- 1Institute for the study of Anthropic Impacts and Sustainability in Marine Environment (IAS), National Research Council (CNR), Genova, Italy
- 2Functional Materials, Department of Applied Physics, School of Engineering Sciences, KTH Royal Institute of Technology, Stockholm, Sweden
Microplastic pollution is receiving increased attention due to the realization of its hazards to aquatic and human life. Researchers across the globe are attempting to remove microplastics before its entry into the ecosystem. Therefore, the present work focused on the removal of microplastic from water and studied the potential risks for marine organisms and the ecosystem. The removal of model microplastics, polypropylene (PP) and polyvinyl chloride (PVC), has been studied by using photo-Fenton process. ZnO nanorods coated with SnOx(x < 2) layer and decorated with zero valent iron (Fe0) nanoparticles was used as heterogeneous catalyst for the removal of the microplastics in continuous water flow device. The obtained results demonstrated that high degradation efficiency of PP and PVC microplastics was achieved in a relatively short time and more than 95% of the average particle volume was reduced after 1 week of irradiation. The environmental impact of the photo-Fenton process of the microplastics degradation was investigated by using an ecotoxicological approach. An ecosafety screening has been performed through a series of experiments (bioassays) under controlled conditions, testing water samples after the photo-Fenton degradation of microparticles using a lab scale device. The ecotoxicological impact has been investigated by applying a battery of certified bioassays (UNI EN ISO/EPA standardized techniques) on aquatic organisms at different trophic levels (bacteria, algae, invertebrates). The results obtained on the three model organisms (A. fischeri, P. subcapitata, and D. magna) revealed no toxic effect for samples collected both before and after the photo-Fenton process, thus showing the absence of toxic by-products development during the degradation process.
Introduction
Interest toward microplastics has increased over the past decade, following a growth in production and subsequent introduction of plastic to the marine environment. It is estimated that 8 million tons of plastic waste enter the ocean each year (Jambeck et al., 2015) and possible outcomes toward more than 690 marine species have been reported (Gall and Thompson, 2015; Lavers and Bond, 2017). Microplastics are represented by plastic particles having a diameter of < 5 mm (Andrady, 2011), some of the microplastics found in the environment derive from the use of personal care products such as cosmetics (Fendall and Sewell, 2009) and man-made plastic fibers such as polyester and additives (Browne et al., 2011; Mato et al., 2001), in other cases, microplastics take origin from the fragmentation of larger plastic pieces (Andrady, 2011). Microplastics direct or indirect (consumption of contaminated preys) ingestion can have chemical, physical and biological impact on organisms (Teuten et al., 2009; Farrell and Nelson, 2013; Wright et al., 2013; Setälä et al., 2014). Different organic pollutants are known to be absorbed on microplastics (i.e., DDT, polychlorinated biphenyls, dioxins, PAHs) also trace element like heavy metals (Brenneke et al., 2016; Gao et al., 2019) and other harmful agents such as pharmaceuticals and pathogenic organisms (Prata, 2018; Bretas-Alvim et al., 2020; Sol et al., 2020). Consequently, through bioaccumulation and biomagnification processes, hazardous pollutants can enter in the human body, with microplastics acting as a vector (Prata et al., 2019; Bretas-Alvim et al., 2020). In the same way, microplastics may act as vectors for pathogens, facilitating the entrance of bacteria and viruses into new habitats and food webs (Zettler et al., 2013). Microplastics, due to their limited size and stable physicochemical properties (Cózar et al., 2014) are difficult to be removed from sewage flows and their degradation is limited.
In the last 5 years, different administrative initiatives have been promoted to reduce microplastics release into the environment. In 2015, the European Commission (EC) presented a comprehensive plan for circular economy (European Commission, 2015). In 2018, the EC launched a strategy for reducing plastic (European Commission, 2018a,b). In many countries, national initiatives for the reduction of the use of plastic have been adopted or are in preparation (Karbalaei et al., 2018). The European Union Marine Strategy Framework Directive (EU MSFD) defines microplastics as a pollutant and requires all member states to promote and implement mitigation measures by 2020 (European Commission, 2018c).
The interest toward microplastics discharged from wastewater treatment plants (WWTPs) is quite recent, even if representing one of the routes by which microplastics reach marine environments. Treated wastewater effluents may importantly contribute to increase aquatic and marine microplastics presence (Sundt et al., 2014; Hashmi, 2021). WWTPs represent focal points in concentrating large amounts of microplastics from urban sources acting as a gateway for microplastics from domestic, commercial, industrial and other sources. Although large quantities of microplastics are removed from the treated wastewater (Koelmans et al., 2019) and retained in sewage sludge, wastewater treatment does not target to microplastics specifically (Irfan et al., 2020). As a consequence, most WWTPs discharge effluents containing microplastics into rivers, lakes and groundwater, with a final destination represented by marine environment.
Up to now, no policies or regulations requiring the removal of microplastics during wastewater treatment have been adopted, but as the interest on microplastics pollution raises, the search for wastewater technologies able to capture particles before they reach surface waters has begun to attract attention. So far, microplastics are included within the Marine Strategy Framework Directive but not within the Urban Wastewater Treatment Directive that is the basis of the regulation for most European WWTPs (SAPEA, 2019). A recent literature research revealed that globally no jurisdiction about the maximum level of microplastics permitted in discharged wastewater has been noticed.
Most commonly used treatment technologies applied in WWTPs are represented by primary treatment processes (primary settling treatment, grit and grease treatment), secondary treatment processes (A2O, biofilters or other bioreactors) and tertiary treatment processes (UV, ozonation, chlorination, membrane bioreactors (MBR), rapid sand filters (RSFs), and micro-nano filtration) (Talvitie et al., 2017).
Recently, reviews summarizing data on the efficiency of WWTPs in removing microplastics have been published (Cristaldi et al., 2020; Iyare et al., 2020; Liu et al., 2021). In general, WWTPs located in different continents (Europe, Asia, and North America) mostly use a primary and secondary type of treatment that allows to obtain a high percentage of microplastics removal from wastewater. According to the literature, it appears that most of the WWTPs investigated display a microplastics removal efficiency with values ranging from 78 to 98% for primary treatments and from 7 to 20% for secondary treatments, however, millions of microplastics continue to be released every day into the aquatic environment. Treatment processes adopted in WWTPs are able to remove substantial quantities of larger microplastics particles but are inefficient in removing smaller particles, having dimension of less than 100 μm; moreover, the particles released in treated wastewater are usually smaller and contain a high proportion of fibers, which represent the most hazardous microplastics typology toward planktonic species and live stages at the base of aquatic food webs. In Freeman et al. (2020), a review of the existing technologies for the removal of microplastics within WWTPs is presented, showing that there is a lack of methods able to remove efficiently very small plastic particles and fibers in a way that is technically, environmentally and economically sustainable in industrial-scale wastewater treatments.
Advanced oxidation processes (AOPs) including Fenton reaction, ozonation or electrochemical treatment are applied in the degradation of organic waste in water (Nakata and Fujishima, 2012) and recently have been extended to microplastic remediation (Hu et al., 2021). In principle, the combination of photocatalysis (Tofa et al., 2019a,b; Uheida et al., 2021) and Fenton processes (Hamd and Dutta, 2020) could lead to a faster photogenerated electron production, and the rapidly transferred electrons to iron reduce its state from Fe3+ to Fe2+ leading to the charge separation and resultant Fenton processes. The regenerated Fe2+ increases a fast production of strong hydroxyl radicals (•OH) leading to a rapid degradation of the organic molecules. Therefore, Fenton and photo-Fenton processes are considered to be highly efficient, feasible to control, and affordable (reactions 1–3).
However, the disadvantages of using the classical homogeneous Fenton process including low working pH (pH < 4), significant generation of iron sludge, and large amounts of Fe2+ are required, leading to the proposal of heterogeneous processes overcoming the drawbacks of the homogenous Fenton reactions (Li et al., 2015).
Therefore, in this work, the degradation of microplastics was performed using heterogeneous photo-Fenton reaction utilizing solid catalyst consisting of zero valent iron oxide (Fe0) nanoparticles as a source of iron ions (Fe2+) and zinc oxide nanorods coated with tin chloride (ZnO/SnOx) to generate electrons as a result of visible light absorption in order to facilitate the recycling of Fe2+ and promotion of the Fenton cycle (Fe3+ + e−→Fe2+) in the presence of hydrogen peroxide (H2O2) and the oxidation reactions. According to our knowledge, the published literature work focused mainly on presenting data about technologies adopted for microplastics photocatalytic degradation (Tofa et al., 2019a,b; Ariza-Tarazona et al., 2020; Llorente-Garcia et al., 2020; Nabi et al., 2020; Hu et al., 2021), so far no studies to assess the toxicity of the degradation by-products have been conducted, which is highly recommended as it represents a knowledge gap for microplastics degradation studies, as reported in the review recently published by Du et al., 2021. In this work we have extended the photocatalytic reactions that are suitable for the degradation of microplastics, by introducing the Fenton reactions through smart design of the catalysts. Since iron nanoparticles are present in the reactor, and as hydrogen peroxide was used for the Fenton reaction, necessity for toxicity determination was essential to reduce any potential risks to human or aquatic environments. The presence of harmful by-products in water samples collected after the degradation of PP and PVC microplastics using a lab scale device was evaluated by applying a battery of certified bioassays (UNI EN ISO/EPA standardized techniques) on aquatic organisms at different trophic levels (bacteria, algae, invertebrates). The aim of this work has been the ecotoxicological screening to validate the potential use of the photo-Fenton process, by excluding any toxicity effect on aquatic environment.
Materials and Methods
Materials
PP microplastics with an average particle size of 155 ± 1.4 μm were provided by PPPolymer AB, Sweden (ELTEX® P HV001PF polypropylene supplied in the form of un-stabilized free flowing powder. Melt flow rate = 10 g/10 min, density = 905 Kg/m3, Flexural modulus = 1,600 MPa). Stabilizer free PVC (molecular weight Σ43,000) with an average particle size of 73 ± 0.5 μm was purchased from Sigma Aldrich. The glass fibers substrate (diameter ∼16 μm), was purchased from Sigma Aldrich. Zinc nitrate hexahydrate [Zn (NO3)2.6H2O; Sigma Aldrich], hexamethylenetetramine [(CH2)6N4; Sigma Aldrich], zinc acetate dehydrates [Zn (CH3COO)2⋅2H2O; Sigma-Aldrich], tin chloride (SnCl2), iron sulfate (FeSO4.7H2O), hydrogen peroxide (30%, sigma Aldrich) and sodium borohydride (NaBH4) were used as received without further purification. High purity water with a resistivity of 18 MΩ⋅cm was used throughout all the experiments.
Fabrication of ZnO NRs/SnO2/Fe0 Nanorods Catalyst
In this work the degradation of PP and PVC microplastics was investigated using ZnO nanorods (NRs) coated with SnOx layer and decorated with Fe0 nanoparticles (NPs) in the presence of H2O2 and visible light system. The ZnO NRs/SnOx/ Fe0 NPs composite was then immobilized on glass fibers substrate in order to tarp the circulated microplastics particles suspended in water. The growth of ZnO NRs on the glass fibers is described elsewhere (Uheida et al., 2021). In brief, ZnO nanocrystallite seeds were deposited on glass fibers substrates of about 1 g, pre-heated to 350 C, by spraying 20 ml of zinc acetate dehydrate solution with concentration of 10 mM at flow rate of about 1 ml/min. The hydrothermal method was used for the growth of ZnO NRs on the glass fibers (Kitsomboonloha et al., 2009). The growth was carried out by placing the seeded glass fibers substrates in a chemical bath containing a solution of zinc nitrate hexahydrate and hexamethylenetetramine with concentrations of 10 mM, at 90°C for 9 h (Kitsomboonloha et al., 2009). The coated glass fibers were then washed with deionized (DI) water and annealed at 350 C for 1 h (Bora et al., 2017).
The preparation of ZnO NRs coated SnOx as follow; the glass fibers coated with ZnO NRs was placed into autoclave containing 75 ml SnCl2 (0.l mM) at pH 5 and the autoclave was heated at 180 C for 15 min (Kumar et al., 2021). The glass fibers was then washed with water several time and air dried. The decoration of Fe0 NPs on ZnO/SnOx NRs was carried out as follow (Raji et al., 2021); the glass fibers containing ZnO NRs/SnOx was placed into 0.6 mM solution of iron sulfate (FeSO4.7H2O) and the mixture was then sonicated for 15 min. The glass fibers were washed with water and then air dried. Afterward the fibers were placed into 1.2 mM solution of sodium borohydride (NaBH4) and sonicated for 15 min. The nanocoated glass fibers was washed with water and then air dried.
