- Department of Cell Morphology and Molecular Neurobiology, Faculty of Biology and Biotechnology, Ruhr-University Bochum, Bochum, Germany
Tenascins represent key constituents of the extracellular matrix (ECM) with major impact on central nervous system (CNS) development. In this regard, several studies indicate that they play a crucial role in axonal growth and guidance, synaptogenesis and boundary formation. These functions are not only important during development, but also for regeneration under several pathological conditions. Additionally, tenascin-C (Tnc) represents a key modulator of the immune system and inflammatory processes. In the present review article, we focus on the function of Tnc and tenascin-R (Tnr) in the diseased CNS, specifically after retinal and optic nerve damage and degeneration. We summarize the current view on both tenascins in diseases such as glaucoma, retinal ischemia, age-related macular degeneration (AMD) or diabetic retinopathy. In this context, we discuss their expression profile, possible functional relevance, remodeling of the interacting matrisome and tenascin receptors, especially under pathological conditions.
Introduction
Numerous studies demonstrate that retina and optic nerve degeneration is highly associated with remodeling of various extracellular matrix (ECM) components. Glycoproteins and proteoglycans that surround retinal cells and optic nerve fibers represent major constituents of the ECM meshwork, known as the matrisome (Reinhard et al., 2015; Naba et al., 2016; Vecino et al., 2016). Various components of the matrisome came into focus as “good cop, bad cop” in de- and regeneration processes after injury or damage of the optic nerve (Isenmann et al., 2003; Ahmed et al., 2005; Ren et al., 2015). Additionally, remodeling of matricellular proteins is evident in the trabecular pathway, for instance in glaucoma pathogenesis (Wallace et al., 2015). In this review article, we focus on tenascin glycoproteins, which raised considerable attention in the context of degenerative processes in the retina and optic nerve.
The Tenascin Family
In vertebrates, the family of tenascins comprises the four members tenascin-C, -R, -W and -X (Chiquet-Ehrismann and Tucker, 2011; Chiquet-Ehrismann et al., 2014). Expression of tenascin-R (Tnr) is restricted to the nervous system, whereas tenascin-C (Tnc) can also be found in non-nervous tissue. Due to the fact that little, if anything, has been reported about the role of tenascin-W and -X in the diseased visual system, this review article mainly focuses on the expression and functional importance of Tnc and Tnr in retinal and optic nerve degeneration and various eye diseases.
Both tenascin molecules exhibit a modular structure (Nies et al., 1991; Siri et al., 1991; Jones F. S. and Jones, 2000; Jones P. L. and Jones, 2000; Joester and Faissner, 2001; Midwood and Orend, 2009). Tnc is an oligomeric protein, which consists of six monomers that are connected via a tenascin assembly (TA) domain at the amino-terminal region (Figure 1A). This constitution is also called hexabrachion. In human, each TNC monomer consists of a TA domain, followed by a cysteine-rich domain, 14.5 epidermal-growth factor (EGF)-like domains, eight fibronectin (FN)-type III domains and a fibrinogen (FG)-like carboxy-terminal part. Additional FN-type III domains, termed A1, A2, A3, A4, B, AD2, AD1, C and D, can be inserted between domain 5 and 6 (Spring et al., 1989; Nies et al., 1991; Dörries and Schachner, 1994; Joester and Faissner, 1999, 2001; Tucker et al., 2006). Via alternative TNC mRNA splicing, and based on a binary combinatorial potential, the generation of up to 512 isoforms in humans is feasible (Joester and Faissner, 1999, 2001; Theocharidis et al., 2014; Midwood et al., 2016; Faissner et al., 2017). One interesting feature of Tnc is that it exhibits both adhesive and anti-adhesive properties (Faissner and Kruse, 1990; Chiquet-Ehrismann et al., 1991; Faissner, 1997). For instance, the FN-type III region exhibits pro-adhesive characteristics, whereas the EGF-like domains show anti-adhesive properties (Spring et al., 1989; Ajemian et al., 1994; Gotz et al., 1996). The latter are also associated with proliferation, growth cone repulsion and migration (Joester and Faissner, 2001; Swindle et al., 2001; Loers and Schachner, 2007). Human TNR exhibits a similar modular structure, but it is composed of three monomers (Figure 1B; Schachner et al., 1994). Each monomer consists of an amino-terminal TA domain, a cysteine-rich domain, 4.5 EGF-like repeats, eight or nine FN-type III domains and a FG carboxy-terminal part.
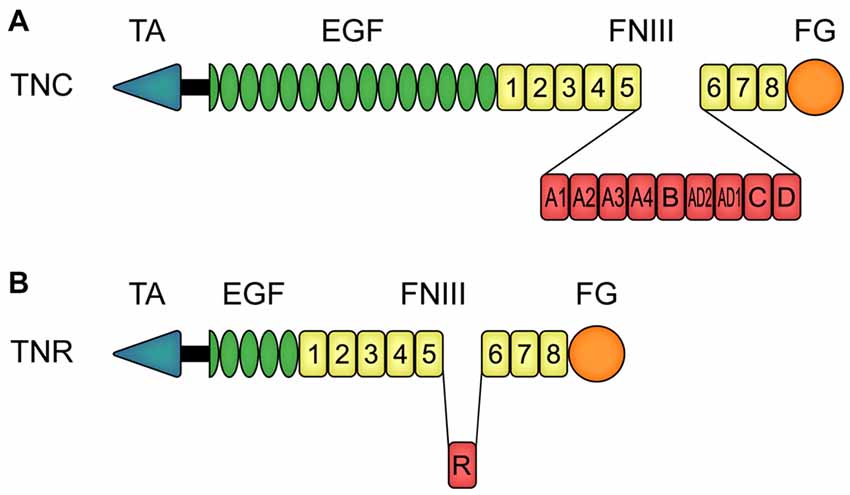
Figure 1. Modular assembly of human tenascin-C (TNC) and tenascin-R (TNR) monomers. (A) In human, each TNC monomer consists of an amino-terminal tenascin assembly (TA) domain, a cysteine-rich domain, 14.5 epidermal-growth factor (EGF)-like domains, eight constitutive fibronectin (FN)-type III homologous domains and a fibrinogen (FG)-like carboxy-terminal part. Between the FN-type III domains 5 and 6, TNC can carry the additional FN-type III domains A1, A2, A3, A4, B, AD2, AD1, C and D due to alternative splicing. (B) Human TNR also consists of a TA domain and a cysteine-rich domain, in this case followed by 4.5 EGF-like domains, eight constitutive FN-type III domains and a FG-like carboxy-terminal part. The alternatively spliced FN-type III domain R can be inserted between the FN-type III domains 5 and 6. Abbreviations: EGF, epidermal-growth factor-like domain; FG, fibrinogen-like domain; FNIII, fibronectin-type III homologous domain; TA, amino-terminal tenascin assembly domain; TNC, human tenascin-C; TNR, human tenascin-R.
Tenascins in the Developing and Adult Healthy Retina and Optic Nerve
As part of the eye (Figure 2A), the retina and the optic nerve develop from neuroectodermal tissue. During retinogenesis, seven main cell types, namely retinal ganglion cells (RGCs), amacrine, bipolar, horizontal, Müller glia as well as cone and rod photoreceptor cells, arise from multipotent retinal progenitor cells in highly conserved and overlapping waves (Cepko et al., 1996; Dyer and Cepko, 2001; Marquardt, 2003; Agathocleous and Harris, 2009; Heavner and Pevny, 2012). Until adulthood, following a maturation and synaptic fine-tuning period, retinal cell nuclei are assigned to specific nuclear layers, while their synaptic processes are arranged in plexiform and nerve fiber layers (Figure 2B).
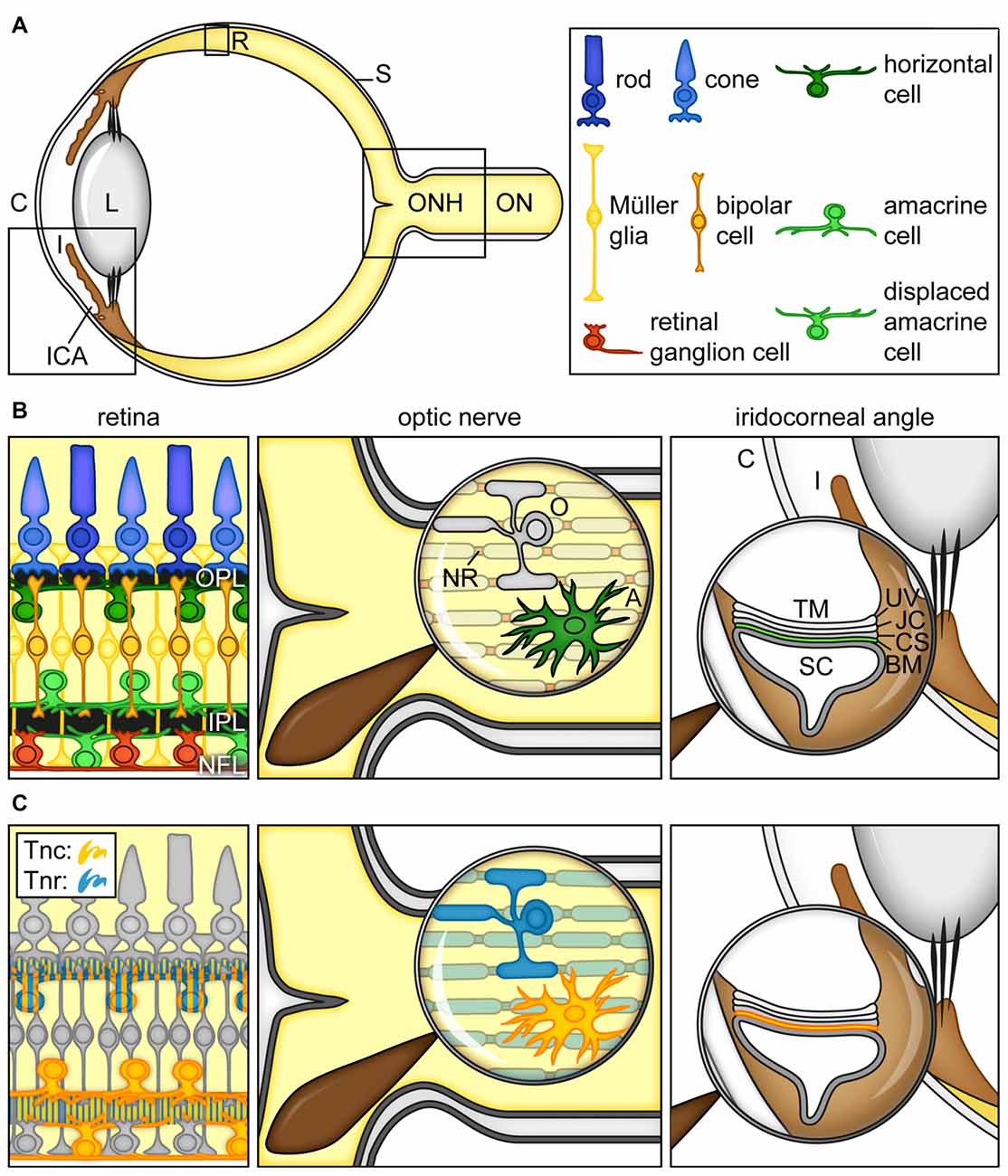
Figure 2. Cartoon summarizing the current view on the expression of tenascins in the retina, optic nerve and iridocorneal angle. (A) Scheme of the adult human eye. (B) Scheme of the retina, optic nerve and iridocorneal angle. (C) Visualization of Tnc- and Tnr-expressing cell types/structures in the retina, optic nerve and iridocorneal angel. In the retina, horizontal, amacrine and displaced amacrine cells are a main source of Tnc expression (orange). Horizontal cells also co-express large amounts of Tnr (blue). Additionally, signals of both proteins can be observed in the plexiform layers (orange/blue). In the optic nerve, astrocytes show a strong Tnc expression (orange). Tnr (blue) is highly expressed by optic nerve oligodendrocytes and localized at myelinated fibers and nodes of Ranvier. In the iridocorneal angle, the basement membrane underlying the inner wall of Schlemm’s canal contains Tnc protein (orange). Abbreviations: A, astrocyte; BM, basement membrane; C, cornea; CS, corneoscleral tissue; I, iris; ICA, iridocorneal angle; IPL, inner plexiform layer; JC, juxtacanalicular tissue; L, lens; NFL, nerve fiber layer; NR, node of Ranvier; O, oligodendrocyte; ON, optic nerve; ONH, optic nerve head; OPL, outer plexiform layer; R, retina; S, sclera; SC, Schlemm’s canal; TM, trabecular meshwork; Tnc, tenascin-C; Tnr, tenascin-R, UV, uveal tissue.
