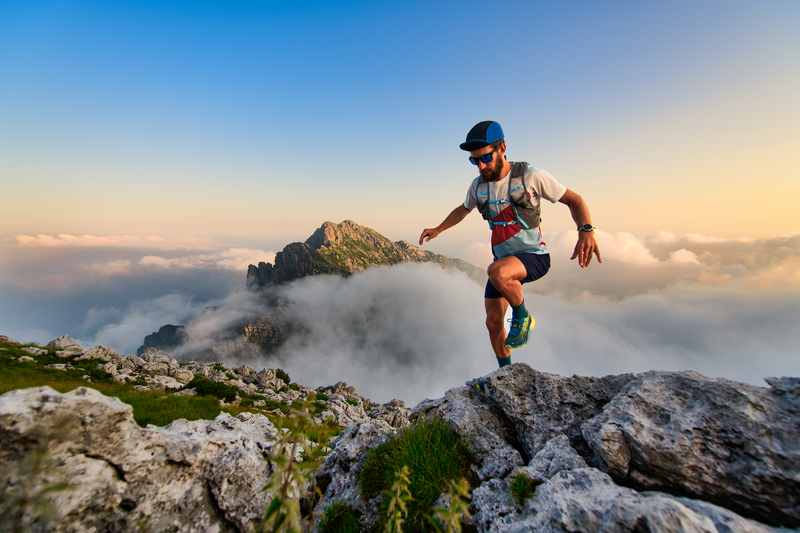
94% of researchers rate our articles as excellent or good
Learn more about the work of our research integrity team to safeguard the quality of each article we publish.
Find out more
ORIGINAL RESEARCH article
Front. Mar. Sci. , 08 May 2023
Sec. Marine Biogeochemistry
Volume 10 - 2023 | https://doi.org/10.3389/fmars.2023.1206776
This article is part of the Research Topic Solute Transport and Transformation Across the Sediment-Water Interface in Coastal Ecosystems View all 7 articles
Coastal wetlands are an important hotspot for nutrient cycling and transport from the land to the ocean. Silicon (Si) as a vital biogenic element affects plant growth and health of coastal ecosystems. The understanding of key factors and processes controlling dissolved silicate (DSi) exchange between the wetlands and coastal water has been limited due to the lack of measured data. We carried out intensive investigations of time-series DSi concentrations and porewater exchange across the Sediment-Water Interface (SWI) along a tidal creek with a mangrove-salt marsh gradient during neap and spring tides in 2020. Seasonal observations of surface water in a tidal creek and Zhangjiang Estuary (Fujian Province, China) were conducted from 2017 to 2020. The results showed that there was a net export of DSi from the mangroves to tidal creek with rates of 2.11 and 2.40 mmol m-2 d-1 in neap and spring tides respectively, suggesting the mangroves served as the source of DSi. However, the salt marshes had a net DSi import with one or two orders of magnitude lower than the export from the mangroves. DSi export across the wetland‐estuarine continuum was largely controlled by porewater exchange, groundwater geochemistry (pH, temperature) and plant root uptake. Groundwater in the mangroves has larger ratios of DSi : DIN (dissolved inorganic nitrogen) (2.5 ± 0.6) and DSi : DRP (dissolved reactive phosphorus) (1257 ± 35) compared with surface water. The net export of DSi from mangroves would modify the nutrient stoichiometry and mitigate the effects of reduced river DSi flux caused by damming on coastal ecosystem. This study provides new insights into the wetland Si cycling for sustaining coastal ecosystem health.
• DSi export from wetlands to estuary driven by porewater exchange was explored.
• Mangroves had a large net export of DSi but salt marshes had a minor import.
• DSi export from mangroves would mitigate effects of reduced river DSi by dam.
Mangroves are known as a critical “blue carbon” system with high productivity of 635 Tg C yr-1 and large carbon storage of 739 Mg ha-1 (Alongi, 2014; Alongi, 2020). Nitrogen (N) and phosphorus (P) cycling and transport in mangroves and adjacent estuary have been well studied, revealing wetland’s positive or negative effects on coastal ecosystems (Wang et al., 2019; Wang et al., 2021). Silicon (Si, the second most abundant element in the earth’s crust) is an important element for wetlands and marine ecosystems (Epstein, 1994; Ma and Takahashi, 2004). Many studies focused on Si concentrations, compositions and their variations in plants and sediments (de Bakker et al., 1999; Elizondo et al., 2021). However, the export-import pattern of dissolved silicate (DSi) in mangrove wetlands was rarely studied. In some parts of the world, such as Asia, Africa, Australia, and N. America, mangroves are invading salt marshes with warming climate (Saintilan et al., 2014). In China, the Spartina alterniflora, a type of salt marshes with high tolerance to salinity and hypoxia, strong capacity for competitiveness, is invading the native mangroves or mudflats, forming a mangrove-salt marsh coexistent ecotone (Li et al., 2009; Zhang et al., 2012; Wang and Lin, 2023). The potential effects of salt marsh invasion on DSi export-import patterns in native wetlands have received little attention.
Wetlands have been recognized as “hot spots” for Si cycling due to the critical role in Si transport and processing (Struyf and Conley, 2009). Plants are efficient in taking up and storing Si, and also release Si into the sediments supporting further biogeochemical cycling (Hou et al., 2010; Liang et al., 2015). Si is involved in the nutrient cycling, organic matter degradation and carbon storage (Xia et al., 2020). This biogeochemical processes about Si in wetlands determined the form and amount available for exchange with coastal ecosystems. The land-ocean fluxes of DSi are controlled by the geochemical, biological, and hydrodynamic processes (Cornelis et al., 2010). The sources or sinks of Si in the wetland ecosystem have been studied with inconsistent conclusion. A study based on sequential extractions of Si and Fe, modeling of the soil aqueous phase, and X-ray diffraction of the fine clay fraction showed that the hypersaline tidal flat soils tend to be the sink of Si due to the adsorption, (co)precipitation, and reactions involving clay minerals (Sartor et al., 2019). The study in Gazi Bay with the growth of mangroves and seagrasses showed that benthic fluxes were the major source of Si4+ with larger fluxes in mangrove than in seagrass, which play a significant role in the functional understanding and protection and restoration of the coastal wetlands (Mwashote and Jumba, 2002). However, the underlying mechanisms controlling DSi export-import pattern in wetlands are still unknown.
Dams are recognized as “in-stream” reactors to impede nutrient transport (Maavara et al., 2020b), altering DSi fluxes along the land-ocean aquatic continuum by retention (Laruelle et al., 2009; Harrison et al., 2012; Chen et al., 2014). A study in the Jiulong River, Southeast China, showed that dam retention caused a 16% decrease in DSi flux (Chen et al., 2014). Studies based on nutrient cycling and mechanistic model predicted that dams will retain 5.3% of global reactive silicon, and more than half of rivers will experience greater removal of Si over N and P by the midcentury (Maavara et al., 2014; Maavara et al., 2020a). The decreased DSi flux from the land to the ocean has altered nutrient stoichiometry and phytoplankton structures, increasing the risk of non-diatom bloom (Li et al., 2019). Our companion research has explored the N and P source-sink pattern in a mangrove-salt marsh-estuary system driven by the porewater exchange and nutrient cycling (Wang et al., 2022b). Under the scenario of a decrease in land-ocean silica fluxes caused by river damming, it is crucial to obtain a better insight into the role of wetlands in the Si transport between land and ocean. Here, we assume wetlands have potential to increase DSi export and the increased fluxes will mitigate the negative effects of reduced global river Si on marine ecosystem caused by worldwide damming.
