deoxyguanosine (8-OHdG), malondialdehyde (MDA), protein carbonylation (PCO), and glutathione (GSH) as described below. For reactive oxygen species (ROS) and DNA-protein crossing-linking (DPC) assays, the ovary was treated differently as described in the relevant sections below.
2.4.1 8-Hydroxy-2’-deoxyguanosine assay
The level of 8-OHdG in the ovary was measured using an anti-8-OHdG antibody. The assay was carried out using an 8-OHdG ELISA kit (mlbio, Shanghai) performed according to the manufacturer’s instructions. Briefly, a sample of the supernatant (from the ovary extract) was added to a microtiter plate precoated with 8-OHdG-anti-8-OHdG antibody complexes, followed by one hour of incubation at room temperature. The plate was then washed three times with a wash buffer provided in the kit, followed by the addition of horse peroxidase (HRP)-conjugated secondary antibody and further incubation. The plate was then washed to remove unbound HRP-conjugated antibody and the substrate TMB was added, and after 30 min incubation, a color reaction appeared, which was quantified by measuring the absorbance of the plate at 450 nm using a BioTeck CytationTM 3 microplate reader (BioTek Instruments, Inc.). The level of 8-OHdG was expressed as ng per mL extract (ng·mL-1).
2.4.2 Malondialdehyde assay
The level of MDA in the ovary was determined from its reaction with thiobarbituric acid in the presence of glacial acetic acid to form a color adduct that can be quantified by absorbance at 532 nm (Zeb and Ullah, 2016). The assay was carried out using an MDA assay kit (Nanjing Jiancheng Biological Engineering Institute) according to the manufacturer’s instructions. The supernatant of the ovary extract was mixed with reagents provided in the kit and incubated at 95°C for 40 min. The sample was cooled and then centrifuged at 1500 × g for 10 min, and the absorbance of the supernatant was then measured at 532 nm using a BioTeck CytationTM 3 microplate reader (BioTek Instruments, Inc.). The level of MDA was expressed in nmol per mg protein (nmol·mg-1).
2.4.3 Reactive oxygen species assay
Reactive oxygen species (ROS) was measured by its oxidation of 2,7-dichlorodihydroflorescein diacetate (DCFH-DA) to 2’7’-dichlorofluorescein (DCF), which emits strong green fluorescence (Ng and Ooi, 2021). The assay was conducted using a ROS assay kit (Beyotime Institute of Biotechnology, Shanghai, China) according to the manufacturer’s instructions. Briefly, the ovary was first minced in cold physiological saline to obtain a cell suspension. The cell suspension was passed through a 300-mesh nylon net and then centrifuged at 200 × g/4°C for 10 min to collect the cells. The cell pellet was washed twice with physiological saline and then resuspended in 10 mmol·L-1 DCFH-DA followed by incubation at 37°C for 20 min. After that, the cells were washed with physiological saline, and the fluorescence intensity of the cells was measured with a BioTeck CytationTM 3 microplate reader (BioTek Instruments, Inc.) using excitation and emission wavelengths of 525 nm and 488 nm, respectively. The level of ROS was expressed as a unit of fluorescence intensity.
2.4.4 Protein carbonylation assay
The extent of protein carbonylation (PCO) was determined as previously described (Xie et al., 2007). In this assay, the reactive carbonyl groups on the proteins are detected by their reaction with 2,4-dinitrophenylhydrazine (DNPH) to form 2,4-dinitrophenyhydrazone, a red precipitate that can be measured by absorbance at 370 nm. The assay was carried out using a protein carbonyl assay kit (Nanjing Jiancheng Bioengineering Institute) according to the manufacturer’s instructions. The supernatant of the ovary extract obtained above was mixed with specific reagents provided in the kit, followed by 30 min of incubation at 37°C in the dark. The samples were then mixed with another reagent, also provided in the kit, followed by 15 min of incubation at 37°C and subsequent centrifugation at 12,000 × g for 15 min. The absorbance of the supernatant at 370 nm was then measured with a BioTeck CytationTM 3 microplate reader (BioTek Instruments, Inc.) to determine the level of PCO, which was then expressed as nmol per mg protein (nmol·mg-1).
2.4.5 DNA-protein crosslinking assay
The extent of DNA-protein crosslinking (DPC) was determined using the method described by Xie et al. (2007). Briefly, the ovary was minced in cold physiological saline to release individual cells. An aliquot (500 µL) of the cells was lysed in 2% SDS to release the free proteins and protein-DNA complexes, both of which were precipitated via centrifugation at 9,000 × g/4°C for 5 min. The supernatant, which contained the free DNA, was retained, and the resulting protein precipitate was then treated with proteinase K to digest the proteins and release the DNA from the protein-DNA complexes (bound DNA). The concentrations of free DNA and protein-bound DNA were quantified using Hoechst 33258 fluorescent dye. Fluorescence was measured with a BioTeck CytationTM 3 microplate reader (BioTek Instruments, Inc.) using excitation and emission wavelengths of 350 nm and 460 nm, respectively. The results were reported as the percentage (DPC coefficient) of bound DNA relative to the total DNA (free DNA plus bound DNA).
2.4.6 Glutathione assay
The level of glutathione (GSH) was measured by its reaction with 5,5’-dithio-bis (2-nitrobenzoic acid) (DTNB) to form 5-thio-2-nitrobenzoic acid (TNB), which can then be quantitated by absorbance at 412 nm (Rahman et al., 2006). The assay was carried out using a commercial GSH assay kit (Nanjing Jiancheng Biological Engineering Institute) according to the manufacturer’s instructions. Briefly, the supernatant of the ovary extract obtained above was mixed with buffer and DTNB provided in the kit and allowed to react for 5 min. The absorbance of the sample at 405 nm was then measured with a BioTeck CytationTM 3 microplate reader (BioTek Instruments, Inc.) and the GSH level was expressed as nmol per mg protein (nmol·mg-1).
2.5 Reverse transcription quantitative PCR (RT-qPCR)
Two clams were randomly taken from each tank on days 1, 3, and 6, and their ovaries were extracted and used for total RNA extraction. Total RNA was extracted from the ovary sample using a UNIQ-10 column Trizol Total RNA Extraction Kit (Shanghai Sangon, China) following the manufacturer’s instructions. The quality of the extracted RNA was determined as previously described (Huang et al., 2020). The RNA was then reverse transcribed into cDNA using a PrimeScript RT Reagent Kit (Takara) as described in the manufacturer’s instructions. The obtained cDNA was then used as a template for qPCR analysis of various M. meretrix genes (Bax, Bcl-2, caspase 3, HO-1, Hsp70, NQO-1, Nrf2, and MT) using gene-specific primers (Table 1). The β-actin gene was also analyzed as a housekeeping gene. Quantitative PCR was carried out using a TB Green PCR kit (Takara, China), which was performed on a Roche LC480 PCR instrument (Switzerland). The PCR conditions were set as follows: an initial step at 95°C for 5 min followed by 40 cycles of 15 s at 95°C, 15 s at 58°C, and 20 s at 72°C. The relative expression level was calculated by using the 2 - ΔΔΔD Ct method (Livak and Schmittgen, 2001; Huang et al., 2020).
2.6 Statistical analysis
The data were statistically analyzed with the SPPSS Statistical Package (Version 23.0, Chicago, USA) and Origin Statistical Package (Version 9.2, Northampton, USA). Significant differences were determined by a one-way ANOVA. All data were expressed as means ± standard errors.
