- Department of Entomology, University of Kentucky, Lexington, KY, United States
In insects and other animals, nutrition-mediated behaviors are modulated by communication between the brain and peripheral systems, a process that relies heavily on the insulin/insulin-like growth factor signaling pathway (IIS). Previous studies have focused on the mechanistic and physiological functions of insulin-like peptides (ILPs) in critical developmental and adult milestones like pupation or vitellogenesis. Less work has detailed the mechanisms connecting ILPs to adult nutrient-mediated behaviors related to survival and reproductive success. Here we briefly review the range of behaviors linked to IIS in insects, from conserved regulation of feeding behavior to evolutionarily derived polyphenisms. Where possible, we incorporate information from Drosophila melanogaster and other model species to describe molecular and neural mechanisms that connect nutritional status to behavioral expression via IIS. We identify knowledge gaps which include the diverse functional roles of peripheral ILPs, how ILPs modulate neural function and behavior across the lifespan, and the lack of detailed mechanistic research in a broad range of taxa. Addressing these gaps would enable a better understanding of the evolution of this conserved and widely deployed tool kit pathway.
Introduction
Nutritional state is a universal factor that alters behavioral expression in animals including insects (1, 2). Adult insects must accrue sufficient energy to support things like somatic maintenance, mate search, egg development, nest construction, oviposition, and parental care (3–7). To do this, individuals combine complex information about their own nutritional state with environmental information like resource and mate availability (8) in order to make prudent decisions about energy acquisition and use.
Insulin/insulin-like growth factor signaling (IIS) is one of the most well-recognized pathways that contributes to the organization and expression of energy-sensitive behaviors (1, 9). This pathway, particularly its satiety signaling function, is conserved across vertebrates and invertebrates (9). However, presumably because of the diverse connections between nutritional state and behavioral expression, IIS has been co-opted to regulate phenotypes like egg production, reproductive tactics, and courtship behavior across taxa (10–12). It thus offers fertile ground for studies that investigate the physiological links between nutritional state and nervous system processes, and how these relationships evolve.
In this mini review, we explore the variety of roles for IIS in regulating behavioral expression in adult insects. One of our major goals is to describe links between IIS activity and the modulation of nervous system function, highlighting knowledge gaps in these areas. To do so, we use known mechanistic examples from Drosophila melanogaster (13, 14), and draw parallels and distinctions with other species where possible. To emphasize the expansion and diversification of IIS over evolutionary time, we focus on behaviors ranging from most conserved (e.g., feeding behaviors) to derived (e.g., social behaviors and polyphenisms).
Insulin/insulin-like growth factor signaling pathway fundamentals
IIS activity is dynamic throughout life. Here we focus on how IIS modulates adult behaviors, but we include some developmental processes that give rise to adult polyphenisms. IIS involves the action of insulin-like peptides (ILPs), which are produced in the brain and peripheral tissues and operate either as circulating hormones or neuromodulators (15–17). These peptides fall into three categories based on their shared homology with their vertebrate counterparts: insulin-like, insulin growth factor-like (IGF), or relaxin-like (18). Most ILPs are insulin-like (18, 19). Studies in some taxa differentiate insulin-like, IGF-like, and relaxin-like peptides, but many others refer to all types collectively as ILPs (18, 20, 21). In keeping with the convention set by D. melanogaster, we will generally refer to ILPs but note IGF and relaxin-like peptides where possible.
Insulin-like and IGF-like peptides activate the tyrosine kinase insulin receptor (InR) causing insulin receptor substrate (IRS) phosphorylation and downstream activation or inhibition of effectors via two major pathways, the phosphoinositide 3-kinase/protein kinase b (PI3K/Akt) pathway, which is associated primarily with cellular energy metabolism (22, 23), and the mitogen-activated protein kinase (MAPK) pathway, which is involved in cell and organismal growth, typically during development, via ecdysone signaling (4, 20). Notably, these pathways can have overlapping effects that are difficult to differentiate (18, 24–26). With PI3K/Akt, IRS binds to PI3K, activating Akt, which phosphorylates and inhibits a class O of forkhead box transcription factor (FOXO) and its downstream targets (27, 28), including developmental growth and differentiation regulators in conserved pathways such as hedgehog signaling (29–31, see 32 for an example of FOXO activity in adults). Akt can also activate the cAMP-response element binding protein (CREB, involved in memory formation) and inactivate glycogen synthase kinase 3 (GSK3), promoting glycogen synthesis and energy storage (23, 33–35). Alternatively, IRS can interact with growth factor receptor bound protein-2 (Grb2), ultimately initiating MAPK signaling (23).
While the identity of insulin-like peptides and IGFs are well-established in a variety of insect species, less is known about relaxin-like peptides outside of D. melanogaster (36). In D. melanogaster, relaxins activate G-protein coupled receptors (GPCRs), specifically leucine-rich repeat-containing GPCRs 3 and 4 (Lgr3 and Lgr4) during metamorphosis and oviposition, respectively (14, 18, 37–40). Recent studies are beginning to investigate relaxin-like peptide GPCRs in other taxa (24, 37, 41, 42).
IIS activity is often manipulated and/or measured using changes in ILP, InR, or IRS mRNA or protein levels. FOXO mRNA levels are also commonly used to infer PI3K/Akt pathway activity (43); other downstream effects of InR and the effects of relaxin-like peptides are less studied. To understand the role of IIS in coordinating nutritional state and behavior, it is necessary to know the location of ILP production and action in the periphery and brain. These are best understood in D. melanogaster (reviewed in 15), although characteristics are likely to be similar in other species (9, 44). In D. melanogaster, some ILPs are released by insulin-producing neurosecretory cells (IPCs) in the brain, where they act locally (45). IPCs respond directly and indirectly to peripheral signals including fat body produced ILPs, hemolymph glucose content, adipokinetic hormone, and other peptides and biogenic amines that can also act independently of nutritional state (17, 18, 37, 46, Figure 1). IPCs project to the heart, corpora cardiaca, and the midgut, stimulating ILP release from those tissues (8, 18, 50). Peripheral ILPs are also produced by ovarian follicle cells and regions of the gut. Some of these ILPs act locally, and others circulate (15, 51, 52). Notably, ILP production and inhibition are impacted by circulating hormones including juvenile hormone (JH) and ecdysteroids, and in turn, ILPs can affect the synthesis of these hormones (38, 47, 53–56). Many details regarding the coordination of ILP production and release among tissues, and the interaction of IIS with other behaviorally relevant pathways, are still under study.