The porewater or groundwater exchange (PEX) is recognized as a major pathway for material transport from the land to the ocean (Chen et al., 2019; Santos et al., 2019; Santos et al., 2021; Hu et al., 2022). The contributions of Si fluxes driven by PEX to total Si fluxes differed in heterogeneous environments with the contributions of 14-32% in the Jiulong River Estuary, 5% in a salt marsh of the Changjiang River Estuary, China, and 58-90% in the Krka River Estuary characterized as oligotrophy (Wang et al., 2015; Liu et al., 2019; Chen et al., 2021). Tides are recognized as a major control for Si fluxes by affecting hydraulic gradient, hydraulic retention time and wave setup (Moore, 1996; Taniguchi, 2002). Studies based on high-resolution time-series observation suggested the water parameters such as salinity, pH, and redox potential varied with tides, and thus affected the microbial activities, nutrient cycling, the distribution of reduced species and the elemental adsorption and desorption (Taniguchi, 2002; Delgard et al., 2012). The exploration of hydrogeological impacts is crucial to understand Si export or import in wetlands.
Herein, we focused on the spatial and temporal distribution of DSi and vertical DSi fluxes across the Sediment-Water Interface (hereafter “SWI”) in a subtropical mangrove wetland system invaded by the salt marshes. We conducted seasonal observations in the mangrove-salt marsh-tidal creek-Zhangjiang Estuary continuum from 2017 to 2020. Two time-series observations of physicochemical parameters and DSi concentrations in surface water and groundwater were conducted during a spring-neap tidal cycle in 2020. The specific objectives of this study were to: (1) explore the seasonal and spatial variations of DSi concentration across the mangrove-salt marsh creek‐estuary continuum; (2) estimate DSi fluxes across the SWI in the mangroves and salt marshes; (3) reveal the key factors and processes controlling DSi export-import pattern in the mangrove-salt marsh ecotone.
The study was conducted in a National Mangrove Reserve and the adjacent Zhangjiang Estuary (117°24′-117°30′E, 23°53′-23°56′N) in Southeast China (Figure 1). The dominant mangrove species are Kandelia candel, Avicennia marina and Aegiceras corniculatum with a total area of 2.6 km2 (Zhou et al., 2010). The wetlands experience a semidiurnal tide with a tidal range of 0.43-4.67 m, and a subtropical monsoon climate with an annual mean temperature of 22.8 ± 5.7°C and precipitation of 1,679 mm (Wang et al., 2019). The salt marshes (mainly Spartina alterniflora) have invaded mudflat areas with an expansion on the edge of mangroves. Zhangjiang Estuary, connected with wetlands, has a length of 58 km with a watershed area of 855 km2.
Figure 1 Study area and sampling sites (modified from Wang et al., 2022b). (A) The location of the study area to the South China Sea. (B) The sampling sites along the mangrove tidal creek and Zhangjiang Estuary. A line with arrows indicates the cruise track and direction. (C) The time-series observation sites of surface water and groundwater in the mangroves (M, left area of the yellow line) and salt marshes (S, right area of the yellow line).
The seasonal observations in the mangrove-salt marsh creek-Zhangjiang Eestuary continuum were carried out from 2017 to 2020. Surface water samples (0.5 m) were collected along the route from the mangrove-salt marsh creek to the adjacent estuary (Figure 1B). The samples in tidal creek were collected in flood and ebb tides and those in the estuary were collected at high tides (the periods of flood, ebb and high tides were shown in Figure S1). Surface water samples were collected during the monitoring periods using a Niskin hydrophore.
Two fixed sites along the creek edges of the mangroves and salt marshes (Figure 1C) were chosen to conduct time-series measurements (25 h) of surface water (0.5 m) and groundwater during neap tides on 11th-12th October and spring tides on 20th-21st October 2020, respectively. A “paired-wells” device (70 cm) equipped with two CTD-Diver loggers (vanEssen, Netherlands) was installed in the creek edge sediments. Water temperature, conductivity and pressure were recorded using upper (5 cm) and lower (65 cm) loggers at a frequency of 30 minutes to quantify PEX rates (See the schematic diagram of the device in Figure S2). The monitoring began two days after installation to minimize the impact of sediment disturbance. Surface water samples were collected hourly in whole tidal cycles using a Niskin hydrophore. Groundwater samples were collected hourly at low tides using a peristaltic pump.
Water samples were filtrated immediately in the field with a GF/F membrane (0.7 μm) and stored at 4°C until analysis. The concentrations of ammonium (NH4-N), nitrate (NO3-N), nitrite (NO2-N), dissolved reactive phosphorus (DRP) and DSi were analyzed using segmented flow colorimetry (San++ analyzer, Germany) (Detailed about the measurement method are showed in Text S1). Dissolved inorganic nitrogen (DIN) was the sum of NH4-N, NO3-N and NO2-N. Salinity, pH, dissolved oxygen (DO) concentration, and temperature were measured in-situ using a multiparameter portable meter (Multi WTW 3430, Germany).
The DSi offset of a mangrove creek in ebb tides from the conservative mixing line of the estuary was calculated with equations (1) and (2).
where DSiOffset is DSi offset from the mixing lines of the estuary (μmol L-1); a and b is the slope and intercept of the mixing line, respectively; x is the observed salinity in tidal creek in ebb tides; DSiExp and DSiObs is the calculated and observed concentration in tidal creek (μmol L-1), respectively.
Linear regression was carried out to analyze the relationship between DSi offset and tidal range in ebb tides.
The vertical PEX rate was calculated by the hydraulic gradient and vertical hydraulic conductivity, which were based on the generalized form of Darcy’s law and in-situ falling head method, respectively. See more details in supporting information (Text S2).
DSi showed spatial variations in mangrove-salt marsh tidal creek-estuary continuum (Figure 2 and Table 1). The average concentration of DSi in the estuary was 154 ± 37 μmol L-1. In salt marsh creek, the average concentration in flood and ebb tides was similar to or slightly larger than in the estuary. In mangrove creek, the average concentration in ebb and flood tides was 19% and 14% larger than in the estuary.
Figure 2 Diagrams of DSi against salinity (2017-2020). The squares and triangles indicate the concentrations in mangrove (M, blue) and salt marsh (S, red) creek in flood and ebb tides, respectively. The green circles mean the concentrations in Zhangjiang Estuary, showing a conservative mixing (black dotted line) along the salinity gradient.
Table 1 The DSi concentration (Mean ± SD) in mangrove (M) and salt marsh (S)-tidal creek, and estuary in flood and ebb tides.
DSi‐salinity diagrams showed that DSi had a mostly conservative behavior against salinity gradient in the estuary (Figure 2). DSi in mangrove creek in flood tides, and in salt marsh creek in both flood and ebb tides were nearly on the mixing line. In ebb tides, DSi in mangrove creek was larger than those in the estuary at a given salinity with the exceptions in autumn 2017 (October) and 2019 (November). There was a strong positive correlation (R2 = 0.58, p<0.05) between DSi offset and tidal range in the mangrove creek in ebb tides (Figure 3).
Figure 3 The relationship between tidal range and DSi offset from conservative mixing line of estuary in mangrove creek in ebb tides during 2017-2020 (refer to Figure 2). The pink shaded area means 95% confidence band.
Water depth during spring tides (0.68-3.93 m) was larger than during neap tides (0.85-3.28 m) (Figure 4). The average pH values during neap and spring tides of mangrove groundwater (6.86 ± 0.14) were smaller than those of salt marsh groundwater (7.44 ± 0.08). The average temperature of mangrove groundwater (27.5 ± 1.8°C) was higher than of salt marsh groundwater (25.4 ± 1.9°C). The salinity and DO of salt marsh groundwater were larger than those of mangrove groundwater (Figure 4; See more details in Wang et al., 2022b).
Figure 4 Tidal variations of water depth, salinity, DO concentrations, pH values, temperature and DSi concentrations in surface water (grey circles) and groundwater (red circles) in the mangroves and salt marshes during neap tides (11th-12th October 2020) and spring tides (20th-21st October 2020). The data on water depth, salinity and DO was derived from our companion article (Wang et al., 2022b).
