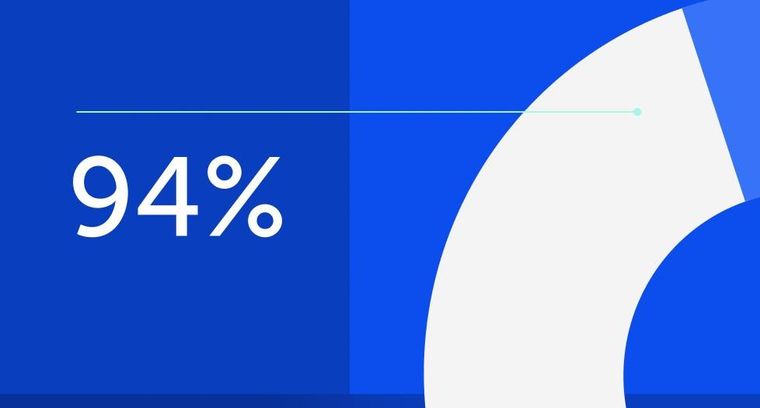
94% of researchers rate our articles as excellent or good
Learn more about the work of our research integrity team to safeguard the quality of each article we publish.
Find out more
ORIGINAL RESEARCH article
Front. Mar. Sci., 04 July 2019
Sec. Marine Ecosystem Ecology
Volume 6 - 2019 | https://doi.org/10.3389/fmars.2019.00370
This article is part of the Research TopicResearch and Management of Eutrophication in Coastal EcosystemsView all 23 articles
The Wadden Sea is a shallow intertidal coastal sea, largely protected by barrier islands and fringing the North Sea coasts of Netherlands, Germany, and Denmark. It is subject to influences from both the North Sea and major European rivers. Nutrient enrichment from these rivers since the 1950s has impacted the Wadden Sea ecology including loss of seagrass, increased phytoplankton blooms, and increased green macroalgae blooms. Rivers are the major source of nutrients causing Wadden Sea eutrophication. The nutrient input of the major rivers impacting the Wadden Sea reached a maximum during the 1980s and decreased at an average pace of about 2.5% per year for total Nitrogen (TN) and about 5% per year for total Phosphorus (TP), leading to decreasing nutrient levels but also increasing N/P ratios. During the past decade, the lowest nutrient inputs since 1977 were observed but these declining trends are leveling out for TP. Phytoplankton biomass (measured as chlorophyll a) in the Wadden Sea has decreased since the 1980s and presently reached a comparatively low level. In tidal inlet stations with a long-term monitoring, summer phytoplankton levels correlate with riverine TN and TP loads but stations located closer to the coast behave in a more complex manner. Regional differences are observed, with highest chlorophyll a levels in the southern Wadden Sea and lowest levels in the northern Wadden Sea. Model data support the hypothesis that the higher eutrophication levels in the southern Wadden Sea are linked to a more intense coastward accumulation of organic matter produced in the North Sea.
Increased nutrient fluxes to the coastal ocean are one of the main drivers for coastal change, having a pronounced impact on phytoplankton biomass and primary production, harmful algae blooms, seagrass, green macroalgae blooms, and water transparency (Cloern, 2001; Boesch, 2002; Orth et al., 2006; Smetacek and Zingone, 2013). Given the continuous increase in anthropogenic nitrogen fixation and increasing needs for nitrogen fertilizers (Battye et al., 2017), a further increase in the above mentioned eutrophication symptoms can be expected (e.g., Breitburg et al., 2018). First signs of coastal eutrophication like enhanced algae blooms were already observed in the 1950s and in Europe. Decisions to combat eutrophication were taken since the 1970s and 1980s (de Jong, 2007). Decreasing eutrophication trends have been observed for instance in European waters like the North Sea, Danish coastal waters or in the Baltic (e.g., Carstensen et al., 2006; Emeis et al., 2015; Andersen et al., 2017) during the 2000s or in Chesapeake Bay (Harding et al., 2016; Lefcheck et al., 2018) showing that a reversal of eutrophication is possible.
In the present paper, we will describe and compare the eutrophication levels and trends of the international Wadden Sea, a shallow coastal sea along the Dutch, German and Danish North Sea coast, based on long-term observations on nutrients and phytoplankton biomass (chlorophyll a). Since the earliest nutrient measurements in the Wadden Sea during the mid-20th century (Postma, 1954, 1966; Hickel, 1989) a strong increase in nitrogen and phosphorus concentrations has been documented (e.g., de Jonge and Postma, 1974; Hickel, 1989) reaching maximum values during the 1980s and 1990s (Jung et al., 2017) and decreasing since (e.g., van Beusekom et al., 2001, 2009; Cadée and Hegeman, 2002). Among the regularly occurring negative effects associated with the increased nutrient inputs are an increased import of organic matter from the North Sea to the Wadden Sea (de Jonge and Postma, 1974), more intense Phaeocystis-blooms (Cadée and Hegeman, 1986), a decline in seagrass distribution (de Jonge and de Jong, 1992), and increased green macroalgal cover (Reise and Siebert, 1994). Measures were taken during the 1970s and 1980s to address eutrophication (de Jong, 2007) through a reduction of riverine nutrient inputs to the North Sea.
Several studies have described local changes in Wadden Sea nutrient dynamics with a focus on the Dutch Wadden Sea (e.g., de Jonge and Postma, 1974; Cadée and Hegeman, 2002; Philippart et al., 2007) and the Northfrisian Wadden Sea (e.g., Hickel, 1989; van Beusekom et al., 2009). A comparison of available data for the entire Wadden Sea on phytoplankton and nutrients was only carried out within the framework of so-called Wadden Sea Quality Status Reports (e.g., van Beusekom et al., 2017). In these reports, the international scientific Wadden Sea community regularly compiles data on the ecological status of the North Sea (see for instance Wolff et al., 2010), showing among others a general decline in eutrophication levels.
Special attention will be given in the present study to long time series and regional differences in eutrophication levels covering the entire Wadden Sea. We will use two previously developed and tested eutrophication proxies (e.g., van Beusekom et al., 2001, 2009; van Beusekom and de Jonge, 2002) to describe temporal trends and regional differences. The proxies include autumn values of NH4 + NO2 as an indicator of organic matter turnover and summer phytoplankton chlorophyll a as an indicator of phytoplankton growth potential.
One of the processes responsible for the high productivity of Wadden Sea ecosystem is import of organic matter from the North Sea (Verwey, 1952; Postma, 1954). van Beusekom et al. (1999) compiled organic matter budgets from the Wadden Sea supporting the heterotrophic nature of the Wadden Sea with a primary production level of around 200–300 g C m–2 y–1, remineralization levels of about 300–450 g C m–2 y–1, and an annual import of about 100 g C m–2 y–1. This import is an important driver of nutrient availability in the Wadden Sea (e.g., de Jonge and Postma, 1974; van Beusekom et al., 1999, 2012; van Beusekom and de Jonge, 2002). The import mechanism is as follows (e.g., van Beusekom et al., 2012): Transport of particles (both inorganic and organic) from the North Sea to the Wadden Sea is induced by residual, baroclinic circulation similar to estuarine circulation (e.g., Burchard et al., 2008; Flöser et al., 2011; Hofmeister et al., 2017) with bottom currents directed on average toward the coast, and surface currents directed toward the sea. This traps sinking particles from a large part of the coastal North Sea, transports them toward the Wadden Sea and prevents particles to escape from the Wadden Sea to the North Sea. By the same token, dissolved nutrients, released after organic matter remineralization and not taken up within in the Wadden Sea, can be transported back to the North Sea (e.g., Grunwald et al., 2010). As light conditions are generally much better outside the Wadden Sea, these nutrients can be taken up by phytoplankton, and are transported back into the Wadden Sea after settling into deeper water layers (e.g., Postma, 1981; Maerz et al., 2016). Hofmeister et al. (2017) demonstrated with a model that the transport of sinking phytoplankton and detritus toward the Wadden Sea is able to keep up a nutrient gradient between the North Sea and the Wadden Sea.
We will use a biogeochemical North Sea model (Kerimoglu et al., 2017) to investigate regional differences in the import of organic matter from the North Sea to the Wadden Sea. Model approaches are needed because available budgets are too coarse to resolve regional differences. Also, no recent carbon budgets are available that demonstrate changes in Wadden Sea eutrophication levels. We will show that with the two proxies -summer chlorophyll a and autumn NH4 + NO2, a general decrease in eutrophication can be linked to decreasing riverine nutrient loads. We will further show that high organic matter import rates in the southern part of the Wadden Sea lead to higher eutrophication level than in the northern part of the Wadden Sea.
The Wadden Sea is a shallow intertidal coastal sea along the Dutch, German, and Danish North Sea coast (Figure 1). Tidal range is about 1 m at the northern and western end and increases to more than 3 m in the central part. At tidal ranges below 3 m, barrier islands can be formed (e.g., Hayes, 1975; Oost and de Boer, 1994), protecting the southern and northern Wadden Sea, but are absent in the central part. About 50% of the sediments emerge during low tide. Sediments are mostly sandy in the more exposed parts, whereas in the more protected parts muddy sediments prevail. Major nutrient discharges directly into the southern Wadden Sea are the IJsselmeer and the Ems river. Major rivers entering the central Wadden Sea are the Weser and Elbe. Residual currents along the Wadden Sea follow the anti-clockwise circulation of the North Sea. Thus, nutrient loads of the Rhine and Maas are carried northward and westward toward the Wadden Sea especially impacting the southern Wadden Sea. The Weser and Elbe nutrient loads are carried northward especially impacting the northern Wadden Sea (Figure 1).