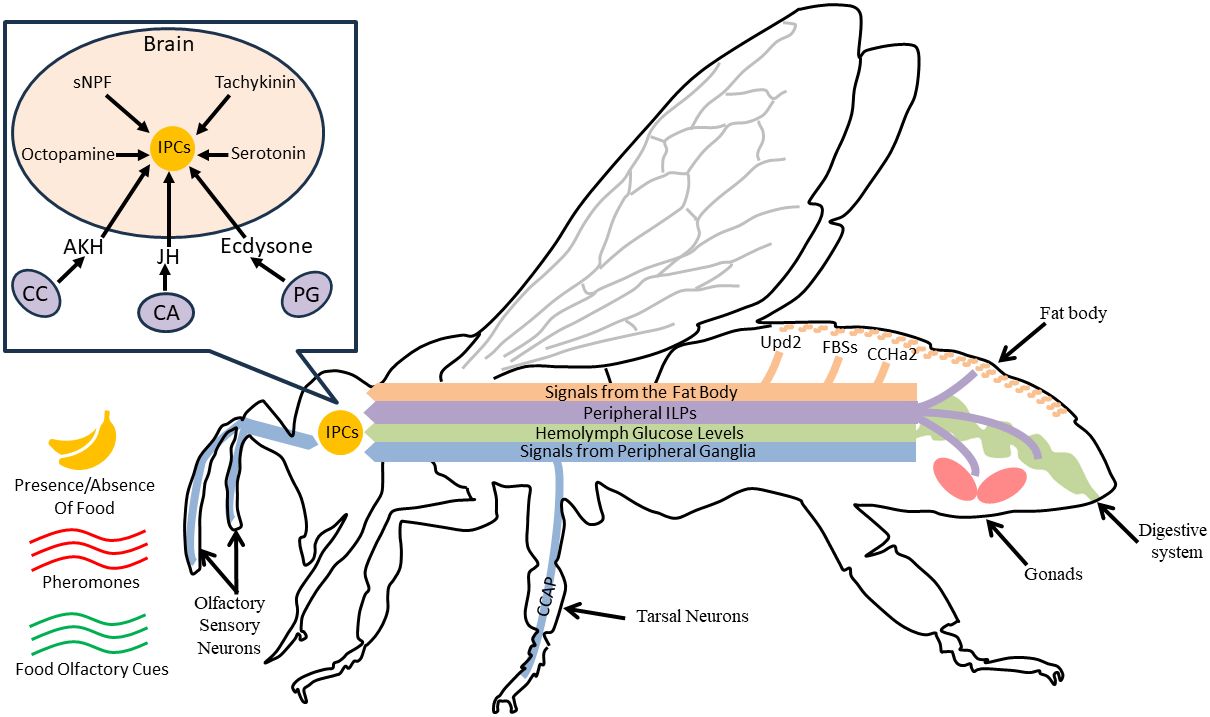
Figure 1 For a hypothetical adult insect, we show various IIS mechanisms that coordinate activity in the brain and periphery to give rise to behavioral variation. In the head (box insert), IPCs release locally acting ILPs to modulate nervous system processes like sensory responses and locomotor activity. Nearby glands such as the corpora cardiaca (CC), corpora allata (CA), and the prothoracic gland (PG) produce hormones that can alter ILP production and release from IPCs (18, 47). IPCs are activated by peptides (sNPF, tachykinin) or biogenic amines (octopamine, serotonin) released by other neurons in the brain in response to neural or peptide signaling from peripheral sensory systems (navy blue lines, (48, 49), or peripheral signals like hemolymph glucose levels (green line, 46); these are modulated by social and nutritional cues and nutritional status (indicated by navy blue lines, 18). The fat body also releases several types of uncharacterized fat body signals (FBSs), as well as Upd2 and CCHa2 in response to changes in available nutrients, and these ultimately stimulate IPC ILP production through unknown mechanisms (17). The fat body, and other tissues including the midgut and gonads (e.g., ovaries), also produce ILPs, shown in purple (15). These ILPs, some of which are also produced by the IPCs, can act on the brain as well as ganglia or other peripheral tissues (15). Notably, although we have depicted all relationships with a directional arrow, various signals can activate or inhibit IPCs depending on environmental context and the specific taxa.
IIS regulation of feeding behavior
Perhaps the most universal function of IIS is in satiety signaling, telling an individual they do not need food (46). IIS activity has been implicated in feeding behaviors in diverse species, including fruit flies (D. melanogaster), locusts (Schistocerca gregaria), and mantids (Tenodera sinensis9, 44, 57). ILPs produced in brain IPCs or peripherally, e.g., in the fat body, alter sensitivity to food cues or food acquisition behaviors through changes in sensory physiology, activity levels, nutrient preferences, and learning and memory processes (1, 9, 58–61). For example, in D. melanogaster, elevated circulating ILPs following food intake inhibit short neuropeptide F (sNPF) expression in the olfactory sensory neurons, reducing sensitivity to food odors and inhibiting food searching behavior (45). Similarly, starvation, and decreased production of ILPs by IPCs, induces hyperactive food search (62), while locomotion inhibits IPC ILP production, increasing sensitivity to food cues (63).
Data from other insects indicate that at least some IIS-mediated satiety mechanisms are generally conserved, although locations of ILP production, signaling relationships, and neural mechanisms giving rise to behavioral variation may differ. For example, in the desert locust Schistocerca gregaria, IIS via both MAPK and PI3K/Akt pathways increases sNPF expression in the optic lobe, leading to decreased feeding (57). Parasite infection at the time of a bloodmeal increases mosquito (Anopheles stephensi) olfactory sensitivity to hosts due to changes in midgut ILP mRNA expression (59). Female mosquitoes alternate between nectar and blood feeding as their nutritional needs change with egg production. In the mosquito Aedes aegypti, nutrient-specific hormone dynamics stimulate different sets of ILPs in the brain and peripheral tissues to synchronize metabolism and reproductive stage (47), as well as activate digestion of blood meals along with the target of rapamycin (TOR) pathway (64). In Western honey bees (Apis mellifera), IIS in the fat body modulates neural sensory systems via unknown mechanisms to cause a preference for lipid and protein-rich pollen over nectar in foragers (65). In this species, increased expression of brain InR mRNA is also correlated with spatiotemporal memory formation and anticipation of encountering known food resources (66), possibly through the MAPK pathway (67). Similarly, in D. melanogaster, IIS has been linked to cAMP-dependent memory formation and aversive learning in both adults and larvae (34, 68–71). In mantids, injection of mammalian insulin causes decreased movement, but rather than decreasing foraging activity, this causes a shift from active prey stalking to a more sedentary ambush strategy (44). It is largely unknown how ILPs modulate nervous system processes in these diverse species and contexts, but clearly IIS is involved in many types of behaviors and preferences related to foraging and diet choice.
IIS regulation of courtship, mate choice, and oviposition
In insects, IIS reflects nutrient availability for reproduction, and as such it affects vitellogenesis and the number of eggs a female produces (43, 72). However, because reproductive individuals perform suites of behaviors required to successfully mate and lay eggs, IIS is also more broadly involved in courtship and mate choice (49, 73). For example, in D. melanogaster males, tarsal contact with pheromones from male competitors or heterospecific females leads to the release of an ILP from the IPCs, inhibiting the P1 neurons that promote courtship (49). Relaxin-like ILPs and associated downstream mechanisms in male glial cells and abdominal ganglion neurons are also required for mating, sexual receptivity, and mate attraction in D. melanogaster (74–76). Similarly, in females, IIS in olfactory sensory neurons responsive to male sex pheromones mediates a starvation-induced decrease in sexual receptivity (12, 48). IIS seems to incorporate individual mating history in the context of mating decisions: inhibiting ILP production in unmated females increases sexual receptivity (77) while following a mating event, decreased InR expression or ILP production reduces willingness to remate (78).