Degradation Experiments
The lab scale device implemented in this study developed from our previous research as shown in Figure 1 (Uheida et al., 2021). A known amount (∼20 mg) of the microplastics (PP/PVC) was suspended in diluted H2O2 solutions in a recipient (35 mM H2O2 solution reservoir). According to the literature, the highest concentration of microplastics in seawater in different locations was reported to be Σ102 particles/Liter (Leslie, 2014). On the other hand, the average concentration of microplastics entering WWTPs was reported to be Σ15.7 particles/liter (Murphy et al., 2016).
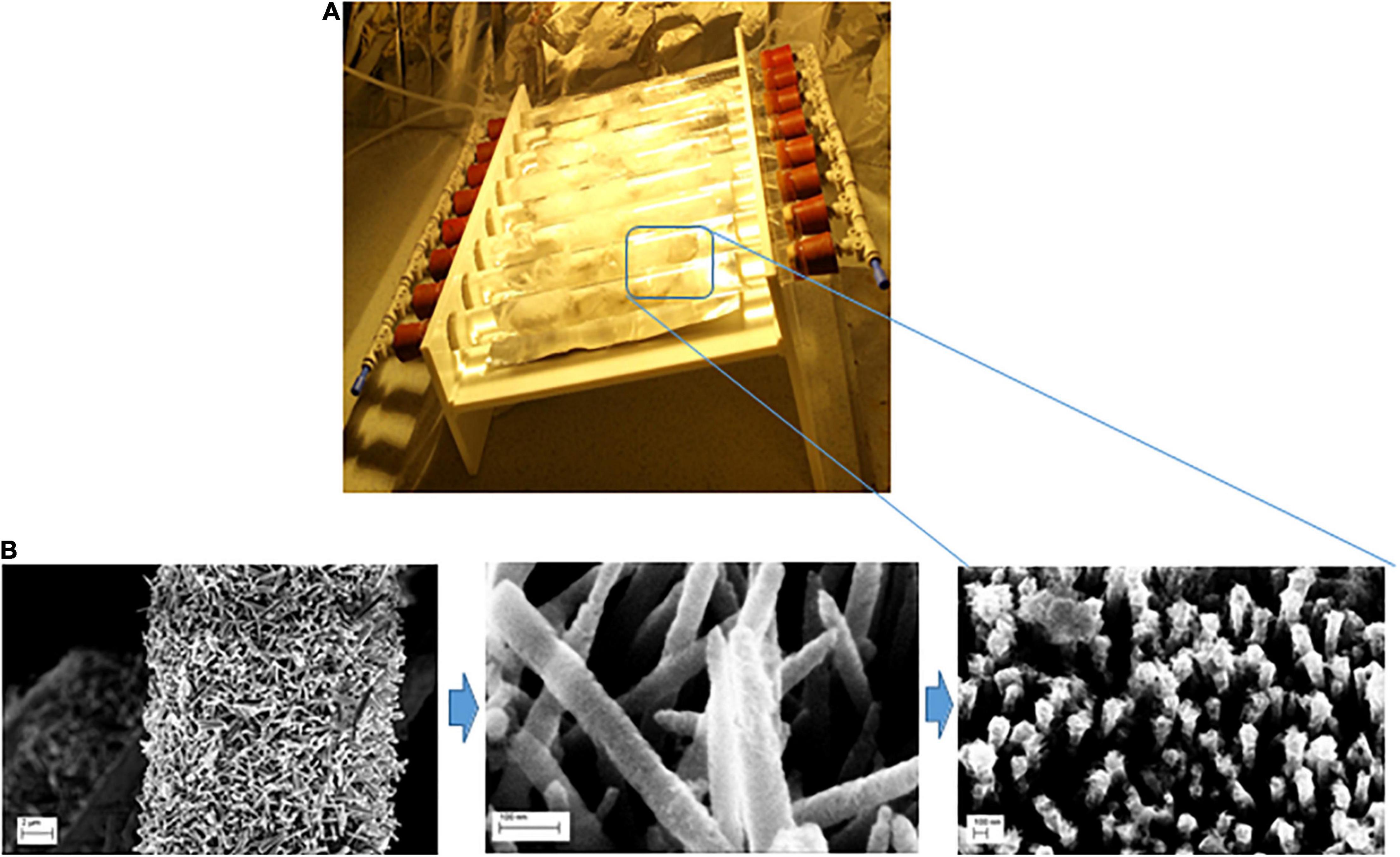
Figure 1. (A) Schematic diagram of the photocatalytic reactor used in this work (Uheida et al., 2021); (B) the fabrication process of ZnO NRs coated with SnO2 layer and decporated decorated with Fe0 NPs grown on glass fibers.
In this experiment, solutions containing microplastic particles with concentration of ∼19 particles/liter (almost corresponding to “real case” conditions) was pumped through the lab scale device using peristaltic pump (Masterflex, Cole-Parmer, United States) at a flow rate of 300 ml/min. The nanocoated material were exposed to visible light using a tungsten-halogen lamp of 120 W (ES-HALOGEN) with light intensity of about 60 mW/cm2 measured by a power meter (IM-750) at a distance of 20 cm from the light source. Microplastics particles were collected from the glass fibers over a period of irradiation time. The particles were then washed with water and air dried prior to further analysis.
Water samples were also withdrawn from the lab scale device at different time intervals (15 and 30 days) of light exposure prior to the ecotoxicity screening. A clean deionized water without microplastics particles (control water samples) subjected to the same degradation process as water samples with microplastics, were collected and tested for toxicity. The samples subjected to bioassays are listed in Table 1.
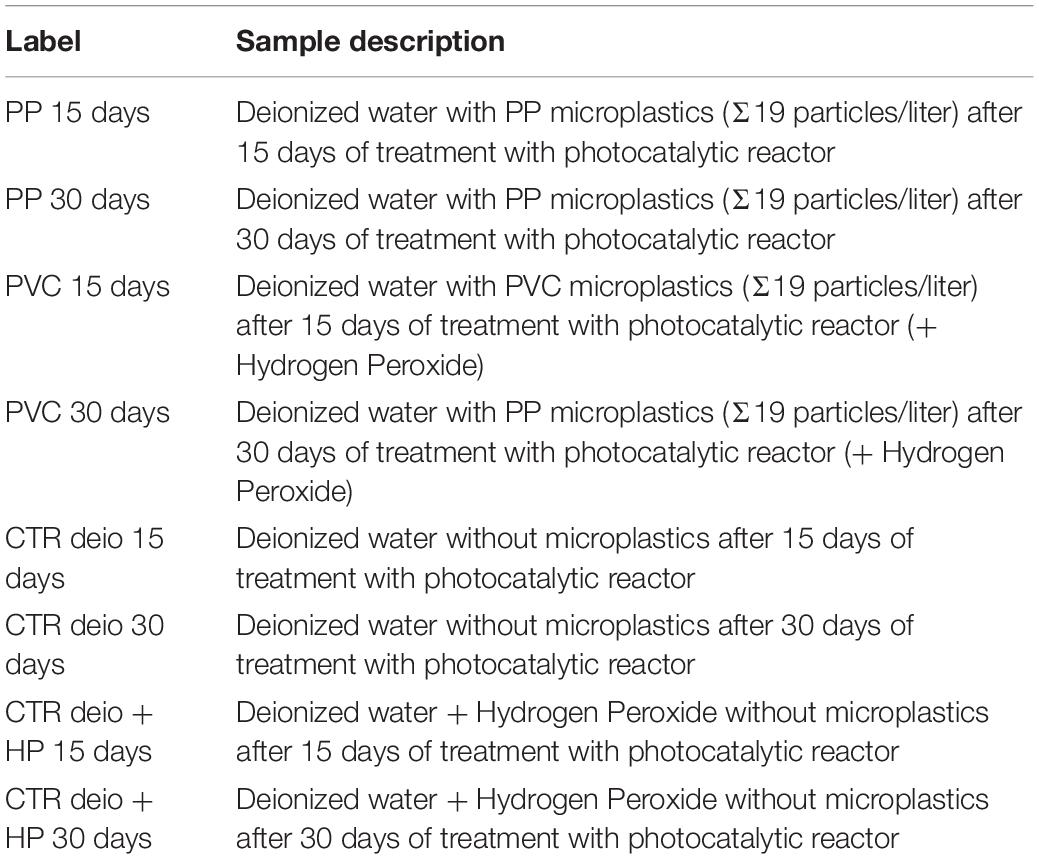
Table 1. List of samples (containing in pre-treatment water a microparticles concentration corresponding to “real case” conditions: Σ19 particles/liter) used for the ecosafety screening of photocatalytic reactor.
The degradation performance of PP and PVC microplastics using ZnO/SnOx/Fe0 NPs/H2O2/visible light system was evaluated and the results obtained were analyzed by monitoring the changes in FTIR spectra in the range 4,000−650 cm–1 with signal averaged over 32 scans at a resolution of 4 cm–1 using Nicolet iS10 spectrophotometer (Thermo Fisher Scientific, United States).
The microplastics average particle size and the diameter of the glass fibers were measured using Leica DML standard optical microscope (Leica Microsystems, Wetzlar, Germany) connected to a digital camera which captured the images. After photodegradation, sample of microplastics particle were collected and dried in air prior to characterization using the optical microscope. The particle size distribution was determined using image analysis software (ImageJ, version k 1.45). The average microplastic particles size was estimated from a sample of 200 particles. Based on the obtained particle sizes, the particle volume reduction percentage was calculated using the following equation;
Scanning electron microscopy (SEM) and energy-dispersive X-ray (EDX) analyses were performed using a GEMINI Ultra 55 electron microscope (Carl Zeiss AG, Germany). SEM was used to confirm the attachment of ZnO NRs to the glass fibers and the presence of Fe0 nanoparticles on the surface of ZnO NRs as well as the microplastics particles morphology analysis. After photodegradation, the microplastics particles were extracted from the glass fibers substrates and dried in air prior to loading in the scanning electron microscope. The microplastic particles were placed on conductive carbon tapes which was stuck on a SEM sample stub. Then the stub with mounted microplastics particles were coated by sputtering a thin layer of gold using JFC-1100 sputtering unit (JEOL Nordic AB) to avoid charging during electron microscopy. Sputtered gold was deposited for 2 min at 1.2 kV and 10 mA.
Ecotoxicity Screening
Aliivibrio fischeri Acute Toxicity Test
The environmental compatibility of the lab scale device was tested by exposing water samples (as listed in Table 1) to Aliivibrio fischeri (also called Vibrio fischeri), a bioluminescent bacterium found globally in marine environments. This bioassay measures the acute toxicity, the end point observed is represented by the percent of photoluminescence inhibition (vs. control sample) of the photobacterium A. fischeri. A commercial test kit according to the UNI EN ISO 11348-3: 2019 test protocol was adopted. The bacteria were stored at −20°C prior testing and activated by hydration following the standard operation procedure of the kit. All the samples were kept on a thermostatic plate at 15°C throughout the entire test, three replicates for each sample were performed. Solution of NaCl (Sigma-Aldrich, Steinheim, Germany; purity 99.9%, 20 g L−1) was used as negative control and 3-5-dichlorphenol (purchased by Agilent Technologies; CAS# 000591-35-5) was used as positive control.
Bioluminescence was measured after 30 min of exposure to samples by using the luminometer Microtox®M500. Samples were considered toxic when 50% reduction (EC50) of the light output (vs. control) was obtained.
P. subcapitata Chronic Toxicity Assay
Since photosynthetic organisms are primary producers, this bioassay was included in the bioassay battery in order to perform a complete toxicity screening. The algal growth inhibition bioassay with a freshwater microalgae was preformed, using stock culture P. subcapitata (previously known as Selenastrum capricornutum) from a commercial toxicity test kit. The assay was performed according to the standardized protocol EN-ISO-8692:2012 (Water Quality-Fresh water algal growth inhibition test with unicellular green algae), by using the commercial Algaltoxkit F™ (purchased by MicroBio Tests Inc.). The following experimental parameters were adopted: T = 22 ± 1°C, pH = 8.1 ± 0.2, continuous side illumination with cool white light (5,000–6,000 lux). Experiments were performed in triplicates in 25 mL incubation vials with an initial algal cell count of 104 cells/mL. For this bioassay, the measured effect (end-point) was the alteration (respect to a control) of algal growth after being exposed for 72 h to tested samples (listed in Table 1). Algal biomass (optical density) was measured in 10 cm path-length cuvettes at 670 nm, using a Onda UV-30 Scan model spectrophotometer.