The DSi concentrations varied with tidal cycles, water types, and habitats (Figure 4 and Table 2, S1). During neap tides, the average DSi concentration at low tides was 10% higher than at high tides in mangrove surface water (Figure 4), and the average DSi concentration in groundwater was 43% higher than in surface water (Table 2). In the salt marshes, the DSi concentration at low tides was 3% higher than at high tides (Table S1), and the average concentration was 31% higher in groundwater than in surface water (Table 2). During spring tides, DSi concentrations were lower than during neap tides in both the mangroves and salt marshes with an exception in mangrove groundwater, which was 39% higher than during neap tides (Figure 4). The DSi concentration at low tides was 19% and 7% larger than at high tides in the mangroves and salt marshes, respectively (Table S1). In the mangroves, the DSi concentration in groundwater was twice as high as during neap tides, 69% larger than in surface water (Figure 4 and Table 2). In the salt marshes, the DSi concentration in groundwater was similar to in surface water, much smaller than in mangrove groundwater (Table 2).
Table 2 Mean ( ± SD) DSi, ratios of DSi : DIN and DSi : DIP in surface water (SW) and groundwater (GW) in the mangroves (M) and salt marshes (S) during neap tides (11th-12th October 2020) and spring tides (20th-21st October 2020).
The DIN and DRP concentrations varied with water types and habitats (Figure S3). In mangroves, the DIN concentrations in surface water was similar during spring (76 ± 14 μoml L-1) and neap tides (74 ± 7 μoml L-1), 64% and 20% smaller than in groundwater. The average DIN in salt marsh surface water (71 ± 1 μoml L-1) was close to that in mangrove surface water (75 ± 1 μoml L-1), but DIN in salt marsh groundwater (56 ± 20 μoml L-1) was 63% smaller than in mangrove groundwater (152 ± 84 μoml L-1). The DRP concentrations in mangrove groundwater was 5-15 times smaller than in surface water (Figure S3). DRP in surface water and groundwater in salt marshes were lower than that in mangroves during neap tides. During spring tides, DRP was similar in salt marsh surface water and groundwater, both higher than that during neap tides.
The ratios of DSi : DIN (dissolved inorganic nitrogen) ranged from 1.77 to 2.12 and 2.07 to 4.98 in surface water and groundwater, respectively (Table 2). The ratios were 29% and 14% larger in mangrove groundwater, and 57% and 15% larger in salt marsh groundwater than in their surface water during neap and spring tides. The ratios of DSi : DRP (dissolved reactive phosphorus) ranged from 58 to 167 and 89 to 1829 in surface water and groundwater, respectively (Table 2). The DSi : DRP ratios were 96% and 94% larger in mangrove groundwater, and 91% and 30% larger in salt marsh groundwater than in their surface water during neap and spring tides.
The PEX across SWI varied with tidal types and habitats (Figure S2). In the mangroves, the average outflow rates during neap and spring tides were 0.75 and 0.41 mm h-1, much larger than inflow rates (0.20 mm h-1 during both neap and spring tides). In the salt marshes, the outflow rates of groundwater were close to the inflow rates of surface water during spring tides. The inflow rate (0.26 mm h-1) was 42% larger than the outflow rate during neap tide.
The magnitude and direction of DSi fluxes across SWI varied with tidal types and habitats (Figure 5). DSi was exported from groundwater to surface water at low tides, while was imported to groundwater from surface water at high tides in both the mangroves and salt marshes. In the mangroves, DSi had a net export, and the efflux during spring tides (2.40 mmol m-2 d-1) was 10% larger than during neap tides (2.11 mmol m-2 d-1) (Figure 5). In the salt marshes, DSi had a net import, and the influx during neap tides (0.38 mmol m-2 d-1) was larger than during spring tides (0.06 mmol m-2 d-1) (Figure 5), both much smaller than the efflux in the mangroves.
Figure 5 Hourly DSi fluxes (A) and net DSi fluxes (B) across the SWI in the mangroves (orange bars) and salt marshes (green bars) during neap tides (11th-12th October 2020) and spring tides (20th-21st October 2020). The positive and negative values indicate the export of groundwater and the import of surface water, respectively.
Tides are recognized as a key factor controlling material transport from wetlands to coastal areas (Liu et al., 2017; Wang et al., 2019). DSi-salinity relationship showed that DSi in mangrove creek in ebb tides mostly fell above the conservative mixing lines of the estuary while fell on the mixing lines in flood tide (Figure 2). This suggested the groundwater discharge from mangrove sediments affected DSi concentration in the tidal creek in ebb tides, while seawater played a leading role in flood tides. The DSi concentrations in salt marsh creek fell on or slightly above the mixing lines in both flood and ebb tides (Figure 2), suggesting the groundwater discharged from salt marsh sediments played a minor role in the creek water. The significant positive relationship between DSi offset and tidal range in the mangroves suggested the control of tidal pumping on the groundwater discharge from the sediments to tidal creek (Figure 3). This was consistent with a previous study showing that porewater exchange significantly contributes to the mangrove source function of dissolved metal, carbon, and nutrient driven by tidal pumping (Sadat-Noori and Glamore, 2019).
The PEX was controlled by the hydraulic conductivity of sediments and hydraulic gradient (Santos et al., 2014; Oh et al., 2020). Salt marshes had a smaller PEX than the mangroves, and the vertical hydraulic conductivity in the salt marsh was slightly larger but the hydraulic gradient was smaller than in the mangroves (Figure S2), suggesting the hydraulic gradient played a more important role in the salt marsh PEX. The larger hydraulic gradient caused by a larger slope of creek bank (22% larger than the salt marshes) and higher surface elevation resulted in a larger PEX in the mangroves (Figure S2). A larger hydraulic gradient during neap tides explained larger net groundwater efflux in the mangroves. All of these demonstrated the hydrological controls on the PEX difference between the mangroves and salt marshes in this study area.
The DSi concentration in porewater of sediments was the net result of equilibrium between the uptake and restitution of Si (Street-Perrott and Barker, 2008). Plants take up Si from porewater released by weathering of mineral or phytolith and seawater during the submerged periods via the transport by roots and stem, and then stored them as the biogenic silica (BSi) (Raven, 2003; Yang and Zhang, 2018). A substantial reactive BSi can return to wetland sediments with litterfall, increasing DSi concentrations via mineralization and weathering (Cornelis et al., 2011; Elizondo et al., 2021). The monocots are recognized as the typical Si-accumulators due to the strong uptake of Si (Ma and Takahashi, 2002). Previous studies showed wetlands dominated by grasses accumulate large amounts of Si compared to other plants, including the mangroves (Epstein, 2001; Hodson et al., 2005). The salt marshes, one type of monocots, likely had a higher Si uptake than mangrove plants, which resulted in lower DSi concentrations in salt marsh groundwater than in mangrove groundwater (Figure 4 and Table 2). The BSi pool in the sediments includes the phytoliths and micro-organism remnants (Sauer et al., 2006; Aoki et al., 2007). The organic matter contents in salt marsh sediments were smaller than in native mangrove sediments, and the labile compound in the salt marshes was richer than in the mangroves in this study area (Gao et al., 2018; Wang et al., 2022a), which likely resulted in less accumulation of phytoliths, and further led to lower DSi in salt marsh groundwater via weathering (Figure 4 and Table 2).