Indeed, the retina is an excellent model system to study developmental aspects such as proliferation and differentiation, but also axonal growth and guidance as well as pathfinding (McLaughlin et al., 2003; Oster et al., 2004). Nasal and temporal projections from the retina are transmitted via RGC axons, which form the optic nerve. RGC axons from both eyes converge in the optic chiasm at the base of the hypothalamus and segregate into ipsi- and contralaterally projecting fibers (Petros et al., 2008; Erskine and Herrera, 2014). Via the optic tract, axons project in a highly topographic manner into subcortical and cortical areas to transfer the visual information.
Intrinsic and extrinsic factors, which comprises transcription factors, growth factors and a variety of ECM components, including tenascins, influence retinogenesis and the growth of optic nerve fibers (Thanos and Mey, 2001; Hatakeyama and Kageyama, 2004; Harada et al., 2007; Agathocleous and Harris, 2009; Xiang, 2013; Reinhard et al., 2015).
In the developing retina, Tnc becomes detectable within the inner neuroblastic layer at embryonic day 13 (Klausmeyer et al., 2007). In the adult retina, it is synthesized by different neuronal subtypes, including horizontal, amacrine and displaced amacrine cells and is prominently enriched in the outer and inner plexiform as well as in the nerve fiber layer (D’Alessandri et al., 1995; Sánchez-López et al., 2004; Figure 2C). As shown by Siddiqui et al. (2009), cultivated postnatal Müller glia cells also express large Tnc isoforms. In the optic nerve, astrocytes secrete huge amounts of the Tnc protein (Bartsch et al., 1995; D’Alessandri et al., 1995; Garwood et al., 2004; Reinhard et al., 2015).
Tnr, also known as janusin/J1-160/180 in rodents or restrictin in the chicken, is initially expressed upon postnatal stages in the developing retina and optic nerve (Ffrench-Constant et al., 1988; Bartsch et al., 1993; Wintergerst et al., 1993; Joester and Faissner, 2001). Later, Tnr expression peaks until the third postnatal week and then decreases again. In the adult retina, horizontal cells are the main cellular source of Tnr (Figure 2C). Due to the proximity of Tnr-expressing cells, large amounts of protein are found in the outer plexiform layer. Nevertheless, the inner plexiform and nerve fiber layer also show detectable levels of the Tnr protein, suggesting low expression by other retinal cell types or intraretinal protein transport. In the optic nerve, it is highly expressed by oligodendrocytes and associated with myelinated fibers and nodes of Ranvier with ongoing age until adulthood. In contrast, Tnr is absent from the unmyelinated proximal, retina-near part of the optic nerve.
Role of Tenascins in Eye Diseases
In the central nervous system (CNS), Tnc exhibits high expression during early development. With ongoing maturation, it is progressively downregulated, but re-expressed under pathological conditions (Garwood et al., 2004; Roll et al., 2012; Reinhard et al., 2015). The role of Tnc remodeling in the neural stem/progenitor compartment has been reviewed comprehensively (Roll and Faissner, 2014; Theocharidis et al., 2014; Faissner and Reinhard, 2015; Faissner et al., 2017). It regulates proliferation and differentiation and is also enriched in the adult neural stem cell niche. Additionally, Tnc is involved in barrier formation, for example in the barrel cortex during development and as a constituent of the glial scar after injury. Also in many cancers, Tnc is highly expressed and promotes migration as well as angiogenesis (Orend and Chiquet-Ehrismann, 2006; Midwood and Orend, 2009; Brösicke and Faissner, 2015; Reinhard et al., 2016). These examples show the huge spectrum of—in part ambivalent—Tnc-mediated functions. Furthermore, various studies suggest that tenascin glycoproteins might be involved in degenerative processes of the retina and optic nerve as well as eye diseases e.g., glaucoma.
Tenascin-C in Glaucoma
Glaucoma is one of the leading causes of visual impairment and irreversible blindness worldwide. It is a neurodegenerative disease characterized by morphological changes of the optic nerve head and retinal nerve fiber layer as well as progressive RGCs loss (EGS, 2017). In 2010, approximately 4.2 million people were visually impaired due to glaucoma (Bourne et al., 2016). This number will likely rise to about 11.2 million people by 2020 (Quigley and Broman, 2006). Among others, age, genetic predisposition and intraocular pressure (IOP) elevation are considered the most important risk factors for glaucoma. However, its pathophysiology is still poorly understood.
Various studies indicate that remodeling of Tnc is strongly associated with high-pressure glaucoma (Table 1). Pena et al. (1999) recognized that enhanced Tnc expression is associated with reactive astrocytes in the human optic nerve head of primary open-angle glaucoma (POAG) patients. Although the precise function of Tnc in glaucoma disease is still unknown, it was assumed that it might act as barrier molecule, which locally restricts detrimental humoral and blood-derived factors, to protect RGC axons. Along these lines, Johnson et al. (2007) described a prominent Tnc upregulation in the pressure-injured optic nerve head of a rat ocular hypertension glaucoma model. Additionally, Tnc might be involved in reactivation of astrocytes, which play a crucial role in glaucomatous optic nerve fibrosis (Schneider and Fuchshofer, 2016).
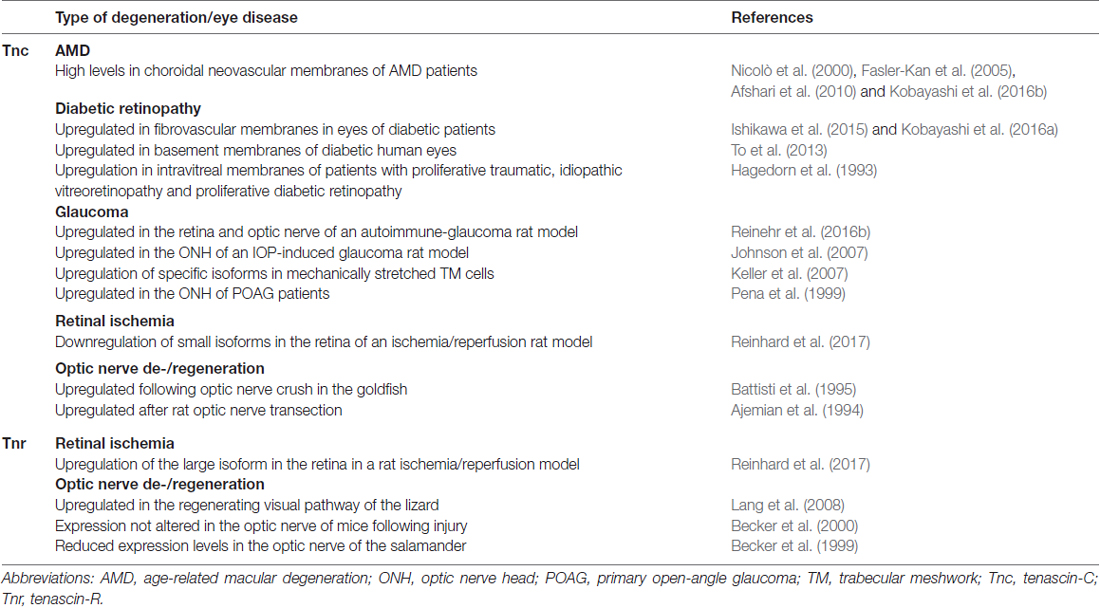
Table 1. Summary of the current knowledge on the regulation of tenascin-C (Tnc) and tenascin-R (Tnr) expression in retinal and optic nerve degeneration and eye diseases.
IOP rises due to impaired aqueous humor outflow via the trabecular pathway in the iridocorneal angle (Abu-Hassan et al., 2014; Dautriche et al., 2014). In this regard, it is interesting to note that Tnc is an extracellular component within the human juxta-canalicular tissue (JCT). In addition, Tnc was detected in trabecular meshwork (TM) cells (Ueda and Yue, 2003; Pattabiraman and Rao, 2010; Keller et al., 2013; Figure 2B). In the JCT, Tnc is predominantly localized in basement membranes underlying the inner wall of Schlemm’s canal (Figure 2C).
An abnormal accumulation of ECM constituents increases aqueous humor outflow resistance through the trabecular pathway (Gabelt and Kaufman, 2005). The functional importance of matricellular protein production and turnover to control outflow resistance in the TM has been reviewed (Wallace et al., 2014, 2015; Tamm et al., 2015). Several matricellular proteins, including the connective tissue growth factor, thrombospondin, Tnc and Tnx, appear to play a role in TM fibrosis. For instance, deficiency of the matrix glycoproteins thrombospondin 1 and SPARC (secreted protein acidic and rich in cysteine) has been shown to enhance outflow facility and lower IOP in mouse models of glaucoma (Wallace et al., 2015).
Keller et al. (2007) noted increased levels of Tnc in response to mechanical stretching of porcine TM cells in a perfusion culture model. Isoforms of Tnc identified in those TM cells included FN-type III 5-D-6, 5-6, A1-B as well as B-D-6. Interestingly, levels of Tnc FN-type III domain D transcripts were also elevated due to mechanical stretching of TM cells, indicating changes in alternative splicing that might affect TM cell-ECM interaction.
The effects of Tnc knockdown on TM outflow resistance were studied in more detail in anterior segment perfusion organ cultures (Keller et al., 2013). Here, Tnc was upregulated in response to IOP elevation. Nevertheless, the outflow rate was not altered by Tnc-silencing in anterior segments following IOP elevation. In addition, IOP was not altered in Tnc knock-out compared to control mice, indicating that Tnc does not directly contribute to the regulation of outflow resistance. However, Yang et al. (2016) described the effects of induction and inhibition of matrix cross-linking on remodeling of the aqueous humor outflow resistance by TM cells. In this study, genipin, a potent inducer of ECM crosslinking and inhibitor of aqueous humor outflow, reduced the levels of Tnc and other ECM components such as collagen I, elastin and the chondroitin sulfate proteoglycan (CSPG) versican. These findings indicate that changes in the ECM composition, crosslinking and turnover are highly dynamic and influence outflow resistance. Recently, the group around Kuehn showed a positive effect on outflow facility after transplantation of induced pluripotent stem cell-derived TM cells into a glaucoma model. Since the transplanted cells do not persist for long, it was theorized that the cells might alter TM conditions (Zhu et al., 2016, 2017). These alterations could also be related to changes in the ECM. The TM serves as an important target for the treatment of IOP elevation in glaucoma (Ferrer, 2006). In a future perspective, further knowledge of ECM-TM physiology is necessary to develop novel and powerful IOP lowering therapies.