D. magna Acute Toxicity Assay
Besides bacteria and microalgae, a third ecotoxicological bioassay was performed with the freshwater crustacean D. magna, in order to complete the battery of organisms belonging to different trophic levels. D. magna acute toxicity bioassay represents a standard test in the United States Environmental Protection Agency (EPA), Organization for Economic Cooperation and Development (OECD), and International Organization for Standardization (ISO) protocols. Dormant eggs (ephippia) of the freshwater crustacean D. magna from a commercial kit (Daphtoxkit F™, purchased by MicroBio Tests Inc.) were used. The end point of this acute toxicity test is the determination of percent death and/or immobilization rates being observed for D. magna after an incubation time of 24 and 48 h. According to the ISO 6341 (1996) and the OECD protocols, crustacean neonates (5 daphnid neonates per test cell) were exposed to samples (listed in Table 1) and incubated in the dark at T = 20°C four replicates were performed for each sample and for control samples. After 24 and 48 h, immobility percentage was assessed, organisms were considered as immobile when they did not show any movement for 15 s of observation (after a gentle agitation of the solution). The test is considered valid when in the test control group, the immobilization/mortality of daphnids do not exceed 10%.
Results
Microplastics Degradation
In this work, the degradation of PP and PVC microplastics were investigated using heterogeneous photo-Fenton reaction system consisting of composites in the presence of H2O2 and visible light. The proposed microplastics degradation system utilizes the advantages of ZnO NRs and Fe0 NPs composites, which has the capability of absorbing light wavelengths within most of the solar spectrum, and carry out electron transfer processes. In addition, the advantages of using Fe0 NPs in the photo-Fenton process include large surface area, fast kinetics and high reactivity. Fe2+ is released upon oxidation of Fe0 NPs in acidic solutions to initiate the Fenton process in the presence of H2O2, followed by reaction of the generated Fe3+ with Fe0 NPs in order to prevail a continuous supply of Fe2+. Furthermore, the produced Fe3+ may capture the excited e– in the conduction band of ZnO and regenerate Fe2+, thus promoting the closed cycle of catalysis and Fe restoration (reactions 5–7) (Raji et al., 2021).
The ZnO NRs decorated with Fe0 NPs composites were immobilized on glass fiber substrate in order to trap the microplastics particles which in parallel support the ZnO/Fe0 and to prevent undesirable release of Fe0 NPs to the environment.
The SEM images of the fabricated ZnO NRs coated with SnOx layer and decorated with Fe0 NPs immobilized on the glass fibers are shown in Figure 2. The glass fibers supports of diameter ∼16 μm were coated with ∼1.6 μm long and ∼200 nm wide ZnO NRs. In addition, Energy-dispersive X-ray (EDX) spectroscopy image (Figure 2) shows the homogeneous distribution of ZnO, Fe0 NPs, and SnOx. The EDX spectroscopy image shows each detected Fe atom (middle image) on surface of the ZnO/SnOx/Fe0 glass fiber sample. The homogeneous spread of the dots (white) indicate a homogenous distribution of the iron particles on the ZnO/Snx nanorods although in a small concentration.
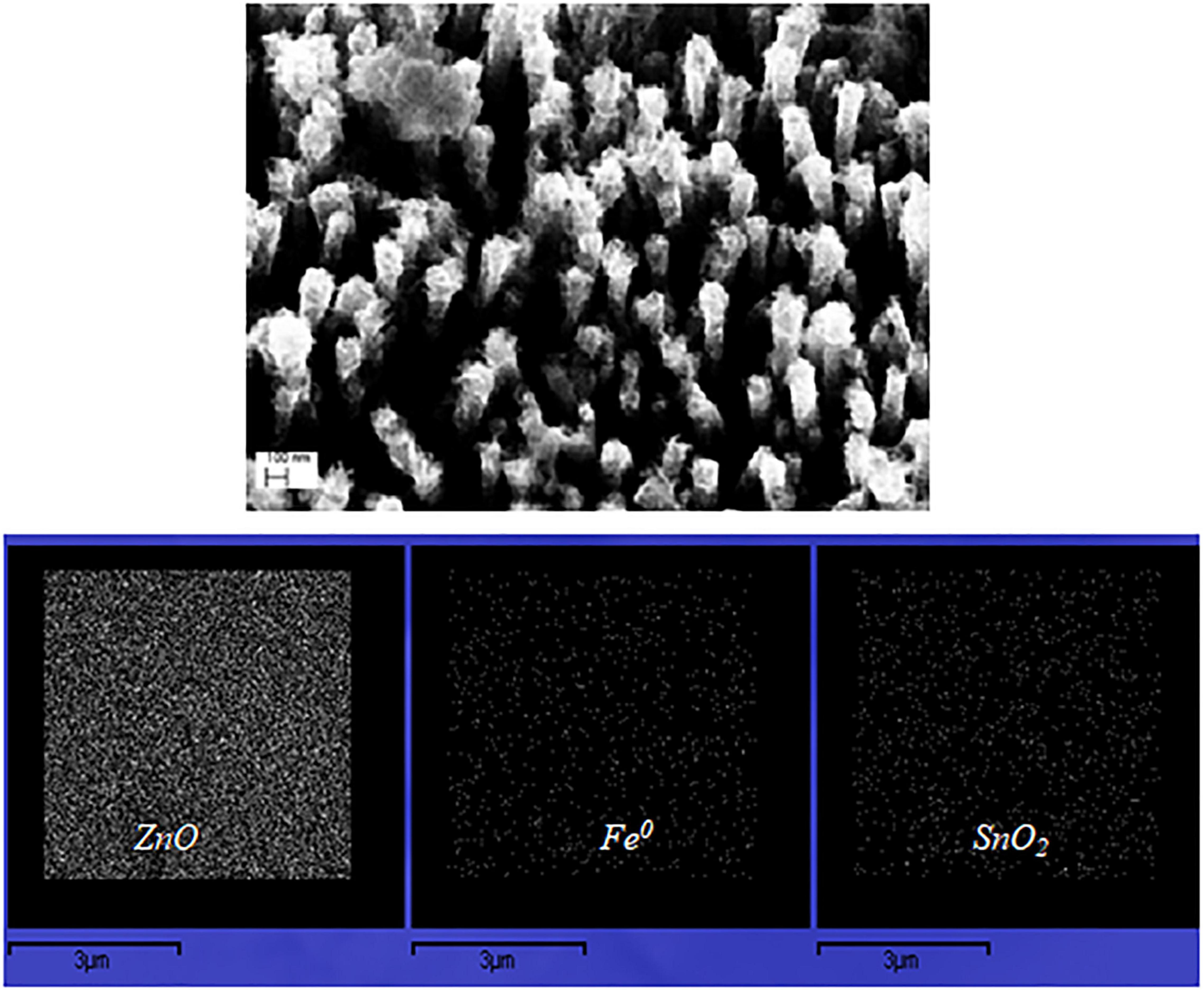
Figure 2. SEM image of ZnO NRs coated with SnO2 layer and decorated with Fe0 NPs; and EDX image of the distribution of ZnO, SnO2, and Fe0 NPs.
The degradation performance of PP and PVC microplastics using ZnO/SnOx/Fe0 NPs/H2O2/visible light system was evaluated and the results obtained were analyzed by monitoring the changes in FTIR spectra and the microplastics average particle size. Figures 3, 4 show the obtained FTIR spectra for PP and PVC microplastics, respectively, after visible light irradiation at different time intervals. It can be seen clearly that by exposing PP and PVC microplastics to visible light over a period of time leads to changes in FTIR spectra. The photodegradation of PP microplastics (Figure 3) has been characterized by the formation of carbonyl (C = O) and hydroxyl (−OH) groups. The absorbance at 1,725 and 3,500 cm–1 assigned to carbonyl and hydroxyl groups, respectively, while the absorbance at 2,722 cm–1 is associated with CH bending and CH3 stretching (Aslanzadeh and Haghighat, 2010; de Carvalho et al., 2013). It can be seen from Figure 3 that the increase in exposure time of ZnO/SnOx/Fe0 NPs in the presence of H2O2 caused an increase in the peak intensities of C = O and −OH groups. The obtained results suggest that the major oxidation products may include hydrogen-bonded hydroperoxides and carbonyl compounds (Verma et al., 2017).
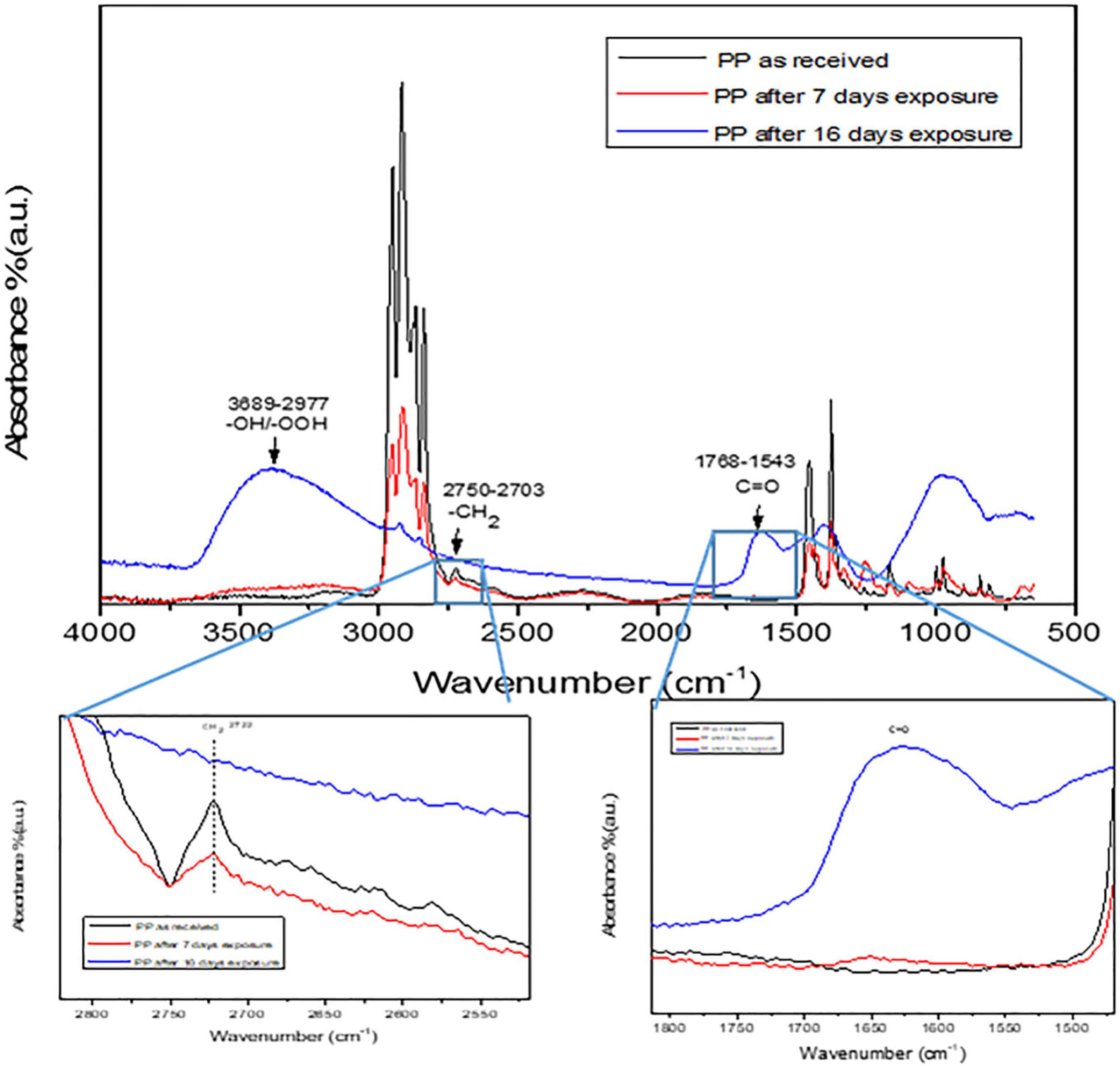
Figure 3. FTIR spectrum of PP microplastics degradation using Photo-Fenton process. The carbonyl and carboxyl groups are highlighted in the spectra.