The dissolution of Si was also determined by environmental factors of sediments or porewater. The pH affect the dissolution of carbonate phase and the liberation of loosely bound silicon (Kellermeier et al., 2012). Previous studies found DSi concentrations increase with pH decrease in the pH range of 4-9 (Qin and Weng, 2006). The significant negative correlation between pH and DSi in the groundwater (p<0.01) demonstrated the strong effects of pH on Si dissolution (Figure S4). Mangroves absorb sulfate ion from sediments during growth and accumulate it in the body as sulfide (Gong and Zhang, 1994). The sulfur accumulated in the sediments with the litter fall degradation can be transferred into hydrogen sulfide (H2S), leading to the decrease of pH in mangrove sediments. The oxidation of pyrite (one of the important products of mangrove residue decompositions) also increased hydrogen ion concentrations of in mangrove groundwater (Ferreira et al., 2007). Furthermore, the mangroves (especially species from the Rhizophoraceae), are characterized by large amounts of tannins (Zhang and Laanbroek, 2020), which was also a key source of acidity substances in mangrove sediments. These explained more acidic environments of mangrove groundwater than salt marsh groundwater, which supported larger DSi concentrations in mangrove groundwater (Figure 4 and Table 2).
The effect of temperature on weathering and dissolution processes is significant (Rickert et al., 2002). The study in the marine system showed that bacterial activities could accelerate BSi dissolution, and this process was closely related to the water temperature (Bidle and Azam, 1999; Bidle et al., 2002). The DSi concentrations were positively correlated to temperature in this study (p<0.01) (Figure S5). Our previous study showed that the inundation time of the mangroves was shorter than salt marshes without obvious difference between neap and spring tides (Wang et al., 2022b). Longer duration of sun exposure of mangrove sediments due to higher elevation and shorter flooding time explained higher temperature in mangrove groundwater (Figure 4), which was conducive to promoting Si dissolution. Moreover, higher temperature promotes microbial activities and respirations in mangrove sediments, which also resulted in lower pH values and larger DSi concentrations than salt marshes (Jin et al., 2013; Liu et al., 2018).
The DSi fluxes were determined by DSi concentrations and PEX across SWI. The mangroves had a net export of DSi during both neap and spring tides (Figure 5) due to larger DSi concentrations in groundwater and larger groundwater outflow than surface water inflow rates (Figure S2). Though a larger hydraulic gradient caused by the low water level resulted in a larger groundwater discharge during neap tides (Figure S2), a 69% larger concentration in groundwater than surface water resulted in a larger net DSi efflux in spring tides than neap tides (Figure 5). In the salt marshes, the minimal difference in DSi concentrations and water flow rates between surface water and groundwater during spring tides caused DSi efflux and influx to be almost in balance. Though DSi concentration in groundwater was greater than in surface water, the surface water inflow was 42% larger than groundwater outflow during neap tides, resulting in a net DSi influx. This suggested that hydrodynamics played a more important role than the biological and chemical processes in salt marshes. The net fluxes suggested that the mangroves acted as a source of DSi while the salt marshes served as a sink in our study area. The positive DSi offset of mangrove creek from the mixing line was another strong evidence for the view that the mangroves mostly served as the source of DSi relative to estuary (Figures 2, 3).
The DSi concentrations showed seasonal variations in mangrove and salt marsh creek (Figure 2) due to the seasonal differences in temperature, rainfall and plant periodicity growth. Our previous study in the same area showed that the nutrient (especially nitrogen) concentrations in tidal creek were affected seriously by the porewater discharged from mangroves in the ebb tides (Wang et al., 2019). The DSi concentrations in mangrove tidal creek showed a similar pattern to nitrogen in this study (Figures 2, 3) in ebb tides, and thus we regarded the creek water in ebb tides as the groundwater, and the creek water in flood tides as the surface water to analyze seasonal DSi fluxes across SWI in the mangroves and salt marshes. The results showed that mangroves always had net DSi effluxes during both neap and spring tides (Figures 6A, B). Salt marshes almost had net DSi influxes with the exception in January, April 2017 and August 2020, and the influxes were one or two orders of magnitude lower than the effluxes in the mangroves. The seasonal export-import pattern was consistent with the pattern derived from time-series observations (Figures 5, 6). Though the uncertainty analysis supported the result that the mangroves were a source while the salt marshes were a sink of DSi, more seasonal field observations are needed in future work to gain more insights into the seasonal DSi source-sink patterns in the wetland-estuarine system.
Figure 6 Estimated vertical fluxes of DSi during neap tides (A) and spring tides (B) based on the seasonal observations in mangrove and salt marsh creek during 2017-2020.
Horizontal advection in the coastal area is recognized as an important contributor to groundwater discharge (Lara et al., 2012; Coluccio et al., 2021). Previous studies found the ratios of the vertical hydraulic conductivity (Kv) to the horizontal hydraulic conductivity (Kh) of the sediments ranged from 2/3 to 4 in coastal areas (Hughes et al., 1998; Xiao et al., 2017). We estimated the horizontal fluxes of DSi using the average ± SD (2 ± 1) of Kv : Kh mentioned above in the mangroves and salt marshes.
Horizontal flux contributed less to the total flux and did not change DSi source-sink pattern of the mangroves (Table S3). DSi was exported to tidal creek from mangrove and salt marsh sediments via the horizontal advection during both neap and spring tides (Table 3). The horizontal flux in the mangroves with a larger slope was greater than in the salt marshes with a smaller slope, which was consistent with a study showing the sloping peatland had a stronger lateral groundwater gradient than the basin peatland (Millar et al., 2018). In the mangroves, the horizontal efflux was much smaller than the vertical efflux, contributing to 23-26% of the total efflux. Nevertheless, the horizontal efflux was almost the same as the vertical influx during neap tides, while 50% greater than the vertical influx during spring tides in the salt marshes (Table 3). As a result, the salt marshes shifted from net import (sink) to net export (source) of DSi. Both the net fluxes with or without horizontal flux in the salt marshes were one or two orders of magnitude smaller than the net efflux of the mangroves, indicating the effects of salt marsh invasion on DSi export from mangrove wetlands to Zhangjiang Estuary is very limited.
Table 3 The horizontal, vertical and total fluxes of DSi across the mangroves, salt marshes and tidal creek interface. The positive and negative values indicate the efflux of groundwater and influx of surface water, respectively.
Not only the nutrient concentration but the nutrient stoichiometry often changes algal cell growth, phytoplankton diversity and abundance, affecting the health of marine ecosystems (Humborg et al., 1997; Garnier et al., 2010; Lin and Lin, 2022). The ratios of DSi : DIN and DSi : DRP observed in this study fell well within the range observed in other estuarine systems (Table 4). However, the observed ratios in the groundwater were larger than in most other studies except for DSi : DIN in Pearl River Estuary, Laizhou Bay and Yeongil Bay. The ratios are much higher than the theoretical Redfield ratio (Si:N:P=16:16:1) (Painter et al., 2017; Wang et al., 2003), suggesting Si is not limiting relative to nitrogen and phosphorus in the study area.
Table 4 Comparisons of DSi : DIN and DSi : DRP ratios in surface water (SW) and groundwater (GW) with previous estuarine studies.
The discharge of aquaculture and domestic wastewater with high nutrients has been a crucial disturbance to the wetland-estuarine system. Excess nitrogen and phosphorus inputs over DSi will decrease the ratios of DSi : DIN and DSi : DRP, leading to a brief frenzy of undesirable algal growth and eutrophication (Billen and Garnier, 1997; Conley, 1999). The groundwater with high DSi concentrations, large DSi : DIN and DSi : DRP ratios was exported from the wetlands to the estuary (Table 2), increasing the contribution to preventing eutrophication in coastal water.
