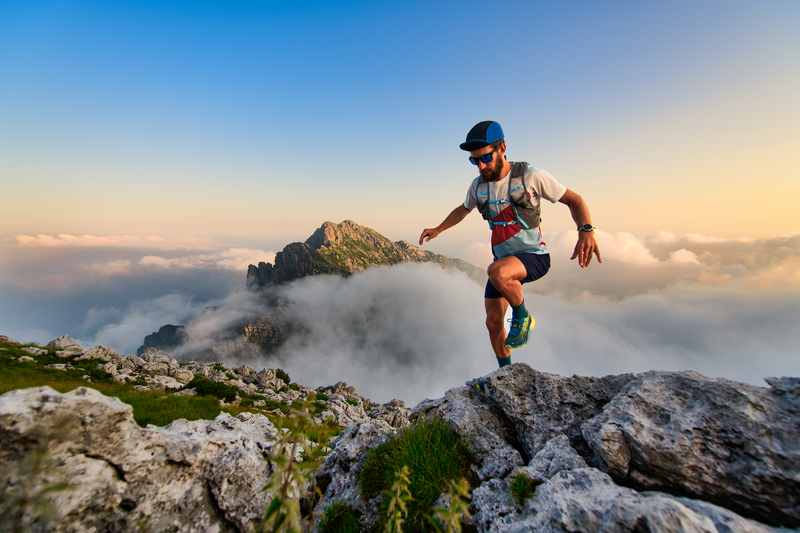
95% of researchers rate our articles as excellent or good
Learn more about the work of our research integrity team to safeguard the quality of each article we publish.
Find out more
ORIGINAL RESEARCH article
Front. Mar. Sci. , 30 March 2016
Sec. Marine Pollution
Volume 3 - 2016 | https://doi.org/10.3389/fmars.2016.00038
This article is part of the Research Topic Shipwreck risk assessment View all 6 articles
This paper is based on a series of measurements taken on WWII historic shipwrecks that resulted from the effects of Operation Hailstone in February 1944 on the Japanese merchant fleet which was assembled in Chuuk lagoon, Federated States of Micronesia. More than 65 shipwrecks and 250 aircraft were sunk during two main bombing raids. The vessels lost covered a wide range of underwater orientation and water depths and so provided a perfect suite of corrosion experiments. Since the fuel on board the aircraft was either readily burnt at the time or was lost through volatilization, the wrecked planes present no pollution problems today. However the bunker fuel kept inside on-board storage tanks does present a real conservation management crisis. In-situ measurements on many vessels have determined how water depth, the localized wreck topography, dissolved oxygen levels, temperature and salinity affects the corrosion rate of cast iron and mild steel. Thus corrosion rates can be calculated with confidence.
The effects of Operation Hailstone in February 1944 were devastating for the residual Japanese merchant fleet which was assembled in Chuuk lagoon, Federated States of Micronesia. Many of the large naval vessels had left the area in response to rumors of an attack, however more than 65 shipwrecks and 250 aircraft were sunk during two main bombing raids. The aerial attacks were placed 2 weeks apart to maximize their impact and launched from the United States Navy carrier fleet. The loss of so many vessels created a perfect suite of corrosion experiments since the wrecks were widely distributed in a variety of depths and aspects. Some of the wrecks are sitting proud on the sea bottom as if they are resting in a graving dock, while other vessels are found lying upside down while others are on their sides on the edges of sloping underwater structures such as cliffs, or simply abutting islands. Since the fuel on board the aircraft was either readily burnt at the time or was lost through volatilization the wrecked planes present no pollution problems today. However the bunker fuel kept inside on-board storage tanks does present a real conservation management crisis that is increasingly significant. The initial in-situ data was collected from 10 shipwrecks in 2002 and complimented by repeat measurements at selected sites and additional five wrecks in 2006, 2007 and finally in 2008. Sufficient information was obtained from these measurements to be able to determine how water depth, the localized wreck topography, dissolved oxygen levels, temperature and salinity affects the corrosion rate of cast iron and mild steel. Corrosion rates can be calculated with confidence since the main parameters determining the rate of deterioration of the iron wrecks have been determined and hence, the latent risk of oil leaking from the bunker fuel containers on board the shipwrecks can be assessed. Leaking aeroplane fuel from storage drums inside cargo holds presents a temporary fire hazard but the diesel fuels and bunker fuels which have the capacity to suffocate sessile organisms and poison fish present lingering environmental problems. Understanding the parameters that determine the corrosion rate of iron alloys in shallow tropical waters will also provide valuable information for marine scientists dealing with the issues of unexploded ordnance by quantifying the decay rates of the shell casings.