Tenascins are also key regulators of the immune system and neuroinflammatory processes (Jakovcevski et al., 2013). A complex interplay and functional relationship between neural and immune cells in various autoimmune diseases, e.g., multiple sclerosis and neuropathies, are evident. Tnc was reported as one major ECM component, which modulates transforming growth factor β (TGFβ)/Smad signaling and myofibroblast generation during wound healing of the corneal stroma (Saika et al., 2016). Also in the TM, TGFβ increases outflow resistance via alteration of ECM homeostasis and cell contractility (Fuchshofer and Tamm, 2012; Prendes et al., 2013; Wang et al., 2017). In addition, Tnc was previously reported to influence the immune system through the toll-like receptor 4 (TLR4; Midwood et al., 2009). It promotes an inflammatory response via macrophage generation, activation of TLR4 and the secretion of proinflammatory cytokines after stimulation with lipopolysaccharide (Piccinini and Midwood, 2012; Piccinini et al., 2016). Indeed, Tnc deficiency protects mice from experimental autoimmune encephalomyelitis and plays a key role in pathogenesis of CNS autoimmunity (Momcilovic et al., 2017). Interestingly, various studies have shown that TLR4 gene polymorphisms are associated with an increased risk of glaucoma (Shibuya et al., 2008; Navarro-Partida et al., 2017a,b). This confirms that TLR4-mediated signaling is involved in this disease.
Several studies have also investigated the possible involvement of the immune system in glaucoma pathogenesis (Tezel and Wax, 2004; Tezel, 2009; Rieck, 2013; Ramirez et al., 2017). Recently, we have noticed Tnc dysregulation in an IOP-independent, experimental autoimmune glaucoma model (Reinehr et al., 2016b). In this glaucoma model, RGC loss, optic nerve damage, reactive gliosis as well as complement activation have been described (Joachim et al., 2013, 2014; Casola et al., 2015; Noristani et al., 2016; Reinehr et al., 2016a). Furthermore, upregulation of Tnc and the CSPG phosphacan, an interaction partner of Tnc, was found in the retina and optic nerve of the autoimmune glaucoma model (Reinehr et al., 2016b). Most interestingly, elevated Tnc levels were observed before RGC loss occurred in this model. Regarding this finding, Tnc might act as an early indicator of glaucomatous neurodegeneration, although the function of Tnc in IOP-independent glaucoma is not well understood yet.
Tenascin-C and Tenascin-R in Retinal Ischemia
Ischemia represents a common pathomechanism in several retinal diseases, like age-related macular degeneration (AMD), diabetic retinopathy, glaucoma and retinal vascular occlusion (Mizener et al., 1997; Coleman et al., 2013; Sim et al., 2013).
Several studies reported on a dysregulation of Tnc following cerebral, hepatic as well as myocardial ischemia (Lu et al., 2003; Taki et al., 2010, 2015; Kuriyama et al., 2011). We recently analyzed the regulation of ECM glycoproteins and proteoglycans in the retina and optic nerve of an ischemia/reperfusion rat model (Reinhard et al., 2017; Table 1). An interesting finding of this study includes the prominent upregulation of several CSPGs in the ischemic optic nerves. Furthermore, in the retina, elevated levels of the large Tnr isoform were found, while reduced levels of smaller Tnc isoforms were observed after ischemia/reperfusion. These findings support the idea of an isoform-dependent regulation of tenascins. In future studies, domain-specific Tnc antibodies (Brösicke et al., 2013; Reinhard et al., 2016) should be used to relate specific isoforms to distinct retinal cell types under pathological conditions.
In the CNS, tenascins represent main structural and functional constituents of synaptic sites (Dityatev et al., 2010; Kwok et al., 2011; Heikkinen et al., 2014; Dzyubenko et al., 2016; Song and Dityatev, 2017). Also in the retina, tenascins are highly associated with synaptic layers (Bartsch et al., 1993; D’Alessandri et al., 1995; Wahlin et al., 2008). We have previously shown a co-localization of Tnc and synaptophysin in the healthy retina (Reinhard et al., 2015). In sum, the dysregulation of tenascins after retinal ischemia might reflect the response or damage of retinal neurons or synaptic reorganization.
Tenascin-X and Tenascin-C in Age-Related Macular Degeneration
AMD is defined by a deterioration of the macula and represents a major cause of vision impairment worldwide (Jager et al., 2008; Ding et al., 2009; Lim et al., 2012). It is a multifactorial disease that affects primarily photoreceptor cells, retinal pigment epithelium (RPE), Bruch’s membrane as well as choriocapillaries (Bhutto and Lutty, 2012). Additionally, AMD is characterized by extracellular depositions between Bruch’s membrane and the RPE, termed drusen, which includes complement components, glycoproteins and lipids (Crabb, 2014; Fernandez-Godino et al., 2016). Choroidal neovascularization is the defining characteristic of wet AMD.
The tenascin family member Tnx was identified in AMD patients in a genome-wide association study (Cipriani et al., 2012). In a plasma protein screen to identify biomarker, Tnx was differentially expressed in AMD patients compared to the healthy controls (Kim et al., 2014).
Additionally, high levels of Tnc were observed in choroidal neovascular membranes from AMD patients (Nicolò et al., 2000; Fasler-Kan et al., 2005; Afshari et al., 2010; Kobayashi et al., 2016b; Table 1). Here, RPE cells restricted to scar areas exhibited a strong staining for Tnc. Tnc was also identified as a candidate to cause RPE adhesion failure in damaged and aged Bruch’s membrane. In this regard, Afshari et al. (2010) described that Tnc inhibits RPE attachment and migration. Interestingly, this inhibition can be overcome via integrin activation or expression of Tnc-binding integrin α9, which allows RPE cells to interact with the AMD-affected Bruch’s membrane (Afshari et al., 2010). Additionally, Tnc secretion by transdifferentiated RPE cells is considered to promote choroidal neovascular membrane formation via integrin αv in a paracrine manner (Kobayashi et al., 2016b). Here, Tnc was discussed as potential target for the inhibition of choroidal neovascular membrane formation in AMD.
Tenascin-C in Diabetic Retinopathy
Diabetic retinopathy is also highly associated with retinal vascular dysfunction. Tnc was found in intravitreal membranes of patients with traumatic and idiopathic proliferative vitreoretinopathy as well as in diabetic retinopathy (Hagedorn et al., 1993; Table 1). In light of these results, it was suggested that Tnc likely controls cellular adhesion and ECM formation under pathological conditions. Structural, morphological as well as biophysical changes of ocular vasculature basement membranes were reported to be accompanied by ECM remodeling (To et al., 2013). Here, a higher Tnc expression was detected in basement membranes of diabetic compared to non-diabetic human eyes. Additionally, Tnc was reported to be involved in inflammatory processes of diabetic retinopathy. Increased Tnc levels were found in retinal endothelia cells following tumor necrosis factor α and interleukin 1β stimulation (Palenski et al., 2013). Expression analysis in fibrovascular membranes from patients with proliferative diabetic retinopathy revealed an upregulation of Tnc (Ishikawa et al., 2015). Recently, Kobayashi et al. (2016a) showed that Tnc, secreted from vascular smooth muscle cells, promotes angiogenesis in fibrovascular membranes associated with diabetic retinopathy.
Role of Tenascin-C and Tenascin-R in Optic Nerve Injury, Degeneration and Regeneration
RGC nerve fibers exhibit a poor regeneration capacity after injury, which often leads to irreversible vision loss. Therefore, multiple studies focused on the improvement of RGC survival as well as axonal regrowth, guidance and pathfinding (Fischer and Leibinger, 2012; Crair and Mason, 2016). Indeed, the optic nerve serves as an ideal research model to follow axonal de- and regeneration processes and RGC survival in order to develop novel therapeutic strategies, for instance after glaucomatous damage (Diekmann and Fischer, 2013; Gauthier and Liu, 2016; Calkins et al., 2017; Tamm and Ethier, 2017). Over the past decades, it has become evident that regeneration capacity differs a lot with age and between various species. Regeneration is more efficient in lower compared to higher vertebrates.
After optic nerve damage, Wallerian degeneration, demyelination, immune activation and glial scar formation can be observed. In this context, it has become evident that next to the intrinsic cellular repertoire, an inhibitory environment prevents regrowth of optic nerve fibers (Fischer, 2012). ECM proteins are main components of this inhibitory environment. Here, tenascins were described as crucial boundary formation molecules in optic nerve degeneration. Those boundaries represent important decision breakpoints to navigate growing axons during development as well as following injury or disease (Silver, 1994). In the adult mammalian CNS, after injury, Tnc and Tnr play opposing roles in regeneration of optic nerve fibers, with Tnc being promotive and chemo-attractive, while Tnr plays an inhibitory and chemo-repulsive role (Jakovcevski et al., 2013). The current knowledge on the regulation of Tnc and Tnr following optic nerve degeneration and regeneration is also summarized in Table 1.
Compared to mammals, the CNS of the zebrafish displays a robust axonal regeneration capacity and allows visualization of axonal regeneration and re-myelination in vivo. Tnr was also described as a repulsive guidance molecule of newly growing as well as regenerating optic nerve fibers in the zebrafish (Becker and Becker, 2002; Becker et al., 2004). Becker et al. (2000) reported that Tnr inhibits regrowth of optic nerve fibers in vitro. In contrast to the reduced Tnr expression levels observed in the optic nerve of the salamander (Becker et al., 1999), it persists in the optic nerve of mice following injury (Becker et al., 2000). Due to the continued expression, it was suggested that Tnr inhibits axonal regeneration in vivo. In addition, Tnr and axon growth-promoting molecules were found upregulated in the regenerating visual pathway of the lizard Gallotia galloti (Lang et al., 2008).
Since Tnr is highly associated with myelinated optic nerve fibers and nodes of Ranvier, it was proposed that it might have a functional relevance in myelination processes. Recordings of action potentials from Tnr knock-out mice revealed reduced axonal conduction velocities compared to control mice. In contrast, no significant differences in the number of myelinated optic nerve fibers or in the myelin ultrastructure were observed in Tnr knock-out compared to wild-type mice (Weber et al., 1999).
A potential role of Tnc in neural repair of the injured rat optic nerve was initially reported by Ajemian et al. (1994). Here, after optic nerve transection, Tnc immunoreactivity appeared in astrocytes at the border of the injury. Additionally, it was proposed to act as important barrier molecule for oligodendrocyte precursor migration during development (Bartsch et al., 1994; Kiernan et al., 1999). Following crush injury of the goldfish optic nerve, Tnc was reported to be associated with activated granular macrophages, although its expression in activated astrocytes and microglia was also assumed (Battisti et al., 1995). In contrast, although Bernhardt et al. (1996) described lesion-induced upregulation of several glia cell-associated genes after axotomy in the adult zebrafish, Tnc levels were not altered. In the embryonic and postnatal rat retina Tnc promotes axonal outgrowth, especially via the alternatively spliced FN-type III D domain (Siddiqui et al., 2008).
Tenascin Signaling and the Interacting Matrisome Under Pathological Conditions
A huge diversity of interacting molecules can be observed for the tenascin proteins. For Tnc this includes the cell adhesion molecules contactin-1 (Rigato et al., 2002; Czopka et al., 2010), various CSPGs of the lectican family such as aggrecan and neurocan, phosphacan/receptor protein tyrosine phosphatase β/ζ (RPTPβ/ζ; Barnea et al., 1994; Milev et al., 1997; Rauch et al., 1997; Garwood et al., 1999; Adamsky et al., 2001; Lundell et al., 2004) but also several integrin family members like α2β1, α7β1, α8β1, α9β1 and αvβ3 (Tucker and Chiquet-Ehrismann, 2015; Faissner et al., 2017).