3 Results
3.1 Bioaccumulation of Cd2+ in M. meretrix ovary
To determine if Cd2+ accumulated in M. meretrix could be eliminated by keeping the clams in clean water for a short time after exposure to water containing Cd2+, the Cd2+ levels in the water where the clams were kept as well as in the ovaries of these clams from the three treatments were measured daily and compared. No changes in the Cd2+ level occurred in the water of the control group over the duration of the experiment (Figure 1A). Despite the fact that no Cd2+ was added to the water in the control group, a minute concentration of Cd2+ was still detected in the water, suggesting that it may have been Cd2+ absorbed by the clams when they were raised in the aquaculture farm and was released into the water during the six-day experiment. In the case of the Cd-1 group, the Cd2+ level in the water varied among the different days, reflecting a different amount of Cd2+ absorbed each day, even though the initial concentration of Cd2+ in the water was the same each day (Figure 1A). The Cd2+ levels in the water on days 1, 3, 4, 5, and 6 were significantly less than the level on day 2, suggesting the least absorption by M. meretrix on day 2. As for the Cd-2 group, the water Cd2+ levels measured for the first three days of the treatment were similar to those of the Cd-1 group, as expected because the water in both groups contained the same Cd2+ concentration at the start of each day. However, during the next three days where the clams in the Cd-2 group were kept in clean water, the Cd2+ level in the water was significantly higher than the Cd2+ level in the water of the control group on days 4, 5, and 6. This indicated the release of Cd2+ from the clams back into the water, but there was significantly more Cd2+ being released on the last two days (days 5 and 6) compared with the first day (day 4), with no significant difference between days 5 and 6. The result showed that Cd2+ in the water continued to be absorbed by the clams as long as the animals were exposed to Cd2+-containing water, and that changing over to clean water allowed some of the previously absorbed Cd2+ to be released back into the water.
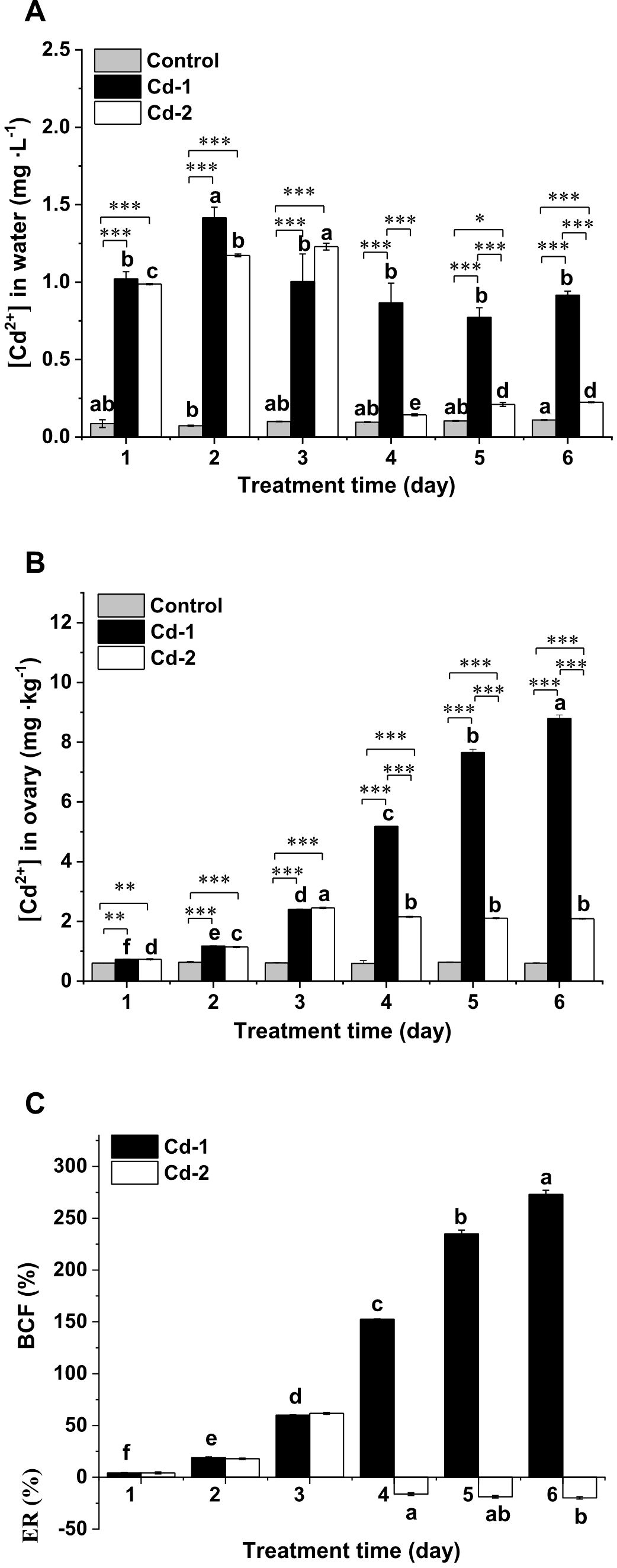
Figure 1. Changes in Cd concentrations in the water and M. meretrix ovary from different treatment groups. Meretrix meretrix individuals were exposed to artificial sea water without (control) or with added Cd (3 mg·L-1) for six days (Cd-1) or with added Cd for three days, followed by depuration in sea water only for another three days (Cd-2), and Cd concentrations in the water and ovary were determined daily. (A) Cd concentration in the water. (B) Cd concentration in the ovary. (C) Bioaccumulation factors (BCFs) of the Cd-1 and Cd-2 groups and elimination rate (ER) of the Cd-2 group. Data are shown as mean ± standard deviations from three determinations. ‘*’, ‘**’, and ‘***’ indicate statistical significance among groups at the P<0.05, P<0.01, and P<0.001 levels, respectively. Letters (a-e) above the bars indicate significant (P<0.05) differences among different days for the same group as revealed by Tukey’s post-hoc multiple comparison tests.
The accumulation of Cd2+ in M. meretrix was evaluated by measuring the changes in Cd2+ levels in the ovary over time. Overall, the ovary Cd2+ level was lowest for the control group, remaining at about 0.6 mg·kg-1 throughout the entire experiment, whereas the ovary Cd2+ level was highest for the Cd-1 group, which continued to increase over the six-day treatment period (Figure 1B). The ovary Cd2+ level of the Cd-1 group ranged from about 0.7 mg·kg-1 on day 1 to almost 9 mg·kg-1 on day 6, more than 10-fold that of the control group. This is reflected in the BCF value, which increased at all exposure times, reaching the highest value on day 6 of the treatment (Figure 1C), indicating that the clams continued to accumulate the metal during the entire exposure period. In contrast, the Cd2+ level in the ovary of the Cd-2 group showed an initial increasing trend, reaching a maximum level after day 3, consistent with the increase in BCF values over the exposure time (Figures 1B, 1C). However, after changing to Cd2+-free water during the depuration period, the Cd2+ level in the ovary started to decrease slightly for the remaining three days, but a significant difference in the elimination rate was only observed between days 1 and 3 of depuration (Figure 1C). The result suggested that the elimination of Cd absorbed by M. meretrix during the three-day depuration was not so significant, although some Cd was released.
3.2 Damage to ovarian tissue
Cadmium accumulation within the tissue can often cause damage or irregularities in the tissue cells. To demonstrate the damage within the ovarian tissue caused by cadmium exposure, the ovaries taken from M. meretrix individuals at the end of the 3-day and 6-day treatment periods were subjected to histological analysis. The structure of the ovarian tissue sustained no damage in the case of the control group (Figure 2A), where the ovarian cells had a normal shape and were tightly arranged, and the number of ovarian cells was higher compared with the ovarian cells of the Cd-exposed groups, either for three days (Figure 2B) or six days (Figure 2C). Since the 3-day exposure could be represented by either the Cd-1 or Cd-2 group, only the Cd-1 result is represented here. The control group also had relatively intact follicular epithelial cells, and the number of follicular epithelial cells was higher than those of the Cd-exposed groups, with 6-day exposure being somewhat more severe than 3-day exposure (Figures 2A–C, red arrows). In the Cd2+-exposed groups, the ovarian tissue structure was relatively loose (white rectangles), the number of ovarian cells was reduced, and the arrangement of these cells was rather loose and irregular (yellow arrows) (Figures 2B–D). The follicular wall became thinner compared with the control group (red arrows) (Figures 2A-D). Some ovarian cells were deformed and damaged (Figures 2B, C). The structure of ovarian cells from the clams subjected to three days of depuration following three days of Cd exposure (Cd-2 group) remained relatively tightly arranged (Figure 2D), and the degree of tissue structure damage was lower than that detected before depuration (Figure 2B) or without depuration (Figure 2C). Thus, the lower extent of tissue damage observed for M. meretrix ovary subjected to Cd2+ exposure followed by depuration may suggest a reversal of the damage caused by Cd2+.