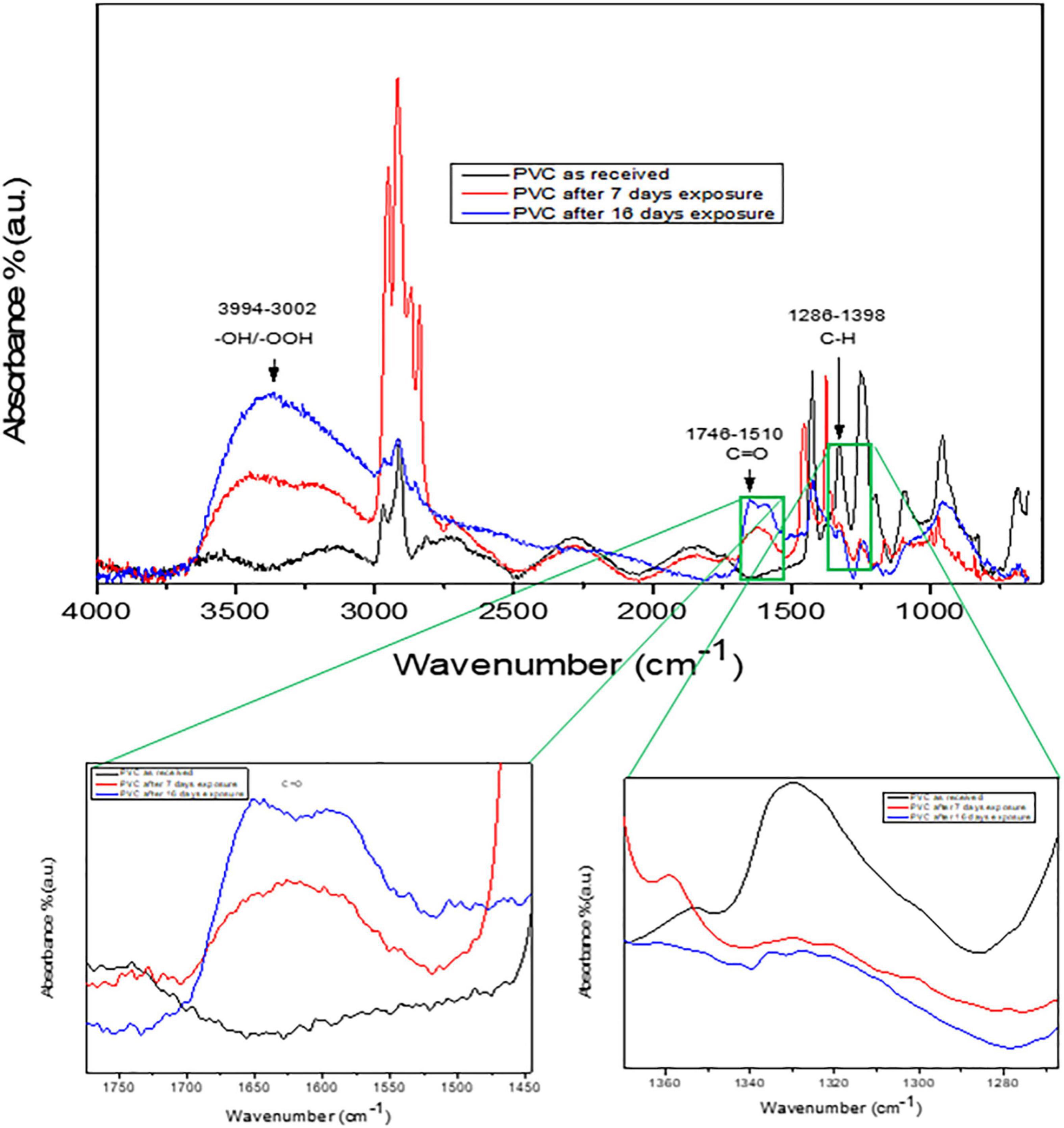
Figure 4. FTIR spectrum of PVC microplastics degradation using Photo-Fenton process. The carbonyl and carboxyl groups are highlighted in the spectra.
Figure 4 shows the FTIR spectra of as received PVC microplastics and upon extended exposure to visible light irradiation. As shown in Figure 4, the absorption bands in the range of 1,746–1,510 cm–1 can be associated to the arise from C = O groups. The absorbance at 1,631 and 3,400 cm–1 may attributed to the formation of a C = C bond conjugated to the C = O group (Ramesh et al., 2011). In Figure 4, the absorbance percent of the peak around 1,700 cm–1 was found to increase indicating the prevalence of aldehyde or carboxylic acid groups. Furthermore, the peak intensity at 2,849 and 966 cm–1 decreased after visible light exposure that is an indicative of the loss of alkane and alkene, respectively, suggesting the scission of polymeric chains of PVC microplastics (Yousif et al., 2015).
Figure 5 shows the optical microscope images and analyzed particle size distribution of PP and PVC microplastics before and after light exposure. The size of the as received PP and PVC microplastics was measured to be 155 ± 1.4 μm and 73 ± 0.5 μm, respectively (over 200 particles were used for the analysis). The particle size of PVC microplastics after 7 days of irradiation was found to be 28 μm, and on the other hand, PP microplastics particle size was reduced to 52 μm. The percentage reduction of microplastics particle volume was calculated to be 94 and 96% for PVC and PP microplastics, respectively, after 7 days of photo-Fenton reaction. It is most likely that elimination of the by-products formed due to the degradation of microplastics contributed to the formation of unoccupied spaces that resulted in the reduction of the particle volume and depletion in the surface layer as we have also observed during the photocatalytic degradation of low density polyethylene (LDPE) (Aslanzadeh and Haghighat, 2010; Tofa et al., 2019a,b).
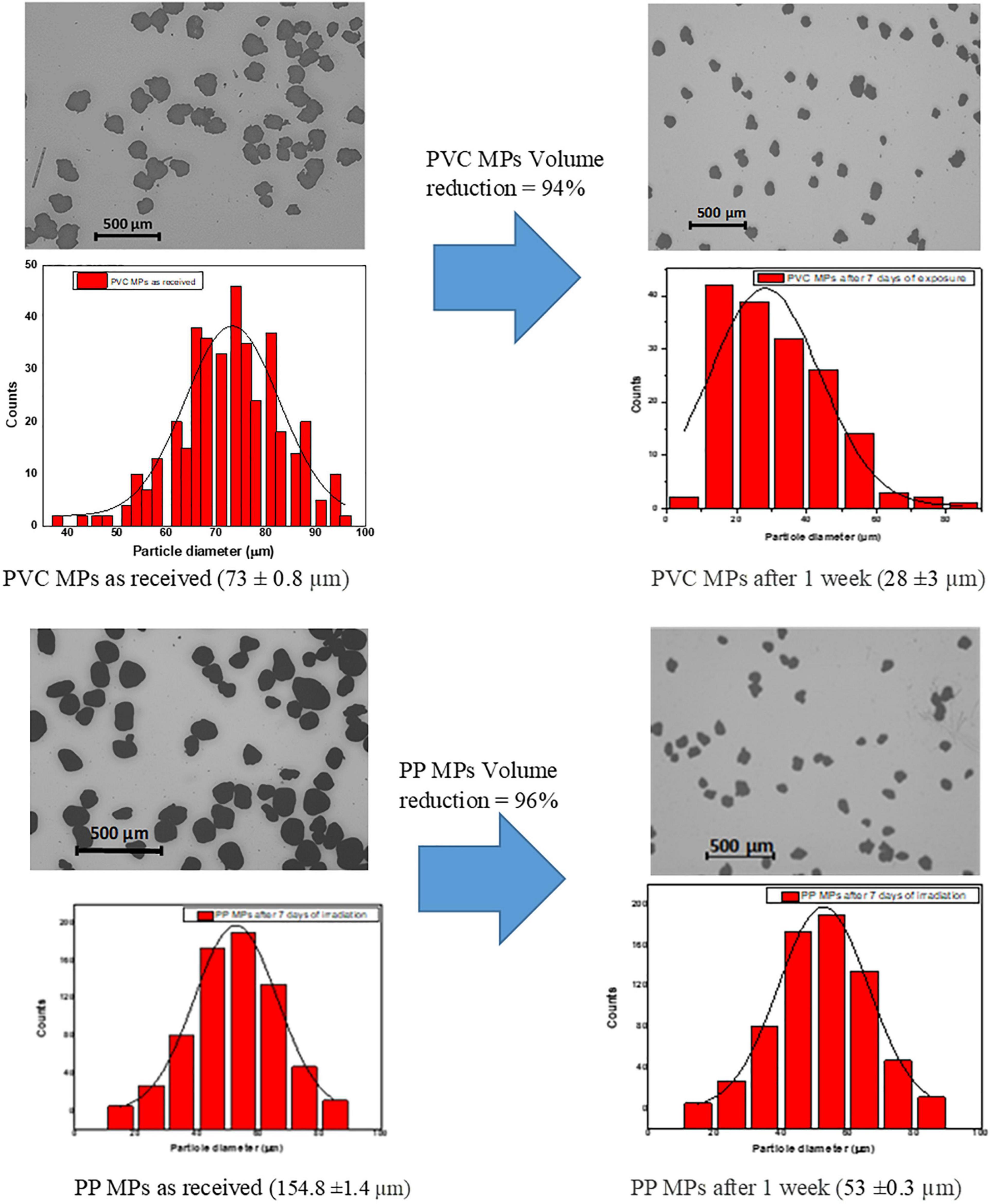
Figure 5. Optical Microscope images and particle size distribution before and after visible light irradiation of PVC and PP microplastics.
Ecotoxicity Screening
Aliivibrio fischeri Acute Toxicity Test
Results of bioluminescence alteration assay with A. fischeri after exposure to samples listed in Table 1 are reported in Figure 6; a complete lack of effect (bioluminescence alteration respect to control) is put in evidence for all tested samples, with effect percentages below 10%.
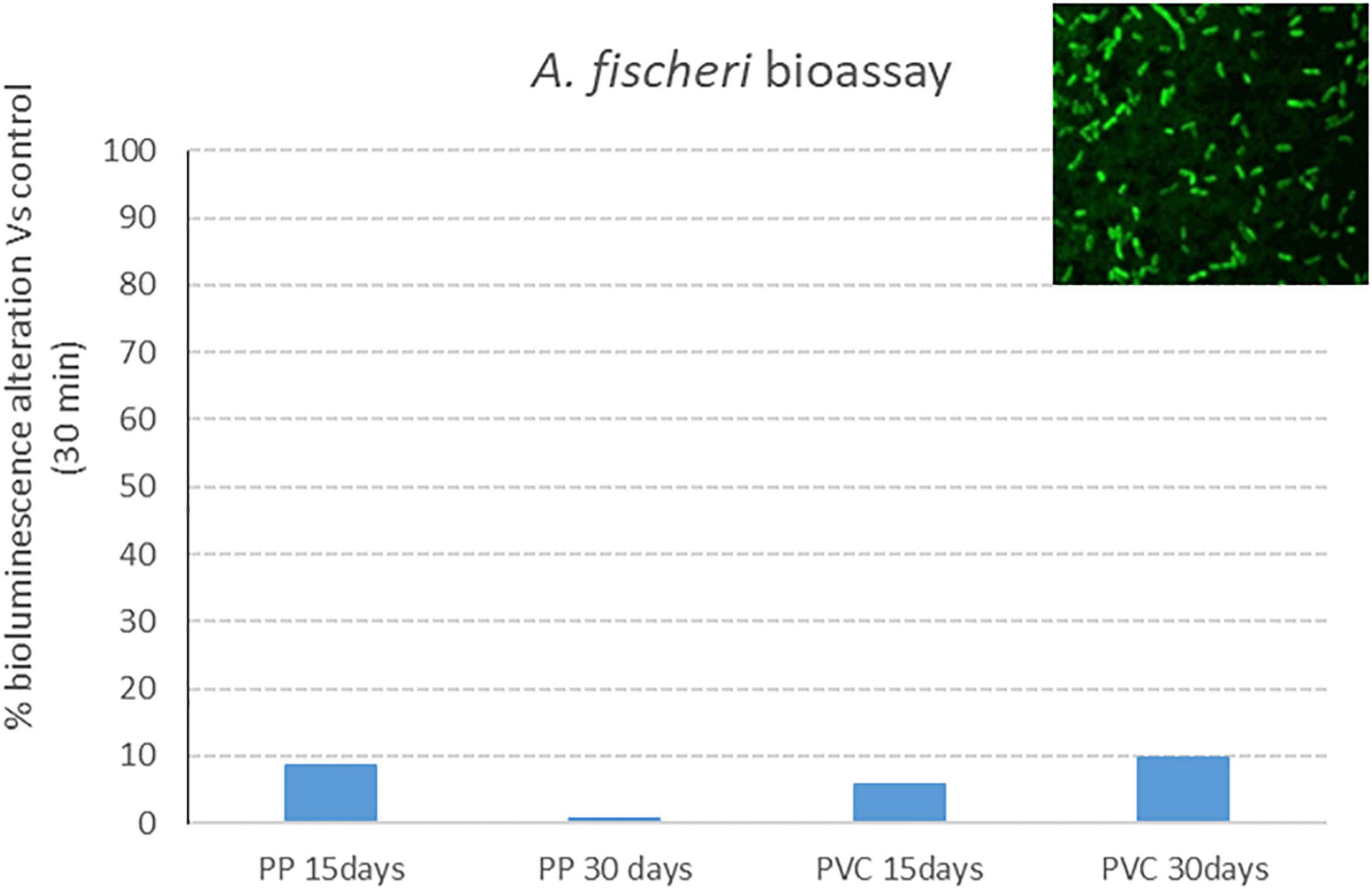
Figure 6. Bacterial bioluminescence alteration: effect percentage (respect to control) after 30 min of exposure to PP 15 days, PP 30 days, PVC 15 days and PVC 30 days (samples after degradation with photocatalytic device).