Figure 1. Map of the Wadden Sea with stations having long time series starting before 1990. The Southern Wadden Sea includes the Dutch and Lower Saxonian part, the northern Wadden includes the Schleswig Holstein and the Danish part. The inset show the position of the rivers Rhine and Maas as well as the general circulation pattern along the Wadden Sea.
Salinity ranges in most tidal inlets on average between about 27 and 31. A clear seasonality is observed with lowest values in winter and highest values in summer (e.g., van Aken, 2008a; van Beusekom et al., 2008; Grunwald et al., 2010). Deviations from long-term means are related to freshwater discharge (e.g., van Beusekom et al., 2008). Closer to the mainland, and especially near fresh water sources, lower salinities prevail (e.g., van Aken, 2008a; Grunwald et al., 2010).
Temperature ranges on average between minimum values of around 3°C in February and around 18°C in July/August (e.g., Diederich et al., 2005; van Aken, 2008b; Grunwald et al., 2010) with an increasing trend (Martens and van Beusekom, 2008).
Phytoplankton shows a clear seasonal cycle, mainly due to light limitation in winter (e.g., Loebl et al., 2009). The spring phytoplankton bloom is dominated by diatoms (e.g., Cadée, 1986; Loebl et al., 2007), mainly occurs during April, but can be earlier during cold winters (van Beusekom et al., 2009). It is often followed by a Phaeocystis-bloom during early summer (e.g., Cadée and Hegeman, 2002; Loebl et al., 2007).
Nutrient seasonal cycles reflect nutrient uptake by primary producers, but the shape depends on the nutrient species. Silica (H4SiO4) and nitrate (NO3) show a simple cycle with highest values during winter and low values during summer due to uptake by phytoplankton (e.g., van Bennekom et al., 1974; van Beusekom et al., 2008; Leote et al., 2016). Ammonium (NH4) and nitrite (NO2) also show high winter and low summer values but with a clear autumn maximum (e.g., Postma, 1966; van Beusekom and de Jonge, 2002; Loebl et al., 2007; Reckhardt et al., 2015). Phosphate (PO4) reaches lowest values during spring and early summer, but concentrations already increase during May/June (e.g., de Jonge and Postma, 1974; Loebl et al., 2007; Grunwald et al., 2010; van Beusekom and de Jonge, 2012). An overview of mean seasonal nutrient cycles in the Wadden Sea until the mid 1990s is given in van Beusekom et al. (2001).
Riverine nutrient input data were compiled by Lenhart and Pätsch (2004) and updated to 2017. Total nitrogen (TN) and total phosphorus (TP) concentrations, taken from stations within the freshwater part of the estuary near the river mouth, were linearly interpolated between observations (mostly about every 2 weeks) to achieve estimates of daily concentrations. These nutrient concentrations estimates were then multiplied with daily discharges values taken from the last tidal free gauge station to derive daily loads. Data are available through https://wiki.cen.uni-hamburg.de/ifm/ECOHAM/DATA_RIVER.
For the Dutch Wadden Sea, a coherent observational program with methods applicable to the Wadden Sea is carried out by the Dutch governmental agency Rijkswaterstaat since 1977. Frequency of the sampling is fortnightly and samples are taken between 2 h before or after high tide. Water samples are filtered and analyzed for dissolved nutrients according to CEN/ISO guidelines and intercalibrated. Chlorophyll is measured by HPLC and is intercalibrated. During the past decades, the number of stations that are regularly sampled has decreased and at present five stations are continuously monitored (Figure 1). All Dutch monitoring data can be downloaded from the internet1.
Nutrients and phytoplankton are monitored at the island of Norderney since 1987. The exact position of the sampling station has changed during the time series within a radius of 2 km around Norderney harbor. Sampling was carried out at high tide, but includes a period with additional sampling at low tide from 2005 to 2014. Differences in chlorophyll a and NH4 + NO2 between these samplings is small (∼1 μg chla/l or ∼6% and ∼2–3 μmol/l or ∼22% for NH4 + NO2; compare van Beusekom et al., 2017). In this study, we have combined the data. Nutrients were filtered (0.4 μm), originally analyzed after Grasshoff et al. (1976, 1983) and later using continuous flow analysis (CFA) using German standard (DIN) methods. Labs are quality assured through interlaboratory comparisons: LÜRV (nationally) and Quasimeme (internationally). Data cannot be retrieved from a public data base but all data can be requested from NLWKN.
Regular sampling in the List tidal basin (Northfrisian Wadden Sea) was started in 1984 by the Biologische Anstalt Helgoland, now part of the Alfred-Wegener-Institut, Helmholtz-Zentrum für Polar- und Meeresforschung (AWI). Two stations (one in the main channel and one in the entrance to the Königshafen bay) were sampled twice weekly irrespective of the tidal phase. Until 1998, unfiltered nutrient samples were analyzed, afterward water samples were filtered through 0.4 Nuclepore polycarbonate filters and stored frozen until analysis. The impact of filtration on nutrient concentrations was not investigated systematically, but except for phosphate no clear shifts were observed in the time series. Nutrients were analyzed manually (NO3 with CFA) after Grasshoff et al. (1983) and intercalibrated (Quasimeme). Chlorophyll was analyzed spectrophotometrically after Jeffrey and Humphrey (1975) and intercalibrated. Data are deposited in the Pangaea database (www.pangaea.de). Details can be found in van Beusekom et al. (2009).
The Danish Wadden Sea has been monitored since 1989. All measurements are according to Danish standards2. In short, chlorophyll was extracted with ethanol and the absorbance is measured spectrophotometrically. Nutrients were filtered (GF/F or Advantec GF75) and analyzed using standard wet chemical techniques. In contrast to the other time series, NO2 is not assessed separately. Labs must participate in quality assurance programs (Quasimeme) to assure comparability of results. Data are accessible3.
We use simulation data from Kerimoglu et al. (2017) to discuss the mechanisms controlling the cross-shore gradients between the North Sea and the Wadden Sea. The three-dimensional coupled model describes the biogeochemical processes within the southeastern North Sea, coupled to the General Estuarine Transport Model (Burchard and Bolding, 2002), defined on a curvilinear grid of 1.5–4 km and terrain-following 20 vertical layers. For the period 2000–2010, his model showed high skill in reproducing the spatial and temporal distribution of salinity, nutrients and chlorophyll; and in particular, capturing the steep cross-shore gradients between the North Sea and the Wadden Sea (Kerimoglu et al., 2017). We extracted transects of particulate organic nitrogen (PON), salinity and residual, cross-shore currents between the ∼40 m depth contour and the Wadden Sea for the summer (May – September) of 2010 near the long-term stations, and present here the temporal average results.
In order to have an equal distribution of data over time, we binned data into monthly means. Handling and statistics were done with R (R Core Team, 2016), using the graphical package ggplot2 (Wickham, 2016). Processing and plotting of the model data was performed with nco4 and Python 2.7.
Two eutrophication proxies were used: summer chlorophyll a and autumn levels of the NH4 + NO2. Summer chlorophyll a levels were the mean of the monthly means for May – September. We did not include spring values, as the intensity of the spring bloom at least in the northern Wadden Sea is closely related to temperature, with highest biomasses after cold winters (van Beusekom et al., 2009) probably due to low grazer activity. Autumn NH4 + NO2 levels were the mean of the monthly means for September to November. As for the Danish time series, no NO2 values were reported and we estimated the NO2 contribution as 25% of the NH4 levels based on the nearby AWI time series from the Northfrisian Wadden Sea. The proxies are based on earlier work (e.g., van Beusekom et al., 2001; van Beusekom and de Jonge, 2002). Support for the usefulness of these proxies was given by van Beusekom and de Jonge (2012), who showed good correlations between summer dissolved organic phosphorus concentrations and the above eutrophication proxies.
As a drivers of the Wadden Sea eutrophication we used riverine TN loads and TP loads as suggested by van Beusekom et al. (2001). Instead of annual loads we used the loads until August as later riverine input cannot impact the production of organic matter during Summer. In case of the rivers Rhine and Maas, we extended the time series to include the December loads of the previous year as suggested by van Beusekom et al. (2001), among others based on the fact that the first high winter discharges are in December and on the longer distance between river mouth and Wadden Sea as compared to the Elbe and Weser. Riverine discharges directly into the Wadden Sea like the river Ems and lake IJsselmeer can have strong local effects (e.g., de Jonge and Postma, 1974; de Jonge, 1990; Jung et al., 2017), but less on the adjacent tidal basins. We therefore used only riverine TN and TP loads by the rivers Rhine/Maas as a common driver for the entire southern Wadden Sea. These two rivers dominate the riverine nutrient concentrations in the North Sea off the southern Wadden Sea. The rivers Rhine and Maas account for around 80% of the nutrient loads, with IJsselmeer, Ems and Noordzeekanaal representing 20%. Moreover, Rhine + Maas loads correlate with the combined loads of Rhine, Maas, IJsselmeer, Ems, and Noordzeekanaal with an r2 > 0.98.