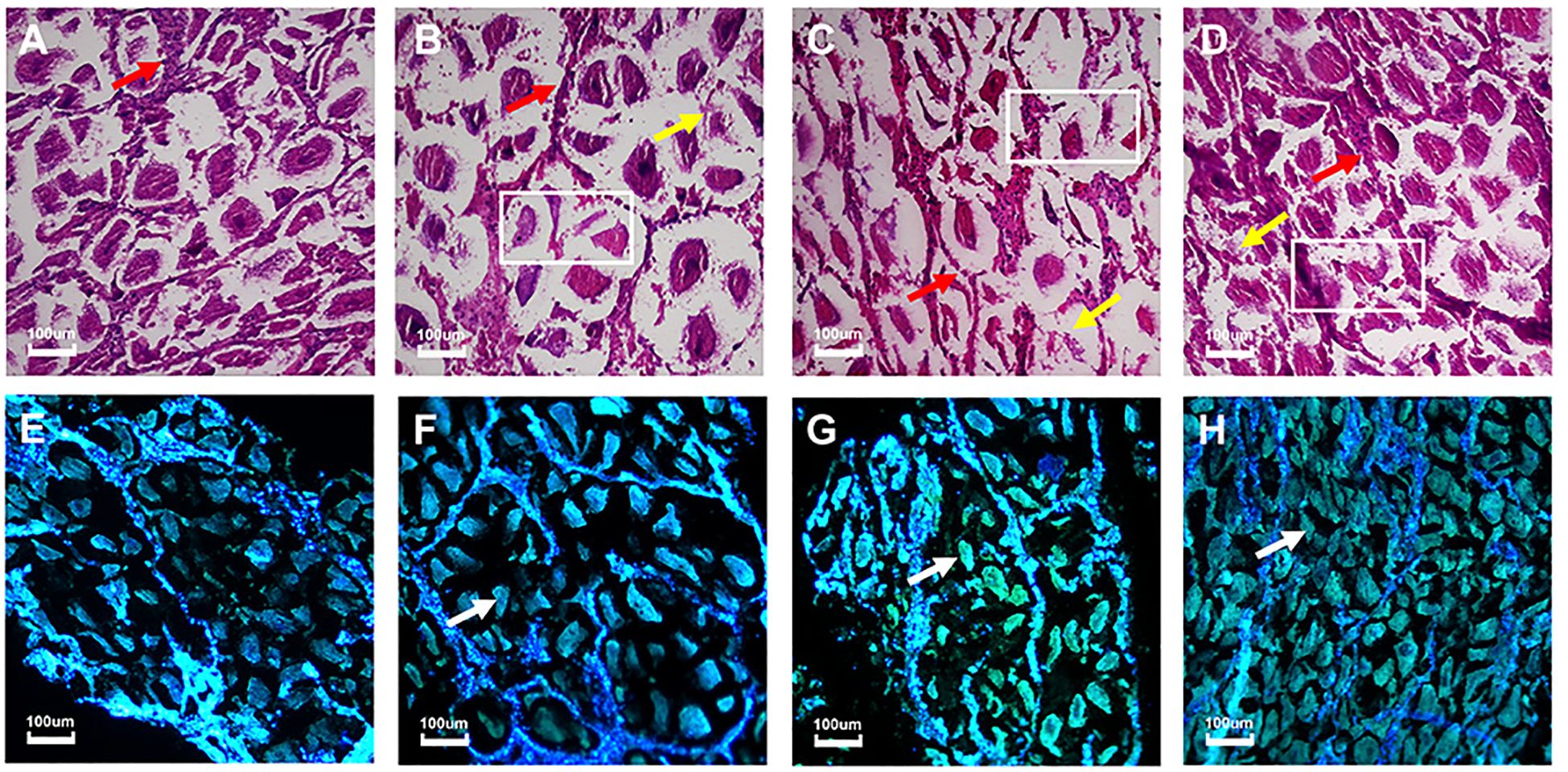
Figure 2. Changes in M. meretrix ovarian tissue structure following exposure to Cd2+ as revealed by histological and Tunel assays. (A–D) show the structure of the ovarian tissue as revealed by histological observation. (D–G) show the apoptosis of clam ovarian cells detected by the Tunel assay. (A, E) Control clams; these clams were kept in water without Cd2+ for six days. (B, F) Clams that were treated with 3 mg·L-1 Cd2+ for three days. (C, G) Clams that were treated with 3 mg·L-1 Cd2+ for six days. (D, H) Clams that were treated with 3 mg·L-1 Cd2+ for three days and then in just water for another three days. The ovaries were taken from the clams at the end of the treatment period. The rectangle within the image indicates the loss of gonadal tissue structure. The yellow arrow indicates irregularly distributed ovarian cells. The red arrow indicates follicular epithelial cells. The white arrow indicates apoptotic cells with green fluorescence.
In the Tunel assay, most of the ovarian cells in the control group exhibited blue fluorescence (healthy cells), and fewer cells exhibited green fluorescence (apoptotic cells), indicating that there was no significant apoptosis (Figure 2E). However, upon Cd2+ exposure for three days (Figure 2F) or six days (Figure 2G), the number of blue fluorescent cells decreased while the number of green fluorescent cells (white arrows) increased, with the 6-day exposure showing relatively more green fluorescent cells, indicating a marked increase in the extent of apoptosis that was more severe with a longer exposure time. When the 3-day Cd2+-exposed group was subjected to depuration for three days (Figure 2H), there were more blue-fluorescent cells and fewer green-fluorescent cells compared with the pre-depuration stage (Figure 2F), although the number of green-fluorescent cells was still higher compared with the control group (Figure 2E). The result of the Tunel assay was consistent with the observation obtained from histological analysis, supporting the mitigation of Cd toxicity during the depuration period.
3.3 Oxidative damage
The extent of oxidative damage induced by Cd2+ in M. meretrix was evaluated by measuring the levels of various oxidative stress indicators in the ovary following Cd2+ exposure. Changes in these oxidative stress indicators are shown in Figure 3. Overall, 8-OHdG, MDA, ROS, PCO, and PDC levels in the ovary remained rather similar across the different time points in the case of the control group, except for ROS, in which some increases were detected on days 3 and 6 compared with day 1, but the increases were not significant. As for the two Cd2+-exposed groups, prominent increases in these indicators were observed on day 3, but for the Cd-1 group, these indicators continued to increase on day 6, whereas for the Cd-2 group, noticeable decreases were detected on day 6, suggesting an alleviation of the state of oxidative stress.