Peripheral IIS activity in females also alters attractiveness to males through cuticular hydrocarbon (CHC) profiles. In D. melanogaster, increased ovary IIS with decreased fat body IIS alters CHC production in fat body oenocytes (73) and increases mate attraction (52, 79). Because diet and nutrition influence IIS and CHC production, CHCs are honest signals of female quality (80). CHCs can indicate female mating status, fertility, and mating compatibility in many other insect species, suggesting this connection between IIS and mating cues may be broadly conserved in species from hymenopterans to coleopterans (81–83).
IIS mediates maternal offspring provisioning and oviposition site selection, combining the classic role of IIS in feeding behavior with its more elaborated reproductive functions. D. melanogaster females use gustatory cues to choose oviposition sites based on substrate sucrose concentrations (40). Interestingly, these decisions are not mediated by IPC-produced ILPs, but rather via relaxin-like ILP7 activity in neurons in the thoracic-abdominal ganglia (15), which have projections to the sub-esophageal ganglia and the female reproductive tract (40). The other D. melanogaster relaxin peptide, ILP8, is expressed in follicle cells and binds to receptors on abdominal ganglia cells, enabling the oviduct muscle to perform the needed oviposition movement (84). Ovary IIS may also modulate provisioning behaviors in social species where sterile workers feed offspring: in honey bees, workers with larger ovaries show a preference for pollen (used to make larval food) over nectar; genetic studies assessing variation in pollen preference have implicated the IIS pathway (85, 86).
IIS regulation of adult polyphenisms
Eusocial insect castes
IIS activity plays a critical developmental role across insects, affecting both juvenile and adult phenotypes (4). Here we highlight the developmental role of IIS in the context of adult polyphenisms, which are well-studied examples of nutrition-mediated behavioral variation in adult insects. For example, across independent evolutionary origins of eusociality, there is a common role for nutrition and IIS in caste determination, although the pathway is implemented differently among taxa (87–91). In honey bees, where colonies contain a single reproductive queen and thousands of sterile female worker bees, the queen larval diet increases IIS and leads to a spike in juvenile hormone (JH) production necessary for queen development (92–94). Later in development, queen IIS drops to worker-like levels (95), suggesting a transient increase in IIS/JH in queens gives rise to persistent effects at multiple levels of biological organization (93). While JH is produced in the corpora allata, it is unclear which tissues are involved in producing the upstream IIS signal and responding to IIS/JH (96).
IIS/JH signaling during larval stages could directly impact the development of the brain and/or other tissues that communicate with the brain throughout adulthood. In honey bees, IRS expression during development is responsible for differentiating queen and worker ovaries, but additional variation in IRS expression throughout adulthood also underpins behavior-relevant variation in ovary size among workers (85, 86, 97). For example, among workers, there is evidence that ovary size modulates the response to social pheromones (98). Enlarged ovaries are associated with increased octopamine signaling in the brain (98); octopamine activates the IPCs and thus could modulate olfactory sensitivity through IIS (8). A similar mechanism appears in the clonal raider ant Ooceraea biroi, where adults can switch between ovary activated (reproductive) and ovary suppressed (brood care) phases. Larval pheromones suppress reproduction and promote brood care by inhibiting ILP expression in adult IPCs (89).
While it is unknown whether or how IIS/JH signaling impacts brain development, differences in IIS expression continue into adulthood in honey bees; queens have decreased brain IIS compared to workers (87). Other social species also show caste differences in brain IIS, but patterns vary. Reproductives have higher brain IIS compared to workers in a wasp (Polistes candensis99), termite (Cavitermes tuberosus100), earwig (Forficula Auricularia101), and many ant species (20, 89, 102–106). IIS could be linked to different, specific functional outcomes in these diverse social species, for example, species-specific trade-offs among egg production, queen behavior, and lifespan (87). Resolving these relationships requires more detailed work, including assessment of the specific mechanisms activated by IIS. For example, in reproductives of the ant Harpegnathos saltator, brain produced ILPs activate MAPK in the fat body and ovaries, but not the PI3K/Akt pathway (20), while ovarian activation of PI3K/Akt signaling occurs in other ant species (102, 103). These different responses to ILPs in the ovaries could mediate divergent phenotypic outcomes.
The unresolved complexities in IIS continue when looking among members of the worker caste in social insects. Honey bee workers show dietary and physiological changes corresponding to adult age-related behavioral shifts (“age polyethism”), including a massive loss of lipid stores in the fat body associated with the transition from nursing to foraging behaviors (107). As the fat body shrinks during aging, increased ILP production leads to increased JH and behavioral changes (97, 108–111). However, while older workers have higher whole-body IIS activity compared to younger workers, they have higher brain IIS (112) but lower fat body IIS (113). IIS activity differences could also correspond to tissue-specific divergence in downstream pathways. For example, a brain biomarker for honey bee foraging behavior is a extracellular signal-regulated kinase (ERK), a member of the MAPK pathway (114), which has been associated with learning and memory processes in the context of food acquisition (67). In contrast, in the fat body, IRS (the PI3K/Akt pathway) is activated in nurse bees who consume an amino acid rich diet compared to foragers; decreased IRS/IIS signaling results in precocious foraging (113). Thus, two different IIS downstream pathways in two different tissues both contribute to the same phenotypic outcome. Other honey bee species, the wasp Polistes metricus, and the ant Temnothorax longispinosus show similar age- and tissue-related patterns (88, 115–117), while the bumble bee Bombus terrestris, stingless bee Tetragonisca angustula, and ant Solenopsis invicta show the opposite, at least in terms of age patterns (118–120). The mechanistic implications of these complexities remain unclear.
Notably, many studies in eusocial insects use gene expression data exclusively to implicate IIS in caste differences. These data do not necessarily reflect circulating ILP levels or the quantity of stored ILPs that could be released to activate IIS. More work examining protein interactions and phosphorylation downstream of ILP receptor binding is necessary to validate and interpret the role of IIS in the context of behavioral differences between queens and workers or among workers.
Wing length and weapon size polyphenisms
Juvenile nutrition and IIS activity are involved in the development of discrete adult polyphenisms in wing length in some hemipterans and weapon size in some coleopterans. When food quality is low, some hemipterans produce long-winged morphs that disperse at a cost to fecundity (121). As hemimetabolous insects, the switch between morphs can happen until the last nymphal instar, allowing for rapid response to environmental conditions (121). IIS patterns and wing morph expression are similar across several species: in soapberry bugs (Jadera haematoloma), linden bugs (Pyrrhocoris apterus), and pea aphids (Acyrthosiphon pisum), high quality food or low population densities lead to elevated IIS activity (inferred by pathway manipulation and gene expression data) and the development of wingless morphs (30, 51, 122, 123). However, in the brown planthopper (Nilaparvata lugens), this pattern is generally reversed (31, 124). Downstream mechanisms could include GSK3, which is associated with wing deformities (125, 126). Wing tissues are particularly sensitive to ILPs and variation in IIS does not affect allometry or growth in other tissues (121). This tissue specificity extends beyond species with conspicuous polyphenisms, e.g., D. melanogaster and the tobacco hornworm Manduca sexta. Thus, developmental nutrition may have other subtle effects on adult flight, dispersal phenotypes, and reproductive capacity across diverse species (19, 127).