We used the TN and TP loads by the rivers Rhine and Maas as the main driver of the southern Wadden Sea nutrient dynamics, and the TN and TP loads by the rivers Weser and Elbe as the main driver of the northern Wadden Sea nutrient dynamics. Discharge (Figure 2) has a major impact on the riverine loads showing a high interannual variability with highest discharges in the 1980s, and at least for the Rhine/Maas rivers a decreasing tendency of peak discharge. Riverine TN and TP loads reflect the annual discharge (Figure 3) but in addition show a clear decreasing trend in both river systems. As discharge during the 1980s was higher than present, riverine TN loads were up to three times higher and the riverine TP loads were up to five times higher than present levels. TP decreased faster than TN. In the Rhine/Maas, molar N/P ratios continuously increased from around 25 in the 1980s to about 80 during recent years. In the rivers Weser/Elbe, N/P molar ratios increased from 40 in the 1980s, to about 60–70 in the 1990s, decreasing to recent levels of about 45 (Figure 4). Flow-weighed TN concentrations (annual nutrient load/annual discharge, Figure 5) reached a maximum around 1985, regularly decreasing at a rate of around 2.5% per year until 2017. Flow-weighed TP was decreasing faster, with a rate of about 8% until 1990 in both system. Whereas in the Rhine/Maas this trend continues, the decrease in TP loads (2.5%) of the Weser/Elbe is much slower after ∼1990. Overall, we observed a decrease in flow-weighed concentrations of about 50% (TN) and 70–85% (TP), respectively.
Figure 2. Annual discharge of major rivers impacting the southern Wadden Sea (Rhine, Maas) and the Northern Wadden Sea (Weser, Elbe).
Figure 3. Total nitrogen (TN) load and total phosphorus (TP) load of major rivers impacting the southern Wadden Sea (Rhine, Maas) and the northern Wadden Sea (Weser, Elbe).
Figure 4. Molar N/P Ratio of the major rivers impacting the southern Wadden Sea (Rhine, Maas) and the northern Wadden Sea (Weser, Elbe).
Figure 5. Flow-weighed TN Load (annual TN load/annual discharge) and Flow-weighed TP Load (annual TP load/annual discharge) of major rivers impacting the southern Wadden Sea (Rhine, Maas) and the Northern Wadden Sea (Weser, Elbe).
Mean summer chlorophyll a concentrations (Chl) in the southern part of the Wadden Sea ranged between 2.6 and 48 μg Chl /l (Figure 6) with a median of 11.8 μg Chl /l. Most Dutch time series showed a significant decrease over time (Figure 6 and Table 1). The clearest trends were observed in the tidal inlet stations “Marsdiep Noord” (NL), Vliestroom (NL), and “Huibertgat oost” (NL). At the station “Doove Balg west” (NL) situated more closer to the coast, the trend was significant but with a higher variability. The time series at Norderney (D) also showed a significant decrease. The combined time series of the four southern Wadden Sea tidal inlet stations with a decreasing trend (Figure 7) reveal a general pattern with initially increasing chlorophyll a levels peaking in 1987, decreasing afterward.
Table 1. Summary statistics of the temporal trends in summer chlorophyll (May – September) and autumn (NH4 and NO2).
Figure 7. Trends of summer chlorophyll a for long-term stations in the tidal inlets of the southern Wadden Sea [boxplot of Marsdiep noord (NL), Vliestroom (NL), Huibertgat oost, (NL) Norderney (D)], and in the northern Wadden Sea (Sylt, D).
In contrast to the southern Wadden Sea, summer chlorophyll a levels were much lower in the northern Wadden Sea ranging, between 2.0 and 16.9 μg Chl /l and with median of 6.9 μg Chl /l. A significant decreasing trend was only observed for the longer Sylt (D) time series and no trends were found for the Danish Wadden Sea time series. The contrast between the Sylt and the southern tidal inlet stations of Netherlands and Lower Saxony is clearly shown in Figure 7. It is noteworthy, that with decreasing chlorophyll a levels during recent years, regional differences were becoming increasingly smaller.
Seasonal dynamics of nitrogen-containing nutrients (NH4, NO2, and NO3) in the Wadden Sea showed decreasing values during spring, low values in summer and increasing concentrations from September onward (e.g., van Beusekom and de Jonge, 2002; van Beusekom et al., 2009), due to decreasing light availability and subsequent decreasing nutrient uptake rates (see section “Area Description”). We used the summed autumn concentrations of NH4 and NO2 as a proxy of organic matter turnover, as these compounds are mainly produced locally by degradation processes. We did use NO3, although NO3 is also produced locally by degradation processes and nitrification. However, in contrast to NH4 and NO2, NO3 presently is the main form of nitrogen in rivers. Thus, both riverine input and local degradation processes may impact Wadden Sea NO3 concentrations (van Beusekom and de Jonge, 2002). Autumn concentrations of NH4 + NO2 have decreased significantly at all southern Wadden Sea stations, whereas in the northern Wadden Sea a significant decrease was only observed at the Danish Wadden Sea station Grådyb (Figure 8 and Table 1). As for chlorophyll a, the autumn NH4 + NO2 concentrations in the southern Wadden Sea (median: 11.9 μmol/l) were substantially higher than in the northern Wadden Sea (median: 6.0 μmol/l). It is important to note that at the Dutch Station Dantziggat, a significant decrease in autumn NH4 + NO2 was observed.
Most summer chlorophyll a time series showed significant correlations with riverine nutrient loads (Figure 9). It made no clear difference whether TN or TP was used as causative factor as evident by very similar r2 values (Table 2 for TN and Table 3 for TP). As for the temporal trends, correlations between riverine loads and chlorophyll a are clearest for the tidal inlet stations of the southern Wadden Sea [Marsdiep noord (NL), Vliestroom (NL), Huibertgat oost (NL), and Norderney(D)], weak for Doove Balg west (NL), and absent for Dantziggat (NL). The northern Wadden Sea stations all show a weak but significant correlation with lower r2 values. In most cases, riverine TN loads provided slightly better predictions than riverine TP loads. We also observed strong correlation between autumn NH4 + NO2 and riverine nutrient loads in the southern Wadden Sea (Figure 10), with no clear differences between TP, and TN as predictors. In the northern Wadden Sea, correlations between autumn NH4 + NO2 and riverine nutrient loads were not significant, except for TP in Grådyb (DK). Both proxies, summer chlorophyll a and autumn NH4 + NO2, were significantly correlated (p << 0.001, r2 = 0.26), and both identify the southern Wadden Sea as having the highest eutrophication levels.
Figure 9. Correlations between riverine TN loads and summer chlorophyll a (μg/l; May – September). For the southern Wadden Sea stations, Marsdiep noord (NL), Vliestroom (NL), Doove Balg west (NL), Dantziggat (NL), Huibertgat oost (NL) and Norderney (D), and the combined loads of the Rhine and Maas were used (December of the previous year until August of a given year). For the northern Wadden Sea stations, Sylt (D), Knude Dyb outer (DK), Grådyb inner (DK), and the combined loads of the Weser and Elbe were used (January – August).
Table 2. Summary statistics of the correlation between riverine Total Nitrogen loads and summer chlorophyll (May – September) and autumn (NH4 and NO2).
Table 3. Summary statistics of the correlation between riverine total phosphorus loads and summer chlorophyll (May – September) and autumn (NH4 and NO2).
Figure 10. Correlations between riverine TN loads and autumn NH4 + NO2 (μmol/l; September – November). For the southern Wadden Sea stations, Marsdiep noord (NL), Vliestroom (NL), Doove Balg west (NL), Dantziggat (NL), Huibertgat oost (NL) and Norderney (D), and the combined loads of the Rhine and Maas were used (December of the previous year until August of a given year). For the northern Wadden Sea stations, Sylt (D), Knude Dyb outer (DK), Grådyb inner (DK), and the combined loads of the Weser and Elbe were used (January – August).
Model results (see Kerimoglu et al., 2017) show, that within the regions where a thermohaline stratification occurs, PON concentrations at the bottom layers are slightly higher than at the surface layers within the deep (>20 m) regions (Figure 11). This, in combination with an estuarine-like circulation mentioned above, where the residual surface currents are directed toward off-shore and the bottom currents directed toward the shore, results in a net PON transport toward the shore, thus contributing to the eutrophication of the Wadden Sea. The efficiency of this transport mechanism varies between different regions: The intensity of the bottom transport is much higher along the southern Wadden Sea with average values of about 2 cm/s compared to the northern Wadden Sea (1 cm/s or less). Also, the PON concentrations are in general higher along the southern Wadden Sea than along the northern Wadden Sea.
Figure 11. Transects of mean currents (arrows) and particulate organic nitrogen (colors) and salinity (isolines; thicker isoline indicates 32 g/kg, thinner ones indicate each 1 g/kg lower increment) averaged over the period May – September 2010 for three areas along the Dutch Wadden Sea (green), along the Lower Saxonian Wadden Sea (red), and along the Schleswig-Holstein Wadden Sea (blue). For a better readability, to one arrow in each transect the current velocity was added.