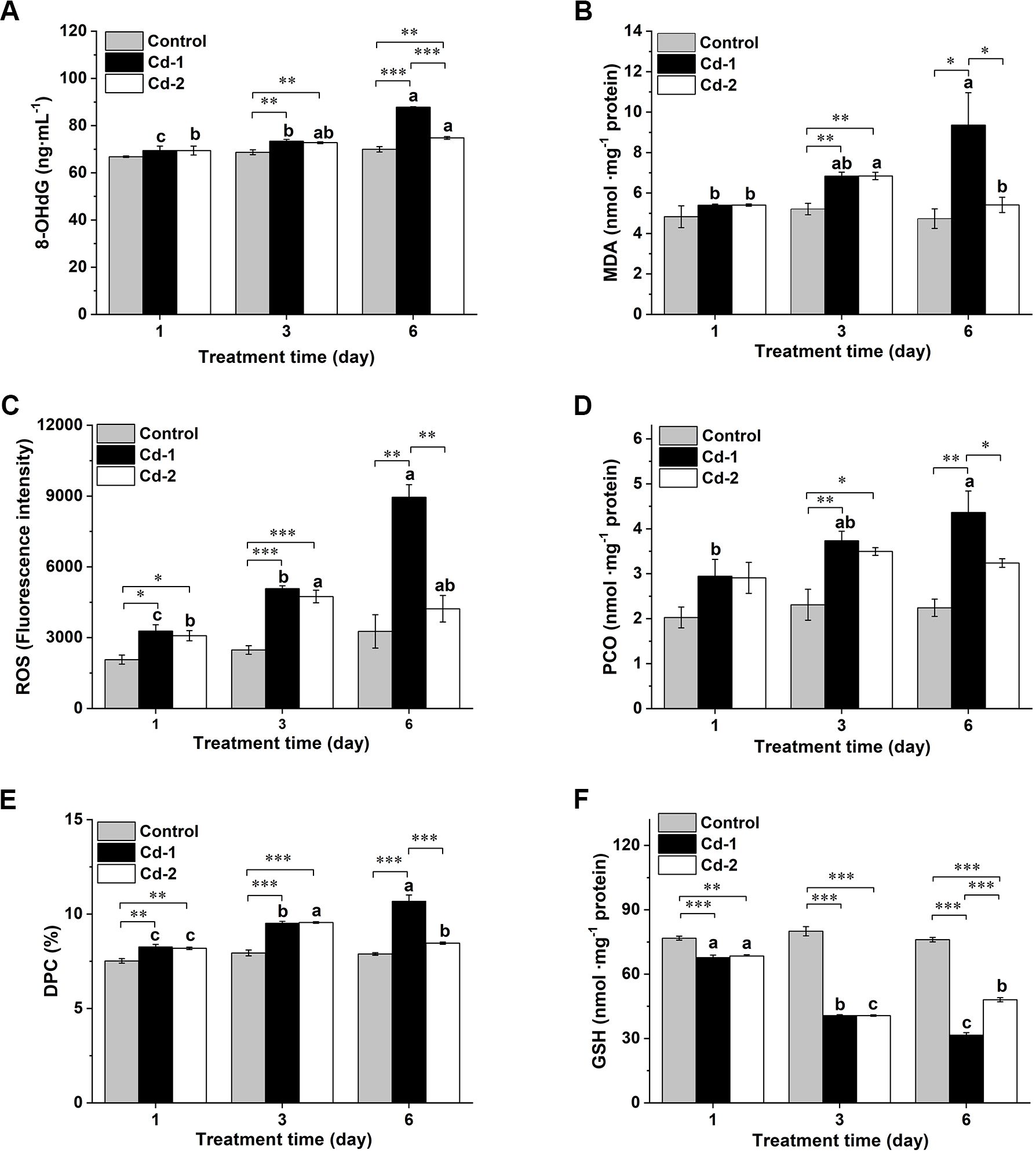
Figure 3. Changes in various oxidative stress indicators in M. merertrix ovary following exposure to Cd2+ and elimination via depuration. Ovaries extracted from each group (control, Cd-1, or Cd-2) were subjected to biochemical assays to measure 8-OHdG (A), MDA (B), ROS (C), PCO (D), DPC (E), and GSH (F). Data are shown as mean ± standard deviations from three determinations. ‘*’, ‘**’, and ‘***’ indicate statistical significance among groups at the P<0.05, P<0.01, and P<0.001 levels, respectively. Letters (a–c) above the bars indicate significant (P<0.05) differences among different days for the same group as revealed by Tukey’s post-hoc multiple comparison tests.
Slight but significant increases in 8-OHdG levels were observed for Cd2+-exposed groups over the control group on day 3. On day 6, the 8-OHdG level in the Cd-1 group continued to increase, whereas that of the Cd-2 group remained similar among the three time points, despite a slight increase even after three days of depuration (Figure 3A). Similar profiles were observed for the changes in the levels of MDA, ROS, PCO, and DPC. Both Cd2+-exposed groups showed significant increases in MDA levels over the control group on day 3, but on day 6, the MDA level of the Cd-1 group continued to increase, reaching about 2-fold the level of the control, while the MDA level of the Cd-2 group decreased to a similar level (Figure 3B). The Cd-1 group showed significantly higher ROS levels than the control group at the end of days 1, 3, and 6, reaching about 2.5-fold the level of control on day 6 (Figure 3C). As for the Cd-2 group, significant increases in ROS level over the control group were detected after days 1 and 3, but after day 6, the increase relative to the control was not significant and was about 11% lower than that of day 3. Similar trends were observed for PCO and DPC profiles, with the control group having the lowest level, while the Cd-1 group had the highest level across all three different time points, with both PCO and DPC levels being significantly higher than those of the control groups at all time points except for day 1 in the case of PCO (Figures 3D, E). As for the Cd-2 group, PCO and DPC levels were about 7% and 12%, respectively, lower than their levels on day 3, though only the decrease in DPC was significant. However, for both indicators, their levels on day 6 were not significantly different from those of the control, despite some increases. Finally, for the GSH profile, the level of GSH pretty much remained unchanged over the duration of the experiment in the case of the control. As for the two Cd2+-exposed groups, GSH levels decreased significantly (P<0.001) relative to the control for all time points measured in the case of the Cd-1 group and up to day 3 for the Cd-2 group, as there was some increase on day 6 relative to day 3, and the increase was significant though the level on day 6 remained significantly (P<0.001) lower than that of the control (Figure 3F). The results from oxidative stress indicators confirmed the induction of oxidative stress in M. meretrix ovary by Cd2+, which is shown here as increased levels of 8-OHdG, MDA, ROS, PCO, and DPC and decreased levels of GSH, but the effect was reduced when the clams were kept in Cd2+-free water after exposure to Cd2+-containing water, as shown by reduced oxidative indicator levels and an increased antioxidant level, consistent with the reduced Cd2+ level in the ovary tissue.
3.4 Analysis of oxidative stress-related gene markers
The withdrawal of the Cd2+ stress also changed the state of oxidative stress via an adjustment of the expression of various oxidative stress and/or apoptosis-related genes. The genes selected for such analysis were Bax, Bcl2, Caspase 3, HO-1, Hsp70, NQO1, Nrf2, and MT. Overall, except for the Hsp70 gene, the transcript levels of the other genes in M. meretrix ovary did not change over the duration of the experiment in the case of the control clams (Figure 4), and this was in accordance with our anticipation since the control clams were not subjected to Cd stress. However, on day 6, the transcript level of the Hsp70 gene appeared to increase relative to the levels on days 1 and 3, attaining more than 2 folds the levels on days 1 and 3, and this might be attributed to the stress contributed by the absence of food for the clams during the experimental period. For the Cd-1 group, the transcript levels of these genes in M. meretrix ovary increased with longer exposure to Cd2+, reaching the highest level on day 6 (Figures 4A, C–E, G, H), except for Bcl-2, which remained similar to day 3, and NQO1, which decreased slightly (Figures 4B, F). The transcript levels of these genes ranged from 1.8-fold (Hsp70) to 8-fold (NQO1) the levels of the control on day 6. These increases were consistent with enhanced oxidative stress caused by continuous exposure to Cd2+. Upon removal of the Cd2+ stress, as demonstrated by the Cd-2 group, a significant reduction in the transcript levels of these genes was observed on day 6 relative to day 3, with the greatest reduction being more than 60% in the cases of NQO1 and Nrf2. Although the transcript levels of these genes were still higher than the levels of the control group, the differences were not significant for Bcl-2, caspase-3, Hsp70, and Nrf2. The increase in MT transcript observed for the Cd-2 group relative to the control on day 6 was the highest among all the genes analyzed, with the increase being over 100% (Figure 4H). Overall, the transcript levels of these oxidative stress-related genes were consistent with the results of the oxidative stress indicators, illustrating that induction of gene expression may play a major role in the response to oxidative stress in M. meretrix ovary.