When an iron ship sinks and the sea water penetrates the protective coatings, corrosion of the underlying metal accelerates until the marine organisms colonize the substrate and begin the process of separating the anodic and cathodic reactions. In the overall corrosion cell oxygen is reduced at the sea water interface and iron is oxidized underneath the protective layer of marine growth (North, 1976). In order to satisfy electrical neutrality, chloride ions migrate in from the sea and so the final concentration of chloride can be easily three times the concentration found in normal sea water. As the concentration of metal ions increases they will undergo hydrolysis and produce a range of iron corrosion products that contain mixtures of oxide, hydroxide, and chloride ions as well as mixtures of iron carbonates since the acid from the hydrolysis reaction will go and dissolve calcareous minerals (North, 1982; Selwyn et al., 1999). As the pH increases, as acid is consumed during these reactions, a number of mixed corrosion products are produced such as siderite, FeCO3 the iron (II) carbonate. The precise characterisation of the nature of the primary and secondary corrosion products took decades of research to determine the precise nature of these corrosion matrices and part of the problem lay in the fact that researchers were working on samples brought to laboratories that were hundreds and thousands of kilometres away from the wrecks (North, 1982; Turgoose, 1982). By conducting research in an in situ environment many of these problems are overcome and the artefacts and wrecks themselves can speak directly to the conservators and “talk to them” in a way that allows the experience of the metal to be understood and interpreted for the benefit of heritage managers.
The primary iron corrosion product in a concretion microenvironment is ferrous chloride which is a weak acid that readily undergoes a hydrolysis reaction to produce protons and iron (II) hydroxy species viz.
These species undergo a series of subsequent reactions both at the metal interfaces and inside the concretion matrix. Since higher concentrations of ferrous ions provide a higher acidity for the interstitial fluids, it has been shown that the pH is a direct measure of the corrosion rate since a greater rate of the decay produces more ferrous ions and this in turn, through hydrolysis, provides a lower pH. Thus measurements of the in-situ acidity give a localized measure of the corrosion. There are a series of steps that need to be developed and undertaken before the precise correlation between in-situ pH and the corrosion rate can be established, but it only takes a few dives and collecting a dozen measurements until this has been established. In-situ pH measurements are done by using a pressure compensated flat surface combination pH electrode (BDH Gelplas) inserted into a hole drilled through the concretion to the metal interface. As the drill bit, which has the same nominal diameter as the plastic body of the electrode, is extracted the buddy diver inserts the pH electrode and gently but firmly pushes it against the exposed metal surface. Best results are obtained by using a silicone rubber annulus which is slid down onto the concretion to minimize water exchange. The pH is recorded as the minimum value which typically takes between 60 and 90 s to equilibrate. Following pH recording, the corrosion potential is measured with a platinum working and a Ag/AgCl flow through reference electrode connected to a digital multimeter housed in the polycarbonate box. The combined depth of concretion and corrosion is measured with a plastic Vernier caliper. Recent work has replaced the compressed air driven drills with a commercially available underwater battery operated drill (Nemo submersible DD180-3LI) which makes for easier and safer underwater operations.
It has been found that the thickness of the marine concretion is directly related to the availability of iron itself and also to the amount of phosphorus that is present in the iron alloy. Marine organisms suffer from the fact that iron, which is needed for the mitochondria in their cells, is a limiting resource in the marine environment. Since the pH of the surrounding sea water is quite alkaline the availability of the essential nutrient of iron is limited because of the insolubility of both iron (II) and iron (III) oxy-hydroxide species. The presence of high concentrations of these important reagents, under the protective envelope of the concretion, and other nutrients promotes the amount of marine growth on the submerged shipwreck. Additionally it has been found that anaerobic bacteria are capable of converting iron phosphide into a volatile form of a phosphine that is a stimulant to marine growth (Iverson and Olson, 1983; MacLeod, 1988). All the concretion data came from wrought and cast iron objects which had all corroded in shallow temperate coastal waters and that had not been subjected to diving tourism or events such as dynamite fishing. It was found that when the total thickness of the marine concretion is divided by the number of years of immersion then there is a linear relationship between the average rate of marine growth and the weight percent of phosphorus in the iron alloy. Because the composition of the steel used in the construction of the ships will naturally vary, the amount of marine growth will change in accordance with the amount of phosphorus in the iron alloys. This is specially noted in the nineteenth century wrought iron anchors which had cast iron balls on the extremities of the wrought iron stocks and the concretion is invariably much thicker on the cast balls than on the wrought sections of the anchor.
Conservators working on a number of the wrecks in Chuuk Lagoon have noted that lower quality steel used in the heavier sectioned elements such as deck knees and gunwales does tend to have thicker concretion cover, but this is in part due to the ease of colonization of such surfaces by marine organisms (see Figure 1). Typically the amount of concretion growing on cast iron is much greater than on wrought iron owing to the much higher concentration of phosphorus in the cast material. The other main variable in determining the thickness of concretion is the profile that the section on metal has in terms of the profile it presents to the flowing sea water. Since the colonizing marine organisms are sessile their growth rate depends on the supply of microscopic food sources such as plankton and so their growth rate will be dependent on the flux of the flowing sea water. Thus higher flux of water caused by objects having a greater profile will result in thicker concretions and these inherently provide more protection from corrosion to the underlying metal substrate.
Figure 1. Bow of the Fujikawa Maru showing thick concretion on the cast iron fittings that are most likely to have high phosphorus contents in the original metal.