The signaling of integrins in RGC-glia interactions is crucial for RGC survival and process extension (Vecino et al., 2016). In the developing retina, β1 integrins mediate RGC neurite outgrowth and α integrin-subunits are expressed in RGCs. Tnc inhibits axonal growth, but also displays axon growth-promoting properties, when appropriate receptors like α9β1 integrin are expressed. The FN-type III domain Nr. 3 of Tnc is a ligand of α9β1 integrin (Yokosaki et al., 1998). However, in the adult, neurite outgrowth-promoting α9β1 integrin is absent in neurons, which counteracts regeneration properties (Wang et al., 1995). Interestingly, in the spinal cord, regeneration of sensory axons can be achieved by expression of Tnc-binding α9 integrin and kindlin-1 (Andrews et al., 2009; Cheah et al., 2016). As recently reported, co-transduction of α9 integrin and the integrin activator kindlin-1 represents a promising approach to promote optic nerve regeneration (Fawcett, 2017). Integrin-Tnc signaling might also play a role in the glaucomatous optic nerve head, as Morrison reported on the crucial importance of integrins in optic neuropathy (Morrison, 2006).
As mentioned above, CSPGs are major interaction partners of Tnc. In the CNS, CSPGs are widely recognized as major inhibitory constituents of the glial scar (Silver and Silver, 2014). Notably, elevated levels of the CSPGs aggrecan, brevican and phosphacan were noted in the optic nerve after retinal ischemia (Reinhard et al., 2017). Following laser lesion, differential expression of RPTPβ/ζ was observed in the retina (Besser et al., 2009). In the non-injured retina of Tnc deficient mice, an upregulation of the DSD-1 epitope, recognized by the monoclonal antibody 473HD and localized on phosphacan/RPTPβ/ζ, was revealed when compared to the wild-type mice (Besser et al., 2012). Since RPTPβ/ζ knock-out mice show a disturbance of Müller glia processes, RPTPβ/ζ might be implicated in the assembly of the retinal structure (Horvat-Bröcker et al., 2008). There is also strong evidence that Tnc interacts with a variety of growth factors. In this context, it has been shown that the FN-type III domain 5 of Tnc displays a high binding affinity for the fibroblast growth factor 2 (FGF2), neurotrophin-3, platelet-derived growth factor-BB as well as TGFβ1 (De Laporte et al., 2013). After brain lesion, TGFβ and FGF2 control Tnc expression in astrocytes and reactive cells (Smith and Hale, 1997; Dobbertin et al., 2010). Therefore, Tnc might contribute to the signaling environment after lesion. Nevertheless, the direct functional contribution of Tnc has not been elucidated yet. Tnc was found to enhance FGF2 sensitivity of de-differentiating Müller glia cells in vitro. Furthermore, Tnc knock-out mice show an impaired de-differentiation capacity (Besser et al., 2012).
Likewise, Tnc displays a complex interactome with other ECM glycoproteins. For instance, its interaction with fibronectin and Tnr was reported (Chiquet-Ehrismann et al., 1991; Chung et al., 1995; Probstmeier et al., 2000; Giuffrida et al., 2004). After CNS damage, glial-released fibronectin exhibits neuroprotective repair function and promotes outgrowth of neurites in vitro (Tom et al., 2004; Tate et al., 2007; Kim et al., 2013). Also the neural transmembrane protein CALEB (chicken acidic leucine-rich EGF-like domain-containing brain protein/neuroglycan C) directly interacts with Tnc and Tnr (Schumacher et al., 2001; Schumacher and Stübe, 2003). Interestingly, CALEB is highly expressed following optic nerve lesion (Schumacher et al., 2001; Schumacher and Stübe, 2003). CALEB expression is strongly associated with developing as well as regenerating RGCs.
The adhesion molecule contactin-1 was identified as an important neuronal receptor for Tnr. Interaction of these two molecules was reported to mediate the repulsion and defasciculation of neurites (Pesheva et al., 1993; Milev et al., 1998; Xiao et al., 1998). Additionally, as shown for Tnc, Tnr displays an overlapping expression pattern with the CSPG phosphacan (Xiao et al., 1997; Milev et al., 1998). Regarding these findings, Tnc and Tnr seem to represent key components of the retina and optic nerve matrisome under pathological conditions. Both molecules partially display an overlapping extracellular distribution and directly contribute to de- and regeneration processes.
Conclusion
In the CNS, tenascin glycoproteins are important constituents of a highly regulated and dynamic matrisome. In sum, the current literature supports the notion that Tnc and Tnr are implicated in various pathological processes following retinal and optic nerve degeneration as well as various eye diseases (Table 1). Under pathological conditions, during development as well as regeneration, the opposed character of Tnc and Tnr is crucial for the growth and guidance of axons. In particular, the manipulation of Tnc-integrin signaling might be a promising approach to enhance axonal regeneration. Additionally, as a structural ECM component of the TM, Tnc and the interacting matrisome might be a target to improve IOP lowering therapies. We provide evidence that TLR4 signaling is involved in glaucoma development. Tnc upregulation observed under these conditions might indicate an immunomodulatory role, mediated by TLR4. Since tenascins are highly enriched at synaptic sites in the retina, it is plausible to speculate that they might play a role in synaptic remodeling, also under pathological conditions. Nevertheless, to verify these potential functions, further analyses have to be performed.
Author Contributions
JR wrote the manuscript. LR designed the figures. LR and AF revised the manuscript. All authors have approved the final article.
Conflict of Interest Statement
The authors declare that the research was conducted in the absence of any commercial or financial relationships that could be construed as a potential conflict of interest.
Acknowledgments
We thank Dr. Stephanie C. Joachim for her helpful and critical comments on our manuscript. We gratefully acknowledge the Stem Cell Network North Rhine-Westphalia, the German Research Foundation (Deutsche Forschungsgemeinschaft, DFG: SFB 509, SFB 642, SPP-1109, SPP-1172, Fa 159/11-1, 2, 3, Fa 159/16-1, GRK 736, GSC 98/1, SPP-1757, Fa 159/20-1, Fa 159/22-1), the Mercur-Foundation (Mercator Research Center Ruhr: Pr 2011-0010), the German Ministry of Education, Research and Technology (Bundesministerium für Bildung und Forschung, BMBF 01GN0503) and the Ruhr-University (International Graduate School of Neuroscience and President’s special programme call 2008) for grant support. We acknowledge support by the DFG Open Access Publication Funds of the Ruhr-Universität Bochum.
Abbreviations
AMD, age-related macular degeneration; CNS, central nervous system; CSPG, chondroitin sulfate proteoglycan; ECM, extracellular matrix; FGF2, fibroblast growth factor 2; IOP, intraocular pressure; JCT, juxta-canalicular tissue; POAG, primary open-angle glaucoma; RGC, retinal ganglion cell; RPE, retinal pigment epithelium; RPTPβ/ζ, receptor protein tyrosine phosphatase β/ζ; TGF, transforming growth factor; TLR4, toll-like receptor 4; TM, trabecular meshwork; Tnc, tenascin-C; Tnr, tenascin-R.
References
Abu-Hassan, D. W., Acott, T. S., and Kelley, M. J. (2014). The trabecular meshwork: a basic review of form and function. J. Ocul. Biol. 2:1. doi: 10.13188/2334-2838.1000017
Adamsky, K., Schilling, J., Garwood, J., Faissner, A., and Peles, E. (2001). Glial tumor cell adhesion is mediated by binding of the FNIII domain of receptor protein tyrosine phosphatase β (RPTPβ) to tenascin C. Oncogene 20, 609–618. doi: 10.1038/sj.onc.1204119
Afshari, F. T., Kwok, J. C., Andrews, M. R., Blits, B., Martin, K. R., Faissner, A., et al. (2010). Integrin activation or alpha 9 expression allows retinal pigmented epithelial cell adhesion on Bruch’s membrane in wet age-related macular degeneration. Brain 133, 448–464. doi: 10.1093/brain/awp319
Agathocleous, M., and Harris, W. A. (2009). From progenitors to differentiated cells in the vertebrate retina. Annu. Rev. Cell Dev. Biol. 25, 45–69. doi: 10.1146/annurev.cellbio.042308.113259
Ahmed, Z., Dent, R. G., Leadbeater, W. E., Smith, C., Berry, M., and Logan, A. (2005). Matrix metalloproteases: degradation of the inhibitory environment of the transected optic nerve and the scar by regenerating axons. Mol. Cell. Neurosci. 28, 64–78. doi: 10.1016/j.mcn.2004.08.013
Ajemian, A., Ness, R., and David, S. (1994). Tenascin in the injured rat optic nerve and in non-neuronal cells in vitro: potential role in neural repair. J. Comp. Neurol. 340, 233–242. doi: 10.1002/cne.903400208
Andrews, M. R., Czvitkovich, S., Dassie, E., Vogelaar, C. F., Faissner, A., Blits, B., et al. (2009). α9 integrin promotes neurite outgrowth on tenascin-C and enhances sensory axon regeneration. J. Neurosci. 29, 5546–5557. doi: 10.1523/JNEUROSCI.0759-09.2009
Barnea, G., Grumet, M., Milev, P., Silvennoinen, O., Levy, J. B., Sap, J., et al. (1994). Receptor tyrosine phosphatase β is expressed in the form of proteoglycan and binds to the extracellular matrix protein tenascin. J. Biol. Chem. 269, 14349–14352.
Bartsch, U., Faissner, A., Trotter, J., Dörries, U., Bartsch, S., Mohajeri, H., et al. (1994). Tenascin demarcates the boundary between the myelinated and nonmyelinated part of retinal ganglion cell axons in the developing and adult mouse. J. Neurosci. 14, 4756–4768.
Bartsch, S., Husmann, K., Schachner, M., and Bartsch, U. (1995). The extracellular matrix molecule tenascin: expression in the developing chick retinotectal system and substrate properties for retinal ganglion cell neurites in vitro. Eur. J. Neurosci. 7, 907–916. doi: 10.1111/j.1460-9568.1995.tb01078.x
Bartsch, U., Pesheva, P., Raff, M., and Schachner, M. (1993). Expression of janusin (J1–160/180) in the retina and optic nerve of the developing and adult mouse. Glia 9, 57–69. doi: 10.1002/glia.440090108
Battisti, W. P., Wang, J., Bozek, K., and Murray, M. (1995). Macrophages, microglia, and astrocytes are rapidly activated after crush injury of the goldfish optic nerve: a light and electron microscopic analysis. J. Comp. Neurol. 354, 306–320. doi: 10.1002/cne.903540211
Becker, T., Anliker, B., Becker, C. G., Taylor, J., Schachner, M., Meyer, R. L., et al. (2000). Tenascin-R inhibits regrowth of optic fibers in vitro and persists in the optic nerve of mice after injury. Glia 29, 330–346. doi: 10.1002/(sici)1098-1136(20000215)29:4<330::aid-glia4>3.0.co;2-l
Becker, C. G., and Becker, T. (2002). Repellent guidance of regenerating optic axons by chondroitin sulfate glycosaminoglycans in zebrafish. J. Neurosci. 22, 842–853.
Becker, C. G., Becker, T., Meyer, R. L., and Schachner, M. (1999). Tenascin-R inhibits the growth of optic fibers in vitro but is rapidly eliminated during nerve regeneration in the salamander Pleurodeles waltl. J. Neurosci. 19, 813–827.