The present results show that riverine nutrients are a major driver of the long term phytoplankton and nitrogen dynamics in the Wadden Sea. An essential link is the primary production of organic matter in the North Sea, import of primary produced organic matter from the North Sea into the Wadden Sea and remineralization within the Wadden Sea (van Beusekom and de Jonge, 2002). Sediments are a major site of organic matter remineralization and constitute a significant sources of nutrients (e.g., Beck and Brumsack, 2012). In shallow coastal settings, benthic, and pelagic contribute about equally to the total organic matter turn-over (Heip et al., 1995). Wadden Sea carbon budgets support this (van Beusekom et al., 1999). Grunwald et al. (2010) estimated nutrient fluxes from the Lower Saxonian Wadden Sea to the North Sea based on high resolution water column nutrient measurement from an automatic measurement station. They concluded, that particulate nutrients have to be imported from the North Sea to balance the export of inorganic nutrients. Grunwald et al. (2010) further identified pore water discharge as a major nutrient source. The release of nutrients from organic matter remineralization in sediments may be fast in permeable sediments, when surface sediments are rapidly flushed (e.g., Huettel and Rusch, 2000; Ehrenhauss et al., 2004; de Beer et al., 2005). Sediments may also trap nutrients on longer time scales, if transport of pore water to deeper sediment layers is involved (e.g., Beck et al., 2008; Røy et al., 2008). This internal storage of nutrients may induce lag effects in the response of the Wadden Sea to changes in eutrophication levels. Jung et al. (2017) constructed a nutrient budget for the Western Dutch Wadden Sea based on long-term nutrient measurements. They explicitly accounted for sediment import from the North Sea as this part of the Wadden Sea has a sediment deficit due to the construction of a dike (Afsluitdijk) between the Wadden Sea and the former Zuiderzee (now IJsselmeer, see Figure 1). Jung et al. (2017) concluded that during the initial eutrophication phase (until 1981) P and N were imported. A net export of N was observed since 1981 and for P since 1992.
Despite the potential role of sediments to buffer nutrients, a rapid response to changing riverine N loads can be deduced from work by van Beusekom and de Jonge (2002). They showed that during wet years substantially more NH4 + NO2 is released in autumn than during dry years. Also phytoplankton dynamics respond quite fast to changes in riverine nutrient loads (compare Figures 2, 7): During the 1980s, high riverine TN and TP concentrations and high discharges caused peak loads and high chlorophyll a levels during the late 1980s. This was followed by a period (1989–1993) of very low discharges, strongly reduced loads, and rapidly decreasing chlorophyll a levels. This suggests a rapid response of the phytoplankton to prevailing nutrient availability despite a potential nutrient buffering by sediments. Also, the present analysis does not suggests a major role of N and P legacies in sediments on the long term eutrophication levels, as the river loads (reflecting interannual dynamics) are a better predictor of the eutrophication level than a simple linear (smoothed) temporal trend. This is in line with the conclusions of Jung et al. (2017).
Nutrient ratios in rivers impacting the Wadden Sea have increased toward high N/P ratios clearly above the Redfield ratio, with potential consequences for the coastal food webs (see below). Wadden Sea sediments play an important role in lowering (and thus improving) these ratios in the Wadden Sea and adjacent North Sea: On the one hand, denitrification rates are high (e.g., Gao et al., 2009; Deek et al., 2012) removing significant amounts of N, on the other hand, sediments have a high capacity to bind and buffer PO4 (e.g., Leote and Epping, 2015).
Phytoplankton dynamics in the Wadden Sea are governed by a multitude of factors including zooplankton grazing (e.g., Loebl and van Beusekom, 2008), macrobenthos filter feeder activity (e.g., Cadée and Hegeman, 1974; Asmus and Asmus, 1993), light availability (Colijn, 1982), nutrient limitation (Loebl et al., 2008), or interactive effects of light limitation and nutrients (Colijn and Cadée, 2003; Loebl et al., 2009). We will discuss, whether trends in these three factors -grazing, light limitation and nutrient limitation- may have impacted the overall decreasing trends observed in the Wadden Sea during the last 3 decades.
Grazing by benthic filter feeders or (micro)zooplankton may have a large impact on phytoplankton dynamics. However, the observed recent decline in phytoplankton biomass is most probably not due to an increase in grazing pressure: For Wadden Sea zooplankton, only one time series is available since 1984 as part of the AWI-Sylt time series. Long term trends show a longer copepod season, an increase in Acartia sp. in April-May but no clear overall trend in copepod abundance (Martens and van Beusekom, 2008). This is in line with observations from the Helgoland road time series, even showing an overall decline in zooplankton densities (Boersma et al., 2015). Macrozoobenthos densities in the Wadden Sea have been relatively stable during the past decades (Drent et al., 2017) or even decreased in the adjacent coastal zone (Meyer et al., 2018). Philippart et al. (2007) did not observe a clear decrease in macrobenthos biomass in the western Dutch Wadden Sea but did observe a decrease in filter capacity by the macrobenthos. Taken together, no convincing evidence exists to support that recent long-term phytoplankton decline is driven by an increased top-down control. Whereas the long term trend in summer phytoplankton biomass is not driven by top-down control, short term dynamics, and interannual difference in summer phytoplankton biomass can be related to grazer-phytoplankton interactions. The tight coupling between phytoplankton growth rates and microzooplankton grazing rates in summer was demonstrated by Loebl and van Beusekom (2008). In the inner German Bight (Helgoland), Wiltshire et al. (2015) concluded that zooplankton grazing may control phytoplankton dynamics.
There has been a debate whether light, N or P limit phytoplankton dynamics in the Wadden Sea (e.g., de Jonge, 1990; Colijn and Cadée, 2003; Philippart et al., 2007). Colijn and Cadée (2003) used a method by Cloern (1999) to derive from time series, whether phytoplankton growth is limited by nitrogen or light and concluded that light is the dominating limiting factor, especially at Wadden Sea stations close to the coast like in the Dollard (inner part of the Ems estuary), with some N-limited periods during summer at Marsdiep, Norderney, Ems, and Büsum Mole (52.12°N, 8.86°E). Loebl et al. (2009) extended this analysis to also cover Si and P. They also concluded that during most of the year light is limiting, followed by Si during most of the growth season for diatoms, P limitation during the summer, and N limitation toward the end of the growth season.
The decreasing trend in phytoplankton biomass could potentially be caused by an decrease in light availability. Available evidence, however, suggests increasing light availability in the Wadden Sea and North Sea. Philippart et al. (2013) analyzed 4 decades of Secchi depth reading and concluded no overall change. Long-term trends (their Figure 7) suggest a slight decrease in light availability in the western Dutch Wadden Sea between the mid-1970s until the mid-1990s followed by an increase. This is in line with de Jonge and de Jong (2002) describing a general increase in annual suspended matter concentrations in the Western Dutch Wadden Sea from the 1950s to the mid 1980s, then decreasing until the 2000s. de Jonge and de Jong (2002) related changes in suspended matter concentrations to dredging activities in the Rhine Estuary and Rhine discharge. In the German Bight, Gebuhr et al. (2009) described a general increase in Secchi depth from the mid 1970s. In general, light conditions in the coastal North Sea and Wadden Sea apparently improved rather than deteriorated, suggesting that light is not the dominant factor in the long-term phytoplankton decrease observed in most of the Wadden Sea. Thus, the observed correlation between summer chlorophyll a levels and riverine nutrient loads supports that nutrients are a dominant factor in the long-term decline of phytoplankton. The low correlation coefficients do indicate, however, that many other factors and their interactions modulate the long-term phytoplankton dynamics. For instance, better light conditions would also increase microphytobenthos activity, possibly leading to reduced nutrient fluxes from the sediment (e.g., Sundbäck et al., 2000).
As in most of the Wadden Sea phytoplankton biomass recently decreased, the increase at the station Danziggat in the Dutch Wadden Sea is striking. The increase can be due to an increased accumulation of phytoplankton, an increased growth potential or reduced loss factors in this part of the Wadden Sea. The decreasing trend in autumn NH4 + NO2 contrasts with the chlorophyll time series and suggests that increased nutrient availability was not responsible for the observed phytoplankton, but hints at improved growth condition e.g., due to increasing light conditions or decreasing grazing rates or filter rates. Suspended matter concentrations at Danziggat are higher than at the other western Dutch Wadden Sea stations (de Jonge and de Jong, 2002), suggesting stronger light limitation here than at the other Dutch long-term stations. Possibly, strong light limitation prevailed also during summer at this station during the early 1980s. We hypothesize that improved light conditions since the 1980s (see section “Discussion”) enabled enhanced phytoplankton production in this part of the Wadden Sea leading to increasing phytoplankton biomass (compare Figure 6).
Both riverine N and P loads have been used to explain long-term trends in phytoplankton biomass and primary production (e.g., de Jonge, 1990; van Beusekom et al., 2001). Our analyses show good correlations between summer chlorophyll a with both N and P. This indicates that N and P availability ultimately determine the eutrophication levels of the Wadden Sea. However, they do not provide conclusive indications whether N or P are ultimately limiting the phytoplankton dynamics.
Riverine P loads decreased faster than the N loads leading to high N/P ratios. Although no large differences were observed in the response of the phytoplankton biomass to decreasing loads of either riverine N or P, the changes of the N/P ratio may have implications on coastal communities. Malzahn et al. (2007) investigated food chain effects of N limited and P limited phytoplankton (Rhodomonas sp.) on Copepods (Acartia sp.) and fishlarvae (Clupea harengus). The P-limited food chain resulted in larval fish with a significantly poorer condition than N-limited or nutrient-sufficient food chains. Meunier et al. (2018) showed that long-term shifts in dissolved PO4 concentration in the North Sea were closely linked to biomass trends of heterotrophic dinoflagellates and corroborated this experimentally. These results are interesting in the light of a study by Philippart et al. (2007) on the impact of nutrient reductions in the western Dutch Wadden Sea on phytoplankton, macrozoobenthos and estuarine birds. In their study, they used the IJsselmeer (see Figure 1) nutrient loads instead of the combined Rhine Maas loads used in the present study. They observed a weak correlation of P loads with the phytoplankton biomass but stronger correlations with macrozoobenthos and estuarine birds. Kerimoglu et al. (2018) studied the potential changes of phytoplankton in the North Sea under pre-industrial nutrient input scenario (about 30% of present levels) and suggested that the nutrient limitation led to disproportionately lower zooplankton biomass relative to the phytoplankton biomass (expressed as C content). This implied reduced grazing pressure, contributing in turn to a limited sensitivity of phytoplankton, and in certain regions, even to a slight phytoplankton increase under those lower nutrient input rates. Although we did not find support whether N or P was the ultimate limiting element for phytoplankton biomass formation, the impact of increasing N/P ratios on food quality definitely needs further attention.