With the knowledge of the pH, corrosion potential (Ecorr) and the depth of the wreck it is possible to determine the basic corrosion parameters that control the rate of deterioration once an additional parameter has been determined. The missing link in the development of a site specific corrosion equation is based on data obtained from the depth of graphitization of cast iron structures on the wrecks. By initially using a masonry bit and drilling into the marine concretion with a compressed air drill it is then possible to insert a micrometer and determine the combined depth of penetration of the concretion and corrosion product matrix. In order to determine the depth of graphitization of the cast iron alone, the drill bit is changed over to a 2.5 mm metal bit that can penetrate the corroded layers of cast iron once a small area of marine growth has been removed to expose the original profile of the object. The freshly drilled hole provides the corrosion depth which can be directly measured by insertion of the micrometer probe. The reason why the corrosion depth is referred to the depth of graphitization is that the corrosion process removes the iron-rich phases, such as ferrite and pearlite, which leaves behind a material that is rich in the graphitic phase, hence “graphitized” zone. When this depth is divided by the number of years of immersion of the wreck, then a corrosion rate expressed in terms of millimeters per year can be readily obtained.
Once this parameter is known it is possible to develop site specific corrosion equation that enables calculation of what a particular corrosion potential means in terms of a localized corrosion rate in millimeters per year. If the original specifications of the scantlings of the vessel are known and readings have been obtained of the total corrosion to date then it is possible to calculate the residual metal thickness and determine how many years remain until total metal loss. Naturally before the total loss of metal the structure will collapse under the combined weight of corrosion products as it reaches the point beyond the yield limit of the residual metal. Site equations take the general form of
i.e., the logarithm of the corrosion rate is related to the corrosion potential in a linear fashion. The value of a can be simply determined through a series of at least four measurements of the depths of graphitization of cast iron elements on a wreck and their corresponding corrosion potentials. At times when the access to parts of a site where appropriate cast iron fittings located is limited by depth constraints or by the tangled mass of wreckage, an alternative to obtaining multiple measurements exists. The value of a has been empirically determined to be controlled by salinity and temperature through the variable of the dissolved oxygen concentration through the equation,
In this expression the concentration of oxygen is recorded in cm3.dm−3 (MacLeod, 1995). This equation has been developed for salinity values ranging from 32 to 37o so additional measurements in areas of lower salinity such as the Baltic Sea and higher salinity found in parts of the Aegean Sea would be prudent.
The fact that the corrosion products hydrolyse in direct response to their analytical concentration means that the resultant level of acidity, as measured by the in-situ pH, is a direct measure of the localized corrosion rate at the point of measurement, provided that a quasi-equilibrium state has been reached. The most regularly visited site of the Fujikawa Maru where the data was collected over the depth range of 10–35 meters showed that there was a linear relationship between the log of the corrosion rate and the pH, viz.,
In this expression dg is the depth of graphitization (mm) divided by the number of years of immersion of the shipwreck. The advantage of this relationship is that the pH provides a localized measure of the corrosion rate, while the Ecorr value is more of an average of the areas that are electrically connected to the local corrosion cell.
Before conservation management decisions on a specific wrecksite can be made heritage managers need some degree of comfort that the data collected by the archaeological team is sufficiently reliable as to provide justification for particular courses of action. When appropriate reference materials are tested it can be seen that it is possible to get reproducible data from such in-situ measurements. The reproducibility of corrosion profiles is best illustrated through a set of measurements on the forward port and starboard bollards on the Fujikawa Maru which gave respective values of 6.5 and 6.6 mm of graphitization. Since underwater measurements on the depth of penetration of a drill-bit into the corroded (graphitized) cast iron can be measured to ±0.1 mm, it can be seen that for objects sitting in the same corrosion microenvironment the depth of decay is the same. Other objects located at the same depth were a windlass which had a corrosion depth of 8.4 mm (1.3 times the bollard rate)—this object was lying on the open deck and was raised approximately 30 cm above the plane of the deck. The base of the forward gun had a corrosion depth of 11.6 mm (1.8 times the bollard rate) and this object naturally had a much higher profile on the wreck so there is a much greater flux of oxygenated seawater moving over the surface of the massive gun than over the windlass which is greater than the low profile bollards. It is noted that within a large object, such as a cannon which may have corroded in several different microenvironments, there can be significant variability in the depth of corrosion. Cannon from the wreck of the American China trader Rapid (1811) had the same depth of corrosion at the muzzle and cascable ends when their archaeological context was the same but 10 mm for the exposed part fell to 5 mm at the cascable end (no 4031) which was buried under ballast (Carpenter and MacLeod, 1993). Part of the elevation of the gun base corrosion rate may be due to galvanic coupling between the cast iron base and the cast steel of the barrel. The Fujikawa Maru data illustrates the basic concept that pertains to the interpretation of the decay rates of different parts of an historic shipwreck. The greater the objects profile the greater the local turbulence obtained from the prevailing currents which results in a greater flux of dissolved oxygen and the concomitant greater corrosion rate (see Figure 2). Shallower parts of the wreck will tend to corrode at a faster rate as the amount of water movement around them is greater than the deeper parts of the vessel due to wave action being higher in shallower waters. Shallow wrecks also tend to have a higher degree of saturation of dissolved oxygen which also enhances the decay rate.