In Scarabaeidae beetles, exaggerated male weapons like horns are common. Males with high-quality larval nutrition have large weapons and engage in male-male fighting over mates, while males with poor nutrition have small or no weapons and rely on sneaker tactics (10). As in the wing example, other tissues are unaffected by variation in ILPs. When nutrition is high-quality, ILPs drive weapon tissue proliferation through InR activation (128, 129). Without these signals, the transcription factor FOXO stops cell proliferation and the development of weapon structures (130). There is some interesting variation in how IIS acts in different beetle species. In the rhinoceros beetle Trypoxylus dichotomus, InR knockdown results in greatly diminished horns (130). In contrast, InR knockdown has no effect on horn growth in the dung beetle Onthophagus nigriventris, but FOXO knockdown suppresses growth in both the horns and genitalia (10, 29, 128, 131). IIS has also been implicated in more subtle variation in flight and fighting capabilities in bark beetles (Dendroctonus ponderosae) and crickets (Gryllus assimilis and Gryllus firmus5, 132–134), suggesting it may play a more generalized role in competition-related behavior and polymorphisms.
In the dimorphic horned beetle examples, developmental IIS leads to differences in adult morphology and behavior, but it is unclear whether IIS exerts organizational effects on the brain during development, or continuously regulates adult behavioral differences. For example, variation in doublesex expression and serotonin levels in the adult brain predict differences in aggression across dimorphic males (135, 136). Doublesex is a target of IIS in developing horn tissues and serotonin impacts the body size threshold that distinguishes the horn morphs (29, 137), but it is unknown whether IIS regulates either mechanism in the adult brain. Similarly, in the pea aphid, differential ILP expression between nymphal winged and wingless individuals occurs in the thorax, but ILPs are also differentially expressed in the brain and thorax during adulthood, suggesting further phenotypic impacts (51, 138). Understanding the relationships in activity of IIS across the life stages could lead to new insights about the evolution and regulation of phenotypic plasticity. IIS appears to integrate environmental cues over the lifetime to modulate behavioral expression, and as such, it could serve as a mechanism that impacts the duration of environmental effects (139, 140).
Discussion
IIS’s role in communicating nutritional state and regulating feeding behaviors has been elaborated over evolutionary time to coordinate reproductive physiology, courtship and mating behaviors, maternal provisioning behaviors, social insect caste differentiation, and the development and adult regulation of dimorphic dispersal and reproductive phenotypes.
Food choice and food-related locomotion are broadly associated with IIS, but there is substantial species-level variation in food cues, nutrients and preferences, locomotion patterns, and the ecological contexts that regulate foraging behaviors. Future studies could investigate the mechanistic bases of this species-level variation, in terms of how both internal state and external information modulate IIS and cause behavioral change. Insects present some particularly interesting and economically relevant contexts where IIS is essential to feeding behavior, including grasshopper (Oedaleus asiaticus) plague activity resulting from sub-optimal diets (141) or changes in feeding behavior due to crowding in armyworms (Mythimna separata142). Examining IIS activation, including ILP production and modes of action in the brain across diverse taxa is critical to understanding the evolution of IIS and may also highlight new tools for pest control.
Substantial gaps remain in understanding the role of IIS in coordinating activities between the brain and peripheral tissues. These mechanisms are diverse and context dependent even in well studied species like D. melanogaster (15). However, certain emergent patterns may be conserved. For example, in D. melanogaster, different ILPs are responsible for within and cross-tissue signaling. ILP number varies greatly among taxa (143), possibly reflecting the diversity of contexts requiring IIS regulation, or the tissues involved. Most species have 1 or 2 InRs that activate tissue-specific downstream targets (144) but the mechanisms that allow specificity in downstream interactions, including how limited numbers of InR receptors give rise to diverse effects from numerous peptides, are still mostly unknown (55). While the most-studied model species D. melanogaster has only one InR, many other species have two, and Blattodea three, which can lead to novel relationships and interactions that should be studied further (144). For example, in the brown planthopper, InR2 directly inhibits InR1 during wing morph development, while the third Blattodea receptor is hypothesized to have a role in social termite evolution (124, 144).
Identifying the downstream pathways activated specifically by IIS is challenging as many of them can be affected by several other signaling pathways (20, 23, 145–147). This is especially problematic in non-model organisms where genetic tools and experimental approaches to manipulate ILP abundance are not well-developed. It is also important to consider the possibility that some peptides identified as insulin-like may belong to other peptide classes (e.g., IGF-like), which could suggest divergent downstream effects (20, 38, 105). Future studies could address these complexities by at least elaborating on the details of tissue-specific IIS and confirming the involvement of IIS using direct measures of ILP abundance and scaffolding or phosphorylation state of downstream targets (24).
Another compelling pattern that emerges from eusocial caste differentiation is that IIS is used to integrate cues associated with seasonal timing and other abiotic factors. For example, in the social paper wasp Polistes metricus, late season larvae become reproductive gynes that will overwinter and establish new nests the following year. As such, larvae are fed more and have activated IIS (148). The ant Pogonomurmex rugosus can only produce new queens after the original queen has hibernated, a transition caused by environmental signals like temperature that induce numerous physiological and behavioral changes in queens, including decreased metabolism and feeding. Hibernated queens have increased ILP expression, which increases the amount of vitellogenin deposited in eggs leading to new queen production (149). These provide additional examples of the ways in which IIS has been co-opted in novel contexts associated with nutrition variation.
Despite broad connections between IIS and behavior, mechanistic work outside of D. melanogaster remains limited. More diverse functional information could elucidate the conserved and divergent aspects of IIS among species and contexts, for example, in terms of where ILPs originate in the body (59), or how the different IIS components interact with each other (121). Our current model systems have highly derived phenotypes that may hinder attempts to form generalizable hypotheses. Broadening work in other taxa will also help explain why IIS is inconsistently used to regulate the same phenotypes across species (89). For example, some fig wasp species have winged and wingless males (150) that differ in aggression and weapon size (151). Although these phenotypes resemble the bugs and beetles discussed above, no link has been made to IIS or nutrition. Is this an independent evolutionary event with repeated co-option of the IIS pathway? Comparative investigations of the evolutionary origins of phenotypes like polyphenisms could help determine whether IIS is a “toolkit pathway” that has been repeatedly deployed over evolutionary time to give rise to similar phenotypes (120, 152). Its ubiquity among species and behaviors suggests this could be the case.
Author contributions
AW: Conceptualization, Writing – original draft, Writing – review & editing. CR: Conceptualization, Funding acquisition, Supervision, Writing – original draft, Writing – review & editing.
Funding
The author(s) declare financial support was received for the research, authorship, and/or publication of this article. This work was supported by a National Science Foundation award to CR (IOS-2049501) and an NSF-GRFP (AW), as well as the National Institute of Food and Agriculture Research, US Department of Agriculture Hatch Program (1012993).
Acknowledgments
We adopted protein and gene names from the Flybase and Uniprot databases, and where appropriate, followed the Drosophila melanogaster protein and gene naming conventions. We thank to Dr. S.R. Palli, Dr. N.M. Teets, Dr. D.F. Westneat, and two reviewers for comments that improved this manuscript.
Conflict of interest
The authors declare that the research was conducted in the absence of any commercial or financial relationships that could be construed as a potential conflict of interest.
Publisher’s note
All claims expressed in this article are solely those of the authors and do not necessarily represent those of their affiliated organizations, or those of the publisher, the editors and the reviewers. Any product that may be evaluated in this article, or claim that may be made by its manufacturer, is not guaranteed or endorsed by the publisher.