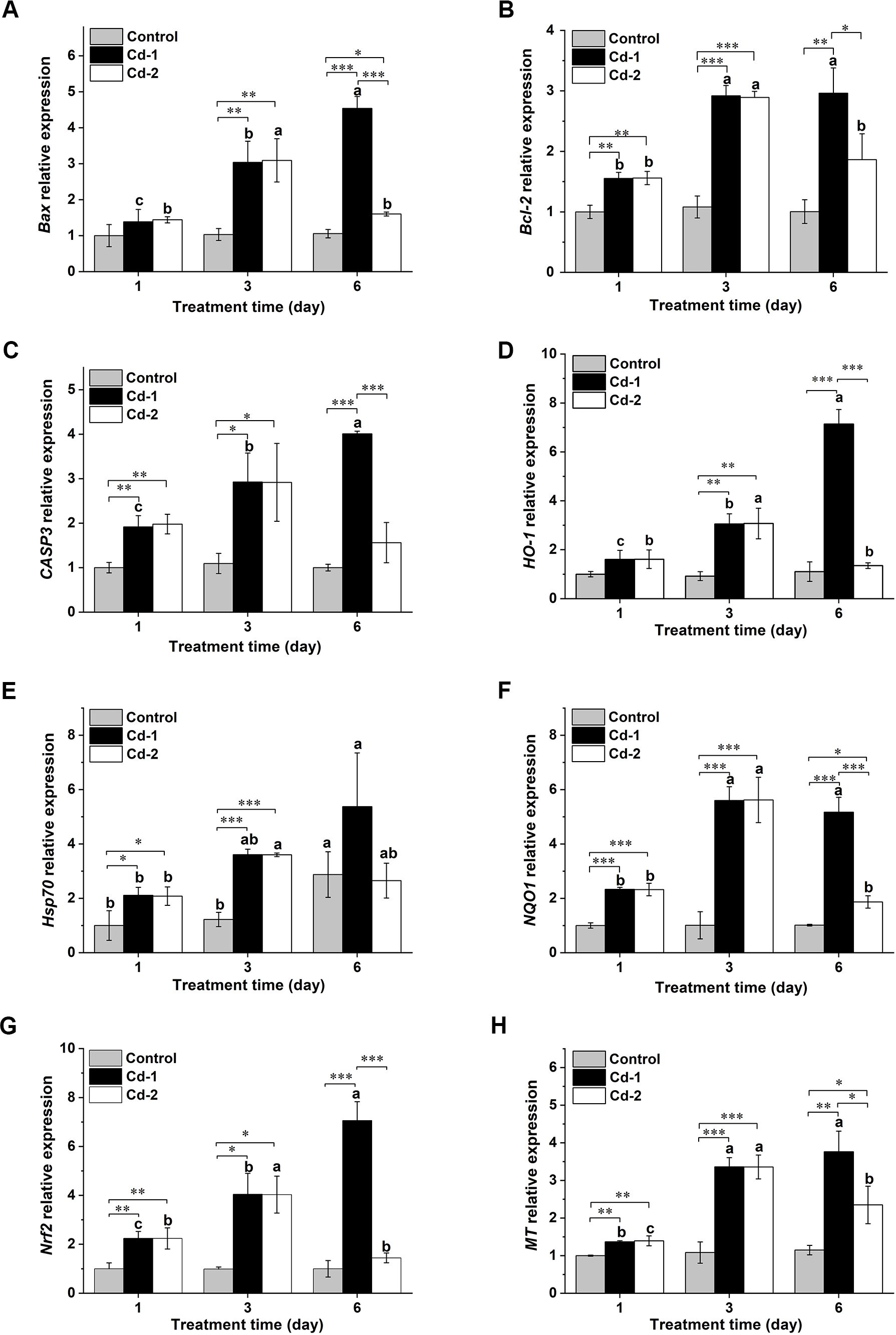
Figure 4. Changes in various oxidative stress-related genes in M. merertrix ovary as determined by real-time quantitative PCR (qRT-PCR) following exposure to Cd2+ and elimination via depuration. Ovaries extracted from each group (control, Cd-1, or Cd-2) were subjected to qRT-PCR analysis to measure the mRNA levels of the Bax (A), Bcl-2 (B), caspase-3 (C), HO-1 (D), Hsp70 (E), NQO1 (F), Nrf2 (G), and MT (H) genes. Data are shown as mean ± standard deviations from three determinations. ‘*’, ‘**’, and ‘***’ indicate statistical significance among groups at the P<0.05, P<0.01, and P<0.001 levels, respectively. Letters (a–c) above the bars indicate significant (P<0.05) differences among different days for the same group as revealed by Tukey’s post-hoc multiple comparison tests.
4 Discussion
4.1 Cd toxicity is partly reversible
The toxic effects of heavy metals on bivalves have been well documented in the past few decades. Such investigations have been prompted by the concern of increasing heavy metal pollution in the marine environment, which can have tremendous effects on aquaculture and the marine ecosystem as a whole. Many of the studies on the toxic effects of heavy metals tend to focus on bivalves such as mussels and oysters (Géret et al., 2002; Escorcia and Chang, 2010; Bartlett et al., 2020), although clams such as M. meretrix have also attracted increasing interest in recent times, especially in China, where the cultivation of this species constitutes a major aquaculture output. Among the different heavy metals that have been investigated for their toxic effects on bivalves is cadmium, which is featured in many studies. Although the toxic effect of cadmium on bivalves, including mussels, oysters, crabs, and clams, has been shown to manifest in the form of oxidative damage, much of the investigation has focused on changes in the levels of oxidative stress indicators and expression levels of oxidative stress-related genes (Huang et al., 2020; Perića et al., 2020; Yang et al., 2023). Cadmium exerts its toxic effect in M. meretrix by causing oxidative stress, but it may also weaken the shells by impacting shell mineralization, as demonstrated for other toxic metals (Stewart et al., 2021). The best way to deal with the problem of heavy metal contamination of the marine environment is probably a combined clean-up via bioremediation and the prevention of further pollution. However, bioremediation of the ocean, even if limited to just coastal regions, can still be a daunting task that is extremely difficult, if not impossible, to implement because the pollutants can be carried by ocean currents over long distances. Thus, an alternative to reducing the level of Cd in the tissues of the contaminated bivalves might be a direct decontamination approach that seeks to release the metals accumulated in the tissues in the so-called depuration process before consumption.
In this study, we have shown that Cd accumulated in the tissue of M. meretrix during exposure could be eliminated by releasing it back to the water upon switching to Cd2+-free water for a few days. However, the elimination was only slight, since after three days of depuration, these clams exhibited only a slight decrease in Cd level in the ovary, about 10% less, compared with the Cd level accumulated after three days of exposure (Figure 1B). Besides, after three days of depuration, the Cd level in the ovary of the clams was still more than threefold that of the control group, suggesting the process to eliminate the Cd was somewhat inadequate. In fact, depuration with purified seawater is the most common method used to reduce the level of waterborne contaminants in bivalves destined for human consumption. However, such a method is considered ineffective for eliminating heavy metals, and other methods, including biological, physical, and chemical methods, have been explored to increase their effectiveness (Martinez-Albores et al., 2020). Previous studies looking at the accumulation and elimination of Cd in marine organisms revealed significant differences in the accumulation and elimination potential among different tissues, with internal tissues showing greater accumulation and less elimination during exposure and depuration, respectively. For example, in the carp Cyprinus carpio, a sharp increase in kidney and liver Cd levels was observed over a long period (up to 127 days) of exposure, while the Cd level in the muscle was only significant after 106 days, and no loss of kidney and liver Cd was observed during the depuration period, whereas the loss of Cd in the muscle was rapid and immediate, reaching a level similar to that of the pre-exposure stage (de Conto et al., 1999). Our data and those previously reported by other investigators may suggest that depuration could only achieve a partial release of Cd from the bivalve tissues once the metal is absorbed, thereby reducing but not eliminating the risk of human exposure via the food web.
Despite only a slight decrease in Cd level in the ovary following three days of depuration, the decrease did have a substantial effect on reversing the toxicity in the clams, as demonstrated by less damage to the ovarian tissue structure and reduced apoptosis (Figure 2), probably via repair and regeneration of cells. At the same time, substantial mitigation of oxidative stress was also observed, as shown by reduced levels of oxidative indicators after depuration (Figure 3). Significant differences in oxidative indicators such as MDA and DPC were reduced by 21 and 12%, respectively, compared with the pre-depuration stage. Although the other oxidative indicators measured, 8-OHdG, ROS, and PCO, showed no significant reduction after depuration, their levels were comparable to those of the control clams, except for GSH and PCO, which remained significantly different from those of the control clams. Overall, the results did suggest that the toxic effects exerted by exposure to Cd2+ stress could at least be partly reversed when the stress factor (tissue Cd level) was slightly reduced. It also implied that damage caused by Cd2+ via oxidative stress is not permanent as long as the organism can survive long enough to have the stress lifted.