Having amassed a set of data from a series of in-situ measurements the overall corrosion mechanism can often be found through plotting the results and finding systematic relationships between the voltage and pH measurements. Plots of the voltage, in our case the Ecorr data, against the pH of the solution at the metal interface were developed by the Belgian electrochemist Marcel Pourbaix to show zones of chemical reactivity and areas of low corrosion rate (passive) and areas where corrosion cannot occur (immune). Practitioners can plot the Ecorr and pH data by placing the corrosion potentials on the ordinate axis and the corresponding pH reading on the abscissa and so plot the data in a type of Pourbaix diagram (Pourbaix, 1974). During the initial inspection of the Fujikawa Maru in 2002 it was found that the slope of the Ecorr vs. pH plot of the data in the Pourbaix diagram was −59 mV/pH, which is twice the average of −29.5 mV/pH most commonly found on historic iron shipwrecks. This slope dictates that the chemistry controlling the corrosion reaction releases one proton (H+) per electron, which is illustrated by the equation below viz.,
The slope of −59 mV/pH means that the initial measurements on the Fujikawa Maru showed that the pH was controlled by a different mechanism to all the other iron wrecks in Chuuk lagoon. The possible reasons why the Fujikawa Maru was apparently anomalous is consistent with a combination of its young age (58 years old at the time of the initial measurements) and the disposition of the wreck on the lagoon seabed. The wreck lies on its keel on a flat bottomed section of the lagoon floor and so it is undergoing very little mechanical stress and so this was a major difference to all the other Chuuk wrecks of the same age.
All the other vessels were found to be located on their sides (port or starboard), on slopes with either bow or stern up on underwater slopes or edges of cliffs, or indeed upside down in the case of the Yubae Maru. All the other wrecks gave data consistent with two protons (hydrogen ions) being released for each electron involved in the oxidation process. The corrosion mechanism for the other iron wrecks in Chuuk lagoon is the same as those found on historic iron shipwrecks in the open ocean viz.,
For this reaction scheme the Pourbaix slope is −29.5 mV/pH and only 4 years after the initial measurements (2006) the Fujikawa Maru had changed its corrosion mechanism to that of all the other historic iron shipwrecks (MacLeod, 2005, 2006). It appears that the initial measurements Fujikawa Maru we caught in the transition of a younger to an older shipwreck decay mechanism.
Having established the basic corrosion mechanism the next step is to assess the impact of water depth on both the values of the pH and the Ecorr. It was found that for the Fujikawa Maru and other wrecks popular with diving tourism such as the Gosei Maru and the Susuki patrol boat the pH versus depth data is characterized by a series of parallel lines. On any particular wrecksite many of the data points have the same dependence on depth (same slope), which indicates that the overall corrosion mechanism is the same, but there are different intercept (pH) values at zero depth. The different intercepts indicate that there have been events that have caused the chronology of decay to be interrupted, which is why there is a different pH value for the same water depth. It has been previously noted that once quasi-equilibrium has been established the pH is a direct measure of the corrosion rate. When concretion is sloughed off as a result of natural events such as typhoons, cyclones and intense storms the dissolved oxygen in the seawater has direct access to the corroding metal and so the corrosion rate is greatly accelerated. The rapid rate of decay continues until the surface is re-colonized and the marine organisms begin their work of encapsulation of the corroding iron and the pH begins to fall as the semi-permeable concretion sets up a diffusion barrier. This means that the amount of protective concretion formed on the surface of the corroding wreck is different on one part of the vessel compared with the other. Other forms of de-concretion include damage from moorings, anchors and from dynamite fishing. The latter practice involves using harvested WWII munitions and making bombs which are thrown into the water to kill fish for easy harvesting. The shock wave from the exploding munitions causes the fish swim bladders to malfunction and stunned and dying fish rapidly float to the surface of the lagoon. The shockwave from these explosions also causes the concretion to come away from the iron metal.
The difference in the intercept values on the pH axis in the case of the Fujikawa Maru is equivalent to a difference in the pH value of 0.83 or a factor of 6.8 times the difference in the underlying acidity of the metal interface (MacLeod, 2006). The greatest acidity levels are associated with the older concretion and these differences in pH in part reflect the differences in thickness of the marine growth. It takes time for equilibrium to be set up between the hydrolysis of the metal ions and the reactions between the acid and the concretion. The Chuuk lagoon data for the Fujikawa Maru shows that a certain minimum concretion thickness is needed before the pH values are truly indicative of the underlying corrosion rate (MacLeod et al., 2007). The impact of the dynamite fishing has resulted in the most popular wrecks, such as the Fujikawa Maru, having a corrosion rate which is approximately 46% higher than similar vessels at the same depth that have not been subjected to such human intervention (MacLeod and Richards, 2009). The effect on the wreck is high and can be dramatically seen in images of the upper works of the bridge, which now have many holes in it, where at a combination of corrosion and damage from mooring of a dive vessels has resulted in periodic shedding of the concretion and its protective coating from the iron metal, as seen in Figure 3.
Figure 3. View of the bridge of the Fujikawa Maru showing extensive corrosion loss due to accumulated effects of years of dynamite fishing.
Marine organisms such as corals have natural habits that cause them to branch out and capture more of the passing microorganisms that represent their food sources. Corals and their symbiotic relationship with marine algae mean that they are very dependent on the amount of light reaching the surface of the colonized shipwreck, since the algae need light for photosynthetic activity. It was found on several wrecks, including the Fujikawa Maru and the Susuki patrol boat, that when the vessel is inclined at an angle the sunny side has greater marine growth than the darker side. On the Susuki patrol boat that the darker port side was principally colonized by clams while the sunny side was dominated by a large variety of hard and soft corals. Measurements of the Ecorr and the pH data on the wreck showed that the pH of the sunny surfaces were more acidic than those found on corresponding parts of the wreck that were lying on the dark side. The increased acidity on the sunny side means a localized increase in corrosion rate has occurred because of the increased flux of dissolved oxygen across the concreted surface which was brought about by the sedentary epifauna.