References
1. Kim SM, Su C-Y, Wang JW. Neuromodulation of innate behaviors in drosophila. Annu Rev Neurosci. (2017) 40:327–48. doi: 10.1146/annurev-neuro-072116-031558
2. Leulier F, MacNeil LT, Lee W, Rawls JF, Cani PD, Schwarzer M, et al. Integrative physiology: at the crossroads of nutrition, microbiota, animal physiology, and human health. Cell Metab. (2017) 25:522–34. doi: 10.1016/j.cmet.2017.02.001
3. Hatle JD, Waskey T, Juliano SA. Plasticity of grasshopper vitellogenin production in response to diet is primarily a result of changes in fat body mass. J Comp Physiol B. (2006) 176:27–34. doi: 10.1007/s00360-005-0028-9
4. Andersen DS, Colombani J, Léopold P. Coordination of organ growth: Principles and outstanding questions from the world of insects. Trends Cell Biol. (2013) 23:336–44. doi: 10.1016/j.tcb.2013.03.005
5. Bertram SM, Yaremchuk DD, Reifer ML, Villarreal A, Muzzatti MJ, Kolluru GR. Tests of the positive and functional allometry hypotheses for sexually selected traits in the Jamaican field cricket. Behav Processes. (2021) 188:104413. doi: 10.1016/j.beproc.2021.104413
6. Leyria J, Orchard I, Lange AB. The involvement of insulin/ToR signaling pathway in reproductive performance of Rhodnius prolixus. Insect Biochem Mol Biol. (2021) 130:103526. doi: 10.1016/j.ibmb.2021.103526
7. Rico-Guevara A, Hurme KJ. Intrasexually selected weapons. Biol Rev. (2019) 94:60–101. doi: 10.1111/brv.12436
8. Erion R, Sehgal A. Regulation of insect behavior via the insulin-signaling pathway. Front Physiol. (2013) 4:353. doi: 10.3389/fphys.2013.00353
9. Nässel DR. Insulin-producing cells and their regulation in physiology and behavior of Drosophila 1. Can J Zoology. (2012) 90:476–88. doi: 10.1139/z2012-009
10. Emlen DJ, Szafran Q, Corley LS, Dworkin I. Insulin signaling and limb-patterning: Candidate pathways for the origin and evolutionary diversification of beetle ‘horns.’. Heredity. (2006) 97:179–91. doi: 10.1038/sj.hdy.6800868
11. Laturney M, Billeter J-C. Neurogenetics of female reproductive behaviors in drosophila melanogaster. Adv Genet. (2014) 85:1–108. doi: 10.1016/B978-0-12-800271-1.00001-9
12. Lebreton S, Carlsson MA, Witzgall P. Insulin signaling in the peripheral and central nervous system regulates female sexual receptivity during starvation in drosophila. Front Physiol. (2017) 8:685. doi: 10.3389/fphys.2017.00685
13. Lin S, Senapati B, Tsao C-H. Neural basis of hunger-driven behaviour in Drosophila. Open Biology. (2019) 9(3):180259.
14. Semaniuk U, Piskovatska V, Strilbytska O, Strutynska T, Burdyliuk N, Vaiserman A, et al. Drosophila insulin-like peptides: From expression to functions – a review. Entomologia Experiment. Applicata. (2021) 169:195–208. doi: 10.1111/eea.12981
15. Nässel DR, Kubrak OI, Liu Y, Luo J, Lushchak OV. Factors that regulate insulin producing cells and their output in Drosophila. Front Physiol. (2013) 4:252. doi: 10.3389/fphys.2013.00252
16. Schoofs L, De Loof A, Van Hiel MB. Neuropeptides as regulators of behavior in insects. Annu Rev Entomol. (2017) 62:35–52. doi: 10.1146/annurev-ento-031616-035500
17. Li S, Yu X, Feng Q. Fat body biology in the last decade. Annu Rev Entomol. (2019) 64:315–33. doi: 10.1146/annurev-ento-011118-112007
18. Chowański S, Walkowiak-Nowicka K, Winkiel M, Marciniak P, Urbański A, Pacholska-Bogalska J. Insulin-like peptides and cross-talk with other factors in the regulation of insect metabolism. Front Physiol. (2021) 12:701203. doi: 10.3389/fphys.2021.701203
19. Okamoto N, Yamanaka N. Nutrition-dependent control of insect development by insulin-like peptides. Curr Opin Insect Sci. (2015) 11:21–30. doi: 10.1016/j.cois.2015.08.001
20. Yan H, Opachaloemphan C, Carmona-Aldana F, Mancini G, Mlejnek J, Descostes N, et al. Insulin signaling in the long-lived reproductive caste of ants. Science. (2022) 377:1092–9. doi: 10.1126/science.1151842
21. Veenstra JA. Differential expression of some termite neuropeptides and insulin/IGF-related hormones and their plausible functions in growth, reproduction and caste determination. PeerJ. (2023) 11:e15259. doi: 10.7717/peerj.15259
22. Brown MR, Clark KD, Gulia M, Zhao Z, Garczynski SF, Crim JW, et al. An insulin-like peptide regulates egg maturation and metabolism in the mosquito Aedes aEgypti. Proc Natl Acad Sci. (2008) 105:5716–21. doi: 10.1073/pnas.0800478105
23. Defferrari MS, Da Silva SR, Orchard I, Lange AB. A rhodnius prolixus insulin receptor and its conserved intracellular signaling pathway and regulation of metabolism. Front Endocrinol. (2018) 9:745. doi: 10.3389/fendo.2018.00745
24. Wen Z, Gulia M, Clark KD, Dhara A, Crim JW, Strand MR, et al. Two insulin-like peptide family members from the mosquito Aedes aEgypti exhibit differential biological and receptor binding activities. Mol Cell Endocrinol. (2010) 328:47–55. doi: 10.1016/j.mce.2010.07.003
25. Han B, Zhang T, Feng Y, Liu X, Zhang L, Chen H, et al. Two insulin receptors coordinate oogenesis and oviposition via two pathways in the green lacewing, Chrysopa pallens. J Insect Physiol. (2020) 123:104049. doi: 10.1016/j.jinsphys.2020.104049
26. Wang Z, Tan D, Wang F, Guo S, Liu J, Cuthbertson AGS, et al. Insulin peptides and their receptors regulate ovarian development and oviposition behavior in Diaphorina citri. Insect Sci. (2022) 30(1):95–108. doi: 10.1111/1744-7917.13048
27. Nijhout HF, Callier V. A new mathematical approach for qualitative modeling of the insulin-TOR-MAPK network. Front Physiol. (2013) 4:245. doi: 10.3389/fphys.2013.00245
28. Walkowiak-Nowicka K, Chowański S, Urbański A, Marciniak P. Insects as a new complex model in hormonal basis of obesity. Int J Mol Sci. (2021) 22:11066. doi: 10.3390/ijms222011066
29. Casasa S, Moczek AP. Insulin signalling’s role in mediating tissue-specific nutritional plasticity and robustness in the horn-polyphenic beetle Onthophagus taurus. Proc R Soc B: Biol Sci. (2018) 285:20181631. doi: 10.1098/rspb.2018.1631
30. Grantham ME, Shingleton AW, Dudley E, Brisson JA. Expression profiling of winged- and wingless-destined pea aphid embryos implicates insulin/insulin growth factor signaling in morph differences. Evol Dev. (2020) 22:257–68. doi: 10.1111/ede.12326
31. Zhang J-L, Fu S-J, Chen S-J, Chen H-H, Liu Y-L, et al. Vestigial mediates the effect of insulin signaling pathway on wing-morph switching in planthoppers. PLOS Genetics. (2021) 17(2):e1009312.