P. subcapitata Chronic Toxicity Assay
Results obtained in the algal growth alteration assay with P. subcapitata after exposure to samples listed in Table 1 are reported in Figure 7. Graphs show the effect percentage (algal growth alteration respect to control) registered after 72 h of exposure to investigated samples. Also for this model organism, the effect is always below 10%, thus displaying a lack of toxicity of tested samples.
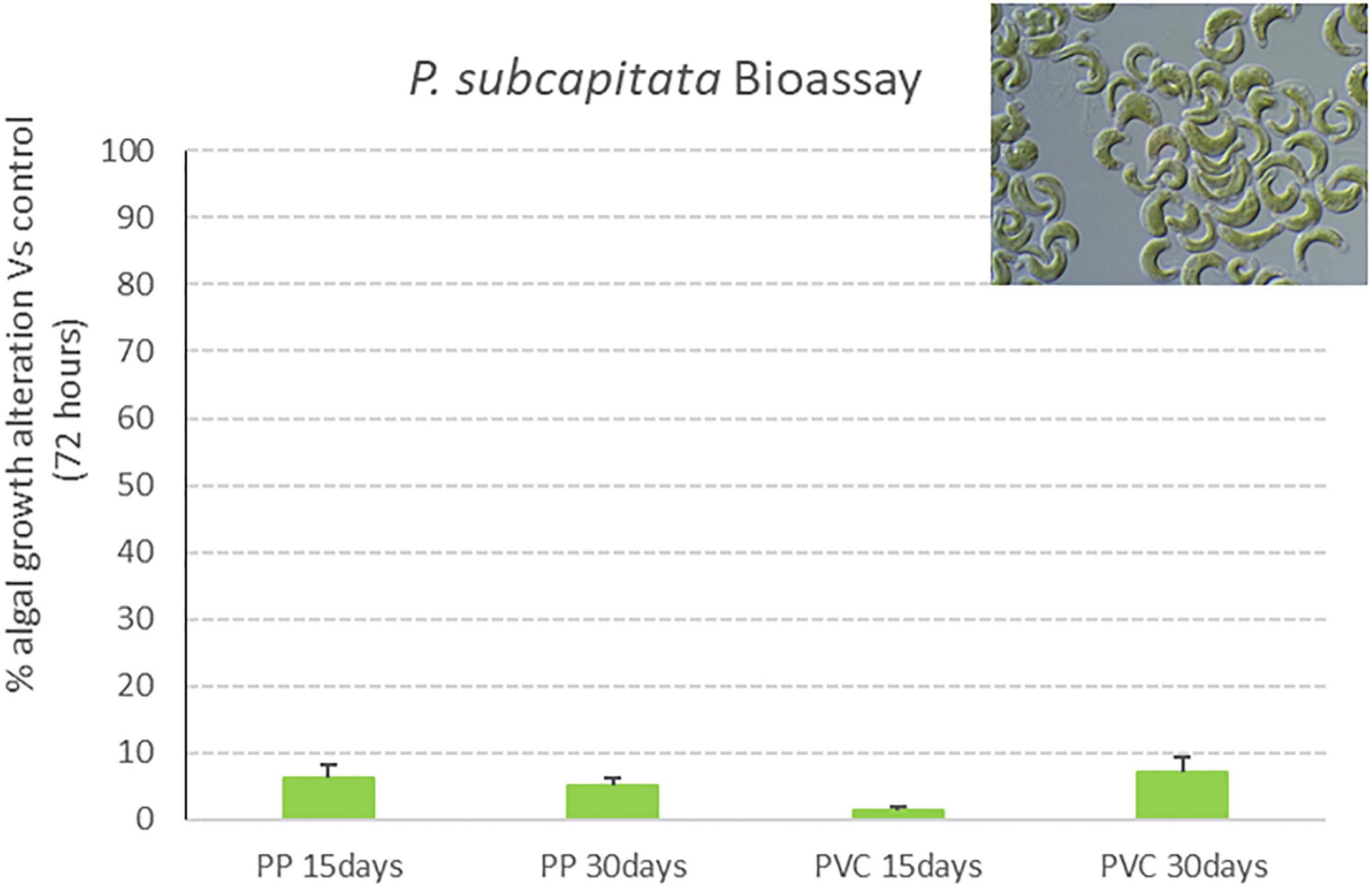
Figure 7. Algal growth alteration percentage (vs. control) of P. subcapitata exposed for 72 h to PP 15 days, PP 30 days, PVC 15 days and PVC 30 days (samples after degradation with photocatalytic device) (M ± ES; n = 3).
D. magna Acute Toxicity Assay
Results obtained in the immobilization test with D. magna after exposure to samples listed in Table 1 are reported in Figure 8. Graphs show the effect percentage (% of immobile organisms) registered after 24 and 48 h of exposure to investigated samples. A negative control (ctr) was prepared too, consisting in organisms exposed in the same testing conditions such as temperature, water and volume to D. magna standard freshwater. According to the ISO protocol, a test is considered valid if, after 48 h, the immobilization percentage in the control group (ctr) is below 10%.
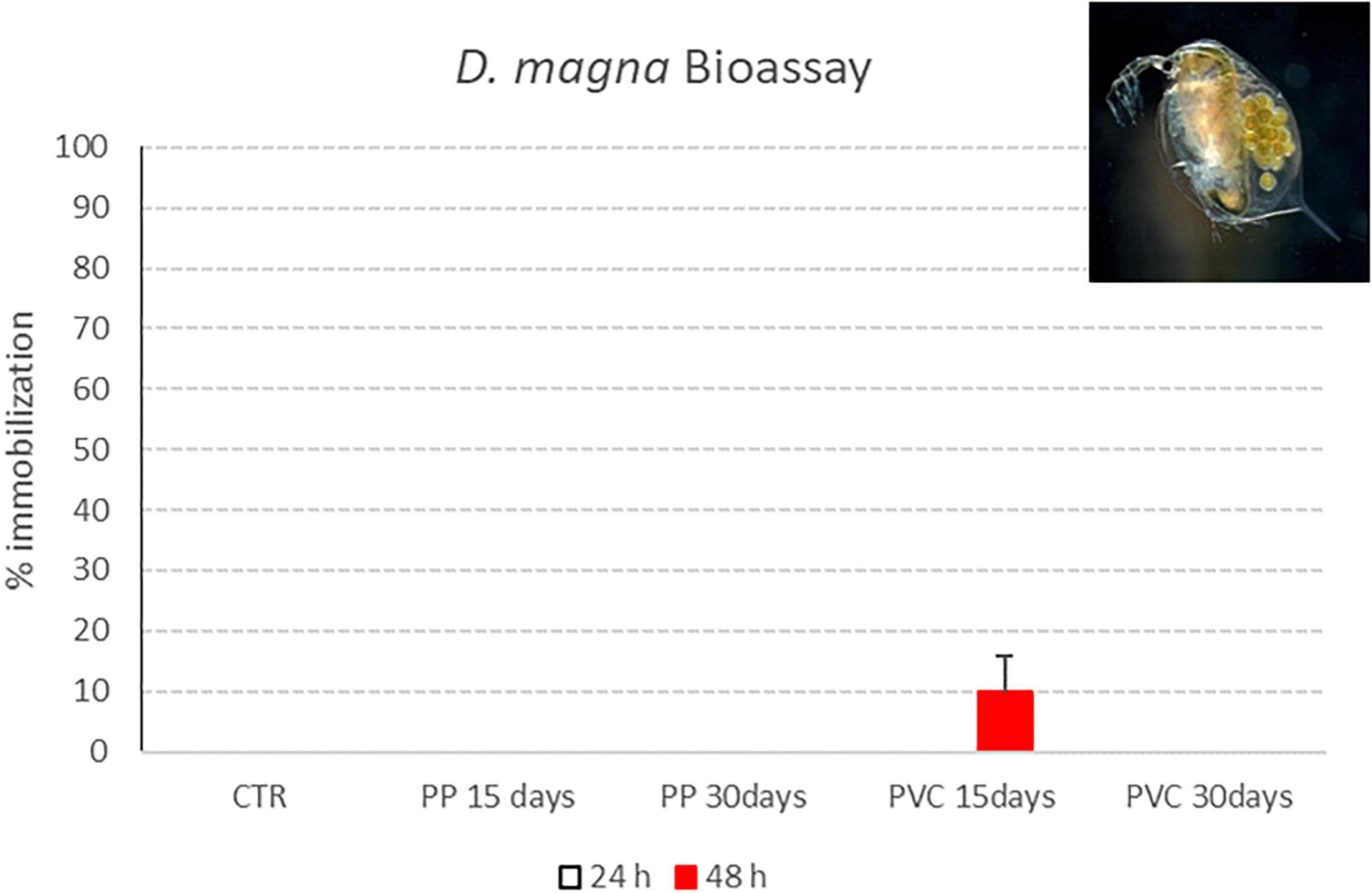
Figure 8. Immobility percentage of D. magna neonates exposed to PP 15 days, PP 30 days, PVC 15 days and PVC 30 days (samples after degradation with photocatalytic device). Red bars indicate immobilization percentages recorded 48 h of exposure (M ± ES; n = 4).
Only for “PVC 15 days” sample, a 10% immobilization is obtained after 48 h of contact with D. magna; for the other samples, all exposed organisms were swimming at the end of exposure period, thus showing a lack of toxic effect for tested samples.
In Table 2 the ecotoxicological effect of all post-treatment samples (PP 15, PP 30, PVC 15 and PVC 30 days) obtained with A. fischeri, P. subcapitata, and D. magna bioassay in terms of effect percentage (bacterial bioluminescence alteration vs. control after a 30 min of exposure; algal growth alteration vs. control after 72 h; immobilization after 48 h) are reported. Effect percentages result to be always lower than 10% toward all selected model organisms.
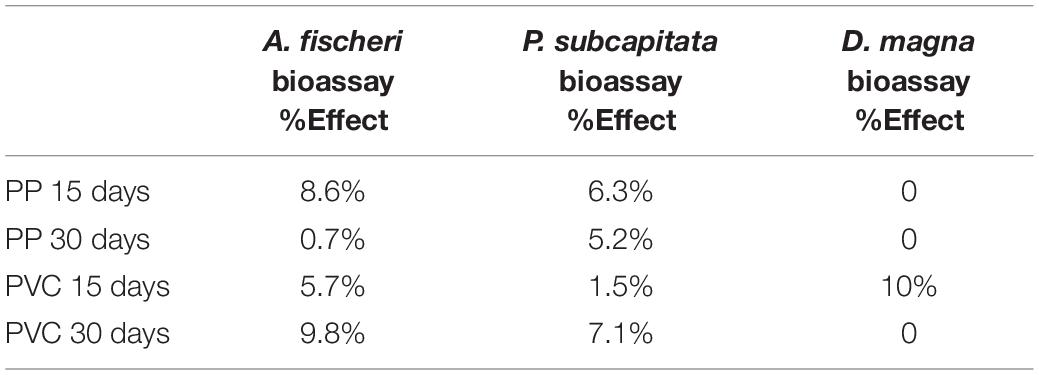
Table 2. Ecotoxicological effect of post-treatment samples obtained with A. fischeri, P. subcapitata, and D. magna bioassay in terms of effect percentage.
Control Samples
Results obtained for the three ecotoxicological bioassays after exposure to the control samples listed in Table 1 (deionized water without microplastics after 15 and 30 days of treatment with photocatalytic reactor and deionized water + Hydrogen Peroxide without microplastics after 15 and 30 days of treatment with photocatalytic reactor) are reported in this section (Figures 9, 10).