Both proxies – summer phytoplankton and autumn NH4 + NO2 – are correlated, but with a large spread (r2 = 0.26). This correlation does not necessarily reflect a causal relation between the two proxies, but rather is the result of a common underlying driver: nutrient availability. Both proxies are impacted by different factors: Phytoplankton is positively influenced by light and nutrient availability but negatively by grazing. Autumn NH4 + NO2 is positively influenced by organic matter accumulation, but negatively by phytoplankton/microphytobenthos nutrient uptake and nitrification rates and high exchange with the North Sea during autumn. Only enhanced organic matter/nutrient availability is a common factor increasing both proxies. Other factors increasing the phytoplankton growth potential like better light conditions or decreasing grazing rates would lead to higher summer chlorophyll a levels but lower autumn levels of NH4 + NO2 due to increased phytoplankton nutrient uptake during autumn. Therefore, similar long-term trends of both proxies but no high correlation coefficient between the proxies is expected.
Based on the above discussion we suggest, that due to decreased riverine nutrient loads both summer chlorophyll a – as an indicator of the phytoplankton biomass and phytoplankton growth potential – and the autumn concentrations of NH4 + NO2 as an indicator of organic matter turnover intensity have declined over the entire Wadden Sea. Both proxies independently identify the southern Wadden Sea as having a higher eutrophication level than the northern Wadden Sea. Model data (Figure 11) support that import of organic matter from the North Sea to the Wadden Sea is larger along the southern Wadden Sea than along the northern Wadden Sea. An analysis of satellite data and in situ suspended matter data (Schartau et al., 2019) supports the model results by showing sharper gradients along the southern Wadden Sea than along the northern Wadden Sea. However, further model analyses including a quantification of transport rates based on multidecadal runs performed on higher resolution setups (see e.g., Gräwe et al., 2016; Androsov et al., 2019; Stanev et al., 2019) are needed to better understand the relative contribution of estuarine circulation and direct river inputs on the maintenance of horizontal gradients within the Wadden Sea.
Both model data and the eutrophication proxies identify the southern Wadden Sea as having a higher eutrophication level than the northern Wadden Sea. We will discuss, whether these patterns are also observed in other ecosystem components that respond to nutrient-enrichment like green macroalgae, seagrass, or benthic filter feeders.
Green macroalgae blooms can be due to coastal eutrophication with consequences for local economies, tourism, and the ecological state of coastal ecosystems (Fletcher, 1996; Smetacek and Zingone, 2013). In the Wadden Sea, green macroalgae blooms increased from the 1970s onward (Reise and Siebert, 1994; Kolbe et al., 1995; Schories et al., 1997), reaching a maximum during the 1990s (Kolbe et al., 1995; van Beusekom et al., 2017). Since the 1990s, a general decline to very low levels is observed for the northern Wadden Sea, but the decline in the German part of the southern Wadden Sea is less clear and a high growth potential is still present (van Beusekom et al., 2017). The decline in the northern Wadden Sea and remaining blooms in the southern Wadden Sea are in line with a higher eutrophication level in the southern Wadden Sea.
Seagrass is an important component of coastal ecosystems (e.g., Costanza et al., 1997; Orth et al., 2006), but globally declining at an increased rate (Waycott et al., 2009). Many factors have contributed to the seagrass decline in the entire Wadden Sea during the 20th century, including Wasting Disease, habitat destruction through embankment, increased turbidity, increased storminess, and eutrophication (e.g., Den Hartog, 1987; de Jonge and de Jong, 1992; Kastler and Michaelis, 1997; Dolch et al., 2013). Since the late 1990s, seagrass is clearly recovering in the northern Wadden Sea (Dolch et al., 2013) but not in the southern Wadden Sea (Dolch et al., 2017), where present seagrass distribution is less than expected on the basis of habitat preferences (Folmer et al., 2016). Although multiple stressors are involved, eutrophication probably has played a major role in seagrass decline (e.g., Bittick et al., 2018). Indeed, the recovery of Chesapeake Bay submerged vegetation is directly related to reduction in nutrient loads (Lefcheck et al., 2018). We hypothesize, that if riverine nutrient loads further decrease, seagrass occurrence in the Southern Wadden Sea will increase.
Recent distribution patterns of mussel beds also show regional differences, with the southern Wadden Sea having highest shares of tidal basin surface occupied by mussel beds (Folmer et al., 2014). The authors stress that many factors are involved, but ruled out temperature and extreme wind effects. Food availability is one of the factors involved and would be in line with the regional difference in organic matter and phytoplankton availability shown in this paper. In this context, it is important to note that macrozoobenthos biomass off the German southern Wadden Sea showed a clear decline, that was linked to a decrease in eutrophication (Meyer et al., 2018).
To summarize, more opportunistic macroalgae, a higher share of mussel beds and less seagrass in the southern Wadden Sea as compared to the northern Wadden Sea are all in agreement with a higher nutrient and organic matter availability in the southern Wadden Sea.
Whereas the overall regional distribution patterns of the eutrophication proxies are in agreement with other ecosystem components that respond to nutrient-enrichment, it should be borne in mind that part of the regional differences can be due to timing of sampling with regard to the tidal phase. Grunwald et al. (2010) reported higher nutrient concentrations at low tide than at high tide. Dutch monitoring stations are sampled around high tide, whereas the other stations [Grådyb (DK) and List tidal basin(D)] are sampled independently of the tidal phase. Since 2005, the Norderney (D) time series were sampled both during low and during high tide. Whereas differences for summer chlorophyll a were small (∼6%), autumn NH4 + NO2 concentrations at high tide were about 22% (2–3 μmol/l) lower than at low tide. The overall effect would be that the Dutch eutrophication levels are slightly underestimated as compared to the other stations. The Norderney temporal trend is probably slightly underestimated due to slightly higher autumn values during recent years by including low tide values since 2005.
Long time series are pivotal for understanding how ecosystems respond to both man-made and natural changes. The long history of Wadden Sea eutrophication is described best by the work done at the Royal Netherlands Institute for Sea Research (NIOZ) with a focus on the western Dutch Wadden Sea. Available data suggest that eutrophication already started from the 1950s onward (e.g., de Jonge, 1990), culminating in the 1980s (e.g., Cadée and Hegeman, 2002). Our study extends the description of Wadden Sea eutrophication to the entire Wadden Sea. The longest time series on phytoplankton/chlorophyll a are available for the Dutch Wadden Sea. They document an increase in phytoplankton from the mid 1970s to the mid 1980s and a decrease since. The Norderney and Sylt time series starting in the 1980s document the decreasing trends, whereas the Danish time series starting at the end of the 1980s only weakly reflect the decreasing eutrophication levels. Riverine nutrient loads are important drivers of phytoplankton dynamics and correlated significantly with summer phytoplankton biomass (as chlorophyll a), but only explained about 30% of interannual variability, suggesting the importance of other factors. These may include both biological factors like the balance between grazing and growth, but also physical factors like the import of organic matter (as a source of nutrients) from the North Sea.
Given the high variability of phytoplankton in response to riverine nutrient loads, it is important to have additional proxies that indicate the intensity of organic matter turnover. We show that the mean autumn concentration of the remineralization products NH4 + NO2 in autumn (when decreasing light levels limit phytoplankton productivity) is a useful proxy. Especially in the southern Wadden Sea, this proxy is significantly linked to riverine nitrogen loads suggesting its driving role in Wadden Sea organic matter turnover. Both proxies – summer chlorophyll a and autumn NH4 + NO2^– are significantly correlated. The best correlations between the eutrophication proxies and riverine nutrient loads are found for stations in tidal inlets, probably because of less stronger interactions with the sediment than at the shallower, more coastward stations.
Regional differences are apparent, with long-term summer chlorophyll levels and autumn NH4 + NO2^– levels clearly higher in the southern Wadden Sea than in the northern Wadden Sea. The model by Kerimoglu et al. (2017) supports, that these differences are driven by a higher organic matter import from the North Sea into the Wadden Sea. These regional differences suggest a lower eutrophication potential in the northern Wadden Sea as evidenced by lower phytoplankton biomass, lower organic matter turnover (autumn release of NH4 and NO2), seagrass recovery and decreasing green algae blooms.
The present data show, that measures taken to reduce riverine nutrient loads have led to a decrease in phytoplankton biomass in the Wadden Sea and adjacent coastal zone, and contributed to an improved ecological status. However, long, well designed long time-series with a high temporal resolution are needed to derive statistically significant correlations due to complex physical and ecological interactions, resulting in a strong interannual variability. Given the world-wide increase in the use of fertilizers (Battye et al., 2017), it can be expected that eutrophication problems will increase world-wide. The present example of the Wadden Sea underlines that measures to reduce nutrient enrichment do lead to an improvement of the coastal ecological status.