Damming alters the hydrodynamic conditions of the channel, extends the hydraulic retention time, and increases the diatom biomass, thus reducing DSi concentration in downstream (Beusen et al., 2009). The observed results in the Zhangjiang river showed that DSi concentrations in the upper reach of the dam were 9-34% larger than that in the lower reach (Table S2), which suggested the retention of DSi and the decrease in DSi flux from land to ocean. Though nitrogen and phosphorus also can be trapped by dams, the discharge of them caused by the human activities in downstream could compensate for or even exceed the amount of retention (Ittekkot et al., 2000). Nevertheless, there was no compensation to DSi. As a result, the diatoms are increasingly outcompeted by the harmful algaes that do not require silicon to grow. Large export of DSi from the wetlands has the potential to mitigate the negative effects (e.g. eutrophication) on marine ecosystem health caused by dams. Given the large number of dams in operation (Grill et al., 2015; Mailhot et al., 2018), the assessments of the resulting DSi retention and the role of wetlands in alleviating the retention have a great significance in the management and restoration of wetlands at global scales.
The alterations in vegetation habitats had a significant impact on nutrient cycling and transport across the coastal area to the ocean (Carey and Fulweiler, 2014). Nevertheless, DSi fluxes in the salt marshes were one or two orders of magnitude lower than in the mangroves, indicating the salt marshes played a minor role in regulating DSi exchange between wetlands and coastal water in our study. Additional observations in other regional or global scales are needed to evaluate the effects of salt marsh invasion or mangrove expansion on DSi export from the coastal wetlands, which were scarcely studied.
A combination of spatial comparative study, time-series observations, and hydrological identification was used to explore DSi export-import pattern in a mangrove-salt marsh creek‐estuary continuum in southeast China. The DSi spatial (mangrove-salt marsh creek-estuary) and temporal (flood-ebb tides) distributions, and the fluxes across the SWI revealed the mangroves had a net DSi export due to large DSi concentrations in groundwater and larger groundwater outflow than surface water inflow. The salt marshes had a net DSi import due to low DSi concentrations in groundwater and low groundwater outflow rates, half that of surface water inflow rates. Lower pH caused by high contents of sulfur and tannin, higher temperature caused by shorter inundation time, lower absorption capacity of plants, and larger hydraulic gradient in the mangroves explained larger DSi export than the salt marshes. The DSi export-import pattern suggested the mangroves served as the source of DSi while the salt marshes served as the sink. Two orders of magnitude lower influxes in the salt marshes than the effluxes in the mangroves indicated the salt marsh invasion would not change the source function of the native wetlands. The study provides new insights into the potential ecological implications of the ongoing salt marsh invasion on mangrove DSi outwelling. Future work on other mangrove-salt marsh transition wetlands is suggested to explore the global effects of salt marsh invasions on Si cycles.
The mangrove-salt marsh ecotone had a large DSi : DIN and DSi : DRP ratio up to 3.0 ± 1.4 and 1108 ± 758 in groundwater, respectively. Porewater exchange driving the net export of DSi from mangroves would modify the nutrient stoichiometry, reduce the eutrophication potential and mitigate the negative effects of reduced river DSi transport caused by damming on the coastal ecosystem.
The raw data supporting the conclusions of this article will be made available by the authors, without undue reservation.
In this study, FW and NC designed the research and performed data analysis. ZL, YW and RY provided supports for experiments and data collection. FW, ZL and YW carried out the investigation. The manuscript was prepared and written mainly by FW and NC, with input from all coauthors. All authors contributed to the article and approved the submitted version.
This research was supported by the National Natural Science Foundation of China (Nos. 41976138; 42276171).
We thank Shaobin Li for the help in English editing, Junou Du for the help in analyzing DSi concentrations, Mingzhen Zhang, Zetao Wu, Qibiao Yu, Pengbao Wu for the help in field observations, Chenjuan Zheng for the help in organization for field work, the Administrative Bureau of Zhangjiang Estuary Mangrove National Nature Reserve, Yunxiao for the support in research.
The authors declare that the research was conducted in the absence of any commercial or financial relationships that could be construed as a potential conflict of interest.
All claims expressed in this article are solely those of the authors and do not necessarily represent those of their affiliated organizations, or those of the publisher, the editors and the reviewers. Any product that may be evaluated in this article, or claim that may be made by its manufacturer, is not guaranteed or endorsed by the publisher.
The Supplementary Material for this article can be found online at: https://www.frontiersin.org/articles/10.3389/fmars.2023.1206776/full#supplementary-material
Alongi D. M. (2014). Carbon cycling and storage in mangrove forests. Ann. Rev. Mar. Sci. 6, 195–219. doi: 10.1146/annurev-marine-010213-135020
Alongi D. M. (2020). Carbon balance in salt marsh and mangrove ecosystems: a global synthesis. J. Mar. Sci. Eng. 8 (10), 21. doi: 10.3390/jmse8100767
Aoki Y., Hoshino M., Matsubara T. (2007). Silica and testate amoebae in a soil under pine-oak forest. Geoderma 142 (1-2), 29–35. doi: 10.1016/j.geoderma.2007.07.009
Beusen A. H. W., Bouwman A. F., Durr H. H., Dekkers A. L. M., Hartmann J. (2009). Global patterns of dissolved silica export to the coastal zone: results from a spatially explicit global model. Global Biogeochem. Cycles 23, 1–13. doi: 10.1029/2008gb003281
Bidle K. D., Azam F. (1999). Accelerated dissolution of diatom silica by marine bacterial assemblages. Nature 397 (6719), 508–512. doi: 10.1038/17351
Bidle K. D., Manganelli M., Azam F. (2002). Regulation of oceanic silicon and carbon preservation by temperature control on bacteria. Science 298 (5600), 1980–1984. doi: 10.1126/science.1076076
Billen G., Garnier J. (1997). The phison river plume: coastal eutrophication in response to changes in land use and water management in the watershed. Aquat. Microbial Ecol. 13 (1), 3–17. doi: 10.3354/ame013003
Carey J. C., Fulweiler R. W. (2014). Salt marsh tidal exchange increases residence time of silica in estuaries. Limnol. Oceanog. 59 (4), 1203–1212. doi: 10.4319/lo.2014.59.4.1203
Chen X. G., Du J. Z., Yu X. Q., Wang X. X. (2021). Porewater-derived dissolved inorganic carbon and nutrient fluxes in a saltmarsh of the changjiang river estuary. Acta Oceanol. Sinica. 40 (8), 32–43. doi: 10.1007/s13131-021-1797-z
Chen X. G., Wang J. L., Cukrov N., Du J. Z. (2019). Porewater-derived nutrient fluxes in a coastal aquifer (Shengsi island, China) and its implication. Estuar. Coast. Shelf Sci. 218, 204–211. doi: 10.1016/j.ecss.2018.12.019
Chen N. W., Wu Y. Q., Wu J. Z., Yan X. L., Hong H. S. (2014). Natural and human influences on dissolved silica export from watershed to coast in southeast China. J. Geophys. Research-Biogeosci. 119 (1), 95–109. doi: 10.1002/2013jg002429
Cho H. M., Kim G., Kwon E. Y., Moosdorf N., Garcia-Orellana J., Santos I. R. (2018). Radium tracing nutrient inputs through submarine groundwater discharge in the global ocean. Sci. Rep. 8, 1–7. doi: 10.1038/s41598-018-20806-2
Coluccio K. M., Santos I. R., Jeffrey L. C., Morgan L. K. (2021). Groundwater discharge rates and uncertainties in a coastal lagoon using a radon mass balance. J. Hydrol. 598, 15. doi: 10.1016/j.jhydrol.2021.126436
Conley D. J. (1999). Biogeochemical nutrient cycles and nutrient management strategies, developments in hydrobiology; man and river systems: the functioning of river systems at the basin scale. Develop. Hydrobiol. pp, 87–96. doi: 10.1023/A:1003784504005