Part of the role of the management team developing in-situ data collection is to ensure that there are marine biologists working underwater in conjunction with the diving conservators. The marine biologists record the local microenvironment at the measurement points by documenting the species, height and complexity of the colonizing organisms including observations on their size and height and as well as frequency. A measure of the complexity of the colonization is gained through measurements of the rugosity (Ru) or surface roughness of the marine concretions. By tracing a fine-linked chain across the diagonal of a rectangular grid near the location points the profile of the marine organisms the differing degrees of colonization can be determined. The rugosity is essentially the fraction of the length of hypotenuse of the square, with no colonization, compared with the biological value. Thus a perfectly smooth surface has a unity value and this fraction falls away with increasing roughness. The Ecorr data showed a direct relationship between the corrosion potential and the rugosity with the Ecorr moving to more anodic (corrosive) values as the rugosity fell. This sensitivity to colonization is best interpreted as being due to the increased turbulence created by the branching marine organism which results in a greater flux of dissolved oxygen to the surface and a common concomitant increase in the corrosion rate. It is interesting to note that the slope of the pH vs. depth for the Fujikawa Maru is 0.085 ± 0.001 pH/meter which is very similar the slope for the Ecorr vs. rugosity on the same wreck is −0.092 as shown in Figure 4. This data clearly supports the supposition that the pH is a direct measure of the corrosion rate and that when the Ecorr relates to separated corrosion cells on the wreck the impact of the increased surface roughness on the corrosion rates is the same regardless of whether the data comes from voltage or pH measurements. Although, the data is somewhat scattered the lines show that the port (sunny) side has a 6 mV more anodic potential and this is equivalent to a difference of a 4.5% increase in the corrosion rate. On all the wreck sites the rate at which the Ecorr moves to a more cathodic direction, less corrosive, varies but it is always the case that the reactivity of the wreck falls at a constant water depth as the roughness of the surface decreases.
Figure 4. Plot of the rate at which the Ecorr values move to more protective values as the roughness of the marine growth decreases with increasing depth.
Since the acidity of the underlying corroding metal interface is known to be dependent on the water depth, as shown above, and the rugosity (Ru) values increase with water depth, i.e., the surface roughness decreases with depth as the amount of light falling on the encrusting marine organisms falls, it was of interest to see if there is any relationship between the slope of the pH with water depth and the slope of the Ru values against the same variable. The results of the analyses are shown in Figure 5 which indicates that the wrecks have a very clear inter-relationship between the slope of the Ru vs. depth and the pH vs. depth data. The most disturbed wrecks, in terms of dynamite fishing damage form one group (the Fujikawa Maru, Susuki, and Shinkoku Maru) where the equation describing the response of the damage wrecks is given by,
For the dynamite damaged wrecks there is a lower square of the correlation coefficient, R2 = 0.6793, owing to the limited number of readings and wrecks that could be readily accessed. The second group covers those undamaged parts of the Susuki patrol boat, the Tonoas Dock Boat, the Unidentified Tonoas Boat and the so called Yamamoto gun boat. For these wrecks the relationship between the sensitivity of the plot of pH as a function of rugosity is given by,
In this equation the slope of the pH plot against rugosity is 2½ times that on the disturbed wrecks. The R2 for the undisturbed parts of the wrecks was 0.9969 which is an extraordinarily high correlation, particularly considering that the data is collected from the natural shipwreck environment. Since the slope of the pH vs. rugosity plot is linear with regard to rugosity the implications of this relationship are that the pH of the corroding iron shipwrecks is connected by the following relationship, namely
This quadratic expression is not unexpected since the slopes of the Ecorr and the pH with depth were both found to be quadratically dependent on the mean water depth.
Figure 5. Plot of the slope of the pH dependence on water depth and the dependence of rugosity on water depth which shows up the differences between sites that are undisturbed and those that have been subjected to “dynamite fishing.”
The wreck of the Gosei Maru, which lies in the lee of a nearby island, shows a corrosion rate that is significantly lower than one would predict on that basis of the depth alone. Owing to operational convenience most of the measurements were made on the sheltered port side of the vessel that lies on the edge of a natural underwater cliff since the former deck of the vessel had a wider range of elements to measure. The stern lies at a depth of 3 m while the bow is down at a depth of greater than 37 m. Dissolved oxygen measurements have confirmed that there is a change in the saturation of oxygen in the water column above the wreck which is consistent with the overall decay being lower for this slightly sheltered site, in terms of the flux of dissolved oxygen. The amount of water movement is significantly less than if the wreck was lying on its keel and it is further lowered by the aforementioned location in the lee of the island. The Gosei Maru is more sheltered from wave action and wind chop which normally increases water movement and the oxygen flux. Wrecks that are found in open waters in the lagoon, such as the Fujikawa Maru, have a turning point in the dissolved oxygen profile with depth that is very close to the bottom of the lagoon. In this way, it can be seen that the physical location of the wreck plays a significant part in the overall rate of deterioration of the vessel (MacLeod et al., 2007).