Becker, C. G., Schweitzer, J., Feldner, J., Schachner, M., and Becker, T. (2004). Tenascin-R as a repellent guidance molecule for newly growing and regenerating optic axons in adult zebrafish. Mol. Cell. Neurosci. 26, 376–389. doi: 10.1016/j.mcn.2004.03.003
Bernhardt, R. R., Tongiorgi, E., Anzini, P., and Schachner, M. (1996). Increased expression of specific recognition molecules by retinal ganglion cells and by optic pathway glia accompanies the successful regeneration of retinal axons in adult zebrafish. J. Comp. Neurol. 376, 253–264. doi: 10.1002/(sici)1096-9861(19961209)376:2<253::aid-cne7>3.0.co;2-2
Besser, M., Horvat-Bröcker, A., Eysel, U. T., and Faissner, A. (2009). Differential expression of receptor protein tyrosine phosphatases accompanies the reorganisation of the retina upon laser lesion. Exp. Brain Res. 198, 37–47. doi: 10.1007/s00221-009-1932-0
Besser, M., Jagatheaswaran, M., Reinhard, J., Schaffelke, P., and Faissner, A. (2012). Tenascin C regulates proliferation and differentiation processes during embryonic retinogenesis and modulates the de-differentiation capacity of Muller glia by influencing growth factor responsiveness and the extracellular matrix compartment. Dev. Biol. 369, 163–176. doi: 10.1016/j.ydbio.2012.05.020
Bhutto, I., and Lutty, G. (2012). Understanding age-related macular degeneration (AMD): relationships between the photoreceptor/retinal pigment epithelium/Bruch’s membrane/choriocapillaris complex. Mol. Aspects Med. 33, 295–317. doi: 10.1016/j.mam.2012.04.005
Bourne, R. R. A., Taylor, H. R., Flaxman, S. R., Keeffe, J., Leasher, J., Naidoo, K., et al. (2016). Number of people blind or visually impaired by glaucoma worldwide and in world regions 1990–2010: a meta-analysis. PLoS One 11:e0162229 doi: 10.1371/journal.pone.0162229
Brösicke, N., and Faissner, A. (2015). Role of tenascins in the ECM of gliomas. Cell Adh. Migr. 9, 131–140. doi: 10.1080/19336918.2014.1000071
Brösicke, N., van Landeghem, F. K., Scheffler, B., and Faissner, A. (2013). Tenascin-C is expressed by human glioma in vivo and shows a strong association with tumor blood vessels. Cell Tissue Res. 354, 409–430. doi: 10.1007/s00441-013-1704-9
Calkins, D. J., Pekny, M., Cooper, M. L., and Benowitz, L. (2017). The challenge of regenerative therapies for the optic nerve in glaucoma. Exp. Eye Res. 157, 28–33. doi: 10.1016/j.exer.2017.01.007
Casola, C., Schiwek, J. E., Reinehr, S., Kuehn, S., Grus, F. H., Kramer, M., et al. (2015). S100 alone has the same destructive effect on retinal ganglion cells as in combination with HSP 27 in an autoimmune glaucoma model. J. Mol. Neurosci. 56, 228–236. doi: 10.1007/s12031-014-0485-2
Cepko, C. L., Austin, C. P., Yang, X., Alexiades, M., and Ezzeddine, D. (1996). Cell fate determination in the vertebrate retina. Proc. Natl. Acad. Sci. U S A 93, 589–595. doi: 10.1016/j.tins.2012.05.004
Cheah, M., Andrews, M. R., Chew, D. J., Moloney, E. B., Verhaagen, J., Fässler, R., et al. (2016). Expression of an activated integrin promotes long-distance sensory axon regeneration in the spinal cord. J. Neurosci. 36, 7283–7297. doi: 10.1523/JNEUROSCI.0901-16.2016
Chiquet-Ehrismann, R., Matsuoka, Y., Hofer, U., Spring, J., Bernasconi, C., and Chiquet, M. (1991). Tenascin variants: differential binding to fibronectin and distinct distribution in cell cultures and tissues. Cell Regul. 2, 927–938. doi: 10.1091/mbc.2.11.927
Chiquet-Ehrismann, R., Orend, G., Chiquet, M., Tucker, R. P., and Midwood, K. S. (2014). Tenascins in stem cell niches. Matrix Biol. 37, 112–123. doi: 10.1016/j.matbio.2014.01.007
Chiquet-Ehrismann, R., and Tucker, R. P. (2011). Tenascins and the importance of adhesion modulation. Cold Spring Harb. Perspect. Biol. 3:a004960. doi: 10.1101/cshperspect.a004960
Chung, C. Y., Zardi, L., and Erickson, H. P. (1995). Binding of tenascin-C to soluble fibronectin and matrix fibrils. J. Biol. Chem. 270, 29012–29017. doi: 10.1074/jbc.270.48.29012
Cipriani, V., Leung, H. T., Plagnol, V., Bunce, C., Khan, J. C., Shahid, H., et al. (2012). Genome-wide association study of age-related macular degeneration identifies associated variants in the TNXB-FKBPL-NOTCH4 region of chromosome 6p21.3. Hum. Mol. Genet. 21, 4138–4150. doi: 10.1093/hmg/dds225
Coleman, D. J., Silverman, R. H., Rondeau, M. J., Lloyd, H. O., Khanifar, A. A., and Chan, R. V. (2013). Age-related macular degeneration: choroidal ischaemia? Br. J. Ophthalmol. 97, 1020–1023. doi: 10.1136/bjophthalmol-2013-303143
Crabb, J. W. (2014). The proteomics of drusen. Cold Spring Harb. Perspect. Med. 4:a017194. doi: 10.1101/cshperspect.a017194
Crair, M. C., and Mason, C. A. (2016). Reconnecting eye to brain. J. Neurosci. 36, 10707–10722. doi: 10.1523/JNEUROSCI.1711-16.2016
Czopka, T., von Holst, A., ffrench-Constant, C., and Faissner, A. (2010). Regulatory mechanisms that mediate tenascin C-dependent inhibition of oligodendrocyte precursor differentiation. J. Neurosci. 30, 12310–12322. doi: 10.1523/JNEUROSCI.4957-09.2010
D’Alessandri, L., Ranscht, B., Winterhalter, K. H., and Vaughan, L. (1995). Contactin/F11 and tenascin-C co-expression in the chick retina correlates with formation of the synaptic plexiform layers. Curr. Eye Res. 14, 911–926. doi: 10.3109/02713689508995131
Dautriche, C. N., Xie, Y., and Sharfstein, S. T. (2014). Walking through trabecular meshwork biology: toward engineering design of outflow physiology. Biotechnol. Adv. 32, 971–983. doi: 10.1016/j.biotechadv.2014.04.012
De Laporte, L., Rice, J. J., Tortelli, F., and Hubbell, J. A. (2013). Tenascin C promiscuously binds growth factors via its fifth fibronectin type III-like domain. PLoS One 8:e62076. doi: 10.1371/journal.pone.0062076
Diekmann, H., and Fischer, D. (2013). Glaucoma and optic nerve repair. Cell Tissue Res. 353, 327–337. doi: 10.1007/s00441-013-1596-8
Ding, X., Patel, M., and Chan, C. C. (2009). Molecular pathology of age-related macular degeneration. Prog. Retin. Eye Res. 28, 1–18. doi: 10.1016/j.preteyeres.2008.10.001
Dityatev, A., Schachner, M., and Sonderegger, P. (2010). The dual role of the extracellular matrix in synaptic plasticity and homeostasis. Nat. Rev. Neurosci. 11, 735–746. doi: 10.1038/nrn2898
Dobbertin, A., Czvitkovich, S., Theocharidis, U., Garwood, J., Andrews, M. R., Properzi, F., et al. (2010). Analysis of combinatorial variability reveals selective accumulation of the fibronectin type III domains B and D of tenascin-C in injured brain. Exp. Neurol. 225, 60–73. doi: 10.1016/j.expneurol.2010.04.019
Dörries, U., and Schachner, M. (1994). Tenascin mRNA isoforms in the developing mouse brain. J. Neurosci. Res. 37, 336–347. doi: 10.1002/jnr.490370306
Dyer, M. A., and Cepko, C. L. (2001). Regulating proliferation during retinal development. Nat. Rev. Neurosci. 2, 333–342. doi: 10.1038/35072555
Dzyubenko, E., Gottschling, C., and Faissner, A. (2016). Neuron-glia interactions in neural plasticity: contributions of neural extracellular matrix and perineuronal nets. Neural Plast. 2016:5214961. doi: 10.1155/2016/5214961
EGS. (2017). European glaucoma society terminology and guidelines for glaucoma, 4th edition—chapter 2: classification and terminologysupported by the EGS foundation: part 1: foreword; introduction; glossary; chapter 2 classification and terminology. Br. J. Ophthalmol. 101, 73–127. doi: 10.1136/bjophthalmol-2016-egsguideline.002
Erskine, L., and Herrera, E. (2014). Connecting the retina to the brain. ASN Neuro 6:1759091414562107. doi: 10.1177/1759091414562107
Faissner, A. (1997). The tenascin gene family in axon growth and guidance. Cell Tissue Res. 290, 331–341. doi: 10.1007/978-3-642-60905-3_19
Faissner, A., and Kruse, J. (1990). J1/tenascin is a repulsive substrate for central nervous system neurons. Neuron 5, 627–637. doi: 10.1016/0896-6273(90)90217-4
Faissner, A., and Reinhard, J. (2015). The extracellular matrix compartment of neural stem and glial progenitor cells. Glia 63, 1330–1349. doi: 10.1002/glia.22839
Faissner, A., Roll, L., and Theocharidis, U. (2017). Tenascin-C in the matrisome of neural stem and progenitor cells. Mol. Cell. Neurosci. 81, 22–31. doi: 10.1016/j.mcn.2016.11.003
Fasler-Kan, E., Wunderlich, K., Hildebrand, P., Flammer, J., and Meyer, P. (2005). Activated STAT 3 in choroidal neovascular membranes of patients with age-related macular degeneration. Ophthalmologica 219, 214–221. doi: 10.1159/000085730
Fawcett, J. W. (2017). An integrin approach to axon regeneration. Eye 31, 206–208. doi: 10.1038/eye.2016.293
Fernandez-Godino, R., Pierce, E. A., and Garland, D. L. (2016). Extracellular matrix alterations and deposit formation in AMD. Adv. Exp. Med. Biol. 854, 53–58. doi: 10.1007/978-3-319-17121-0_8
Ferrer, E. (2006). Trabecular meshwork as a new target for the treatment of glaucoma. Drug News Perspect. 19, 151–158. doi: 10.1358/dnp.2006.19.3.985929
Ffrench-Constant, C., Miller, R. H., Burne, J. F., and Raff, M. C. (1988). Evidence that migratory oligodendrocyte-type-2 astrocyte (O-2A) progenitor cells are kept out of the rat retina by a barrier at the eye-end of the optic nerve. J. Neurocytol. 17, 13–25. doi: 10.1007/bf01735374
Fischer, D. (2012). Stimulating axonal regeneration of mature retinal ganglion cells and overcoming inhibitory signaling. Cell Tissue Res. 349, 79–85. doi: 10.1007/s00441-011-1302-7
Fischer, D., and Leibinger, M. (2012). Promoting optic nerve regeneration. Prog. Retin. Eye Res. 31, 688–701. doi: 10.1016/j.preteyeres.2012.06.005
Fuchshofer, R., and Tamm, E. R. (2012). The role of TGF-β in the pathogenesis of primary open-angle glaucoma. Cell Tissue Res. 347, 279–290. doi: 10.1007/s00441-011-1274-7
Gabelt, B. T., and Kaufman, P. L. (2005). Changes in aqueous humor dynamics with age and glaucoma. Prog. Retin. Eye Res. 24, 612–637. doi: 10.1016/j.preteyeres.2004.10.003
Garwood, J., Garcion, E., Dobbertin, A., Heck, N., Calco, V., ffrench-Constant, C., et al. (2004). The extracellular matrix glycoprotein Tenascin-C is expressed by oligodendrocyte precursor cells and required for the regulation of maturation rate, survival and responsiveness to platelet-derived growth factor. Eur. J. Neurosci. 20, 2524–2540. doi: 10.1111/j.1460-9568.2004.03727.x
Garwood, J., Schnadelbach, O., Clement, A., Schütte, K., Bach, A., and Faissner, A. (1999). DSD-1-proteoglycan is the mouse homolog of phosphacan and displays opposing effects on neurite outgrowth dependent on neuronal lineage. J. Neurosci. 19, 3888–3899.