32. Al Baki M, Jung JK, Kim Y. Regulation of hemolymph trehalose titers by insulin signaling in the legume pod borer, Maruca vitrata (Lepidoptera: Crambidae). Peptides. (2018) 106:28–36. doi: 10.1016/j.peptides.2018.06.006
33. Lin J-L, Lin P-L, Gu S-H. Phosphorylation of glycogen synthase kinase-3β in relation to diapause processing in the silkworm, Bombyx mori. J Insect Physiol. (2009) 55:593–8. doi: 10.1016/j.jinsphys.2009.03.007
34. Nakai J, Chikamoto N, Fujimoto K, Totani Y, Hatakeyama D, Dyakonova VE, et al. Insulin and memory in invertebrates. Front Behav Neurosci. (2022) 16:882932. doi: 10.3389/fnbeh.2022.882932
35. Pan X, Pei Y, Zhang C, Huang Y, Chen L, Wei L, et al. Effect of insulin receptor on juvenile hormone signal and fecundity in spodoptera litura (F.). Insects. (2022) 13:701. doi: 10.1128/MCB.24.11.4909-4919.2004
36. Veenstra JA. Arthropod IGF, relaxin and gonadulin, putative orthologs of Drosophila insulin-like peptides 6, 7 and 8, likely originated from an ancient gene triplication. PeerJ. (2020) 8:e9534. doi: 10.7717/peerj.9534
37. Nässel DR, Broeck JV. Insulin/IGF signaling in Drosophila and other insects: Factors that regulate production, release and post-release action of the insulin-like peptides. Cell Mol Life Sci. (2016) 73:271–90. doi: 10.1093/beheco/arz192
38. Gontijo AM, Garelli A. The biology and evolution of the Dilp8-Lgr3 pathway: A relaxin-like pathway coupling tissue growth and developmental timing control. Mech Dev. (2018) 154:44–50. doi: 10.1016/j.mod.2018.04.005
39. Heredia F, Volonté Y, Pereirinha J, Fernandez-Acosta M, Casimiro AP, Belém CG, et al. The steroid-hormone ecdysone coordinates parallel pupariation neuromotor and morphogenetic subprograms via epidermis-to-neuron Dilp8-Lgr3 signal induction. Nat Commun. (2021) 12:3328. doi: 10.1038/s41467-021-23218-5
40. Yang C, Belawat P, Hafen E, Jan LY, Jan Y-N. Drosophila egg-laying site selection as a system to study simple decision-making processes. Science. (2008) 319(5870):1679–83.
41. Gao H, Li Y, Zhang H, Wang S, Feng F, Tang J, et al. Comparative study of neuropeptide signaling systems in Hemiptera. Insect Sci. (2023) 30:705–24. doi: 10.1111/1744-7917.13120
42. Kazemi Alamouti M, Majdi M, Talebi R, Dastranj M, Bandani A, Hossini Salekdeh G, et al. Transcriptome wide identification of neuropeptides and G protein-coupled receptors (GPCRs) in Sunn pest, Eurygaster integriceps Puton. Gene. (2024) 893:147911. doi: 10.1016/j.gene.2023.147911
43. Badisco L, Van Wielendaele P, Vanden Broeck J. Eat to reproduce: A key role for the insulin signaling pathway in adult insects. Front Physiol. (2013) 4:202. doi: 10.3389/fphys.2013.00202
44. Bertsch DJ, Martin JP, Svenson GJ, Ritzmann RE. Predatory behavior changes with satiety or increased insulin levels in the praying mantis (Tenodera sinensis). J Exp Biol. (2019) 222(11):197673. doi: 10.1242/jeb.197673
45. Root CM, Ko KI, Jafari A, Wang JW. Presynaptic facilitation by neuropeptide signaling mediates odor-driven food search. Cell. (2011) 145:133–44. doi: 10.1016/j.cell.2011.02.008
46. Qi W, Wang G, Wang L. A novel satiety sensor detects circulating glucose and suppresses food consumption via insulin-producing cells in Drosophila. Cell Res. (2021) 31:580–8. doi: 10.1038/s41422-020-00449-7
47. Ling L, Raikhel AS. Cross-talk of insulin-like peptides, juvenile hormone, and 20-hydroxyecdysone in regulation of metabolism in the mosquito Aedes aEgypti. Proc Natl Acad Sci USA. (2021) 118(6):e2023470118. doi: 10.1073/pnas.2023470118
48. Lebreton S, Trona F, Borrero-Echeverry F, Bilz F, Grabe V, Becher PG, et al. Feeding regulates sex pheromone attraction and courtship in Drosophila females. Sci Rep. (2015) 5:13132. doi: 10.1038/srep13132
49. Zhang L, Guo X, Zhang W. Nutrients and pheromones promote insulin release to inhibit courtship drive. Sci Adv. (2022) 8:eabl6121. doi: 10.1126/sciadv.abl6121
50. Badisco L, Claeys I, Van Hiel M, Clynen E, Huybrechts J, Vandersmissen T, et al. Purification and characterization of an insulin-related peptide in the desert locust, Schistocerca gregaria: Immunolocalization, cDNA cloning, transcript profiling and interaction with neuroparsin. J Mol Endocrinol. (2008) 40:137–50. doi: 10.1677/JME-07-0161
51. Guo S-S, Zhang M, Liu T-X. Insulin-related peptide 5 is involved in regulating embryo development and biochemical composition in pea aphid with wing polyphenism. Front Physiol. (2016) 7:31. doi: 10.3389/fphys.2016.00031
52. Fedina TY, Arbuthnott D, Rundle HD, Promislow DEL, Pletcher SD. Tissue-specific insulin signaling mediates female sexual attractiveness. PloS Genet. (2017) 13:e1006935. doi: 10.1371/journal.pgen.1006935
53. Mirth CK, Tang HY, Makohon-Moore SC, Salhadar S, Gokhale RH, Warner RD, et al. Juvenile hormone regulates body size and perturbs insulin signaling in Drosophila. Proc Natl Acad Sci. (2014) 111:7018–23. doi: 10.1073/pnas.1313058111