Obviously, the ovary is not the only tissue affected by Cd2+ exposure, since all soft tissues of M. meretrix are capable of accumulating Cd2+ in the water (Huang et al., 2020), and this is heavily influenced by its filter feeding lifestyle. However, the ovary is a vital organ since it is the core of the reproductive process. Thus, even though oxidative damage does not result in the death of the organism, damage to the ovary might lead to a loss of reproductive ability and a gradual reduction in population size. We have previously reported the gradual loss of follicular cells and gonadal tissue structure for the ovary after exposure of the clams to 3 mg·L-1 Cd2+ (Bai et al., 2022), consistent with what we observed in this study, and this was accompanied by a loss in the number of normal cells and an increase in the number of apoptotic cells, and the severity of both increased with increasing Cd concentrations. Other aquatic animals, such as the crabs Eriocheir sinensis and Sinopotamon henanense, have also been found to accumulate Cd2+ in the ovary following exposure to Cd2+ (Xu et al., 2016; Chen et al., 2019). Under laboratory conditions, the Cd2+ concentration to which the clams were exposed exceeded those that are found in the marine environment. They were chosen to produce a clear effect of toxicity under short-term exposure (a few days), since a level that better reflects the environmental concentration might take a long time (months or even years) to produce the effect. The Cd2+ concentration in this study was chosen from the concentrations used in our previous studies (Wang et al., 2021; Bai et al., 2022), where 3 mg/L appears to cause obvious damage to tissues while ensuring that the level of damage is not too severe.
The induction of oxidative damage in M. meretrix ovary was also marked by changes in the expression levels of oxidative stress-related genes. A number of these genes are not merely involved in oxidative stress response but also play a role in inflammation, such as the HO-1 gene (Chiang et al., 2021) or apoptosis, which include the Bax (Robin et al., 2018), Bcl-2 (Singh et al., 2019), NQO1 (Beaver et al., 2019), Capase-3 (Eskandari and Eaves, 2022), and Hsp70 (Beere et al., 2000), all of which were upregulated by Cd2+-exposure (Figure 4), demonstrating that Cd2+-induced toxicity affected various aspects of the cell status. Reduction in Cd2+-upregulated gene expression following depuration was most pronounced for the Bax, HO-1, NQO1, and Nfr-2 genes, in which the depuration resulted in almost 50% reduction or more in expression, and the reduction was statistically significant (Figures 4A, D, F, G). The Nrf2 gene codes for a pleiotropic transcription factor that regulates the expression of genes involved in the response to oxidative stress and drug detoxification as well as metabolism and inflammation (Ma, 2013; He et al., 2020; Hassanein et al., 2023), and rises in its expression level demonstrated the wide effect of Cd toxicity. Furthermore, depuration also resulted in down-regulated expression levels of the Nrf2 and HO-1 genes to levels similar to or comparable to those of the control clams. This may suggest that the expression of oxidative stress-related or inflammation-related genes was more or less restored to the control levels after three days of depuration, despite the fact that the level of Cd in the tissue of the depurated clams remained significantly higher, almost 3.5-fold the level of the control clams. Besides the large reduction in the Nrf2 gene following depuration, the HO-1 and NQO1 genes were the other two genes that showed a large (>50%) reduction in expression. Both genes are subjected to regulation by the Nrf2 gene (Araujo et al., 2012; Ross and Siegel, 2021), reflecting their similar reduction in magnitude. In contrast, the expression level of the MT gene, which codes for a metal-binding protein, remained significantly higher in the ovary of the Cd2+-exposed clams compared with the control clams, even after the former had been depurated for three days, although it was significantly lower compared with the level before depuration. This could be due to the direct metal scavenging function of MT in addition to its other functions (Wang et al., 2014), which better reflects the level of Cd in the tissue than the other genes, which are not involved in direct metal binding but their expression could be induced by the stress caused by an increased level of Cd2+.
4.2 Ecological implications
Besides the toxic effects induced in M. meretrix by Cd2+ following exposure, there is also concern that the metal accumulated in the tissues of the clam might find its way into humans via the food chain. Thus, the release of Cd2+ from the clams upon being kept in Cd2+-free water clearly indicated that the contamination might be partly reversible, and the extent of recovery might depend on the length of the depuration period. A longer depuration period would allow the clams to continuously eliminate the metal or repair the damage when the stress factor is no longer present or is largely mitigated. The bioaccumulation of Cd2+ and its elimination have also been carried out in the fresh-water mussel Anodonta woodina, where the mussels were subjected to a long period (up to 28 days) of depuration following a long exposure (28 days) to Cd2+. Although the accumulation of Cd2+ continued to increase with time during the exposure period, elimination did not continue to increase with prolonged depuration time, and the level of Cd2+ in the tissues still remained a number of folds higher than the level found in the control tissues (Jing et al., 2019). This suggests that complete elimination of the metal to the pre-exposure level might be impossible. Since bivalves are also an important food source for humans and other marine organisms (Vaughn and Hoellein, 2018), contamination by toxic heavy metals will have important consequences for the marine food web. Thus, the effect of pollution associated with Cd or other toxic heavy metals can present a threat to the health of humans and other marine organisms dependent on bivalves as a source of food, since the absorbed metals in the tissues of the bivalves can be transmitted through the food chain.
The absorption of Cd2+ by M. meretrix may be considered a form of natural bioremediation whereby the clams are used as biofilters to remove the metals from the water. Bivalves in general can tolerate and accumulate contaminants, including toxic heavy metals, and because of their sessile nature and wide distribution, they can be used to remove the contaminants in addition to serving as indicators of contaminant monitoring. Deepthi et al. (2020) have reported the use of bivalves in the bioremediation of wastewater, and over 80% of contaminants such as COD, total nitrogen, and phosphorus have all been removed within seven days. The ability of M. meretrix to absorb and release Cd2+ reported here may point to the potential of the use of the clams as recyclable biofilters to harvest and release Cd2+ through cycles of exposure and depuration in a controlled manner.
5 Conclusion
The short-term exposure of M. meretrix to a high concentration of Cd2+ in artificial seawater, followed by short-term depuration performed in Cd2+-free seawater, conducted in this study has demonstrated that toxicity in a marine bivalve caused by a toxic metal could be partly reversed through the combined action of metal elimination and mitigation of toxic effects induced by the metal. This included the alleviation of oxidative stress, manifested through a reduction in oxidative stress, enhanced antioxidant capacity, and reduced expression of genes that regulate oxidative stress and/or apoptosis. The overall effect consisted of less oxidative damage and repair of damaged tissue, as exemplified by the ovary, which is a vital reproductive organ. Although the recovery from Cd2+-induced damage, both in terms of oxidative status and tissue morphology, did not restore the animal to the non-exposed stage, the findings may still imply that restoration to undo the tissue damage caused by Cd or other toxic metals may play an important role in maintaining a viable population in the presence of low-level contamination in the context of aquaculture. Further study looking at the reproductive potential of M. meretrix under Cd2+ exposure and depuration might shed further light on the toxicological effect of Cd2+ on the survival of the clams and the major mechanisms that are involved in restoring the health status of the animals and give a better indication of the resilience of a bivalve-based ecosystem under the threat of toxic heavy metal pollution.
Data availability statement
The original contributions presented in the study are included in the article/supplementary material. Further inquiries can be directed to the corresponding authors.
Ethics statement
The animal study was approved by Wenzhou University’s Animal Ethical and Welfare Committee. The study was conducted in accordance with the local legislation and institutional requirements.
Author contributions
JZ: Writing – original draft, Software, Investigation, Funding acquisition, Data curation. HC: Writing – original draft, Funding acquisition, Data curation. YNZ: Writing – original draft, Software, Funding acquisition, Data curation. YZ: Writing – original draft, Software, Investigation, Data curation. YW: Writing – original draft, Software, Investigation, Formal analysis. AKC: Writing – review & editing, Supervision, Formal analysis, Conceptualization. XY: Writing – review & editing, Supervision, Funding acquisition, Conceptualization.
Funding
The author(s) declare that financial support was received for the research, authorship, and/or publication of this article. This study was supported by the National Natural Science Foundation of China under Grant number 32071514 (to XY), the Nature Science Foundation of Zhejiang Province (CN) under Grant number LY18C030005 (to XY), the National Student Innovation Training Project under Grant number JWXC2023136 (to YNZ), the Student Innovation Training Project of Zhejiang Province under Grant number 2023R020 (to HC) and the 2024 Graduate Scientific Research Foundation of Wenzhou University Grant number 3162024004087 (to JZ).
Conflict of interest
The authors declare that the research was conducted in the absence of any commercial or financial relationships that could be construed as a potential conflict of interest.