This article is an extended version of a contribution to the Wadden Sea Quality Status Report on Eutrophication lead by JvB with contributions from JC, TD, AG, HL, KK, JP, HR, and JR. JvB conceived the study, analyzed the data, and wrote the first draft of the manuscript. JC, AG, LR, HR, and JR provided the chlorophyll and nutrient data. TD and KK specifically contributed to the discussion of seagrass and macroalgae. HL and JP provided the riverine input data. RH and OK contributed with the analysis of the model data. All authors contributed to the discussion of the manuscript.
The authors declare that the research was conducted in the absence of any commercial or financial relationships that could be construed as a potential conflict of interest.
We thank the three reviewers for their constructive comments that significantly improved the manuscript.
Andersen, J. H., Carstensen, J., Conley, D. J., Dromph, K., Fleming-Lehtinen, V., Gustafsson, B. G., et al. (2017). Long-term temporal and spatial trends in eutrophication status of the Baltic Sea. Biol. Rev. 92, 135–149. doi: 10.1111/brv.12221
Androsov, A., Fofonova, V., Kuznetsov, I., Danilov, I., Rakowsky, N., Harig, S., et al. (2019). FESOM-C v.2: coastal dynamics on hybrid unstructured meshes. Geosci. Model Dev. 12, 1009–1028. doi: 10.5194/gmd-12-1009-2019
Asmus, H., and Asmus, R. M. (1993). Phytoplankton-mussel bed interactions in intertidal ecosystems. Estuar. Coast. Ecosyst. Process. 33, 57–84. doi: 10.1007/978-3-642-78353-1_3
Battye, W., Aneja, V. P., and Schlesinger, W. H. (2017). Is nitrogen the next carbon? Earths Future 5, 894–904. doi: 10.1002/2017ef000592
Beck, M., and Brumsack, H.-J. (2012). Biogeochemical cycles in sediment and water column of the wadden sea: the example spiekeroog island in a regional context. Ocean Coast. Manage. 68, 102–113. doi: 10.1016/j.ocecoaman.2012.05.026
Beck, M., Dellwig, O., Liebezeit, G., Schnetger, B., and Brumsack, H.-J. (2008). Spatial and seasonal variations of sulphate, dissolved organic carbon, and nutrients in deep pore waters of intertidal flat sediments. Estuar. Coast. Shelf Sci. 79, 307–316. doi: 10.1016/j.ecss.2008.04.007
Bittick, S. J., Sutula, M., and Fong, P. (2018). A tale of two algal blooms: negative and predictable effects of two common bloom-forming macroalgae on seagrass and epiphytes. Mar. Environ. Res. 140, 1–9. doi: 10.1016/j.marenvres.2018.05.018
Boersma, M., Wiltshire, K. H., Kong, S.-M., Greve, W., and Renz, J. (2015). Long-term change in the copepod community in the southern German bight. J. Sea Res. 101, 41–50. doi: 10.1016/j.seares.2014.12.004
Boesch, D. F. (2002). Challenges and opportunities for science in reducing nutrient over-enrichment of coastal ecosystems. Estuaries 25, 886–900. doi: 10.1007/bf02804914
Breitburg, D., Levin, L. A., Oschlies, A., Grégoire, M., Chavez, F. P., Conley, D. J., et al. (2018). Declining oxygen in the global ocean and coastal waters. Science 359:eaam7240. doi: 10.1126/science.aam7240
Burchard, H., and Bolding, K. (2002). GETM: A General Estuarine Transport Model Scientific Documentation. Technical Report EUR 20253EN. Brussels: European Commission.
Burchard, H., Flöser, G., Staneva, J., Badewien, T. H., and Riethmüller, R. (2008). Impact of density gradients on net sediment transport into the wadden Sea. J. Phys. Oceanogr. 38, 566–587. doi: 10.1175/2007jpo3796.1
Cadée, G. C. (1986). Recurrent and changing seasonal patterns in phytoplankton of the westernmost inlet of the Dutch Wadden Sea from 1969 to 1985. Mar. Biol. 93, 281–289. doi: 10.1007/BF00508265
Cadée, G. C., and Hegeman, J. (1974). Primary production of phytoplankton in the dutch wadden Sea. Neth. J. Sea. Res. 3, 240–259. doi: 10.1016/0077-7579(74)90019-2
Cadée, G. C., and Hegeman, J. (1986). Seasonal and annual variation in Phaeocystis pouchetii (Haptophyceae) in the westernmost inlet of the wadden sea during the 1973 to 1985 period. Neth. J. Sea Res. 20, 29–36. doi: 10.1016/0077-7579(86)90058-x
Cadée, G. C., and Hegeman, J. (2002). Phytoplankton in the Marsdiep at the end of the 20th century; 30 years monitoring biomass, primary production, and Phaeocystis blooms. J. Sea Res. 48, 97–110. doi: 10.1016/s1385-1101(02)00161-2
Carstensen, J., Conley, D. J., Andersen, J. H., and Ærtebjerg, G. (2006). Coastal eutrophication and trend reversal: a danish case study. Limnol. Oceanogr. 51, 398–408. doi: 10.4319/lo.2006.51.1_part_2.0398
Cloern, J. E. (1999). The relative importance of light and nutrient limitation of phytoplankton growth: a simple index of coastal ecosystem sensitivity to nutrient enrichment. Aquat. Ecol. 33, 3–16.
Cloern, J. E. (2001). Our evolving conceptual model of the coastal eutrophication problem. Mar. Ecol. Prog. Ser. 210, 223–253. doi: 10.3354/meps210223
Colijn, F. (1982). Light absorption in the waters of the ems - dollard estuary and its consequences for the growth of phytoplankton and microphytobenthos. Neth. J. Sea Res. 15, 196–216. doi: 10.1016/0077-7579(82)90004-7
Colijn, F., and Cadée, G. C. (2003). Is phytoplankton growth in the wadden sea light or nitrogen limited? J. Sea Res. 49, 83–93. doi: 10.1016/s1385-1101(03)00002-9
Costanza, R., d’Arge, R., De Groot, R., Farber, S., Grasso, M., Hannon, B., et al. (1997). The value of the world’s ecosystem services and natural capital. Nature 387:253.
de Beer, D., Wenzhöfer, F., Ferdelman, T., Boehme, S. E., Huettel, M., van Beusekom, J. E. E., et al. (2005). Transport and mineralization rates in north sea sandy intertidal sediments, sylt-rømø basin, wadden Sea. Limnol. Oceanogr. 50, 113–127. doi: 10.4319/lo.2005.50.1.0113
de Jong, F. (2007). Marine Eutrophication in Perspective: on the Relevance of Ecology for Environmental Policy. Berlin: Springer Science & Business Media.
de Jonge, V. N. (1990). Response of the dutch wadden sea ecosystem to phosphorus discharges from the river rhine. Hydrobiologia 195, 49–62. doi: 10.1007/978-94-009-2000-2_5
de Jonge, V. N., and de Jong, D. J. (1992). Role of tide, light and fisheries in the decline of Zostera marina L. in the dutch wadden sea. Neth. Inst. Sea Res. Pub. Ser. 20, 161–176.
de Jonge, V. N., and de Jong, D. J. (2002). ’Global change’ impact of inter-annual variation in water discharge as a driving factor to dredging and spoil disposal in the river rhine system and of turbidity in the Wadden Sea. Estuar. Coast. Shelf Sci. 55, 969–991. doi: 10.1006/ecss.2002.1039
de Jonge, V. N., and Postma, H. (1974). Phosphorus compounds in the Dutch Wadden Sea. Neth. J. Sea Res. 8, 139–153. doi: 10.1016/0077-7579(74)90014-3
Deek, A., Emeis, K., and van Beusekom, J. E. E. (2012). Nitrogen removal in coastal sediments of the German Wadden Sea. Biogeochemistry 108, 467–483. doi: 10.1007/s10533-011-9611-1
Den Hartog, C. (1987). “Wasting disease” and other dynamic phenomena in zostera beds. Aquat. Bot. 27, 3–14. doi: 10.1016/0304-3770(87)90082-9
Diederich, S., Nehls, G., van Beusekom, J. E. E., and Reise, K. (2005). Introduced Pacific oysters (Crassostrea gigas) in the northern Wadden Sea: invasion accelerated by warm summers? Helgol. Mar. Res. 59, 97–106. doi: 10.1007/s10152-004-0195-1
Dolch, T., Buschbaum, C., and Reise, K. (2013). Persisting intertidal seagrass beds in the northern Wadden Sea since the 1930s. J. Sea Res. 82, 134–141. doi: 10.1016/j.seares.2012.04.007
Dolch, T., Folmer, E. O., Frederiksen, M. S., Herlyn, M., van Katwijk, M. M., Kolbe, K., et al. (2017). “Seagrass,” in Wadden Sea Quality Status Report 2017, eds S. Kloepper, et al. (Wilhelmshaven: Common Wadden Sea Secretariat).
Drent, J., Bijkerk, R., Herlyn, M., Grotjahn, M., Voß, J., Carausu, M.-C., et al. (2017). “Macrozoobenthos,” in Wadden Sea Quality Status Report 2017, eds S. Kloepper, et al. (Wilhelmshaven: Common Wadden Sea Secretariat).