Cornelis J. T., Delvaux B., Georg R. B., Lucas Y., Ranger J., Opfergelt S. (2011). Tracing the origin of dissolved silicon transferred from various soil-plant systems towards rivers: a review. Biogeosciences 8 (1), 89–112. doi: 10.5194/bg-8-89-2011
Cornelis J. T., Ranger J., Iserentant A., Delvaux B. (2010). Tree species impact the terrestrial cycle of silicon through various uptakes. Biogeochemistry 97 (2-3), 231–245. doi: 10.1007/s10533-009-9369-x
de Bakker N. V. J., Hemminga M. A., Van Soelen J. (1999). The relationship between silicon availability, and growth and silicon concentration of the salt marsh halophyte spartina anglica. Plant Soil. 215 (1), 19–27. doi: 10.1023/a:1004751902074
Delgard M. L., Deflandre B., Metzger E., Nuzzio D., Capo S., Mouret A., et al. (2012). In situ study of short-term variations of redox species chemistry in intertidal permeable sediments of the arcachon lagoon. Hydrobiologia 699 (1), 69–84. doi: 10.1007/s10750-012-1154-5
Elizondo E. B., Carey J. C., Al-Haj A. N., Lugo A. E., Fulweiler R. W. (2021). High productivity makes mangroves potentially important players in the tropical silicon cycle. Front. Mar. Sci. 8. doi: 10.3389/fmars.2021.652615
Epstein E. (1994). The anomaly of silicon in plant biology. Proc. Natl. Acad. Of Sci. Of United States Of America 91 (1), 11–17. doi: 10.1073/pnas.91.1.11
Epstein E. (2001). “Silicon in plants: facts vs. concepts,” In Studies in plant science. Elsevier 8, 1–15. doi: 10.1016/S0928-3420(01)80005-7
Ferreira T. O., Otero X. L., Vidal-Torrado P., Macias F. (2007). Effects of bioturbation by root and crab activity on iron and sulfur biogeochemistry in mangrove substrate. Geoderma 142, 36–46. doi: 10.1016/j.geoderma.2007.07.010
Gao G. F., Li P. F., Shen Z. J., Qin Y. Y., Zhang X. M., Ghoto K., et al. (2018). Exotic spartina alterniflora invasion increases CH4 while reduces CO2 emissions from mangrove wetland soils in southeastern China. Sci. Rep. 8 (1), 9243. doi: 10.1038/s41598-018-27625-5
Garnier J. A., Mounier E. M., Laverman A. M., Billen G. F. (2010). Potential denitrification and nitrous oxide production in the sediments of the Seine River drainage network (France). J Environ Qual. 39 (2), 449–459. doi: 10.2134/jeq2009.0299
Gong Z. T., Zhang X. P. (1994). Mangrove and acid sulphate soils in China (in Chinese). Acta Pedol Sin. 1), 86–94. doi: CNKI:SUN:TRXB.0.1994-01-010
Grill G., Lehner B., Lumsdon A. E., MacDonald G. K., Zarfl C., Liermann C. R. (2015). An index-based framework for assessing patterns and trends in river fragmentation and flow regulation by global dams at multiple scales. Environ. Res. Lett. 10 (1), 15001. doi: 10.1088/1748-9326/10/1/015001
Harrison J. A., Frings P. J., Beusen A. H. W., Conley D. J., McCrackin M. L. (2012). Global importance, patterns, and controls of dissolved silica retention in lakes and reservoirs. Global Biogeochem. Cycles. 26 (2), 1–12. doi: 10.1029/2011GB004228
Hodson M. J., White P. J., Mead A., Broadley M. R. (2005). Phylogenetic variation in the silicon composition of plants. Ann. Bot-london. 96 (6), 1027–1046. doi: 10.1093/aob/mci255
Hou L. J., Liu M., Yang Y., Ou D. N., Lin X., Chen H. (2010). Biogenic silica in intertidal marsh plants and associated sediments of the Yangtze estuary. J. Environ. Sci. 22 (3), 374–380. doi: 10.1016/s1001-0742(09)60118-2
Hu M. J., Sardans J., Le Y. X., Yan R. B., Zhong Y., Huang J. F., et al. (2022). Biogeochemical behavior of p in the soil and porewater of a low-salinity estuarine wetland: availability, diffusion kinetics, and mobilization mechanism. Water Res. 219, 118617. doi: 10.1016/j.watres.2022.118617
Hughes C. E., Binning P., Willgoose G. R. (1998). Characterisation of the hydrology of an estuarine wetland. J. Hydrol. 211 (1-4), 34–49. doi: 10.1016/s0022-1694(98)00194-2
Humborg C., Ittekkot V., Cociasu A., Bodungen B.v. (1997). Effect of danube river dam on black sea biogeochemistry and ecosystem structure. Nature 386 (6623), 385–388. doi: 10.1038/386385a0
Hwang D. W., Kim G. B., Lee Y. W., Yang H. S. (2005). Estimating submarine inputs of groundwater and nutrients to a coastal bay using radium isotopes. Mar. Chem. 96 (1-2), 61–71. doi: 10.1016/j.marchem.2004.11.002
Ittekkot V., Humborg C., Schafer P. (2000). Hydrological alterations and marine biogeochemistry: a silicate issue? Bioscience 50 (9), 776–782. doi: 10.1641/0006-3568(2000)050[0776:Haamba]2.0.Co;2
Jin L., Lu C. Y., Ye Y., Ye G. F. (2013). Soil respiration in a subtropical mangrove wetland in the jiulong river estuary, China. Pedosphere 23 (5), 678–685. doi: 10.1016/S1002-0160(13)60060-0
Kellermeier M., Gebauer D., Melero-Garcia E., Drechsler M., Talmon Y., Kienle L., et al. (2012). Colloidal stabilization of calcium carbonate prenucleation clusters with silica. Advanced Funct. Materials. 22 (20), 4301–4311. doi: 10.1002/adfm.201200953
Kim G., Ryu J. W., Hwang D. W. (2008). Radium tracing of submarine groundwater discharge (SGD) and associated nutrient fluxes in a highly-permeable bed coastal zone, Korea. Mar. Chem. 109 (3-4), 307–317. doi: 10.1016/j.marchem.2007.07.002
Kroeger K. D., Swarzenski P. W., Greenwood W. J., Reich C. (2007). Submarine groundwater discharge to Tampa bay: nutrient fluxes and biogeochemistry of the coastal aquifer. Mar. Chem. 104 (1), 85–97. doi: 10.1016/j.marchem.2006.10.012
Lara M., Peralta G., Alonso J. J., Morris E. P., Gonzalez-Ortiz V., Rueda-Marquez J. J., et al. (2012). Effects of intertidal seagrass habitat fragmentation on turbulent diffusion and retention time of solutes. Mar. pollut. Bulletin. 64 (11), 2471–2479. doi: 10.1016/j.marpolbul.2012.07.044
Laruelle G. G., Roubeix V., Sferratore A., Brodherr B., Ciuffa D., Conley D. J., et al. (2009). Anthropogenic perturbations of the silicon cycle at the global scale: key role of the land-ocean transition. Global Biogeochem. Cycles. 23, 1–17. doi: 10.1029/2008gb003267
Li D. D., Dong M. F., Liu S. M., Chen H. T., Yao Q. Z. (2019). Distribution and budget of biogenic silica in the Yangtze estuary and its adjacent sea. Sci. Total Environment. 669, 590–599. doi: 10.1016/j.scitotenv.2019.03.144
Li G., Li H. L., Wang X. J., Qu W. J., Zhang Y., Xiao K., et al. (2018). Groundwater-surface water exchanges and associated nutrient fluxes in dan’ao estuary, daya bay, China. Continental Shelf Res. 166, 83–91. doi: 10.1016/j.csr.2018.06.014
Li B., Liao C. H., Zhang X. D., Chen H. L., Wang Q., Chen Z. Y., et al. (2009). Spartina alterniflora invasions in the Yangtze river estuary, China: an overview of current status and ecosystem effects. Ecol. Engineer. 35 (4), 511–520. doi: 10.1016/j.ecoleng.2008.05.013
Liang Y., Nikolic M., Bélanger R., Gong H., Song A. (2015). Silicon biogeochemistry and bioavailability in soil, silicon in agriculture: from theory to practice. Springer Netherlands Dordrecht pp, 45–68. doi: 10.1007/978-94-017-9978-2_3
Liang C., Xian W. W. (2018). Changjiang nutrient distribution and transportation and their impacts on the estuary. Continental Shelf Res. 165, 137–145. doi: 10.1016/j.csr.2018.05.001
Lin G. M., Lin X. B. (2022). Bait input altered microbial community structure and increased greenhouse gases production in coastal wetland sediment. Water Res. 218 (3), 118520. doi: 10.1016/j.watres.2022.118520
Liu J. A., Hrustic E., Du J. Z., Gasparovic B., Cankovic M., Cukrov N., et al. (2019). Net submarine groundwater-derived dissolved inorganic nutrients and carbon input to the oligotrophic stratified karstic estuary of the krka river (Adriatic Sea, Croatia). J. Geophys. Research-Oceans. 124 (6), 4334–4349. doi: 10.1029/2018jc014814
Liu Y., Jiao J. J., Liang W. Z. (2018). Tidal fluctuation influenced physicochemical parameter dynamics in coastal groundwater mixing zone. Estuar. coasts. 41 (4), 988–1001. doi: 10.1007/s12237-017-0335-x
Liu Y., Jiao J. J., Liang W., Luo X. (2017). Tidal pumping-induced nutrients dynamics and biogeochemical implications in an intertidal aquifer. J. Geophys. Res.: Biogeosci. 122 (12), 3322–3342. doi: 10.1002/2017JG004017
Ma J. F., Takahashi E. (2002). Soil, fertilizer, and plant silicon research in Japan (Amsterdam: Elsevier).