Publisher’s note
All claims expressed in this article are solely those of the authors and do not necessarily represent those of their affiliated organizations, or those of the publisher, the editors and the reviewers. Any product that may be evaluated in this article, or claim that may be made by its manufacturer, is not guaranteed or endorsed by the publisher.
References
Araujo J. A., Zhang M., Yin F. (2012). Heme oxygenase-1, oxidation, inflammation, and atherosclerosis. Front. Pharm. 3, 119. doi: 10.3389/fphar.2012.00119
Bai B. B., Yang Y. Q., Wei J. Y., Zheng Q., Wang M. C., Chang A. K., et al. (2022). Cadmium-induced toxicity in Meretrix meretrix ovary is characterized by oxidative damage with changes in cell morphology and apoptosis-related factors. Front. Mar. Sci. doi: 10.3389/fmars.2022.1080516
Bandara R. V. K., Manage M. P. (2023). Heavy metal contamination in the coastal environment and trace level identification. Intech Open. doi: 10.5772/intechopen.106653
Bartlett J., Maher W., Ubrihien R., Krikowa F., Edge K., Potts J., et al. (2020). Fitness of two bivalves Saccostrea glomerata and Ostrea angasi exposed to a metal contamination gradient in Lake Macquarie, NSW Australia: Integrating subcellular, energy metabolism and embryo development responses. Ecol. Indic. 110, 105869. doi: 10.1016/j.ecolind.2019.105869
Beaver S. K., Mesa-Torres N., Pey A. L., Timson D. J. (2019). NQO1: A target for the treatment of cancer and neurological diseases, and a model to understand loss of function disease mechanisms. Biochim. Biophys. Acta Proteins Proteom. 1867, 663–676. doi: 10.1016/j.bbapap.2019.05.002
Beere H., Wolf B., Cain K., Mosser D. D., Mahboubi A., Kuwana T., et al. (2000). Heat-shock protein 70 inhibits apoptosis by preventing recruitment of procaspase-9 to the Apaf-1 apoptosome. Nat. Cell Biol. 2, 469–475. doi: 10.1038/35019501
Candéias S., Pons B., Viau M., Caillat S., Sauvaigo S. (2010). Direct inhibition of excision/synthesis DNA repair activities by cadmium: analysis on dedicated biochips. Mutat. Res. 694, 539. doi: 10.1016/j.mrfmmm.2010.10.001
Chahouri A., Yacoubi B., Moukrim A., Banaoui A. (2023). Bivalve molluscs as bioindicators of multiple stressors in the marine environment: Recent advances. Continental Shelf Res. 264, 105056. doi: 10.1016/j.csr.2023.105056
Chen R. Q., Ye F. Y., Lin J. H., Zhou J. Y., Cai J. J., Ying X. P. (2019). Effects of cadmium and mercury on oxidative stress indexes in the ovary of Eriocheir sinensis. Acta Hydrobiologica Sin. 43, 554–562. doi: 10.7541/2019.067
Chen M. X., Zhou J. Y., Lin J. H., Tang H. C., Shan Y. F., Chang A. K., et al. (2020). Changes in oxidative stress biomarkers in Sinonovacula constricta in response to toxic metal accumulation during growth in an aquaculture farm. Chemosphere 248, 125974. doi: 10.1016/j.chemosphere.2020.125974
Chiang S. K., Chen S. E., Chang L. C. (2021). The Role of HO-1 and its crosstalk with oxidative stress in cancer cell survival. Cells 10, 2401. doi: 10.3390/cells.10092401
de Conto C. C., Petit-Ramel M., Faure R., Garin D., Bouvet Y. (1999). Kinetics of cadmium accumulation and elimination in carp Cyprinus carpio tissues. Comp. Biochem. Physiol. C Pharmacol. Toxicol. Endocrinol. 122, 345–352. doi: 10.1016/S0742-8413(98)10132-9
Deepthi D., Lakshmi V., Babu M. (2020). Bioremediation of wastewater using invasive bivalves. IJITEE. 9, 3077–3079. doi: 10.35940/ijitee.C8475.019320
Escorcia G. B., Chang I. W. (2010). Lipid peroxidation and metallothionein induction by chromium and cadmium in Oyster Crassostrea virginica (Gmelin) from Mandinga Lagoon, Veracruz. Hidrobiológica 20, 31–40.
Eskandari E., Eaves C. J. (2022). Paradoxical roles of caspase-3 in regulating cell survival, proliferation, and tumorigenesis. J. Cell. Biol. 221, e202201159. doi: 10.1083/jcb.202201159
Freitas R., Ramos Pinto L., Sampaio M., Costa A., Silva M., Rodrigues A. M., et al. (2012). Effects of depuration on the element concentration in bivalves: Comparison between sympatric Ruditapes decussatus and. Ruditapes philippinarum. Estuarine Coast. Shelf Sci. 110, 43–53. doi: 10.1016/j.ecss.2012.01.011
Gao Y. L., Hong J. M., Guo Y. K., Chen M. X., Chang A. K., Xie L., et al. (2021b). Assessment spermatogenic cell apoptosis and the transcript levels of metallothionein and p53 in Meretrix meretrix induced by cadmium. Ecotoxicol. Environ. Saf. 217, 12230. doi: 10.1016/j.ecoenv.2021.112230
Gao Y., Wang R., Li Y., Ding X., Jiang Y., Feng J., et al. (2021a). Trophic transfer of heavy metals in the marine food web based on tissue residuals. Sci. Total Environ. 772, 145064. doi: 10.1016/j.scitotenv.2021.145064
Genchi G., Sinicropi M. S., Lauria G., Carocci A., Catalano A. (2020). The effects of cadmium toxicity. Int. J. Environ. Res. Public Health 17, 3782. doi: 10.3390/ijerph17113782
Géret F., Jouan A., Bebianno M. J., Cosson R. P. (2002). Influence of metal exposure on metallothionein synthesis and lipid peroxidation in two bivalve mollusks: the oyster (Crassostrea gigas) and the mussel (Mytilus edulis). Aqua. Living Resour. 15, 61–66. doi: 10.1016/S0990-7440(01)01147-0
Hassanein E. H. M., Ibrahim I. M., Abd-Alhameed E. K., Sharawi Z. W., Jaber F. A., Althagafy H. S. (2023). Nrf2/HO-1 as a therapeutic target in renal fibrosis. Life Sci. 334, 122209. doi: 10.1016/j.lfs.2023.122209
He F., Ru X., Wen T. (2020). NRF2, a transcription factor for stress response and beyond. Int. J. Mol. Sci. 21, 4777. doi: 10.3390/ijms21134777
Huang Y., Tang H. C., Jin J. Y., Fan M. B., Chang A. K., Ying X. P. (2020). Effects of waterborne cadmium exposure on its internal distribution in Meretrix meretrix and detoxification by metallothionein and antioxidant enzymes. Front. Mar. Sci. 7, 2020502. doi: 10.3389/fmars.2020.00502
Jing W., Lang L., Lin Z., Liu N., Wang L. (2019). Cadmium bioaccumulation and elimination in tissues of the freshwater mussel. Anodonta woodiana. Chemosphere 219, 321–327. doi: 10.1016/j.chemosphere.2018.12.033
Lakshmanna B., Jayaraju N., Sreenivasulu G., Lakshmi Prasad T., Nagalakshmi K., Pramod Kumar M., et al. (2022). Evaluation of heavy metal pollution from coastal water of Nizampatnam Bay and Lankevanidibba, East Coast of India. J. Sea Res. 186, 102232. doi: 10.1016/j.seares.2022.102232
Li W., Gu X., Zhang X., Kong J., Ding N., Qi Y., et al. (2015). Cadmium delays non-homologous end joining (NHEJ) repair via inhibition of DNA-PKcs phosphorylation and downregulation of XRCC4 and Ligase IV. Mutat. Res-Fund Mol. M. 779, 112–123. doi: 10.1016/j.mrfmmm.2015.07.002
Liu H., Tian X., Jiang L., Han D., Hu S., Cui Y., et al. (2023). Sources, bioaccumulation, and toxicity mechanisms of cadmium in Chlamys farreri. J. Hazardous Materials 453, 131395. doi: 10.1016/j.jhazmat.2023.131395
Livak K. J., Schmittgen T. D. (2001). Analysis of relative gene expression data using real-time quantitative PCR and the 2(T) (-delta delta c) method. Methods 25, 402–408. doi: 10.1006/meth.2001.1262