Gauthier, A. C., and Liu, J. (2016). Neurodegeneration and neuroprotection in glaucoma. Yale J. Biol. Med. 89, 73–79.
Giuffrida, A., Scarpa, S., Birarelli, P., and Modesti, A. (2004). The interaction of tenascin-C with fibronectin modulates the migration and specific metalloprotease activity in human mesothelioma cell lines of different histotype. Int. J. Oncol. 25, 745–750. doi: 10.3892/ijo.25.3.745
Gotz, B., Scholze, A., Clement, A., Joester, A., Schutte, K., Wigger, F., et al. (1996). Tenascin-C contains distinct adhesive, anti-adhesive, and neurite outgrowth promoting sites for neurons. J. Cell Biol. 132, 681–699. doi: 10.1083/jcb.132.4.681
Hagedorn, M., Esser, P., Wiedemann, P., and Heimann, K. (1993). Tenascin and decorin in epiretinal membranes of proliferative vitreoretinopathy and proliferative diabetic retinopathy. Ger. J. Ophthalmol. 2, 28–31.
Harada, T., Harada, C., and Parada, L. F. (2007). Molecular regulation of visual system development: more than meets the eye. Genes Dev. 21, 367–378. doi: 10.1101/gad.1504307
Hatakeyama, J., and Kageyama, R. (2004). Retinal cell fate determination and bHLH factors. Semin. Cell Dev. Biol. 15, 83–89. doi: 10.1016/j.semcdb.2003.09.005
Heavner, W., and Pevny, L. (2012). Eye development and retinogenesis. Cold Spring Harb. Perspect. Biol. 4:a008391. doi: 10.1101/cshperspect.a008391
Heikkinen, A., Pihlajaniemi, T., Faissner, A., and Yuzaki, M. (2014). Neural ECM and synaptogenesis. Prog. Brain Res. 214, 29–51. doi: 10.1016/B978-0-444-63486-3.00002-5
Horvat-Bröcker, A., Reinhard, J., Illes, S., Paech, T., Zoidl, G., Harroch, S., et al. (2008). Receptor protein tyrosine phosphatases are expressed by cycling retinal progenitor cells and involved in neuronal development of mouse retina. Neuroscience 152, 618–645. doi: 10.1016/j.neuroscience.2008.01.016
Isenmann, S., Kretz, A., and Cellerino, A. (2003). Molecular determinants of retinal ganglion cell development, survival, and regeneration. Prog. Retin. Eye Res. 22, 483–543. doi: 10.1016/s1350-9462(03)00027-2
Ishikawa, K., Yoshida, S., Kobayashi, Y., Zhou, Y., Nakama, T., Nakao, S., et al. (2015). Microarray analysis of gene expression in fibrovascular membranes excised from patients with proliferative diabetic retinopathy. Invest. Ophthalmol. Vis. Sci. 56, 932–946. doi: 10.1167/iovs.14-15589
Jager, R. D., Mieler, W. F., and Miller, J. W. (2008). Age-related macular degeneration. N. Engl. J. Med. 358, 2606–2617. doi: 10.1056/NEJMra0801537
Jakovcevski, I., Miljkovic, D., Schachner, M., and Andjus, P. R. (2013). Tenascins and inflammation in disorders of the nervous system. Amino. Acids 44, 1115–1127. doi: 10.1007/s00726-012-1446-0
Joachim, S. C., Mondon, C., Gramlich, O. W., Grus, F. H., and Dick, H. B. (2014). Apoptotic retinal ganglion cell death in an autoimmune glaucoma model is accompanied by antibody depositions. J. Mol. Neurosci. 52, 216–224. doi: 10.1007/s12031-013-0125-2
Joachim, S. C., Reinehr, S., Kuehn, S., Laspas, P., Gramlich, O. W., Kuehn, M., et al. (2013). Immune response against ocular tissues after immunization with optic nerve antigens in a model of autoimmune glaucoma. Mol. Vis. 19, 1804–1814.
Joester, A., and Faissner, A. (1999). Evidence for combinatorial variability of tenascin-C isoforms and developmental regulation in the mouse central nervous system. J. Biol. Chem. 274, 17144–17151. doi: 10.1074/jbc.274.24.17144
Joester, A., and Faissner, A. (2001). The structure and function of tenascins in the nervous system. Matrix Biol. 20, 13–22. doi: 10.1016/s0945-053x(00)00136-0
Johnson, E. C., Jia, L., Cepurna, W. O., Doser, T. A., and Morrison, J. C. (2007). Global changes in optic nerve head gene expression after exposure to elevated intraocular pressure in a rat glaucoma model. Invest. Ophthalmol. Vis. Sci. 48, 3161–3177. doi: 10.1167/iovs.06-1282
Jones, F. S., and Jones, P. L. (2000). The tenascin family of ECM glycoproteins: structure, function, and regulation during embryonic development and tissue remodeling. Dev. Dyn. 218, 235–259. doi: 10.1002/(sici)1097-0177(200006)218:2<235::aid-dvdy2>3.3.co;2-7
Jones, P. L., and Jones, F. S. (2000). Tenascin-C in development and disease: gene regulation and cell function. Matrix Biol. 19, 581–596. doi: 10.1016/s0945-053x(00)00106-2
Keller, K. E., Kelley, M. J., and Acott, T. S. (2007). Extracellular matrix gene alternative splicing by trabecular meshwork cells in response to mechanical stretching. Invest. Ophthalmol. Vis. Sci. 48, 1164–1172. doi: 10.1167/iovs.06-0875
Keller, K. E., Vranka, J. A., Haddadin, R. I., Kang, M. H., Oh, D. J., Rhee, D. J., et al. (2013). The effects of tenascin C knockdown on trabecular meshwork outflow resistance. Invest. Ophthalmol. Vis. Sci. 54, 5613–5623. doi: 10.1167/iovs.13-11620
Kiernan, B. W., Garcion, E., Ferguson, J., Frost, E. E., Torres, E. M., Dunnett, S. B., et al. (1999). Myelination and behaviour of tenascin-C null transgenic mice. Eur. J. Neurosci. 11, 3082–3092. doi: 10.1046/j.1460-9568.1999.00729.x
Kim, H., Ahn, M., Choi, S., Kim, M., Sim, K. B., Kim, J., et al. (2013). Potential role of fibronectin in microglia/macrophage activation following cryoinjury in the rat brain: an immunohistochemical study. Brain Res. 1502, 11–19. doi: 10.1016/j.brainres.2013.01.043
Kim, H. J., Woo, S. J., Suh, E. J., Ahn, J., Park, J. H., Hong, H. K., et al. (2014). Identification of vinculin as a potential plasma marker for age-related macular degeneration. Invest. Ophthalmol. Vis. Sci. 55, 7166–7176. doi: 10.1167/iovs.14-15168
Klausmeyer, A., Garwood, J., and Faissner, A. (2007). Differential expression of phosphacan/RPTPβ isoforms in the developing mouse visual system. J. Comp. Neurol. 504, 659–679. doi: 10.1002/cne.21479
Kobayashi, Y., Yoshida, S., Zhou, Y., Nakama, T., Ishikawa, K., Arima, M., et al. (2016a). Tenascin-C promotes angiogenesis in fibrovascular membranes in eyes with proliferative diabetic retinopathy. Mol. Vis. 22, 436–445.
Kobayashi, Y., Yoshida, S., Zhou, Y., Nakama, T., Ishikawa, K., Kubo, Y., et al. (2016b). Tenascin-C secreted by transdifferentiated retinal pigment epithelial cells promotes choroidal neovascularization via integrin αV. Lab. Invest. 96, 1178–1188. doi: 10.1038/labinvest.2016.99
Kuriyama, N., Duarte, S., Hamada, T., Busuttil, R. W., and Coito, A. J. (2011). Tenascin-C: a novel mediator of hepatic ischemia and reperfusion injury. Hepatology 54, 2125–2136. doi: 10.1002/hep.24639
Kwok, J. C., Dick, G., Wang, D., and Fawcett, J. W. (2011). Extracellular matrix and perineuronal nets in CNS repair. Dev. Neurobiol. 71, 1073–1089. doi: 10.1002/dneu.20974
Lang, D. M., Monzon-Mayor, M., Del Mar Romero-Aleman, M., Yanes, C., Santos, E., and Pesheva, P. (2008). Tenascin-R and axon growth-promoting molecules are up-regulated in the regenerating visual pathway of the lizard (Gallotia galloti). Dev. Neurobiol. 68, 899–916. doi: 10.1002/dneu.20624
Lim, L. S., Mitchell, P., Seddon, J. M., Holz, F. G., and Wong, T. Y. (2012). Age-related macular degeneration. Lancet 379, 1728–1738. doi: 10.1016/S0140-6736(12)60282-7
Loers, G., and Schachner, M. (2007). Recognition molecules and neural repair. J. Neurochem. 101, 865–882. doi: 10.1111/j.1471-4159.2006.04409.x
Lu, A., Tang, Y., Ran, R., Clark, J. F., Aronow, B. J., and Sharp, F. R. (2003). Genomics of the periinfarction cortex after focal cerebral ischemia. J. Cereb. Blood Flow Metab. 23, 786–810. doi: 10.1097/01.WCB.0000062340.80057.06
Lundell, A., Olin, A. I., Mörgelin, M., al-Karadaghi, S., Aspberg, A., and Logan, D. T. (2004). Structural basis for interactions between tenascins and lectican C-type lectin domains: evidence for a crosslinking role for tenascins. Structure 12, 1495–1506. doi: 10.1016/j.str.2004.05.021
Marquardt, T. (2003). Transcriptional control of neuronal diversification in the retina. Prog. Retin. Eye Res. 22, 567–577. doi: 10.1016/s1350-9462(03)00036-3
McLaughlin, T., Hindges, R., and O’Leary, D. D. (2003). Regulation of axial patterning of the retina and its topographic mapping in the brain. Curr. Opin. Neurobiol. 13, 57–69. doi: 10.1016/s0959-4388(03)00014-x
Midwood, K. S., Chiquet, M., Tucker, R. P., and Orend, G. (2016). Tenascin-C at a glance. J. Cell Sci. 129, 4321–4327. doi: 10.1242/jcs.190546
Midwood, K. S., and Orend, G. (2009). The role of tenascin-C in tissue injury and tumorigenesis. J. Cell Commun. Signal. 3, 287–310. doi: 10.1007/s12079-009-0075-1
Midwood, K., Sacre, S., Piccinini, A. M., Inglis, J., Trebaul, A., Chan, E., et al. (2009). Tenascin-C is an endogenous activator of Toll-like receptor 4 that is essential for maintaining inflammation in arthritic joint disease. Nat. Med. 15, 774–780. doi: 10.1038/nm.1987
Milev, P., Chiba, A., Haring, M., Rauvala, H., Schachner, M., Ranscht, B., et al. (1998). High affinity binding and overlapping localization of neurocan and phosphacan/protein-tyrosine phosphatase-ζ/β with tenascin-R, amphoterin, and the heparin-binding growth-associated molecule. J. Biol. Chem. 273, 6998–7005. doi: 10.1074/jbc.273.12.6998
Milev, P., Fischer, D., Häring, M., Schulthess, T., Margolis, R. K., Chiquet-Ehrismann, R., et al. (1997). The fibrinogen-like globe of tenascin-C mediates its interactions with neurocan and phosphacan/protein-tyrosine phosphatase-ζ/β. J. Biol. Chem. 272, 15501–15509. doi: 10.1074/jbc.272.24.15501
Mizener, J. B., Podhajsky, P., and Hayreh, S. S. (1997). Ocular ischemic syndrome. Ophthalmology 104, 859–864. doi: 10.1016/S0161-6420(97)30221-8
Momcilovic, M., Stamenkovic, V., Jovanovic, M., Andjus, P. R., Jakovcevski, I., Schachner, M., et al. (2017). Tenascin-C deficiency protects mice from experimental autoimmune encephalomyelitis. J. Neuroimmunol. 302, 1–6. doi: 10.1016/j.jneuroim.2016.12.001
Morrison, J. C. (2006). Integrins in the optic nerve head: potential roles in glaucomatous optic neuropathy (an American Ophthalmological Society thesis). Trans. Am. Ophthalmol. Soc. 104, 453–477.