The colonization of substrates by marine organisms is dependent upon a balance between opportunity and toxicity of the substrate that presents itself to the floating larvae. It the surface is very smooth there is little chance of coral polyps to colonize the object. This is very readily seen in the wrecked aeroplanes of the lagoon when a combination of rivets and metal sheeting is of identical composition and there is minimal stress difference that could initiate localized pitting reactions. The corrosion mounds associated with pitting reactions dramatically increases the micro-roughness of the surface and thereby enhances colonization by marine organisms. There is little chance for a foothold for marine larvae on smooth aluminum alloys which is why there are sections of the nose cone and cockpit of the Emily flying bomber (a four engine armed marine reconnaissance aircraft of the Imperial Japanese Navy) is essentially devoid of colonization. However when there is a difference in composition of rivets and the alloys of the metal sheet subtle changes in corrosion can create precipitates around the surface of the riveted zones that enable a roughened substrate to form which is why some areas of the were colonized and others appear to be quite bare even after 60 years of immersion in warm tropical sea water (see Figure 6). When a series of data sets are being examined some systematic trends and responses become apparent, which would appear to be otherwise “invisible” on the basis of a few measurements of a random site visit.
Figure 6. Colonization on the Emily flying bomber with areas of no growth of marine organisms contrasting with other areas of significant growth after 62 years of immersion in seawater.
When the rate at which the pH falls with rugosity is plotted for each wreck it was found that the slopes become greater with increasing average water depth. When all the data from the different wrecks was plotted it showed that rather than being idiosyncratic there was a systematic trend. There is a linear relationship between the rate at which the pH vs. depth plots varied with mean water depth according to the equation below, viz.,
In this relationship dm is the mean depth of the wreck sites. This second order slope means that the rate at which the rugosity varies as a function of the pH is a quadratic rather than a linear relationship. The integrated value of the equation enables the real dependence of the rugosity on pH (and vice versa) to be determined and rather than being apparently linear the data is best expressed by the expression
which is similar to the quadratic dependence of the values of Ecorr with water depth that was found for the wrecks in Chuuk Lagoon (MacLeod, 2006).
This confirms the intuitively understood position that the rate of corrosion and marine concretion coverage falls with increasing water depth as the effect of wave action and wind chop decreases. In the case of the Susuki patrol boat the slopes of the rugosity versus the pH for both the port and starboard sides of the vessel was a 11.3 ± 0.4 while the difference in the intercepts, −1.9 pH for the port side and −2.7 pH for the sunny starboard side are related to the relative amount of light that each area receives. The greater the amount of light penetrating the sea water to the concreted metal surfaces the greater the amount of coral growth and the greater the localized turbulence and corrosion. This explains why when looking at the deck plates of a vessel lying in an exposed underwater aspect these elements are the first to be lost to corrosion. Apart from being initially thinner construction due to following naval architecture rules, the amount of light on the hatches causes increased colonization and ease of deposition on horizontal surfaces and thus more corrosion is experienced. With the increased turbulence these areas suffer from a locally increased corrosion rate they will collapse faster than vertical elements of identical original thickness and composition.
During the studies on the wreck of the Yubae Maru it was noted that this vessel has a greater corrosion rate than would be expected on the basis of the depth alone. The average depth of the site is 28 m and the corrosion rate was 0.095 mm/year which is relatively high for a wreck at that depth since it is very close to the long term marine corrosion rate for iron of 0.1 mm/year, which is generally found for wrecks at relatively shallow depths. One of the underlying reasons for the accelerated rate of decay is because the ship is lying upside down. Thus, there are many stresses in the vessel which are not those for which the Naval architects designed the ship. The bottom of the wreck site is littered with large plates of structural steel that had become detached from the frames that form the basis of the ship's structure (Figure 7). The calculations of the present corrosion rate indicate that the vessel is likely to undergo significant collapse in the next 5 years. This information is vitally important when one is considering the risk of oil escaping from within the wreck.
Figure 7. The upside down Yubae Maru wreck undergoing accelerated corrosion due to unrelieved stress in the structure.
When all the data available from corrosion profiles of all the depths of graphitization from across the lagoon at varying water depths is reviewed the data shows some systematic trends. When the wrecks are sorted into the shallower cohort of less than or equal to 20 m it is apparent that there is a logarithmic fall in corrosion rate with increasing depth. The following relationship holds for the impact of water depth on the corrosion rate over the first 20 m, viz.,
where d is the average water depth of the measurements and dg is the annualized depth of graphitization. This equation can be compared with similar data obtained from shipwrecks in the open ocean which has the statistically identical slope of −0.016, i.e., the open ocean sensitivity to water depth is within experimental error of the slope observed in Chuuk lagoon. The difference between the two corrosion equations is primarily in the intercept values, which simply indicate that the open ocean corrosion rate is higher by 100.102 or 26.5% higher than the waters of the sheltered lagoon. The equation for the open ocean wrecks is log dg = − 0.630 − 0.0156 d and this can be used to estimate the rate of decay of any unknown ship so long as the depth of the wreck is known.
In the deeper waters of the lagoon, fewer measurements were made owing to the diving limits imposed on the research programme by the volunteer funding organization, the Earthwatch Institute. The volunteer divers were only insured and protected to a depth of 20 m. Although, the data is limited mainly to that obtained in the initial survey in 2002 the overall corrosion rate seems to undergo a major change when the depth increases from 20 to 38 m since the logarithm of the corrosion rate falls by only 0.0052 per meter, which is 3.5 times less than in the shallower parts of the lagoon. The fundamental physical chemical reason behind this difference in apparent materials performance is that the amount of water movement and the amount of dissolved oxygen reaching the depths, significantly falls as the wind driven wave chop is limited in its penetration of the sheltered waters.