Luo M., Zhang Y., Li H., Hu W., Xiao K., Yu S., et al. (2021). Pollution assessment and sources of dissolved heavy metals in coastal water of a highly urbanized coastal area: The role of groundwater discharge. Sci. Total Environ. 807, 151070. doi: 10.1016/j.scitotenv.2021.151070
Ma Q. (2013). Role of nrf2 in oxidative stress and toxicity. Annu. Rev. Pharmacol. Toxicol. 53, 401–426. doi: 10.1146/annurev-pharmtox-011112-140320
Martinez-Albores A., Lopez-Santamarina A., Rodriguez J. A., Ibarra I. S., Del Carmen Mondragón A., Miranda J. M., et al. (2020). Complementary methods to improve the depuration of bivalves: A Review. Foods. 9, 129. doi: 10.3390/foods9020129
Ng N. S., Ooi L. (2021). A simple microplate assay for reactive oxygen species generation and rapid cellular protein normalization. Bio Protoc. 11, e3877. doi: 10.21769/BioProtoc.3877
Patchaiyappan A., Arulkumar A., Shynshiang K., Anandkumar A., Prabakaran K., Basu A., et al. (2023). Bioaccumulation of heavy metals in commercially important marine species from Puducherry coast, Southeast India. Regional Stud. Mar. Sci. 65, 103080. doi: 10.1016/j.rsma.2023.103080
Perića L., Perusco V. T., Nerlović V. (2020). Differential response of biomarkers in the native European flat oyster Ostrea edulis and the non-indigenous Pacific oyster Crassostrea gigas co-exposed to cadmium and copper. J. Exp. Mar. Biol. Ecol. 523, 151271. doi: 10.1016/j.jembe.2019.151271
Qian Y., Zhang W., Yu L., Feng H. (2015). Metal pollution in coastal sediments. Curr. pollut. Rep. 1, 203–219. doi: 10.1007/s40726-015-0018-9
Rahman I., Kode A., Biswas S. K. (2006). Assay for quantitative determination of glutathione and glutathione disulfide levels using enzymatic recycling method. Nat. Protoc. 1, 3159–3165. doi: 10.1038/nprot.2006.378
Robin A. Y., Iyer S., Birkinshaw R. W., Sandow J., Wardak A., Luo C. S., et al. (2018). Ensemble properties of Bax determine its function. Structure 26, 1346–1359. doi: 10.1016/j.str.2018.07.006
Ross D., Siegel D. (2021). The diverse functionality of NQO1 and its roles in redox control. Redox Biol. 41, 101950. doi: 10.1016/j.redox.2021.101950
Singh R., Letai A., Sarosiek K. (2019). Regulation of apoptosis in health and disease: the balancing act of BCL-2 family proteins. Nat. Rev. Mol. Cell Biol. 20, 175–193. doi: 10.1038/s41580-018-0089-8
Stewart B. D., Jenkins S. R., Boig C., Sinfield C., Kennington K., Brand A. R., et al. (2021). Metal pollution as a potential threat to shell strength and survival in marine bivalves. Sci. Total Environ. 755, 143019. doi: 10.1016/j.scitotenv.2020.143019
Sun S., Jing Y., Zhang T., Hu F., Chen Q., Liu G. (2024). Effect of salinity on the toxicokinetics, oxidative stress, and metallothionein gene expression in Meretrix meretrix exposed to cadmium. Comp. Biochem. Physiol. Part C 279, 109863. doi: 10.1016/j.cbpc.2024.109863
Vaughn C. C., Hoellein T. J. (2018). Bivalve impacts in freshwater and marine ecosystems. Annu. Rev. Ecol. Evol. Syst. 49, 183–208. doi: 10.1146/annurev-ecolsys-110617-062703
Wang J. H., Deng W. F., Zou T., Bai B. B., Chang A. K., Ying X. P. (2021). Cadmium-induced oxidative stress in Meretrix meretrix gills leads to mitochondria-mediated apoptosis. Ecotoxicology 30, 2011–2023. doi: 10.1007/s10646-021-02465-8
Wang W. C., Mao H., Ma D. D., Yang W. X. (2014). Characteristics, functions, and applications of metallothionein in aquatic vertebrates. Front. Mar. Sci. 1. doi: 10.3389/fmars.2014.00034
Whiteside J. R., Box C. L., McMillan T. J., Allinson S. L. (2010). Cadmium and copper inhibit both DNA repair activities of polynucleotide kinase. DNA Repair (Amst). 9, 8389. doi: 10.1016/j.dnarep.2009.11.004
Wu X., Yalowich J. C., Hasinoff B. B. (2011). Cadmium is a catalytic inhibitor of DNA topoisomerase II. J. Inorg. Biochem. 105, 833838. doi: 10.1016/j.jinorgbio.2011.02.007
Xie J., Fan R., Meng Z. (2007). Protein oxidation and DNA-protein crosslink induced by sulfur dioxide in lungs, livers, and hearts from mice. Inhal Toxicol. 19, 759–765. doi: 10.1080/08958370701399885
Xu P., Chen H., Xi Y., Mao X., Wang L. (2016). Oxidative stress induced by acute and sub-chronic cadmium exposure in the ovaries of the freshwater crab Sinopotamon henanense (DAI 1975). Crustaceana 89, 1041–1055. doi: 10.1163/15685403-00003573
Xu Y. Y., Wu C. H., Jin J. Y., Tang W. H., Chen Y. T., Chang A. K., et al. (2024). Transcriptome analysis and identification of cadmium-induced oxidative stress response genes in different. Meretrix meretrix Dev. stages. Anim. 14, 352. doi: 10.3390/ani14020352
Yang Y. Q., Wang M. C., Yu X. Y., Wei J. Y., Wu S. W., Wu C. H., et al. (2023). Assessment of toxic metal pollution in Yueqing Bay and the extent of metal-induced oxidative stress in Tegillarca granosa raised in this water. Mar. pollut. Bull. 194, 115444. doi: 10.1016/j.marpolbul.2023.115444
Yunus K. (2020). A review on the accumulation of heavy metals in coastal sediment of Peninsular Malaysia. Ecofeminism Climate Change 1, 21–35. doi: 10.1108/EFCC-03-2020-0003
Zeb A., Ullah F. (2016). A simple spectrophotometric method for the determination of thiobarbituric acid reactive substances in fried fast foods. J. Anal. Methods Chem. doi: 10.1155/2016/9412767
Zhan J., Sun T., Wang X., Wu H., Yu J. (2023). Meta-analysis reveals the species-does- and duration-dependent effects of cadmium toxicities in marine bivalves. Sci. Total Environ. 859, 160164. doi: 10.1016/j.scitotenv.2022.160164
Keywords: Meretrix meretrix, cadmium, bioaccumulation, oxidative stress, depuration, apoptosis
Citation: Zhou J, Cai H, Zhong Y, Zheng Y, Wu Y, Chang AK and Ying X (2024) Reversal of cadmium-induced toxicity in Meretrix meretrix as determined by alleviation of oxidative damage following short-term depuration. Front. Mar. Sci. 11:1444061. doi: 10.3389/fmars.2024.1444061
Received: 05 June 2024; Accepted: 05 August 2024;
Published: 22 August 2024.
Edited by:
Sara Zafar, Government College University, PakistanReviewed by:
Noreen Khalid, Government College Women University Sialkot, PakistanRenfei Chen, Shanxi Normal University, China
Kuan Yan, Yibin University, China
Copyright © 2024 Zhou, Cai, Zhong, Zheng, Wu, Chang and Ying. This is an open-access article distributed under the terms of the Creative Commons Attribution License (CC BY). The use, distribution or reproduction in other forums is permitted, provided the original author(s) and the copyright owner(s) are credited and that the original publication in this journal is cited, in accordance with accepted academic practice. No use, distribution or reproduction is permitted which does not comply with these terms.
*Correspondence: Alan Kueichieh Chang, YWtjY2hhbmdAMTYzLmNvbQ==; Xueping Ying, eHB5aW5nMjAwOEB3enUuZWR1LmNu
†These authors have contributed equally to this work