Naba, A., Clauser, K. R., Ding, H., Whittaker, C. A., Carr, S. A., and Hynes, R. O. (2016). The extracellular matrix: tools and insights for the “omics” era. Matrix Biol. 49, 10–24. doi: 10.1016/j.matbio.2015.06.003
Navarro-Partida, J., Alvarado Castillo, B., Martinez-Rizo, A. B., Rosales-Diaz, R., Velazquez-Fernandez, J. B., and Santos, A. (2017a). Association of single-nucleotide polymorphisms in non-coding regions of the TLR4 gene with primary open angle glaucoma in a Mexican population. Ophthalmic Genet. 38, 325–329. doi: 10.1080/13816810.2016.1227454
Navarro-Partida, J., Martinez-Rizo, A. B., Ramirez-Barrera, P., Velazquez-Fernandez, J. B., Mondragon-Jaimes, V. A., Santos-Garcia, A., et al. (2017b). Association of Toll-like receptor 4 single-nucleotide polymorphisms Asp299Gly and Thr399Ile with the risk of primary open angle glaucoma. Graefes Arch. Clin. Exp. Ophthalmol. 255, 995–1001. doi: 10.1007/s00417-017-3610-4
Nicolò, M., Piccolino, F. C., Zardi, L., Giovannini, A., and Mariotti, C. (2000). Detection of tenascin-C in surgically excised choroidal neovascular membranes. Graefes Arch. Clin. Exp. Ophthalmol. 238, 107–111. doi: 10.1007/s004170050018
Nies, D. E., Hemesath, T. J., Kim, J. H., Gulcher, J. R., and Stefansson, K. (1991). The complete cDNA sequence of human hexabrachion (Tenascin). A multidomain protein containing unique epidermal growth factor repeats. J. Biol. Chem. 266, 2818–2823.
Noristani, R., Kuehn, S., Stute, G., Reinehr, S., Stellbogen, M., Dick, H. B., et al. (2016). Retinal and optic nerve damage is associated with early glial responses in an experimental autoimmune glaucoma model. J. Mol. Neurosci. 58, 470–482. doi: 10.1007/s12031-015-0707-2
Orend, G., and Chiquet-Ehrismann, R. (2006). Tenascin-C induced signaling in cancer. Cancer Lett. 244, 143–163. doi: 10.1016/j.canlet.2006.02.017
Oster, S. F., Deiner, M., Birgbauer, E., and Sretavan, D. W. (2004). Ganglion cell axon pathfinding in the retina and optic nerve. Semin. Cell Dev. Biol. 15, 125–136. doi: 10.1016/j.semcdb.2003.09.006
Palenski, T. L., Sorenson, C. M., and Sheibani, N. (2013). Inflammatory cytokine-specific alterations in retinal endothelial cell function. Microvasc. Res. 89, 57–69. doi: 10.1016/j.mvr.2013.06.007
Pattabiraman, P. P., and Rao, P. V. (2010). Mechanistic basis of Rho GTPase-induced extracellular matrix synthesis in trabecular meshwork cells. Am. J. Physiol. Cell Physiol. 298, C749–C763. doi: 10.1152/ajpcell.00317.2009
Pena, J. D., Varela, H. J., Ricard, C. S., and Hernandez, M. R. (1999). Enhanced tenascin expression associated with reactive astrocytes in human optic nerve heads with primary open angle glaucoma. Exp. Eye Res. 68, 29–40. doi: 10.1006/exer.1998.0577
Pesheva, P., Gennarini, G., Goridis, C., and Schachner, M. (1993). The F3/11 cell adhesion molecule mediates the repulsion of neurons by the extracellular matrix glycoprotein J1–160/180. Neuron 10, 69–82. doi: 10.1016/0896-6273(93)90243-k
Petros, T. J., Rebsam, A., and Mason, C. A. (2008). Retinal axon growth at the optic chiasm: to cross or not to cross. Annu. Rev. Neurosci. 31, 295–315. doi: 10.1146/annurev.neuro.31.060407.125609
Piccinini, A. M., and Midwood, K. S. (2012). Endogenous control of immunity against infection: tenascin-C regulates TLR4-mediated inflammation via microRNA-155. Cell Rep. 2, 914–926. doi: 10.1016/j.celrep.2012.09.005
Piccinini, A. M., Zuliani-Alvarez, L., Lim, J. M., and Midwood, K. S. (2016). Distinct microenvironmental cues stimulate divergent TLR4-mediated signaling pathways in macrophages. Sci. Signal. 9:ra86. doi: 10.1126/scisignal.aaf3596
Prendes, M. A., Harris, A., Wirostko, B. M., Gerber, A. L., and Siesky, B. (2013). The role of transforming growth factor β in glaucoma and the therapeutic implications. Br. J. Ophthalmol. 97, 680–686. doi: 10.1136/bjophthalmol-2011-301132
Probstmeier, R., Braunewell, K., and Pesheva, P. (2000). Involvement of chondroitin sulfates on brain-derived tenascin-R in carbohydrate-dependent interactions with fibronectin and tenascin-C. Brain Res. 863, 42–51. doi: 10.1016/s0006-8993(00)02075-8
Quigley, H. A., and Broman, A. T. (2006). The number of people with glaucoma worldwide in 2010 and 2020. Br. J. Ophthalmol. 90, 262–267. doi: 10.1136/bjo.2005.081224
Ramirez, A. I., de Hoz, R., Salobrar-Garcia, E., Salazar, J. J., Rojas, B., Ajoy, D., et al. (2017). The role of microglia in retinal neurodegeneration: Alzheimer’s disease, Parkinson, and glaucoma. Front. Aging Neurosci. 9:214. doi: 10.3389/fnagi.2017.00214
Rauch, U., Clement, A., Retzler, C., Fröhlich, L., Fässler, R., Göhring, W., et al. (1997). Mapping of a defined neurocan binding site to distinct domains of tenascin-C. J. Biol. Chem. 272, 26905–26912. doi: 10.1074/jbc.272.43.26905
Reinehr, S., Reinhard, J., Gandej, M., Kuehn, S., Noristani, R., Faissner, A., et al. (2016a). Simultaneous complement response via lectin pathway in retina and optic nerve in an experimental autoimmune glaucoma model. Front. Cell. Neurosci. 10:140. doi: 10.3389/fncel.2016.00140
Reinehr, S., Reinhard, J., Wiemann, S., Stute, G., Kuehn, S., Woestmann, J., et al. (2016b). Early remodelling of the extracellular matrix proteins tenascin-C and phosphacan in retina and optic nerve of an experimental autoimmune glaucoma model. J. Cell. Mol. Med. 20, 2122–2137. doi: 10.1111/jcmm.12909
Reinhard, J., Brösicke, N., Theocharidis, U., and Faissner, A. (2016). The extracellular matrix niche microenvironment of neural and cancer stem cells in the brain. Int. J. Biochem. Cell Biol. 81, 174–183. doi: 10.1016/j.biocel.2016.05.002
Reinhard, J., Joachim, S. C., and Faissner, A. (2015). Extracellular matrix remodeling during retinal development. Exp. Eye Res. 133, 132–140. doi: 10.1016/j.exer.2014.07.001
Reinhard, J., Renner, M., Wiemann, S., Shakoor, D. A., Stute, G., Dick, H. B., et al. (2017). Ischemic injury leads to extracellular matrix alterations in retina and optic nerve. Sci. Rep. 7:43470. doi: 10.1038/srep43470
Ren, T., van der Merwe, Y., and Steketee, M. B. (2015). Developing extracellular matrix technology to treat retinal or optic nerve injury (1,2,3). eNeuro 2:ENEURO.0077-15.2015. doi: 10.1523/ENEURO.0077-15.2015
Rieck, J. (2013). The pathogenesis of glaucoma in the interplay with the immune system. Invest. Ophthalmol. Vis. Sci. 54, 2393–2409. doi: 10.1167/iovs.12-9781
Rigato, F., Garwood, J., Calco, V., Heck, N., Faivre-Sarrailh, C., and Faissner, A. (2002). Tenascin-C promotes neurite outgrowth of embryonic hippocampal neurons through the alternatively spliced fibronectin type III BD domains via activation of the cell adhesion molecule F3/contactin. J. Neurosci. 22, 6596–6609.
Roll, L., and Faissner, A. (2014). Influence of the extracellular matrix on endogenous and transplanted stem cells after brain damage. Front. Cell. Neurosci. 8:219. doi: 10.3389/fncel.2014.00219
Roll, L., Mittmann, T., Eysel, U. T., and Faissner, A. (2012). The laser lesion of the mouse visual cortex as a model to study neural extracellular matrix remodeling during degeneration, regeneration and plasticity of the CNS. Cell Tissue Res. 349, 133–145. doi: 10.1007/s00441-011-1313-4
Saika, S., Yamanaka, O., Okada, Y., and Sumioka, T. (2016). Modulation of Smad signaling by non-TGFβ components in myofibroblast generation during wound healing in corneal stroma. Exp. Eye Res. 142, 40–48. doi: 10.1016/j.exer.2014.12.015
Sánchez-López, A., Cuadros, M. A., Calvente, R., Tassi, M., Marín-Teva, J. L., and Navascues, J. (2004). Radial migration of developing microglial cells in quail retina: a confocal microscopy study. Glia 46, 261–273. doi: 10.1002/glia.20007
Schachner, M., Taylor, J., Bartsch, U., and Pesheva, P. (1994). The perplexing multifunctionality of janusin, a tenascin-related molecule. Perspect. Dev. Neurobiol. 2, 33–41.
Schneider, M., and Fuchshofer, R. (2016). The role of astrocytes in optic nerve head fibrosis in glaucoma. Exp. Eye Res. 142, 49–55. doi: 10.1016/j.exer.2015.08.014
Schumacher, S., Jung, M., Nörenberg, U., Dorner, A., Chiquet-Ehrismann, R., Stuermer, C. A., et al. (2001). CALEB binds via its acidic stretch to the fibrinogen-like domain of tenascin-C or tenascin-R and its expression is dynamically regulated after optic nerve lesion. J. Biol. Chem. 276, 7337–7345. doi: 10.1074/jbc.M007234200
Schumacher, S., and Stübe, E. M. (2003). Regulated binding of the fibrinogen-like domains of tenascin-R and tenascin-C to the neural EGF family member CALEB. J. Neurochem. 87, 1213–1223. doi: 10.1046/j.1471-4159.2003.02112.x
Shibuya, E., Meguro, A., Ota, M., Kashiwagi, K., Mabuchi, F., Iijima, H., et al. (2008). Association of Toll-like receptor 4 gene polymorphisms with normal tension glaucoma. Invest. Ophthalmol. Vis. Sci. 49, 4453–4457. doi: 10.1167/iovs.07-1575
Siddiqui, S., Horvat-Bröcker, A., and Faissner, A. (2008). The glia-derived extracellular matrix glycoprotein tenascin-C promotes embryonic and postnatal retina axon outgrowth via the alternatively spliced fibronectin type III domain TNfnD. Neuron Glia Biol. 4, 271–283. doi: 10.1017/s1740925x09990020
Siddiqui, S., Horvat-Broecker, A., and Faissner, A. (2009). Comparative screening of glial cell types reveals extracellular matrix that inhibits retinal axon growth in a chondroitinase ABC-resistant fashion. Glia 57, 1420–1438. doi: 10.1002/glia.20860
Silver, J. (1994). Inhibitory molecules in development and regeneration. J. Neurol. 242, S22–S24. doi: 10.1007/bf00939236
Silver, D. J., and Silver, J. (2014). Contributions of chondroitin sulfate proteoglycans to neurodevelopment, injury and cancer. Curr. Opin. Neurobiol. 27, 171–178. doi: 10.1016/j.conb.2014.03.016
Sim, D. A., Keane, P. A., Zarranz-Ventura, J., Fung, S., Powner, M. B., Platteau, E., et al. (2013). The effects of macular ischemia on visual acuity in diabetic retinopathy. Invest. Ophthalmol. Vis. Sci. 54, 2353–2360. doi: 10.1167/iovs.12-11103
Siri, A., Carnemolla, B., Saginati, M., Leprini, A., Casari, G., Baralle, F., et al. (1991). Human tenascin: primary structure, pre-mRNA splicing patterns and localization of the epitopes recognized by two monoclonal antibodies. Nucleic Acids Res. 19, 525–531. doi: 10.1093/nar/19.3.525
Smith, G. M., and Hale, J. H. (1997). Macrophage/Microglia regulation of astrocytic tenascin: synergistic action of transforming growth factor-β and basic fibroblast growth factor. J. Neurosci. 17, 9624–9633.