Ma J. F., Takahashi E. (2004). Effect of silicon on the growth and phosphorus uptake of rice. Plant Soil. 126, 115–119. doi: 10.1007/BF00041376
Maavara T., Akbarzadeh Z., Van Cappellen P. (2020a). Global dam-driven changes to riverine n:P:Si ratios delivered to the coastal ocean. Geophys. Res. Lett. 47 (15), e2020GL088288. doi: 10.1029/2020GL088288
Maavara T., Chen Q. W., Van Meter K., Brown L. E., Zhang J. Y., Ni J. R., et al. (2020b). River dam impacts on biogeochemical cycling. Nat. Rev. Earth Environment. 1 (2), 103–116. doi: 10.1038/s43017-019-0019-0
Maavara T., Durr H. H., Van Cappellen P. (2014). Worldwide retention of nutrient silicon by river damming: from sparse data set to global estimate. Global Biogeochem. Cycles. 28 (8), 842–855. doi: 10.1002/2014gb004875
Mailhot A., Talbot G., Ricard S., Turcotte R., Guinard K. (2018). Assessing the potential impacts of dam operation on daily flow at ungauged river reaches. J. Hydrology-Regional Stud. 18, 156–167. doi: 10.1016/j.ejrh.2018.06.006
Millar D. J., Cooper D. J., Ronayne M. J. (2018). Groundwater dynamics in mountain peatlands with contrasting climate, vegetation, and hydrogeological setting. J. Hydrol. 561, 908–917. doi: 10.1016/j.jhydrol.2018.04.050
Moore W. S. (1996). Large Groundwater inputs to coastal waters revealed by 226Ra enrichments. Nature 380 (6575), 612–614. doi: 10.1038/380612a0
Mwashote B. M., Jumba I. O. (2002). Quantitative aspects of inorganic nutrient fluxes in the gazi bay (Kenya): implications for coastal ecosystems. Mar. pollut. Bulletin. 44 (11), 1194–1205. doi: 10.1016/s0025-326x(02)00176-5
Oh Y. H., Kim D. H., Hwane S., Lee H., Moon S. H., Cho S. Y., et al. (2020). Determining groundwater inflow and Si behavior in a wetland using Rn-222 mass balance and multidisciplinary approach. J. Hydrol. 591, 13. doi: 10.1016/j.jhydrol.2020.125575
Painter S. C., Hartman S. E., Kivimae C., Salt L. A., Clargo N. M., Daniels C. J., et al. (2017). The elemental stoichiometry (C, Si, N, P) of the Hebrides shelf and its role in carbon export. Prog. Oceanog. 159, 154–177. doi: 10.1016/j.pocean.2017.10.001
Prakash R., Srinivasamoorthy K., Gopinath S., Saravanan K., Vinnarasi F. (2021). Approximation of submarine groundwater discharge and allied nutrient fluxes to the bay of Bengal, India using nutrient mass balance. Environ. Earth Sci. 80 (9), 1–22. doi: 10.1007/s12665-021-09669-5
Qin Y. C., Weng H. X. (2006). Silicon release and its speciation distribution in the surficial sediments of the pearl river estuary, China. Estuar. Coast. Shelf Sci. 67 (3), 433–440. doi: 10.1016/j.ecss.2005.11.015
Raven J. A. (2003). Cycling silicon – the role of accumulation in plants. New Phytologist. 158 (3), 419–421. doi: 10.1046/j.1469-8137.2003.00778.x
Rickert D., Schluter M., Wallmann K. (2002). Dissolution kinetics of biogenic silica from the water column to the sediments. Geochim Cosmochim Acta 66 (3), 439–455. doi: 10.1016/s0016-7037(01)00757-8
Sadat-Noori M., Glamore W. (2019). Porewater exchange drives trace metal, dissolved organic carbon and total dissolved nitrogen export from a temperate mangrove wetland. J. Environ. Manag. 248, 1–11. doi: 10.1016/j.jenvman.2019.109264
Santos I. R., Bryan K. R., Pilditch C. A., Tait D. R. (2014). Influence of porewater exchange on nutrient dynamics in two new Zealand estuarine intertidal flats. Mar. Chem. 167, 57–70. doi: 10.1016/j.marchem.2014.04.006
Santos I. R., Chen X. G., Lecher A. L., Sawyer A. H., Moosdorf N., Rodellas V., et al. (2021). Submarine groundwater discharge impacts on coastal nutrient biogeochemistry. Nat. Rev. Earth Environment. 2 (5), 307–323. doi: 10.1038/s43017-021-00152-0
Saintilan N., Wilson N. C., Rogers K., Rajkaran A., Krauss K. W. (2014). Mangrove expansion and salt marsh decline at mangrove poleward limits. Global Change Biol. 20 (1), 147–157. doi: 0.1111/gcb.12341
Santos I. R., Maher D. T., Larkin R., Webb J. R., Sanders C. J. (2019). Carbon outwelling and outgassing vs. burial in an estuarine tidal creek surrounded by mangrove and saltmarsh wetlands. Limnol. Oceanog. 64 (3), 996–1013. doi: 10.1002/lno.11090
Sartor L. R., Graham R. C., Ying S. C., Andrade G. R. P., Montes C. R., Ferreira T. O. (2019). Are hypersaline tidal flat soils potential silicon sinks in coastal wetlands? Geoderma 337, 215–224. doi: 10.1016/j.geoderma.2018.08.028
Sauer D., Saccone L., Conley D. J., Herrmann L., Sommer M. (2006). Review of methodologies for extracting plant-available and amorphous Si from soils and aquatic sediments. Biogeochemistry 80 (1), 89–108. doi: 10.1007/s10533-005-5879-3
Street-Perrott F. A., Barker P. A. (2008). Biogenic silica: a neglected component of the coupled global continental biogeochemical cycles of carbon and silicon. Earth Surface Processes Landforms. 33 (9), 1436–1457. doi: 10.1002/esp.1712
Struyf E., Conley D. J. (2009). Silica: an essential nutrient in wetland biogeochemistry. Front. Ecol. Environment. 7 (2), 88–94. doi: 10.1890/070126
Taniguchi M. (2002). Tidal effects on submarine groundwater discharge into the ocean. Geophys. Res. Lett. 29 (12), 3. doi: 10.1029/2002gl014987
Tao W., Niu L. X., Dong Y. H., Fu T., Lou Q. S. (2021). Nutrient pollution and its dynamic source-sink pattern in the pearl river estuary (south china). Front. Mar. Sci. 8. doi: 10.3389/fmars.2021.713907
Wang X. L., Baskaran M., Su K. J., Du J. Z. (2018). The important role of submarine groundwater discharge (SGD) to derive nutrient fluxes into river dominated ocean margins-the East China Sea. Mar. Chem. 204, 121–132. doi: 10.1016/j.marchem.2018.05.010
Wang F. F., Chen N. W., Yan J., Lin J. J., Guo W. D., Cheng P., et al. (2019). Major processes shaping mangroves as inorganic nitrogen sources or sinks: insights from a multidisciplinary study. J. Geophys. Research-Biogeosci. 124 (5), 1194–1208. doi: 10.1029/2018jg004875