The practical implications of these two sets of corrosion equations, describing the rates of decay as a function of water depth for the wrecks in Chuuk lagoon, is that the bulk of the efforts in obtaining suitable site stabilization methods should be given to shallower shipwrecks, such as the Hoyo Maru which is already leaking oil and has an average depth of only 8.5 m, which makes it very vulnerable to normal high corrosion rates. In addition the Hoyo Maru is very susceptible to natural catastrophic events such as massive wave action associated with typhoons. It is possible to use the open ocean corrosion equation with a reasonable degree of confidence to predict the overall rate of decay of iron shipwrecks. Oil samples trapped inside the engine room of the Sankisan Maru at a depth of 28 m showed it to be a complex mixture of aviation fuel, diesel and bunker fuel oils (Chemistry Centre of Western Australia, 2016). The open ocean corrosion equation accurately predicted the corrosion rate of the Australian WWI submarine AE2 which lies at a depth of 72 m in the Sea of Marmara in Turkey. It is interesting to note that the American Civil War vessel the USS Monitor (1862) had the same corrosion rate as the AE2 and it was found at the same water depth (MacLeod, 2010).
Since there is a dearth of data pertaining to the thickness of the steel used in the construction of bunker fuel tanks the little data that can be gleaned from company records of the commercial and known naval vessels should be used as a conservative indicator of the chances of the site becoming a potential site for leaking fuel that was not consumed by fires prior to the initial sinking events. The Australian historic submarine from WWI which lies in 73 m of water in the Sea of Marmara in Turkey is now being actively treated with 10 tons of zinc sacrificial anodes. Each of three pods containing a set of inter connected anodes is attached through insulated cables to areas aft, amidships and forward sections of the wreck. Present measurements show that the vessel is undergoing cathodic protection and active removal of an estimated 50 tons of chloride ions. Although this project has consumed more than $240,000 for the combined maritime and conservation archaeological surveys using surface supplied tri-mix gases to the divers it is a small cost compared with the environment cost of cleaning up major oil spills and pales into comparison with the ecological cost of bio remediation of contaminated sites. The advantage of the shallow water wrecks in Chuuk lagoon and in other parts of the world's oceans is that these areas can be readily accessed without the need for expensive commercial diving processes associated with deep wrecks. Sacrificial anodes can be sourced at relatively cheap prices so all that is required is the political will to undertake this form of preventive environmental conservation.
A series of in-situ corrosion studies on historic WWII shipwrecks and sunken aircraft in Chuuk Lagoon, Federated States of Micronesia, have been conducted over the past 14 years to determine the importance of water depth, aspect of the wrecks, the accumulation and periodic shedding of protective marine concretions on the overall rate of deterioration of the vessels. The wrecked remnants of the Imperial Japanese naval and merchant ships and aeroplanes provide a major source of foreign exchange through the mechanism of diving tourism. The studies by the museum teams has shown that the rate of decay of iron is logarithmically dependent on water depth for the first 20 m and then the rate at which the corrosion rate falls becomes less dependent on depth owing to less flux of dissolved oxygen to the corroding interfaces. The in-situ pH measurements provide a direct measure of the localized corrosion rates which can be quantified through the relationship between pH and the accumulated corrosion profiles measured as the annualized depth of graphitization of cast iron. Corrosion potentials and pH data have demonstrated the biodynamic nature of the interaction of colonizing marine organisms and the localized corrosion rate. On shipwrecks with pronounced differences in the aspect of the port and starboard sides, it is possible to discern the impact of colonization by branching corals on the sunny side and clams on the darker side of the ship. The increased flux of seawater over the corals results from the more turbulent flow created by the colonizing organisms and results in an increase in the underlying corrosion rate of the iron wreck. It should be noted that this is a second order effect and that the primary protective role of marine concretion stands.
The data from the corrosion of wrecks in Chuuk Lagoon has quantified the way in which the growth rate of sedentary epifauna varies with the average depth of the wrecks. The surface roughness, as measured by the rugosity, shows that there is a quadratic dependence of rugosity and pH i.e., as the roughness increases the pH falls due to increased flux of dissolved oxygen associated with the less laminar flow of passing seawater. Vessels lying upside down on the seabed suffer from accelerated corrosion due to the impact of unresolved stress associated with their inverted aspect. It can be seen that corroding iron from historic shipwrecks provides a supportive microenvironment for colonizing calcareous organisms to create artificial reefs with many nooks and crannies which can act as shelter for small fish from predating species. The complex colonies of hard and soft corals, anemones, bryozoans, molluscs, and serpulids are inherently at risk from the increasing likelihood that corroding iron and steel bunker fuel tanks will collapse during a typhoon or other natural event. Given that the technology exists to prolong the life of steel structures underwater through the application of cathodic protection by use of either impressed current (for vessels close to an island) or sacrificial, it is incumbent on supporting nations to assist the Chuuk Government to implement such management schemes.