Ehrenhauss, S., Witte, U., Janssen, F., and Huettel, M. (2004). Decomposition of diatoms and nutrient dynamics in permeable North Sea sediments. Cont. Shelf Res. 24, 721–737. doi: 10.1016/j.csr.2004.01.002
Emeis, K. C., van Beusekom, J., Callies, U., Ebinghaus, R., Kannen, A., Kraus, G., et al. (2015). The north sea–a shelf sea in the anthropocene. J. Mar. Syst. 141, 18–33.
Fletcher, R. (1996). “The occurrence of “green tides” – a review,” in Marine Benthic Vegetation -Ecological Studies, ed. P. Schramm Nienhuis (Berlin: Springer).
Flöser, G., Burchard, H., and Riethmüller, R. (2011). Observational evidence for estuarine circulation in the German Wadden Sea. Cont. Shelf Res. 31, 1633–1639. doi: 10.1016/j.csr.2011.03.014
Folmer, E. O., Drent, J., Troost, K., Büttger, H., Dankers, N., Jansen, J., et al. (2014). Large-scale spatial dynamics of intertidal mussel (Mytilus edulis L.) bed coverage in the German and Dutch Wadden Sea. Ecosystems 17, 550–566. doi: 10.1007/s10021-013-9742-4
Folmer, E. O., van Beusekom, J. E. E., Dolch, T., Grawe, U., van Katwijk, M. M., Kolbe, K., et al. (2016). Consensus forecasting of intertidal seagrass habitat in the Wadden Sea. J. Appl. Ecol. 53, 1800–1813. doi: 10.1111/1365-2664.12681
Gao, H., Schreiber, F., Collins, G., Jensen, M. M., Kostka, J. E., and Lavik, G. (2009). Aerobic denitrification in permeable Wadden Sea sediments. ISME J. 4:417. doi: 10.1038/ismej.2009.127
Gebuhr, C., Wiltshire, K. H., Aberle, N., van Beusekom, J. E. E., and Gerdts, G. (2009). Influence of nutrients, temperature, light and salinity on the occurrence of Paralia sulcata at helgoland roads. North Sea. Aquat. Biol. 7, 185–197. doi: 10.3354/ab00191
Grasshoff, K., Erhardt, M., and Kremling, K. (1976). Methods of Seawater Analysis. Weinheim: Verlag Chemie.
Grasshoff, K., Erhardt, M., and Kremling, K. (1983). Methods of Seawater Analysis. Weinheim: Verlag Chemie.
Gräwe, U., Flöser, G., Gerkema, T., Duran-Matute, M., Badewien, T., Schulz, E., et al. (2016). A numerical model for the entire Wadden Sea: skill assessment and analysis of hydrodynamics. J. Geophys. Res. Oceans 121, 5231–5251. doi: 10.1002/2016JC011655
Grunwald, M., Dellwig, O., Kohlmeier, C., Kowalski, N., Beck, M., Badewien, T. H., et al. (2010). Nutrient dynamics in a back barrier tidal basin of the southern north sea: time-series, model simulations, and budget estimates. J. Sea Res. 64, 199–212. doi: 10.1016/j.seares.2010.02.008
Harding, L. W., Gallegos, C. L., Perry, E. S., Miller, W. D., Adolf, J. E., Mallonee, M. E., et al. (2016). Long-term trends of nutrients and phytoplankton in chesapeake bay. Estuaries Coast. 39, 664–681. doi: 10.1007/s10661-008-0680-0
Hayes, M. O. (1975). “Morphology and sand accumulations in estuaries,” in Estuarine Research 2: Geology and Engineering, ed. L. E. Cronin (New York, NY: Academic Press), 3–22. doi: 10.1016/b978-0-12-197502-9.50006-x
Heip, C. H. R., Goosen, N. K., Herman, P. M. J., Kromkamp, J., Middelburg, J. J., and Soetaerd, K. (1995). Production and consumption of biological particles in temperate tidal estuaries. Oceanogr. Mar. Biol. 33, 1–149.
Hickel, W. (1989). “Inorganic micronutrients and the eutrophication in the wadden sea of sylt (german bight, north sea),” in Proceedings of the 21st Conference of EMBS, (Gdansk: Polish Academy of Science).
Hofmeister, R., Flöser, G., and Schartau, M. (2017). Estuary-type circulation as a factor sustaining horizontal nutrient gradients in freshwater-influenced coastal systems. Geo Mar. Lett. 37, 179–192. doi: 10.1007/s00367-016-0469-z
Huettel, M., and Rusch, A. (2000). Transport and degradation of phytoplankton in permeable sediments. Limol. Oceanogr. 43, 534–549. doi: 10.4319/lo.2000.45.3.0534
Jeffrey, S. A., and Humphrey, G. (1975). New spectrophotometric equation for determining chlorophyll a, b, c1 and c2. Biochem. Physiol. Pflanzen. 167, 191–194. doi: 10.1016/s0015-3796(17)30778-3
Jung, A., Brinkman, A., Folmer, E., Herman, P. M., van der Veer, H. W., and Philippart, C. (2017). Long-term trends in nutrient budgets of the western Dutch Wadden Sea (1976–2012). J. Sea Res. 127, 82–94. doi: 10.1016/j.seares.2017.02.007
Kastler, T., and Michaelis, H. (1997). The declining segrass beds in the Wadden Sea area of niedersachsen. Bericht Forschungsstelle Küste 41, 119–139.
Kerimoglu, O., Große, F., Kreus, M., Lenhart, H.-J., and van Beusekom, J. E. E. (2018). A model-based projection of historical state of a coastal ecosystem: relevance of phytoplankton stoichiometry. Sci. Total Environ. 639, 1311–1323. doi: 10.1016/j.scitotenv.2018.05.215
Kerimoglu, O., Hofmeister, R., Maerz, J., Riethmüller, R., and Wirtz, K. W. (2017). The acclimative biogeochemical model of the southern North Sea. Biogeosciences 14, 4499–4531. doi: 10.5194/bg-14-4499-2017
Kolbe, K., Kaminski, E., Michaelis, H., Obert, B., and Rahmel, J. (1995). Macroalgal development in the Wadden Sea: first experiences with a monitoring system. Helgol. Meeresunters 49, 519–528. doi: 10.1007/bf02368379
Lefcheck, J. S., Orth, R. J., Dennison, W. C., Wilcox, D. J., Murphy, R. R., Keisman, J., et al. (2018). Long-term nutrient reductions lead to the unprecedented recovery of a temperate coastal region. Proc. Natl. Acad. Sci. U.S.A. 115, 3658–3662. doi: 10.1073/pnas.1715798115
Lenhart, H.-J., and Pätsch, J. (eds) (2004). Daily loads of Nutrients, Total Alkalinity, Dissolved Inorganic Carbon and Dissolved Organic Carbon of the European Continental Rivers for the years 1977 - 2002. Berichte Aus Dem Zentrum für Meeres- und Klimaforschung. Reihe B: Ozeanographie. Berlin: Springer.
Leote, C., and Epping, E. H. G. (2015). Sediment–water exchange of nutrients in the marsdiep basin, western wadden sea: phosphorus limitation induced by a controlled release? Cont. Shelf Res. 92, 44–58. doi: 10.1016/j.csr.2014.11.007
Leote, C., Mulder, L. L., Philippart, C. J. M., and Epping, E. H. G. (2016). Nutrients in the western wadden sea: freshwater input versus internal recycling. Estuaries Coast. 39, 40–53. doi: 10.1007/s12237-015-9979-6
Loebl, M., Colijn, F., and van Beusekom, J. E. E. (2008). Increasing nitrogen limitation during summer in the list tidal basin (Northern Wadden Sea). Helgol. Mar. Res. 62, 59–65. doi: 10.1007/s10152-007-0089-0
Loebl, M., Colijn, F., van Beusekom, J. E. E., Baretta-Bekker, J. G., Lancelot, C., Philippart, C. J. M., et al. (2009). Recent patterns in potential phytoplankton limitation along the Northwest European continental coast. J. Sea Res. 61, 34–43. doi: 10.1016/j.seares.2008.10.002
Loebl, M., Dolch, T., and van Beusekom, J. E. E. (2007). Annual dynamics of pelagic primary production and respiration in a shallow coastal basin. J. Sea Res. 58, 269–282. doi: 10.1016/j.seares.2007.06.003
Loebl, M., and van Beusekom, J. E. E. (2008). Seasonality of microzooplankton grazing in the northern Wadden Sea. J. Sea Res. 59, 203–216. doi: 10.1016/j.seares.2008.01.001
Maerz, J., Hofmeister, R., Lee, E. M., Gräwe, U., Riethmüller, R., and Wirtz, K. W. (2016). Maximum sinking velocities of suspended particulate matter in a coastal transition zone. Biogeosciences 13, 4863–4876. doi: 10.5194/bg-13-4863-2016
Malzahn, A. M., Aberle, N., and Clemmesen, C. (2007). Nutrient limitation of primary producers affects planktivorous fish condition. Limnol. Oceanogr. 52, 2062–2071. doi: 10.4319/lo.2007.52.5.2062
Martens, P., and van Beusekom, J. E. E. (2008). Zooplankton response to a warmer northern Wadden Sea. Helgol. Mar. Res. 62, 67–75. doi: 10.1007/s10152-007-0097-0
Meunier, C. L., Alvarez-Fernandez, S., Cunha-Dupont, A. Ö, Geisen, C., Malzahn, A. M., Boersma, M., et al. (2018). The craving for phosphorus in heterotrophic dinoflagellates and its potential implications for biogeochemical cycles. Limnol. Oceanogr. 63, 1774–1784. doi: 10.1002/lno.10807
Meyer, J., Nehmer, P., Moll, A., and Kröncke, I. (2018). Shifting southern north sea macrofauna community structure since 1986: a response to de-eutrophication and regionally decreasing food supply? Estuar. Coast. Shelf Sci. 213, 115–127. doi: 10.1016/j.ecss.2018.08.010
Oost, A. P., and de Boer, P. L. (1994). Sedimentology and development of barrier islands, ebb-tidal deltas, inlets and backbarrier areas of the Dutch Wadden Sea. Senckenbergiana Maritima 24, 65–115.