54. Roy S, Saha TT, Zou Z, Raikhel AS. Regulatory pathways controlling female insect reproduction. Annu Rev Entomol. (2018) 63:489–511. doi: 10.1146/annurev-ento-020117-043258
55. Kannangara JR, Mirth CK, Warr CG. Regulation of ecdysone production in Drosophila by neuropeptides and peptide hormones. Open Biol. (2021) 11:200373. doi: 10.1098/rsob.200373
56. Leyria J, Benrabaa S, Nouzova M, Noriega FG, Tose LV, Fernandez-Lima F, et al. Crosstalk between nutrition, insulin, juvenile hormone, and ecdysteroid signaling in the classical insect model, rhodnius prolixus. Int J Mol Sci. (2022) 24:7. doi: 10.3390/ijms24010007
57. Dillen S, Chen Z, Vanden Broeck J. Nutrient-dependent control of short neuropeptide F transcript levels via components of the insulin/IGF signaling pathway in the desert locust, Schistocerca gregaria. Insect Biochem Mol Biol. (2016) 68:64–70. doi: 10.1016/j.ibmb.2015.11.007
58. Stafford JW, Lynd KM, Jung AY, Gordon MD. Integration of taste and calorie sensing in drosophila. J Neurosci. (2012) 32:14767–74. doi: 10.1523/JNEUROSCI.1887-12.2012
59. Cator LJ, Pietri JE, Murdock CC, Ohm JR, Lewis EE, Read AF, et al. Immune response and insulin signalling alter mosquito feeding behaviour to enhance malaria transmission potential. Sci Rep. (2015) 5:11947. doi: 10.1038/srep11947
60. Semaniuk UV, Gospodaryov DV, Feden’ko KM, Yurkevych IS, Vaiserman AM, Storey KB, et al. Insulin-like peptides regulate feeding preference and metabolism in drosophila. Front Physiol. (2018) 9:1083. doi: 10.3389/fphys.2018.01083
61. Ganguly A, Dey M, Scott C, Duong V-K, Arun Dahanukar A. Dietary macronutrient imbalances lead to compensatory changes in peripheral taste via independent signaling pathways. J Neurosci. (2021) 41:10222–46. doi: 10.1523/JNEUROSCI.2154-20.2021
62. Yu Y, Huang R, Ye J, Zhang V, Wu C, Cheng G, et al. Regulation of starvation-induced hyperactivity by insulin and glucagon signaling in adult Drosophila. eLife. (2016) 5:e15693. doi: 10.7554/eLife.15693
63. Liessem S, Held M, Bisen RS, Haberkern H, Lacin H, Bockemühl T, et al. Behavioral state-dependent modulation of insulin-producing cells in Drosophila. Curr Biol. (2022) 33(3):449-463.e5. doi: 10.1016/j.cub.2022.12.005
64. Gulia-Nuss M, Robertson AE, Brown MR, Strand MR. Insulin-like peptides and the target of rapamycin pathway coordinately regulate blood digestion and egg maturation in the mosquito aedes aEgypti. PloS One. (2011) 6:e20401. doi: 10.1371/journal.pone.0020401
65. Wang Y, Mutti NS, Ihle KE, Siegel A, Dolezal AG, Kaftanoglu O, et al. Down-regulation of honey bee IRS gene biases behavior toward food rich in protein. PloS Genet. (2010) 6:e1000896. doi: 10.1371/journal.pgen.1000896
66. Naeger NL, Van Nest BN, Johnson JN, Boyd SD, Southey BR, Rodriguez-Zas SL, et al. Neurogenomic signatures of spatiotemporal memories in time-trained forager honey bees. J Exp Biol. (2011) 214:979–87. doi: 10.1242/jeb.053421
67. Qin Q, Wang Z, Tian L, Gan H, Zhang S, Zeng Z. The integrative analysis of microRNA and mRNA expression in Apis mellifera following maze-based visual pattern learning. Insect Sci. (2014) 21:619–36. doi: 10.1111/1744-7917.12065
68. Naganos S, Horiuchi J, Saitoe M. Mutations in the Drosophila insulin receptor substrate, CHICO, impair olfactory associative learning. Neurosci Res. (2012) 73:49–55. doi: 10.1016/j.neures.2012.02.001
69. Chambers DB, Androschuk A, Rosenfelt C, Langer S, Harding M, Bolduc FV. Insulin signaling is acutely required for long-term memory in Drosophila. Front Neural Circuits. (2015) 9:8. doi: 10.3389/fncir.2015.00008
70. Naganos S, Ueno K, Horiuchi J, Saitoe M. Learning defects in Drosophila growth restricted chico mutants are caused by attenuated adenylyl cyclase activity. Mol Brain. (2016) 9:37. doi: 10.1186/s13041-016-0217-3
71. Eschment M, Franz HR, Güllü N, Hölscher LG, Huh K-E, Widmann A. Insulin signaling represents a gating mechanism between different memory phases in Drosophila larvae. PloS Genet. (2020) 16:e1009064. doi: 10.1371/journal.pgen.1009064
72. Smykal V, Raikhel AS. Nutritional control of insect reproduction. Curr Opin Insect Sci. (2015) 11:31–8. doi: 10.1016/j.cois.2015.08.003
73. Lin W, Yeh S, Fan S, Chen L, Yen J, Fu T, et al. Insulin signaling in female Drosophila links diet and sexual attractiveness. FASEB J. (2018) 32:3870–7. doi: 10.1096/fsb2fj201800067r
74. Meissner GW, Luo SD, Dias BG, Texada MJ, Baker BS. Sex-specific regulation of Lgr3 in Drosophila neurons. Proc Natl Acad Sci. (2016) 113(9):E1256-E1265. doi: 10.1073/pnas.1600241113
75. Miao H, Wei Y, Lee SG, Wu Z, Kaur J, Kim WJ. Glia- specific expression of neuropeptide receptor Lgr4 regulates development and adult physiology in Drosophila. J Neurosci Res. (2024) 102(1):e25271.