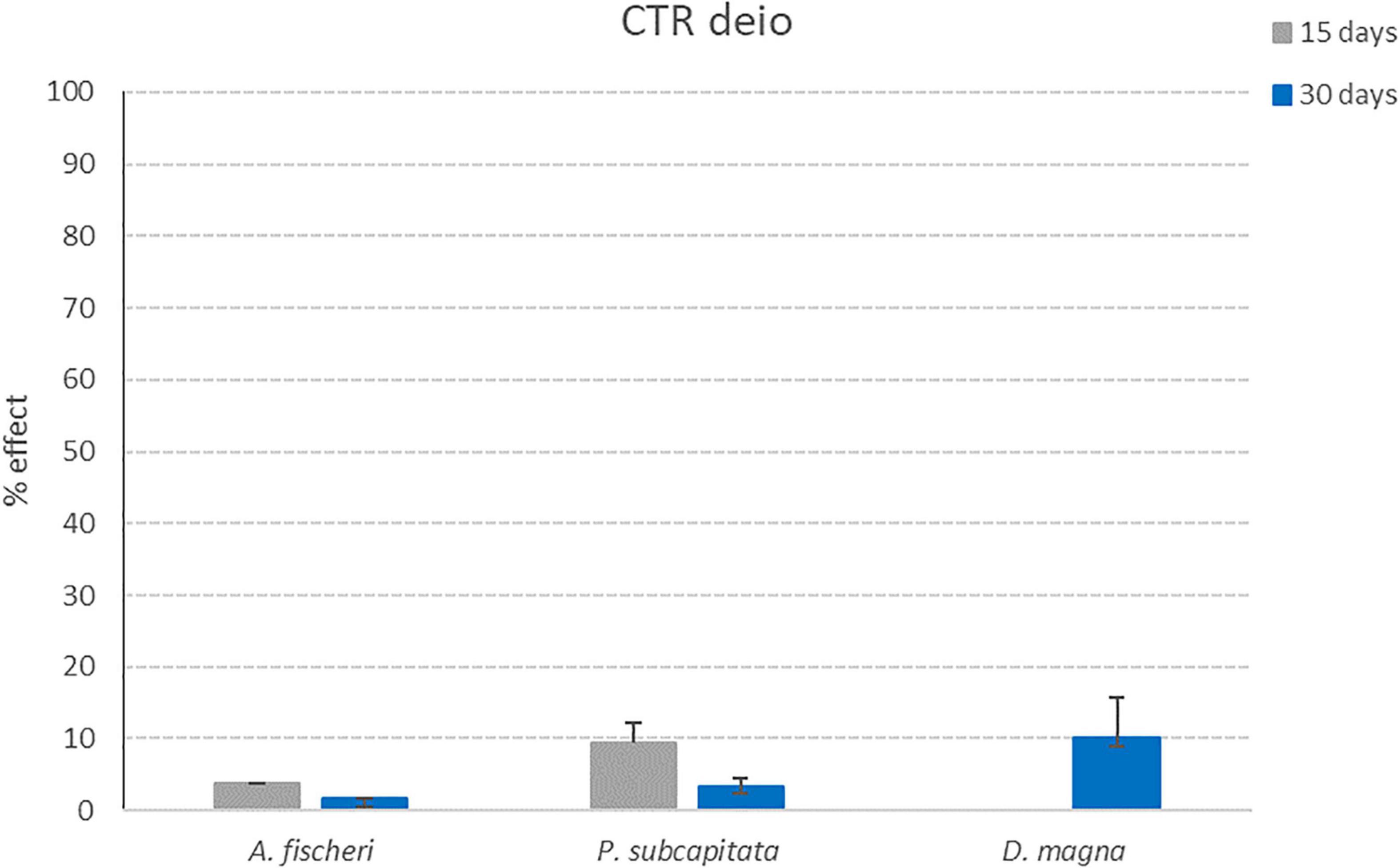
Figure 9. Effect percentages obtained for CTR deio sample (deionized water without microplastics after 15 and 30 days of treatment with photocatalytic reactor) after exposure to the three model organisms (A. fischeri; P. subcapitata; D. magna). Bars indicate effect percentages recorded for the observed end-points (bioluminescence alteration; algal growth alteration; immobilization) for CTR deio sample collected after 15 (grey bars) and 30 days (blue bars) of treatment with the photocatalytic reactor (M ± ES; n = 3/4 for D. magna).
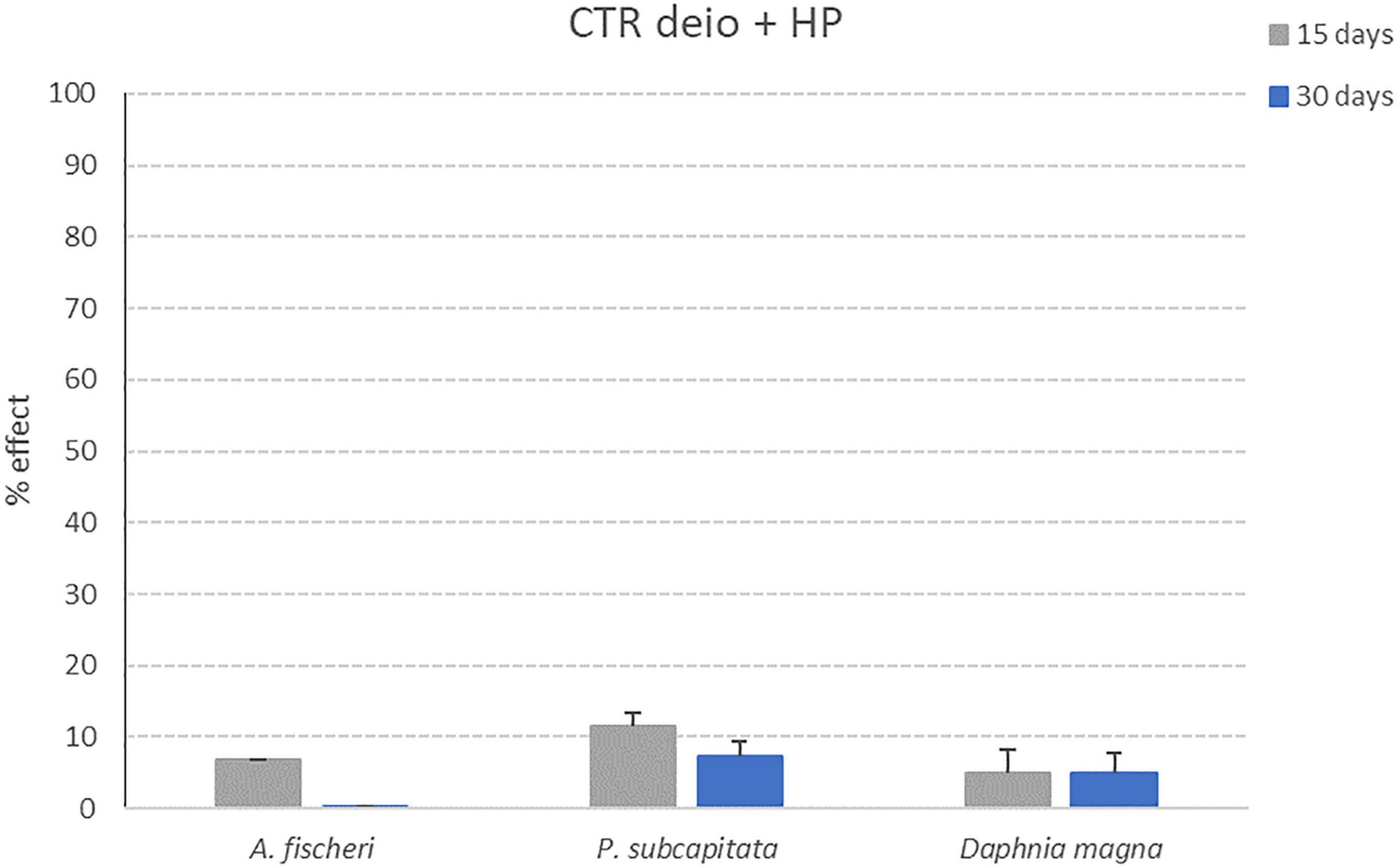
Figure 10. Effect percentages obtained for CTR deio + HP sample (deionized water + Hydrogen Peroxide without microplastics after 15 and 30 days of treatment with photocatalytic reactor) after exposure to the three model organisms (A. fischeri; P. subcapitata; D. magna). Bars indicate effect percentages recorded for the observed end-points (bioluminescence alteration; algal growth alteration; immobilization) for CTR deio + HP sample collected after 15 (grey bars) and 30 days (blue bars) of treatment with the photocatalytic reactor (M ± ES; n = 3/4 for D. magna).
For all control samples, the effect percentages obtained after exposure to the three tested model organisms are below 10% (11.5% for P. subcapitata after 72 h of exposure to CTR deio + HP sample collected after 15 days of treatment with photocatalytic reactor). These results put in evidence how the photocatalytic treatment is not able to generate toxic products due to the reactor itself (and not caused by the degradation process of microplastics).
Discussion
The evaluation of the environmental impacts of the by-products generated during microplastics degradation process is essential in understanding the mineralization mechanism. Generally, microplastics degradation is initialized by the polymer backbone cleavage to generate the hydrocarbon radicals. The reactive oxygen species (ROS) attack the metastable hydrocarbon species and led to the formation of smaller microplastics segments with low molecular weight like carbonyl and hydroperoxides (Kang et al., 2019). The generated degradation by-products are finally mineralized to CO2 and H2O by the active ROS to achieve high microplastics removal efficiency. However, in this work, complete mineralization was not achieved since the degradation efficiency (based on particle volume reduction) was determined to be 94 and 96% for PVC and PP microplastics, respectively, after 1 week of visible light irradiation. In this process microplastics degradation may produce some toxic by-products, therefore, the impact assessment of the photo-Fenton process is needed. This can be accomplished using ecotoxicity test of the treated water after the degradation of the microplastics using lab scale device.
The ecosafety assessment of the photo-Fenton technology proposed for the removal of microplastics from WWTPs effluent is evaluated and performed by means of an ecotoxicological approach. The aim of this study was to quantify if the visible light photo-Fenton process carried out with lab-scale device is able to generate environmentally harmful by-products, thus if the device can be considered as “eco-friendly.” Water samples before and after the degradation process of PP and PVC microplastics were collected as reported in “Degradation experiments” section and then submitted to a battery of three ecotoxicological bioassays that includes commonly adopted model organisms, representing different parts of the trophic chain (taxonomic groups) (Czech et al., 2014; Filella, 2015; Freitas et al., 2017; Arefi-Oskoui et al., 2021). The microparticles concentration adopted for “pre-treatment” samples (Σ19 microplastics particles/liter) was established on the basis of literature data, that reports an average concentration of microplastics entering waste water treatment plants of Σ15.7 particles/liter (Murphy et al., 2016). The “real-case samples” were determined by a careful consideration of the published literature. This is substantiated in a recent paper where 19 studies were reviewed by Schmidt et al. (2020) from 2016 to 2020, the concentrations of observed microplastics from 79 WWTP effluents was estimated to average 6,400 items/m3. Thus, 10,000–15,000 particles/m3 of simulated waste water is reasonably justified.
Proposed bioassays are generally used to evaluate toxicity effects of several chemical substances in homogenous aquatic test media (water or wastewater samples), but they are also suitable to perform an evaluation of potential toxic effects carried out by the photocatalysis-Fenton synergistic process. Ecotoxicology is commonly applied to determine the impact of contaminants on living organisms (Manusadzianas et al., 2003; Naddy et al., 2007; Fisher et al., 2010). The use of ecotoxicity bioassays can be proposed as a sensitive, quick and reliable tool to evaluate the potential contamination during wastewater treatment (Fisher et al., 2010). The great popularity of Daphtoxkit F™ (with Daphnia magna as tested organism) and Microtox® (A. fischeri) can be ascribed to their advantages, such as the availability of commercial kits and the ease to be performed (Sponza, 2006).
D. magna represents one of most commonly used ecotoxicological tests due to the high sensitivity of the model organism toward a wide range of chemicals, and also to the fact that it occupies a central position in the lentic food chain (Bervoets et al., 1996; Naddy et al., 2007). Also the bioluminescent bacteria A. fischeri is commonly adopted as model organism in toxicity tests that is internationally recognized and standardized as ISO (2007) (Libralato et al., 2010); the Microtox® acute toxicity assay results to be sensitive, reproducible and it possesses high discriminant power for organic and inorganic pollutants. Lastly growth inhibition bioassay with freshwater microalga P. subcapitata is quite frequently used as a bioindicator for toxic substances, including micropollutants and nanoparticles (Aruoja et al., 2009).
The employment of ecotoxicity bioassays is reported in the qualitative analysis of photocatalytically treated wastewater containing pharmaceuticals and personal care products (PPCPs) (Schnell et al., 2009; Marugan et al., 2010; Czech et al., 2014; Antonopoulou and Konstantinou, 2016; Candido et al., 2017; Calza et al., 2021) and micropollutants (Freitas et al., 2017).
A critical review on the application of photocatalysis for the treatment of wastewaters has been recently published (Rueda-Marquez et al., 2020). Since it is well known that during photocatalytic decomposition of target pollutants in water generation of more toxic by-products can occur, the evaluation of environmental impact of discharged treated effluents is of primary importance. In his review, Rueda-Marquez includes a number of studies investigating the toxicity of the TPs (transformation products) deriving from the photocatalytic degradation of different pollutants. In more than 20 works cited, 14 report for the use of the same bioassays selected for this study in the toxicity assessment of the photocatalysis process, even if no literature specifically related to Microplastics degradation is reported. Both acute and chronic toxicity bioassays were applied for photocatalytically treated wastewater effluents toxicity evaluation, such as bioassays with bacteria (He et al., 2016; Nogueira et al., 2017; Talwar et al., 2018), freshwater invertebrates (Çifçi and Meriç, 2015) and microalgae (He et al., 2016). An important outcome of this review is that, in some cases, the ecotoxicological assessment may be even more sensitive than chemical analysis. So it is suggested that studies aimed to evaluate the toxicity of wastewater after photocatalysis treatment should include batteries of bioassays for a more comprehensive and complete evaluation of water toxicity.