Orth, R. J., Carruthers, T. J. B., Dennison, W. C., Duarte, C. M., Fourqurean, J. W., Heck, K. L., et al. (2006). A global crisis for seagrass ecosystems. BioScience 56, 987–996.
Philippart, C. J., Salama, M. S., Kromkamp, J. C., van der Woerd, H. J., Zuur, A. F., and Cadée, G. C. (2013). Four decades of variability in turbidity in the western Wadden Sea as derived from corrected secchi disk readings. J. Sea Res. 82, 67–79. doi: 10.1016/j.seares.2012.07.005
Philippart, C. J. M., Beukema, J. J., Cadée, G. C., Dekker, R., Goedhart, P. W., van Iperen, J. M., et al. (2007). Impact of nutrients on coastal communities. Ecosystems 10, 95–118.
Postma, H. (1954). Hydrography of the Dutch Wadden Sea. Arch. Néerl. Zool. 10, 405–511. doi: 10.1163/036551654x00087
Postma, H. (1966). The cycle of nitrogen in the Wadden Sea and adjacent areas. Neth. J. Sea Res. 3, 186–221. doi: 10.1016/0077-7579(66)90012-3
Postma, H. (1981). Exchange of materials between the North Sea and the Wadden sea. Mar. Geol. 40, 199–215.
R Core Team (2016). R. A Language and Environment for Statistical Computing. Vienna: R Foundation for Statistical Computing.
Reckhardt, A., Beck, M., Seidel, M., Riedel, T., Wehrmann, A., Bartholomä, A., et al. (2015). Carbon, nutrient and trace metal cycling in sandy sediments: a comparison of high-energy beaches and backbarrier tidal flats. Estuar. Coast. Shelf Sci. 159, 1–14. doi: 10.1016/j.ecss.2015.03.025
Reise, K., and Siebert, I. (1994). Mass occurrence of green algae in the German Wadden Sea. Deutsche Hydrographische Zeitschrift 1, 171–188.
Røy, H., Lee, J. S., Jansen, S., and de Beer, D. (2008). Tide-driven deep pore-water flow in intertidal sand flats. Limnol. Oceanogr. 53, 1521–1530. doi: 10.3389/fmicb.2017.02526
Schartau, M., Riethmüller, R., Flöser, G., van Beusekom, J. E. E., Krasemann, H., Hofmeister, R., et al. (2019). On the separation between inorganic and organic fractions of suspended matter in a marine coastal environment. Prog. Oceanogr. 171, 231–250. doi: 10.1016/j.pocean.2018.12.011
Schories, D., Albrecht, A., and Lotze, H. (1997). Historical changes and inventory of macroalgae from königshafen bay in the northern Wadden Sea. Helgol. Meeresunters 5, 321–341. doi: 10.1007/bf02908718
Smetacek, V., and Zingone, A. (2013). Green and golden seaweed tides on the rise. Nature 504, 84–88. doi: 10.1038/nature12860
Stanev, E. V., Jacob, B., and Pein, J. (2019). German bight estuaries: an inter-comparison on the basis of numerical modeling. Cont. Shelf Res. 174, 48–65. doi: 10.1016/j.csr.2019.01.001
Sundbäck, K., Miles, A., and Göransson, E. (2000). Nitrogen fluxes, denitrification and the role of microphytobenthos in microtidal shallow-water sediments: an annual study. Mar. Ecol. Prog. Ser. 200, 59–76. doi: 10.3354/meps200059
van Aken, H. M. (2008a). Variability of the salinity in the western Wadden Sea on tidal to centennial time scales. J. Sea Res. 59, 121–132. doi: 10.1016/j.seares.2007.11.001
van Aken, H. M. (2008b). Variability of the water temperature in the western Wadden Sea on tidal to centennial time scales. J. Sea Res. 60, 227–234. doi: 10.1016/j.seares.2008.09.001
van Bennekom, A. J., Krijgsman-van Hartingsveld, E., van der Veer, G. C. M., and van Voorst, H. F. J. (1974). The seasonal cycle of reactive silicate and suspended diatoms in the Dutch Wadden Sea. Neth. J. Sea Res. 8, 174–207. doi: 10.1016/0077-7579(74)90016-7
van Beusekom, J. E. E., Bot, P., Carstensen, J., Grage, A., Kolbe, K., Lenhart, H.-J., et al. (2017). “Eutrophication,” in Wadden Sea Quality Status Report, eds S. Kloepper, et al. (Wilhelmshaven: Common Wadden Sea Secretariat).
van Beusekom, J. E. E., Brockmann, U. H., Hesse, K. J., Hickel, W., Poremba, K., and Tillmann, U. (1999). The importance of sediments in the transformation and turnover of nutrients and organic matter in the Wadden Sea and German bight. Germ. J. Hydrogr. 51, 245–266. doi: 10.1007/bf02764176
van Beusekom, J. E. E., Buschbaum, C., and Reise, K. (2012). Wadden Sea tidal basins and the mediating role of the North Sea in ecological processes: scaling up of management? Ocean Coast. Manage. 68, 69–78. doi: 10.1016/j.ocecoaman.2012.05.002
van Beusekom, J. E. E., and de Jonge, V. N. (2002). Long-term changes in Wadden Sea nutrient cycles: importance of organic matter import from the North Sea. Hydrobiologia 475/476, 185–194. doi: 10.1007/978-94-017-2464-7_15
van Beusekom, J. E. E., and de Jonge, V. N. (2012). Dissolved organic phosphorus: an indicator of organic matter turnover? Estuar. Coast. Shelf Sci. 108, 29–36. doi: 10.1016/j.ecss.2011.12.004
van Beusekom, J. E. E., Fock, H., de Jong, F., Diel-Christiansen, S., and Christiansen, B. (2001). Wadden sea specific eutrophication criteria. Wadden Sea Ecosystem 14. Wilhelmshaven: Common Wadden Sea Secretariat.
van Beusekom, J. E. E., Loebl, M., and Martens, P. (2009). Distant riverine nutrient supply and local temperature drive the long-term phytoplankton development in a temperate coastal basin. J. Sea Res. 61, 26–33. doi: 10.1016/j.seares.2008.06.005
van Beusekom, J. E. E., Weigelt-Krenz, S., and Martens, P. (2008). Long-term variability of winter nitrate concentrations in the Northern Wadden Sea driven by freshwater discharge, decreasing riverine loads and denitrification. Helgol. Mar. Res. 62, 49–57.
Verwey, J. (1952). On the ecology of cockle and mussel in the Dutch Wadden Sea. Arch. Néerl. Zool. 10, 171–239.
Waycott, M., Duarte, C. M., Carruthers, T. J. B., Orth, R. J., Dennison, W. C., Olyarnik, S., et al. (2009). Accelerating loss of seagrasses across the globe threatens coastal ecosystems. Proc. Natl. Acad. Sci. U.S.A. 106, 12377–12381. doi: 10.1073/pnas.0905620106
Wiltshire, K. H., Boersma, M., Carstens, K., Kraberg, A. C., Peters, S., and Scharfe, M. (2015). Control of phytoplankton in a shelf sea: determination of the main drivers based on the helgoland roads time series. J. Sea Res. 105, 42–52.
Keywords: eutrophication indicators, Wadden Sea, North Sea, nutrients, long-term trends, phytoplankton, submersed vegetation, sediments
Citation: van Beusekom JEE, Carstensen J, Dolch T, Grage A, Hofmeister R, Lenhart H, Kerimoglu O, Kolbe K, Pätsch J, Rick J, Rönn L and Ruiter H (2019) Wadden Sea Eutrophication: Long-Term Trends and Regional Differences. Front. Mar. Sci. 6:370. doi: 10.3389/fmars.2019.00370
Received: 03 October 2018; Accepted: 14 June 2019;
Published: 04 July 2019.
Edited by:
Alberto Basset, University of Salento, ItalyReviewed by:
Paolo Magni, Italian National Research Council (CNR), ItalyCopyright © 2019 van Beusekom, Carstensen, Dolch, Grage, Hofmeister, Lenhart, Kerimoglu, Kolbe, Pätsch, Rick, Rönn and Ruiter. This is an open-access article distributed under the terms of the Creative Commons Attribution License (CC BY). The use, distribution or reproduction in other forums is permitted, provided the original author(s) and the copyright owner(s) are credited and that the original publication in this journal is cited, in accordance with accepted academic practice. No use, distribution or reproduction is permitted which does not comply with these terms.
*Correspondence: Justus E. E. van Beusekom, justus.van.beusekom@hzg.de
Disclaimer: All claims expressed in this article are solely those of the authors and do not necessarily represent those of their affiliated organizations, or those of the publisher, the editors and the reviewers. Any product that may be evaluated in this article or claim that may be made by its manufacturer is not guaranteed or endorsed by the publisher.
Research integrity at Frontiers
Learn more about the work of our research integrity team to safeguard the quality of each article we publish.