Song, I., and Dityatev, A. (2017). Crosstalk between glia, extracellular matrix and neurons. Brain Res. Bull. doi: 10.1016/j.brainresbull.2017.03.003 [Epub ahead of print].
Spring, J., Beck, K., and Chiquet-Ehrismann, R. (1989). Two contrary functions of tenascin: dissection of the active sites by recombinant tenascin fragments. Cell 59, 325–334. doi: 10.1016/0092-8674(89)90294-8
Swindle, C. S., Tran, K. T., Johnson, T. D., Banerjee, P., Mayes, A. M., Griffith, L., et al. (2001). Epidermal growth factor (EGF)-like repeats of human tenascin-C as ligands for EGF receptor. J. Cell Biol. 154, 459–468. doi: 10.1083/jcb.200103103
Taki, J., Inaki, A., Wakabayashi, H., Imanaka-Yoshida, K., Ogawa, K., Hiroe, M., et al. (2010). Dynamic expression of tenascin-C after myocardial ischemia and reperfusion: assessment by 125I-anti-tenascin-C antibody imaging. J. Nucl. Med. 51, 1116–1122. doi: 10.2967/jnumed.109.071340
Taki, J., Inaki, A., Wakabayashi, H., Matsunari, I., Imanaka-Yoshida, K., Ogawa, K., et al. (2015). Effect of postconditioning on dynamic expression of tenascin-C and left ventricular remodeling after myocardial ischemia and reperfusion. EJNMMI Res. 5:21. doi: 10.1186/s13550-015-0100-8
Tamm, E. R., Braunger, B. M., and Fuchshofer, R. (2015). Intraocular pressure and the mechanisms involved in resistance of the aqueous humor flow in the trabecular meshwork outflow pathways. Prog. Mol. Biol. Transl. Sci. 134, 301–314. doi: 10.1016/bs.pmbts.2015.06.007
Tamm, E. R., Ethier, C. R., and Lasker/IRRF Initiative on Astrocytes and Glaucomatous Neurodegeneration Participants. (2017). Biological aspects of axonal damage in glaucoma: a brief review. Exp. Eye Res. 157, 5–12. doi: 10.1016/j.exer.2017.02.006
Tate, C. C., Tate, M. C., and LaPlaca, M. C. (2007). Fibronectin and laminin increase in the mouse brain after controlled cortical impact injury. J. Neurotrauma 24, 226–230. doi: 10.1089/neu.2006.0043
Tezel, G., and Fourth ARVO/Pfizer Ophthalmics Research Institute Conference Working Group. (2009). The role of glia, mitochondria and the immune system in glaucoma. Invest. Ophthalmol. Vis. Sci. 50, 1001–1012. doi: 10.1167/iovs.08-2717
Tezel, G., and Wax, M. B. (2004). The immune system and glaucoma. Curr. Opin. Ophthalmol. 15, 80–84. doi: 10.1097/00055735-200404000-00003
Thanos, S., and Mey, J. (2001). Development of the visual system of the chick. II. Mechanisms of axonal guidance. Brain Res. Rev. 35, 205–245. doi: 10.1016/S0165-0173(01)00049-2
Theocharidis, U., Long, K., ffrench-Constant, C., and Faissner, A. (2014). Regulation of the neural stem cell compartment by extracellular matrix constituents. Prog. Brain Res. 214, 3–28. doi: 10.1016/b978-0-444-63486-3.00001-3
To, M., Goz, A., Camenzind, L., Oertle, P., Candiello, J., Sullivan, M., et al. (2013). Diabetes-induced morphological, biomechanical and compositional changes in ocular basement membranes. Exp. Eye Res. 116, 298–307. doi: 10.1016/j.exer.2013.09.011
Tom, V. J., Doller, C. M., Malouf, A. T., and Silver, J. (2004). Astrocyte-associated fibronectin is critical for axonal regeneration in adult white matter. J. Neurosci. 24, 9282–9290. doi: 10.1523/jneurosci.2120-04.2004
Tucker, R. P., and Chiquet-Ehrismann, R. (2015). Tenascin-C: its functions as an integrin ligand. Int. J. Biochem. Cell Biol. 65, 165–168. doi: 10.1016/j.biocel.2015.06.003
Tucker, R. P., Drabikowski, K., Hess, J. F., Ferralli, J., Chiquet-Ehrismann, R., and Adams, J. C. (2006). Phylogenetic analysis of the tenascin gene family: evidence of origin early in the chordate lineage. BMC Evol. Biol. 6:60. doi: 10.1186/1471-2148-6-60
Ueda, J., and Yue, B. Y. (2003). Distribution of myocilin and extracellular matrix components in the corneoscleral meshwork of human eyes. Invest. Ophthalmol. Vis. Sci. 44, 4772–4779. doi: 10.1167/iovs.02-1002
Vecino, E., Rodriguez, F. D., Ruzafa, N., Pereiro, X., and Sharma, S. C. (2016). Glia-neuron interactions in the mammalian retina. Prog. Retin. Eye Res. 51, 1–40. doi: 10.1016/j.preteyeres.2015.06.003
Wahlin, K. J., Moreira, E. F., Huang, H., Yu, N., and Adler, R. (2008). Molecular dynamics of photoreceptor synapse formation in the developing chick retina. J. Comp. Neurol. 506, 822–837. doi: 10.1002/cne.21582
Wallace, D. M., Murphy-Ullrich, J. E., Downs, J. C., and O’Brien, C. J. (2014). The role of matricellular proteins in glaucoma. Matrix Biol. 37, 174–182. doi: 10.1016/j.matbio.2014.03.007
Wallace, D. M., Pokrovskaya, O., and O’Brien, C. J. (2015). The function of matricellular proteins in the lamina cribrosa and trabecular meshwork in glaucoma. J. Ocul. Pharmacol. Ther. 31, 386–395. doi: 10.1089/jop.2014.0163
Wang, J., Harris, A., Prendes, M. A., Alshawa, L., Gross, J. C., Wentz, S. M., et al. (2017). Targeting transforming growth factor-β signaling in primary open-angle glaucoma. J. Glaucoma 26, 390–395. doi: 10.1097/IJG.0000000000000627
Wang, A., Patrone, L., McDonald, J. A., and Sheppard, D. (1995). Expression of the integrin subunit α9 in the murine embryo. Dev. Dyn. 204, 421–431. doi: 10.1002/aja.1002040408
Weber, P., Bartsch, U., Rasband, M. N., Czaniera, R., Lang, Y., Bluethmann, H., et al. (1999). Mice deficient for tenascin-R display alterations of the extracellular matrix and decreased axonal conduction velocities in the CNS. J. Neurosci. 19, 4245–4262.
Wintergerst, E. S., Fuss, B., and Bartsch, U. (1993). Localization of janusin mRNA in the central nervous system of the developing and adult mouse. Eur. J. Neurosci. 5, 299–310. doi: 10.1111/j.1460-9568.1993.tb00497.x
Xiang, M. (2013). Intrinsic control of mammalian retinogenesis. Cell. Mol. Life Sci. 70, 2519–2532. doi: 10.1007/s00018-012-1183-2
Xiao, Z. C., Bartsch, U., Margolis, R. K., Rougon, G., Montag, D., and Schachner, M. (1997). Isolation of a tenascin-R binding protein from mouse brain membranes. A phosphacan-related chondroitin sulfate proteoglycan. J. Biol. Chem. 272, 32092–32101. doi: 10.1074/jbc.272.51.32092
Xiao, Z. C., Revest, J. M., Laeng, P., Rougon, G., Schachner, M., and Montag, D. (1998). Defasciculation of neurites is mediated by tenascin-R and its neuronal receptor F3/11. J. Neurosci. Res. 52, 390–404. doi: 10.1002/(sici)1097-4547(19980515)52:4<390::aid-jnr3>3.3.co;2-6
Yang, Y. F., Sun, Y. Y., Acott, T. S., and Keller, K. E. (2016). Effects of induction and inhibition of matrix cross-linking on remodeling of the aqueous outflow resistance by ocular trabecular meshwork cells. Sci. Rep. 6:30505. doi: 10.1038/srep30505
Yokosaki, Y., Matsuura, N., Higashiyama, S., Murakami, I., Obara, M., Yamakido, M., et al. (1998). Identification of the ligand binding site for the integrin α9 β1 in the third fibronectin type III repeat of tenascin-C. J. Biol. Chem. 273, 11423–11428. doi: 10.1074/jbc.273.19.11423
Zhu, W., Gramlich, O. W., Laboissonniere, L., Jain, A., Sheffield, V. C., Trimarchi, J. M., et al. (2016). Transplantation of iPSC-derived TM cells rescues glaucoma phenotypes in vivo. Proc. Natl. Acad. Sci. U S A 113, E3492–E3500. doi: 10.1073/pnas.1604153113
Zhu, W., Jain, A., Gramlich, O. W., Tucker, B. A., Sheffield, V. C., and Kuehn, M. H. (2017). Restoration of aqueous humor outflow following transplantation of iPSC-derived trabecular meshwork cells in a transgenic mouse model of glaucoma. Invest. Ophthalmol. Vis. Sci. 58, 2054–2062. doi: 10.1167/iovs.16-20672
Keywords: extracellular matrix, glaucoma, glycoprotein, neurodegeneration, optic nerve, retina, tenascin-C, tenascin-R
Citation: Reinhard J, Roll L and Faissner A (2017) Tenascins in Retinal and Optic Nerve Neurodegeneration. Front. Integr. Neurosci. 11:30. doi: 10.3389/fnint.2017.00030
Received: 15 August 2017; Accepted: 03 October 2017;
Published: 23 October 2017.
Edited by:
Harry Pantazopoulos, McLean Hospital, United StatesReviewed by:
Denise M. Inman, Northeast Ohio Medical University, United StatesEric D. Laywell, Florida State University College of Medicine, United States
Copyright © 2017 Reinhard, Roll and Faissner. This is an open-access article distributed under the terms of the Creative Commons Attribution License (CC BY). The use, distribution or reproduction in other forums is permitted, provided the original author(s) or licensor are credited and that the original publication in this journal is cited, in accordance with accepted academic practice. No use, distribution or reproduction is permitted which does not comply with these terms.
*Correspondence: Andreas Faissner, YW5kcmVhcy5mYWlzc25lckBydWIuZGU=