All bioassays performed in this study have pointed out a lack of toxicity effects for all tested samples (containing PP or PVC microparticles), both obtained after 15 and 30 days of treatment. Effect percentages reported for all considered end points are always below 10%, this threshold is considered in ecotoxicological protocols as a “no effect” limit, comparable to control values. These results show how the photoreactor is able to significantly reduce microparticles volume (94 and 96% for PVC and PP microplastics, respectively) without generating any harmful by-product. These results obtained for Control samples, also having effect percentages always below 10%, confirm the environmentally friendliness of nanocoating material used in this work.
Conclusion
Aim of this work, was to validate the use of the photo-Fenton process for the degradation of microplastics discharged from WWTPs by excluding any toxic effect on aquatic environment by means of an ecotoxicological screening. Results pointed out that high degradation efficiency of PP and PVC microplastics in relatively short time was achieved. However, complete mineralization was not achieved and the ecotoxicity bioassays performed on the treated solutions revealed that the degradation by-products of the microplastics investigated in this work did not show any toxic effect to the microorganisms.
The ecotoxicological screening applied included a battery of three aquatic model organisms and was aimed to check the absence of any toxic effect related to by-products formed during the degradation process, which may cause environmental risks. The obtained results demonstrated that after the degradation, no toxicity toward bacteria, algae and crustaceans was detected, thus showing the absence of any toxicological effect related to the photo-Fenton degradation process, with both polymer typologies tested (PP and PVC). The results obtained are encouraging for the implementation of the photoreactor in large-scale water and wastewater treatment plants, for sustainable removal of microplastics from water sources prior to its use or discharge to the environment.
Data Availability Statement
The original contributions presented in the study are included in the article/supplementary material, further inquiries can be directed to the corresponding author/s.
Author Contributions
MF and FG designed the ecotoxicological experimental study and reviewed the manuscript. VP performed the ecotoxicological bioassays and wrote the manuscript initial draft. CG performed the ecotoxicological bioassays and reviewed the manuscript. AU and JD designed the photo-Fenton experimental study and wrote in the manuscript the part concerning the study. All authors contributed to the article and approved the submitted version.
Funding
This work was financially supported by CLAIM project: “Cleaning Litter by developing and Applying Innovative Methods in European seas” (H2020-BG-2016-2017, Grant No. 774586).
Conflict of Interest
The authors declare that the research was conducted in the absence of any commercial or financial relationships that could be construed as a potential conflict of interest.
Publisher’s Note
All claims expressed in this article are solely those of the authors and do not necessarily represent those of their affiliated organizations, or those of the publisher, the editors and the reviewers. Any product that may be evaluated in this article, or claim that may be made by its manufacturer, is not guaranteed or endorsed by the publisher.
Acknowledgments
We would like to thank Fei Ye and Esteban Carrillo for providing SEM images.
References
Andrady, A. L. (2011). Microplastics in the marine environment. Mar. Pollut. Bull. 62, 1596–1605. doi: 10.1016/j.marpolbul.2011.05.030
Antonopoulou, M., and Konstantinou, I. (2016). Photocatalytic degradation and mineralization of tramadol pharmaceutical in aqueous TiO2 suspensions: evaluation of kinetics, mechanisms and ecotoxicity. Appl. Cataly. General 515, 136–143. doi: 10.1016/j.scitotenv.2015.12.088
Arefi-Oskoui, S., Khataee, A., Koba Ucun, O., Kobya, M., Olmez Hanci, T., and Arslan-Alaton, I. (2021). Toxicity evaluation of bulk and nanosheet MoS2 catalysts using battery bioassays. Chemosphere 268:128822. doi: 10.1016/j.chemosphere.2020.128822
Ariza-Tarazona, M. C., Villarreal-Chiu, J. F., Hernández-López, J. M., Rivera De la Rosa, J., Barbieri, V., Siligardi, C., et al. (2020). Microplastic pollution reduction by a carbon and nitrogen-doped TiO2: effect of pH and temperature in the photocatalytic degradation process. J. Hazard. Mater. 395:122632. doi: 10.1016/j.jhazmat.2020.122632
Aruoja, V., Dubourguier, H. C., Kasemets, K., and Kahru, A. (2009). Toxicity of nanoparticles of CuO, ZnO and TiO2 to microalgae Pseudokirchneriella subcapitata. Sci. Total Environ. 407, 1461–1468. doi: 10.1016/j.scitotenv.2008.10.053
Aslanzadeh, S., and Haghighat, M. K. (2010). Photo-oxidation of polypropylene fibers exposed to short wavelength UV radiations. Fibers Polym. 11, 710–718. doi: 10.1007/s12221-010-0710-8
Bervoets, L., Baillieul, M., Blust, R., and Verheyen, R. (1996). Evaluation of effluent toxicity and ambient toxicity in a polluted lowland river. Environ. Pollut. 91, 333–341. doi: 10.1016/0269-7491(96)80915-8
Bora, T., Sathe, P., Laxman, K., Dobretsov, S., and Dutta, J. (2017). Defect engineered visible light active ZnO nanorods for photocatalytic treatment of water. Cataly. Today 284, 11–18. doi: 10.1016/j.colsurfb.2019.110541
Brenneke, D., Duarte, B., Paiva, F., Caçador, I., and Canning-Clode, J. (2016). Microplastics as vector for heavy metal contamination from the marine environment. Estuar. Coast. Shelf Sci. 178, 189–195. doi: 10.1016/j.ecss.2015.12.003
Bretas-Alvim, C., Mendoza-Roca, J. A., and Bes-Piá, A. (2020). Wastewater treatment plant as microplastics release source–quantification and identification techniques. J. Environ. Manag. 255:109739. doi: 10.1016/j.jenvman.2019.109739
Browne, M. A., Crump, P., Niven, S. J., Teuten, E., Tonkin, A., Galloway, T., et al. (2011). Accumulation of microplastic on shorelines worldwide: sources and sinks. Environ. Sci. Technol. 45, 9175–9179. doi: 10.1021/es201811s
Calza, P., Jiménez-Holgado, C., Coha, M., Chrimatopoulos, C., Dal Bello, F., Medana, C., et al. (2021). Study of the photoinduced transformations of sertraline in aqueous media. Sci. Total Environ. 756:143805. doi: 10.1016/j.scitotenv.2020.143805
Candido, J. P., Andrade, S. J., Fonseca, A. L., Silva, F. S., Silva, M. R. A., and Kondo, M. M. (2017). Ibuprofen removal by heterogeneous photocatalysis and ecotoxicological evaluation of the treated solutions. Environ. Sci. Pollut. Res. 24:6397. doi: 10.1007/s11356-017-8623-3
Çifçi, D. I., and Meriç, S. (2015). Optimization of suspended photocatalytic treatment of two biologically treated textile effluents using TiO2 and ZnO catalysts. Global NEST J. 17, 653–663.
Cózar, A., Echevarría, F., Gonzálezgordillo, J. I., Irigoien, X., Ubeda, B., Hernández, L. S., et al. (2014). Plastic debris in the open ocean. Proc. Natl. Acad. Sci. U.S.A. 111, 10239–10244. doi: 10.1073/pnas.1314705111
Cristaldi, A., Fiore, M., Zuccarello, P., Oliveri Conti, G., Grasso, A., Nicolosi, I., et al. (2020). Efficiency of wastewater treatment plants (WWTPs) for microplastic removal: a systematic review. Int. J. Environ. Res. Public Health 17:8014. doi: 10.3390/ijerph17218014
Czech, B., Jośko, I., and Oleszczuk, P. (2014). Ecotoxicological evaluation of selected pharmaceuticals to vibrio fischeri and daphnia magna before and after photooxidation process. Ecotoxicol. Environ. Safety 104, 247–253. doi: 10.1016/j.ecoenv.2014.03.024
de Carvalho, C. L., Silveira, A. F., and Rosa, D. D. S. (2013). A study of the controlled degradation of polypropylene containing pro-oxidant agents. Springerplus 2, 623–623. doi: 10.1186/2193-1801-2-623
Du, H., Xie, Y., and Wang, J. (2021). Review: microplastic degradation methods and corresponding degradation mechanism: research status and future perspectives. J. Hazard. Mater. 418:126377. doi: 10.1016/j.jhazmat.2021.126377
European Commission (2015). Closing the Loop—An EU Action Plan for the Circular Economy. Communication No. 52015DC0614.
European Commission (2018a). Directive No 2018/851 of the European parliament and of the council of 30 May 2018 amending directive 2008/98/EC on waste. O. J. Eur. Union 2018, 109–140.
European Commission (2018b). European council decision no 2018/340 of 6 march 2018 establishing the list of projects to be developed under PESCO. O. J. Eur. Union 2018:24.
European Commission (2018c). Plastic Waste: A European Strategy to Protect the Planet, Defend Our Citizens and Empower Our Industries. Strasbourg, 16.01.2018 European Commission. Brussels: European Commission.
Farrell, P., and Nelson, K. (2013). Trophic level transfer of microplastic: Mytilus edulis (L.) to Carcinus maenas (L.). Environ. Pollut. 177, 1–3. doi: 10.1016/j.envpol.2013.01.046
Fendall, L. S., and Sewell, M. A. (2009). Contributing to marine pollution by washing your face: microplastics in facial cleansers. Mar. Pollut. Bull. 58, 1225–1228. doi: 10.1016/j.marpolbul.2009.04.025
Filella, M. (2015). Questions of size and numbers in environmental research on microplastics: methodological and conceptual aspects. Environ. Chem. 12, 527–538. doi: 10.1071/en15012
Fisher, J. C., Belden, J. B., and Bidwell, J. R. (2010). Can site-specific heuristic models predict the toxicity of produced water? Chemosphere 80, 542–547. doi: 10.1016/j.chemosphere.2010.04.040
Freeman, S., Booth, A. M., Sabbah, I., Tiller, R., Dierking, J., Klun, K., et al. (2020). Review. between source and sea: the role of wastewater treatment in reducing marine microplastics. J. Env. Manage. 266:110642. doi: 10.1016/j.jenvman.2020.110642
Freitas, A. M., Rivas, G., Campos-Mañas, M. C., Casas López, J. L., Agüera, A., and Sánchez Pérez, J. A. (2017). Ecotoxicity evaluation of a WWTP effluent treated by solar photo-fenton at neutral pH in a raceway pond reactor. Environ. Sci. Pollut. Res. 24, 1093–1104. doi: 10.1007/s11356-016-7101-7
Gall, S. C., and Thompson, R. C. (2015). The impact of debris on marine life. Mar. Pollut. Bull. 92, 170–179. doi: 10.1016/j.marpolbul.2014.12.041
Gao, F., Li, J., Sun, C., Zhang, L., Jiang, F., Cao, W., et al. (2019). Study on the capability and characteristics of heavy metals enriched on microplastics in marine environment. Mar. Pollut. Bull. 144, 61–67. doi: 10.1016/j.marpolbul.2019.04.039
Hamd, W. S., and Dutta, J. (2020). “Chapter 11 - heterogeneous photo-fenton reaction and its enhancement upon addition of chelating agents,” in Book Chapter in Nanomaterials for the Detection and Removal of Wastewater Pollutants, Micro and Nano Technologies, eds B. Bonelli, F. S. Freyria, I. Rossetti, and R. Sethi (Amsterdam: Elsevier), 303–330. doi: 10.1016/b978-0-12-818489-9.00011-6
Hashmi, M. Z. (2021). Microplastic Pollution: Environmental Occurrence and Treatment Technologies: ISBN 978-3-030-89220-3.
He, Y., Sutton, N. B., Rijnaarts, H. H. H., and Langenhoff, A. A. M. (2016). Degradation of pharmaceuticals in wastewater using immobilized TiO2 photocatalysis under simulated solar irradiation. Appl. Catal. B Environ 182, 132–141. doi: 10.1016/j.apcatb.2015.09.015
Hu, K., Tian, W., Yang, Y., Nie, G., Zhou, P., Wang, Y., et al. (2021). Microplastics remediation in aqueous systems: strategies and technologies. Water Res. 198, 117–144. doi: 10.1016/j.watres.2021.117144
Irfan, T., Khalid, S., Taneez, M., and Hashmi, M. Z. (2020). Plastic driven pollution in pakistan: the first evidence of environmental exposure to microplastic in sediments and water of rawal lake. Environ. Sci. Pollut. Res. 27, 5083–15092. doi: 10.1007/s11356-020-07833-1
Iyare, P. U., Ouki, S. K., and Bond, T. (2020). Microplastics removal in wastewater treatment plants: a critical review. Environ. Sci. Water Res. Technol. 6:2664.