It would be advisable to harness the local ordnance recovery skills of the local divers to lead the safe disposal of many dozens of torpedoes and unexploded artillery shells. Hitherto such recovery operations have been for the purpose of creating “bombs” for “dynamite fishing” which not only accelerates corrosion of the wrecks and leads to premature collapse of superstructures. With effective corrosion management in place diving tourism will increase and the Chuuk teams will then be able to export their experience and skills to other jurisdictions and hemispheres.
The author confirms being the sole contributor of this work and approved it for publication.
Chalmers University of Technology. Invoice service. 41296 Gothenburg, Ref 48050, Ida-Maja Hassellov.
The author declares that the research was conducted in the absence of any commercial or financial relationships that could be construed as a potential conflict of interest.
I am deeply indebted to the assistance of my colleagues Bill Jeffery, Maria Beger, Vicki Richards, and the volunteers from the Earthwatch Institute and the staff of the Heritage Protection Office in Moen, Federated States of Micronesia who were invaluable in locating the wrecks and in being my diving buddies.
Carpenter, J., and MacLeod, I. D. (1993). “Conservation of corroded iron cannon and the influence of degradation on treatment times,” in ICOM-Committee for Conservation, Preprints 10th Triennial Conference, Vol. II (Washington), 759–766.
Chemistry Centre of Western Australia (2016). Report on Analysis of Oil Sample from Chuuk Lagoon Shipwreck, no 15S1588 Leif Cooper. Unpublished report, 25 January 2016.
Iverson, W. P., and Olson, G. J. (1983). “Anaerobic corrosion by sulphate reducing bacteria due to a highly reactive volatile phosphorus compound,” in Microbial Corrosion (Teddington: The National Physics Laboratory), 46–53.
MacLeod, I. D. (1988). “Conservation of corroded concreted iron,” in Proceedings of Conference 28, Australasian Corrosion Association, Vol. 1 (Perth, WA: Australasian Corrosion Association), 2–6:2–9.
MacLeod, I. D. (1995). In-situ corrosion studies on the Duart Point wreck 1994. Int. J. Naut. Archaeol. 24, 53–59.
MacLeod, I. D. (2005). “A new corrosion mechanism for iron shipwrecks in seawater: a study of the Fujikawa Maru (1944) in Chuuk Lagoon, Federated States of Micronesia,” in Preprints for ICOM-CC Triennial Meeting, Vol. II (Den Haag), 310–316.
MacLeod, I. D. (2006). Corrosion and conservation management of iron shipwrecks in Chuuk Lagoon. Conserv. Manag. Archaeol. Sites 7, 203–223. doi: 10.1179/135050306793137359
MacLeod, I. D. (2010). “Corrosion and conservation management of the HMAS AE2 (1915) submarine in the sea of marmara, turkey,” in METAL 2010, Proceedings of the Interim Meeting of the ICOM-CC Metal Working Group, eds P. Mardikian, C. Chemello, C. Watters, and P. Hull (Charleston, SC: Clemson University), 56–62.
MacLeod, I. D., Beger, M., Richards, V., Jeffery, B., and Hengeveld, M. (2007). “Dynamic interactions of marine ecosystems with wrecks in Chuuk Lagoon, Federated States of Micronesia,” in Metal 07, Preprints of the ICOM-CC Metals Working Group Meeting, Vol. 3. eds C. Degrigny, B. Ankersmit, I. Yolanda and R. van Langh (Amsterdam), 51–54.
MacLeod, I. D., and Richards, V. L. (2009). Corrosion monitoring in Chuuk Lagoon, quantitative analysis of the impact of “dynamite fishing” on historic iron shipwrecks. AICCM Bull. 32, 106–122. doi: 10.1179/bac.2011.32.1.015
North, N. A. (1976). The formation of coral concretions on marine iron. Int. J. Nautical Archaeol. Underw. Explorat. 5, 253–258.
Pourbaix, M. (1974). Atlas of Electrochemical Equilibria in Aqueous Solutions, 2nd Edn. Houston: NACE.
Keywords: shipwrecks, corrosion, WWII, Chuuk lagoon, pollution, Iron
Citation: MacLeod ID (2016) In-situ Corrosion Measurements of WWII Shipwrecks in Chuuk Lagoon, Quantification of Decay Mechanisms and Rates of Deterioration. Front. Mar. Sci. 3:38. doi: 10.3389/fmars.2016.00038
Received: 20 December 2015; Accepted: 11 March 2016;
Published: 30 March 2016.
Edited by:
Ida-Maja Hassellöv, Chalmers University of Technology, SwedenReviewed by:
Bernardo Duarte, Marine and Environmental Sciences Centre, PortugalCopyright © 2016 MacLeod. This is an open-access article distributed under the terms of the Creative Commons Attribution License (CC BY). The use, distribution or reproduction in other forums is permitted, provided the original author(s) or licensor are credited and that the original publication in this journal is cited, in accordance with accepted academic practice. No use, distribution or reproduction is permitted which does not comply with these terms.
*Correspondence: Ian D. MacLeod, ian.macleod@museum.wa.gov.au
Disclaimer: All claims expressed in this article are solely those of the authors and do not necessarily represent those of their affiliated organizations, or those of the publisher, the editors and the reviewers. Any product that may be evaluated in this article or claim that may be made by its manufacturer is not guaranteed or endorsed by the publisher.
Research integrity at Frontiers
Learn more about the work of our research integrity team to safeguard the quality of each article we publish.