Wang F. F., Cheng P., Chen N. W., Kuo Y. M. (2021). Tidal driven nutrient exchange between mangroves and estuary reveals a dynamic source-sink pattern. Chemosphere 270, 10. doi: 10.1016/j.chemosphere.2020.128665
Wang X. L., Du J. Z., Ji T., Wen T. Y., Liu S. M., Zhang J. (2014). An estimation of nutrient fluxes via submarine groundwater discharge into the sanggou bay-a typical multi-species culture ecosystem in China. Mar. Chem. 167, 113–122. doi: 10.1016/j.marchem.2014.07.002
Wang Q., Li H., Zhang Y., Wang X., Xiao K., Zhang X., et al. (2020). Submarine groundwater discharge and its implication for nutrient budgets in the western bohai bay, China. J. Environ. Radioactivity. 212, 106132. doi: 10.1016/j.jenvrad.2019.106132
Wang B., Lin X. B. (2023). Exotic spartina alterniflora invasion enhances sediment n-loss while reducing n retention in mangrove wetland. Geoderma 431 (7), 116362. doi: 10.1016/j.geoderma.2023.116362
Wang F. F., Song A., Zhang Y., Lin X. B., Yan R. F., Wang Y., et al. (2022a). Saltmarsh sediments with wastewater input emit more carbon greenhouse gases but less N2O than mangrove sediments. CATENA 213, 106205. doi: 10.1016/j.catena.2022.106205
Wang G. Z., Wang Z. Y., Zhai W. D., Moore W. S., Li Q., Yan X. L., et al. (2015). Net subterranean estuarine export fluxes of dissolved inorganic C, N, P, Si, and total alkalinity into the jiulong river estuary, China. Geochim. Et Cosmochim. Acta 149, 103–114. doi: 10.1016/j.gca.2014.11.001
Wang B. D., Wang X. L., Zhan R. (2003). Nutrient conditions in the yellow Sea and the East China Sea. Estuar. Coast. Shelf Sci. 58 (1), 127–136. doi: 10.1016/s0272-7714(03)00067-2
Wang F. F., Xiao K., Santos I. R., Lu Z. Y., Tamborski J., Wang Y., et al. (2022b). Porewater exchange drives nutrient cycling and export in a mangrove-salt marsh ecotone. J. Hydrol. 606, 11. doi: 10.1016/j.jhydrol.2021.127401
Xia S. P., Song Z. L., Van Zwieten L., Guo L. D., Yu C. X., Hartley I. P., et al. (2020). Silicon accumulation controls carbon cycle in wetlands through modifying nutrients stoichiometry and lignin synthesis of phragmites australis. Environ. Exp. Botany. 175, 11. doi: 10.1016/j.envexpbot.2020.104058
Xiao K., Li G., Li H. L., Zhang Y., Wang X. J., Hu W. L., et al. (2019). Combining hydrological investigations and radium isotopes to understand the environmental effect of groundwater discharge to a typical urbanized estuary in China. Sci. Total Environment. 695, 1–15. doi: 10.1016/j.scitotenv.2019.133872
Xiao K., Li H. L., Wilson A. M., Xia Y. Q., Wan L., Zheng C. M., et al. (2017). Tidal groundwater flow and its ecological effects in a brackish marsh at the mouth of a large sub-tropical river. J. Hydrol. 555, 198–212. doi: 10.1016/j.jhydrol.2017.10.025
Yan X. L., Zhai W. D., Hong H. S., Li Y., Guo W. D., Huang X. (2012). Distribution, fluxes and decadal changes of nutrients in the jiulong river estuary, southwest Taiwan strait. Chin. Sci. Bulletin. 7 (18), 2307–2318. doi: 10.1007/s11434-012-5084-4
Yang J. L., Zhang G. L. (2018). Silicon cycling by plant and its effects on soil Si translocation in a typical subtropical area. Geoderma 310, 89–98. doi: 10.1016/j.geoderma.2017.08.014
Zhang J. F., Gao X. L. (2016). Nutrient distribution and structure affect the acidification of eutrophic ocean margins: a case study in southwestern coast of the laizhou bay, China. Mar. pollut. Bulletin. 111 (1-2), 295–304. doi: 10.1016/j.marpolbul.2016.06.095
Zhang Y. H., Huang G. M., Wang W. Q., Chen L. Z., Lin G. H. (2012). Interactions between mangroves and exotic spartina in an anthropogenically disturbed estuary in southern China. Ecology 93 (3), 588–597. doi: 10.1890/11-1302.1
Zhang Q. F., Laanbroek H. J. (2020). Tannins from senescent rhizophora mangle mangrove leaves have a distinctive effect on prokaryotic and eukaryotic communities in a distichlis spicata salt marsh soil. FEMS Microbiol. Ecol. 96 (9). doi: 10.1093/femsec/fiaa148
Zhang Y., Santos I. R., Li H. L., Wang Q. Q., Xiao K., Guo H. M., et al. (2020). Submarine groundwater discharge drives coastal water quality and nutrient budgets at small and large scales. Geochim Cosmochim Acta 290, 201–215. doi: 10.1016/j.gca.2020.08.026
Keywords: nutrient fluxes, mangrove, salt marsh, coastal water quality, Zhangjiang Estuary
Citation: Wang F, Lu Z, Wang Y, Yan R and Chen N (2023) Porewater exchange drives the dissolved silicate export across the wetland‐estuarine continuum. Front. Mar. Sci. 10:1206776. doi: 10.3389/fmars.2023.1206776
Received: 16 April 2023; Accepted: 24 April 2023;
Published: 08 May 2023.
Edited by:
Minjie Hu, Fujian Normal University, ChinaReviewed by:
Xianbiao Lin, Ocean University of China, ChinaCopyright © 2023 Wang, Lu, Wang, Yan and Chen. This is an open-access article distributed under the terms of the Creative Commons Attribution License (CC BY). The use, distribution or reproduction in other forums is permitted, provided the original author(s) and the copyright owner(s) are credited and that the original publication in this journal is cited, in accordance with accepted academic practice. No use, distribution or reproduction is permitted which does not comply with these terms.
*Correspondence: Nengwang Chen, bndjaGVuQHhtdS5lZHUuY24=
Disclaimer: All claims expressed in this article are solely those of the authors and do not necessarily represent those of their affiliated organizations, or those of the publisher, the editors and the reviewers. Any product that may be evaluated in this article or claim that may be made by its manufacturer is not guaranteed or endorsed by the publisher.
Research integrity at Frontiers
Learn more about the work of our research integrity team to safeguard the quality of each article we publish.