Jambeck, J. R., Geyer, R., Wilcox, C., Siegler, T. R., Perryman, M., and Andrady, A. (2015). Plastic waste inputs from land into the ocean. Science 347, 768–771. doi: 10.1126/science.1260352
Kang, J., Zhou, L., Duan, X., Sun, H., Ao, Z., and Wang, S. (2019). Degradation of cosmetic microplasticsvia functionalized carbon nanosprings. Matter 1, 745–758.
Karbalaei, S., Hanachi, P., Walker, T. R., and Cole, M. (2018). Occurrence, sources, human health impacts and mitigation of microplastic pollution. Environ. Sci. Pollut. Res. Int. 25, 36046–36063. doi: 10.1007/s11356-018-3508-7
Kitsomboonloha, R., Baruah, S., Myint, M. T. Z., Subramanian, V., and Dutta, J. (2009). Selective growth of zinc oxide nanorods on inkjet printed seed patterns. J. Cryst. Growth 311, 2352–2358.
Koelmans, A. A., Mohamed Nor, N. H., Hermsen, E., Kooi, M., Mintenig, S. M., and De France, J. (2019). Microplastics in freshwaters and drinking water: critical review and assessment of data quality. Water Res. 155, 410–422. doi: 10.1016/j.watres.2019.02.054
Kumar, S., Ye, F., Mazinani, B., Dobretsov, S., and Dutta, J. (2021). Chitosan nanocomposite coatings containing chemically resistant ZnO–SnOx core–shell nanoparticles for photocatalytic antifouling. Int. J. Mol. Sci. 22:4513. doi: 10.3390/ijms22094513
Lavers, J. L., and Bond, A. L. (2017). Exceptional and rapid accumulation of anthropogenic debris on one of the world’s most remote and pristine islands. Proc. Natl. Acad. Sci. U.S.A. 114, 6052–6055. doi: 10.1073/pnas.1619818114
Leslie, H. A. (2014). “Review of Microplastics in Cosmetics”: Scientific Background on a Potential Source of Plastic Particulate Marine Litter to Support Decision-making. Report in E&H: Environmental Chemistry and Toxicology, AIMMS. Amsterdam: Vrije Universiteit Amsterdam, 30.
Li, X., Wang, J., Rykov, A. I., Sharma, V. K., Wei, H., and Jin, C. et al, (2015). Prussian blue/TiO2 nanocomposites as a heterogeneous photo-Fenton catalyst for degradation of organic pollutants in water. Catal. Sci. Technol. 5, 504.
Libralato, G., Volpi Ghirardini, A., and Avezzù, F. (2010). How toxic is toxic? A proposal for waste water toxicity hazard assessment. Ecotox. Environ. Safe. 73, 1602–1611.
Liu, W., Zhang, J., Liu, H., Guo, X., Zhang, X., Yao, X., et al. (2021). A review of the removal of microplastics in global wastewater treatment plants: characteristics and mechanisms. Environ. Int. 146:106277. doi: 10.1016/j.envint.2020.106277
Llorente-Garcia, B. E., Hernandez-Lopez, J. M., Zaldivar-Cadena, A. A., Siligardi, C., and Cedillo-Gonzalez, E. I. (2020). First insights into photocatalytic degradation of HDPE and LDPE microplastics by a mesoporous N-TiO(2) coating: effect of size and shape of microplastics. Coatings 10:658. doi: 10.3390/coatings10070658
Manusadzianas, L., Balkelyte, L., Sadauskas, K., Blinova, I., Polluma, L., and Kahru, A. (2003). Ecotoxicological study of lithuanian and estonian wastewaters: selection of the biotests, and correspondence between toxicity and chemical-based indices. Aquat. Toxicol. 63, 27–41. doi: 10.1016/s0166-445x(02)00132-7
Marugan, J., Achilleos, A., Hapeshi, E., Xekoukoulotakis, N. P., Mantzavinos, D., and Fatta-Kassinos, D. (2010). Factors affecting diclofenac decomposition in water by UV-A/TiO2 photocatalysis. Chem. Eng. J. 161, 53–59.
Mato, Y., Isobe, T., Takada, H., Kanehiro, H., Ohtake, C., and Kaminuma, T. (2001). Plastic resin pellets as a transport medium for toxic chemicals in the marine environment. Environ. Sci. Technol. 35, 318–324. doi: 10.1021/es0010498
Murphy, F., Ewins, C., Carbonnier, F., and Quinn, B. (2016). Wastewater treatment works (WwTW) as a source of microplastics in the aquatic environment. Environ. Sci. Technol. 50, 5800-5808. doi: 10.1021/acs.est.5b05416
Nabi, I., Bacha, A.-U.-R., Li, K., Cheng, H., Wang, T., Liu, Y., et al. (2020). Complete photocatalytic mineralization of microplastic on TiO2 nanoparticle film. Iscience 23:101326. doi: 10.1016/j.isci.2020.101326
Naddy, R. B., Gorsuch, J. W., Rehner, A. B., McNerney, G. R., Bell, R. A., and Kramer, J. R. (2007). Chronic toxicity of silver nitrate to Ceriodaphnia dubia and Daphnia magna, and potential mitigating factors. Aquat. Toxicol. 84, 1–10. doi: 10.1016/j.aquatox.2007.03.022
Nakata, K., and Fujishima, A. (2012). TiO2 photocatalysis: design and applications. J. Photochem. Photobiol. C Photochem. Rev. 13, 169–189.
Nogueira, V., Lopes, I., Rocha-Santos, T., Gonçalves, F., and Pereira, R. (2017). Treatment of real industrial wastewaters through nano-TiO2 and nano-Fe2O3 photocatalysis: case study of mining and kraft pulp mill effluents. Environ. Technol. 39, 1586–1596. doi: 10.1080/09593330.2017.1334093
Prata, J. C. (2018). Microplastics in wastewater: state of the knowledge on sources, fate and solutions. Mar. Pollut. Bull. 129, 262–265. doi: 10.1016/j.marpolbul.2018.02.046
Prata, J. C., da Costa, J. P., Duarte, A. C., and Rocha-Santos, T. (2019). Methods for sampling and detection of microplastics in water and sediment: a critical review. TrAC Trends Anal. Chem. 110, 150–159. doi: 10.1007/s11356-018-1692-0
Raji, M., Mirbagheri, S. A., Ye, F., and Dutta, J. (2021). Nano zero-valent iron on activated carbon cloth support as fenton-like catalyst for efficient color and COD removal from melanoidin wastewater. Chemosphere 263:127945. doi: 10.1016/j.chemosphere.2020.127945
Ramesh, S., Tang, S. Y., and Liew, C. W. (2011). Effect of dibutyl phthalate as plasticizer on high-molecular weight poly(vinyl chloride)–lithium tetraborate-based solid polymer electrolytes. Ionics 17:705. doi: 10.1007/s11581-011-0568-9
Rueda-Marquez, J. J., Levchuk, I., Fernandez Ibanez, P., and Sillanpää, M. (2020). A critical review on application of photocatalysis for toxicity reduction of real wastewaters. J. Cleaner Produ. 258;120694. doi: 10.3390/ijerph18010077
SAPEA. (2019). A Scientific Perspective on Microplastics in Nature and Society. Evidence Review Report No. 4. Berlin: Science Advice for Policy by European Academies (SAPEA), 173.
Schmidt, C., Kumar, R., Yang, S., and Büttner, O. (2020). Microplastic particle emission from wastewater treatment plant effluents into river networks in germany: loads, spatial patterns of concentrations and potential toxicity. Sci. Total Environ. 737:139544. doi: 10.1016/j.scitotenv.2020.139544
Schnell, S., Bols, N. C., Barata, C., and Porte, C. (2009). Single and combined toxicity of pharmaceuticals and personal care products (PPCPs) on the rainbow trout liver cell lineRTL-W1. Aquat. Toxicol. 93, 244–252. doi: 10.1016/j.aquatox.2009.05.007
Setälä, O., Fleming-Lehtinen, V., and Lehtiniemi, M. (2014). Ingestion and transfer of microplastics in the planktonic food web. Environ. Pollut. 185, 77–83. doi: 10.1016/j.envpol.2013.10.013
Sol, D., Laca, A., Laca, A., and Díaz, M. (2020). Approaching the environmental problem of microplastics: importance of WWTP treatments. Sci. Total Environ. 740:140016. doi: 10.1016/j.scitotenv.2020.140016
Sponza, D. T. (2006). Toxicity studies in a chemical dye production industry in Turkey. J. Hazard. Mater. 138, 438–447. doi: 10.1016/j.jhazmat.2006.05.120
Sundt, P., Schulze, P. E., and Syversen, F. (2014). Sources of Microplastics Pollution to the Marine Environment. Report No. M-321| 2015.
Talvitie, J., Mikola, A., Koistinen, A., and Setälä, O. (2017). Solutions to microplastic pollution—removal of microplastics from wastewater effluent with advanced wastewater treatment technologies. Water Res. 123, 401–407. doi: 10.1016/j.watres.2017.07.005
Talwar, S., Sangal, V. K., and Verma, A. (2018). Feasibility of using combined TiO2 photocatalysis and RBC process for the treatment of real pharmaceutical wastewater. J. Photochem. Photobiol. Chem. 189, 263–270. doi: 10.1016/j.jphotochem.2017.11.013
Teuten, E. L., Saquing, J. M., Knappe, D. R. U., Barlaz, M. A., Jonsson, S., Bjorn, A., et al. (2009). Transport and release of chemicals from plastics to the environment and to wildlife. Philos. Trans. R Soc. B Biol. Sci. 364, 2027–2045. doi: 10.1098/rstb.2008.0284
Tofa, T. S., Kunjali, K. L., Paul, S., and Dutta, J. (2019a). Visible light photocatalytic degradation of microplastic residues with zinc oxide nanorods. Environ. Chem. Lett. 17, 1341–1346.
Tofa, T. S., Ye, F., Kunjali, K. L., and Dutta, J. (2019b). Enhanced visible light photodegradation of microplastic fragments with plasmonic platinum/zinc oxide nanorod photocatalysts. Catalysts 9:819.
Uheida, A., Giraldo Mejía, H., Abdel-Rehim, M., Hamd, H., and Dutta, J. (2021). Visible light photocatalytic degradation of polypropylene microplastics in a continuous water flow system. J. Haz. Mat. 406:124299. doi: 10.1016/j.jhazmat.2020.124299
Verma, R., Singh, S., Dalai, M. K., Saravanan, M., Agrawal, V. V., and Srivastava, A. K. (2017). Photocatalytic degradation of polypropylene film using TiO2-based nanomaterials under solar irradiation. Mater. Des. 133, 10–18. doi: 10.1016/j.matdes.2017.07.042
Wright, S. L., Thompson, R. C., and Galloway, T. S. (2013). The physical impact of microplastics on marine organisms: a review. Environ. Poll. 178, 483–492. doi: 10.1016/j.envpol.2013.02.031
Yousif, E., Al-Amiery, A. A., Kadihum, A., Kadhum, A. H., and Mohamad, A. (2015). Photostabilizing efficiency of PVC in the presence of schiff bases as photostabilizers. Molecules 20, 19886–19899. doi: 10.3390/molecules201119665
Keywords: microplastics, ecotoxicity, photo-fenton system, aquatic organisms, degradation, by-products
Citation: Piazza V, Uheida A, Gambardella C, Garaventa F, Faimali M and Dutta J (2022) Ecosafety Screening of Photo-Fenton Process for the Degradation of Microplastics in Water. Front. Mar. Sci. 8:791431. doi: 10.3389/fmars.2021.791431
Received: 08 October 2021; Accepted: 28 December 2021;
Published: 04 February 2022.
Edited by:
Cristina Panti, University of Siena, ItalyReviewed by:
Muhammad Zaffar Hashmi, COMSATS University, Islamabad Campus, PakistanDomingos Lusitâneo Pier Macuvele, Federal University of Santa Catarina, Brazil
Copyright © 2022 Piazza, Uheida, Gambardella, Garaventa, Faimali and Dutta. This is an open-access article distributed under the terms of the Creative Commons Attribution License (CC BY). The use, distribution or reproduction in other forums is permitted, provided the original author(s) and the copyright owner(s) are credited and that the original publication in this journal is cited, in accordance with accepted academic practice. No use, distribution or reproduction is permitted which does not comply with these terms.
*Correspondence: Abdusalam Uheida, salam@kth.se