76. Li H, Luo X, Li N, Liu T, Zhang J. Insulin-like peptide 8 (Ilp8) regulates female fecundity in flies. Front Cell Dev Biol. (2023) 11:1103923. doi: 10.3389/fcell.2023.1103923
77. Sakai T, Watanabe K, Ohashi H, Sato S, Inami S, Shimada N, et al. Insulin-producing cells regulate the sexual receptivity through the painless TRP channel in drosophila virgin females. PloS One. (2014) 9:e88175. doi: 10.1371/journal.pone.0088175
78. Wigby S, Slack C, Grönke S, Martinez P, Calboli FCF, Chapman T, et al. Insulin signalling regulates remating in female Drosophila. Proc R Soc B: Biol Sci. (2011) 278:424–31. doi: 10.1098/rspb.2010.1390
79. Kuo T-H, Fedina TY, Hansen I, Dreisewerd K, Dierick HA, Yew JY, et al. Insulin signaling mediates sexual attractiveness in drosophila. PloS Genet. (2012) 8:e1002684. doi: 10.1371/journal.pgen.1002684
80. Ingleby F. Insect cuticular hydrocarbons as dynamic traits in sexual communication. Insects. (2015) 6:732–42. doi: 10.3390/insects6030732
81. Smith AA, Liebig J. The evolution of cuticular fertility signals in eusocial insects. Curr Opin Insect Sci. (2017) 22:79–84. doi: 10.1016/j.cois.2017.05.017
82. Blomquist GJ, Ginzel MD. Chemical ecology, biochemistry, and molecular biology of insect hydrocarbons. Annu Rev Entomol. (2021) 66:45–60. doi: 10.1146/annurev-ento-031620-071754
83. Yan H, Liebig J. Genetic basis of chemical communication in eusocial insects. Genes Dev. (2021) 35:470–82. doi: 10.1101/gad.346965.120
84. Liao S, Nässel DR. Drosophila insulin-like peptide 8 (DILP8) in ovarian follicle cells regulates ovulation and metabolism. Front Endocrinol. (2020) 11:461. doi: 10.3389/fendo.2020.00461
85. Hunt GJ, Amdam GV, Schlipalius D, Emore C, Sardesai N, Williams CE, et al. Behavioral genomics of honeybee foraging and nest defense. Naturwissenschaften. (2007) 94:247–67. doi: 10.1007/s00114-006-0183-1
86. Ihle KE, Rueppell O, Huang ZY, Wang Y, Fondrk MK, Page RE, et al. Genetic architecture of a hormonal response to gene knockdown in honey bees. J Heredity. (2015) 106:155–65. doi: 10.1093/jhered/esu086
87. Corona M, Velarde RA, Remolina S, Moran-Lauter A, Wang Y, Hughes KA, et al. Vitellogenin, juvenile hormone, insulin signaling, and queen honey bee longevity. Proc Natl Acad Sci. (2007) 104:7128–33. doi: 10.1073/pnas.0701909104
88. Daugherty THF, Toth AL, Robinson GE. Nutrition and division of labor: Effects on foraging and brain gene expression in the paper wasp Polistes metricus. Mol Ecol. (2011) 20:5337–47. doi: 10.1111/j.1365-294X.2011.05344.x
89. Chandra V, Fetter-Pruneda I, Oxley PR, Ritger AL, McKenzie SK, Libbrecht R, et al. Social regulation of insulin signaling and the evolution of eusociality in ants. Science. (2018) 361:398–402. doi: 10.1126/science.aar5723
90. Hattori A, Sugime Y, Sasa C, Miyakawa H, Ishikawa Y, Miyazaki S, et al. Soldier morphogenesis in the damp-wood termite is regulated by the insulin signaling pathway. J Exp Zoology Part B: Mol Dev Evol. (2013) 320:295–306. doi: 10.1002/jez.b.22501
91. Saiki R, Hayashi Y, Toga K, Yaguchi H, Masuoka Y, Suzuki R, et al. Comparison of gene expression profiles among caste differentiations in the termite Reticulitermes speratus. Sci Rep. (2022) 12:11947. doi: 10.1038/s41598-022-15984-z
92. Wheeler DE, Buck N, Evans JD. Expression of insulin pathway genes during the period of caste determination in the honey bee, Apis mellifera. Insect Mol Biol. (2006) 15:597–602. doi: 10.1111/j.1365-2583.2006.00681.x
93. Mutti NS, Dolezal AG, Wolschin F, Mutti JS, Gill KS, Amdam GV. IRS and TOR nutrient-signaling pathways act via juvenile hormone to influence honey bee caste fate. J Exp Biol. (2011) 214:3977–84. doi: 10.1242/jeb.061499
94. Wolschin F, Mutti NS, Amdam GV. Insulin receptor substrate influences female caste development in honeybees. Biol Lett. (2011) 7:112–5. doi: 10.1098/rsbl.2010.0463
95. de Azevedo SV, Hartfelder K. The insulin signaling pathway in honey bee (Apis mellifera) caste development—Differential expression of insulin-like peptides and insulin receptors in queen and worker larvae. J Insect Physiol. (2008) 54:1064–71. doi: 10.1016/j.jinsphys.2008.04.009
96. Rachinsky A, Hartfelder K. Corpora allata activity, a prime regulating element for caste-specific juvenile hormone titre in honey bee larvae (Apis mellifera carnica). J Insect Physiol. (1990) 36:189–94. doi: 10.1016/0022-1910(90)90121-U
97. Amdam GV. Social context, stress, and plasticity of aging: Social context, stress, and plasticity of aging. Aging Cell. (2011) 10:18–27. doi: 10.1111/j.1474-9726.2010.00647.x
98. Galbraith DA, Wang Y, Amdam GV, Page RE, Grozinger CM. Reproductive physiology mediates honey bee (Apis mellifera) worker responses to social cues. Behav Ecol Sociobiol. (2015) 69:1511–8. doi: 10.1007/s00265-015-1963-4
99. Ferreira PG, Patalano S, Chauhan R, Ffrench-Constant R, Gabaldón T, Guigó R, et al. Transcriptome analyses of primitively eusocial wasps reveal novel insights into the evolution of sociality and the origin of alternative phenotypes. Genome Biol. (2013) 14:R20. doi: 10.1186/gb-2013-14-2-r20
100. De Souza Araujo N, Hellemans S, Roisin Y, Fournier D. Transcriptomic profiling of castes and of sexually and parthenogenetically produced reproductive females in the termite Cavitermes tuberosus. Entomologia Experiment. Applicata. (2023) 171:350–60. doi: 10.1111/eea.13285
101. Wu M, Walser J-C, Sun L, Kölliker M. The genetic mechanism of selfishness and altruism in parent-offspring coadaptation. Sci Adv. (2020) 6:eaaw0070. doi: 10.1126/sciadv.aaw0070
102. Okada Y, Miyazaki S, Miyakawa H, Ishikawa A, Tsuji K, Miura T. Ovarian development and insulin-signaling pathways during reproductive differentiation in the queenless ponerine ant Diacamma sp. J Insect Physiol. (2010) 56:288–95. doi: 10.1016/j.jinsphys.2009.10.013
103. Okada Y, Watanabe Y, Tin MMY, Tsuji K, Mikheyev AS. Social dominance alters nutrition-related gene expression immediately: Transcriptomic evidence from a monomorphic queenless ant. Mol Ecol. (2017) 26:2922–38. doi: 10.1111/mec.13989
104. Gospocic J, Glastad KM, Sheng L, Shields EJ, Berger SL, Bonasio R. Kr-h1 maintains distinct caste-specific neurotranscriptomes in response to socially regulated hormones. Cell. (2021) 184:5807–5823.e14. doi: 10.1016/j.cell.2021.10.006
105. Opachaloemphan C, Mancini G, Konstantinides N, Parikh A, Mlejnek J, Yan H, et al. Early behavioral and molecular events leading to caste switching in the ant Harpegnathos. Genes Dev. (2021) 35:410–24. doi: 10.1101/gad.343699.120
106. Feldmeyer B, Gstöttl C, Wallner J, Jongepier E, Séguret A, Grasso DA, et al. Evidence for a conserved queen-worker genetic toolkit across slave-making ants and their ant hosts. Mol Ecol. (2022) 31:4991–5004. doi: 10.1111/mec.16639
107. Münch D, Amdam GV, Wolschin F. Ageing in a eusocial insect: Molecular and physiological characteristics of life span plasticity in the honey bee. Funct Ecol. (2008) 22:407–21. doi: 10.1111/j.1365-2435.2008.01419.x
108. Nilsen K-A, Ihle KE, Frederick K, Fondrk MK, Smedal B, Hartfelder K, et al. Insulin-like peptide genes in honey bee fat body respond differently to manipulation of social behavioral physiology. J Exp Biol. (2011) 214:1488–97. doi: 10.1242/jeb.050393
109. Guan C, Barron AB, He XJ, Wang ZL, Yan WY, Zeng ZJ. A comparison of digital gene expression profiling and methyl DNA immunoprecipitation as methods for gene discovery in honeybee (Apis mellifera) behavioural genomic analyses. PloS One. (2013) 8:e73628. doi: 10.1371/journal.pone.0073628
110. Ihle KE, Baker NA, Amdam GV. Insulin-like peptide response to nutritional input in honey bee workers. J Insect Physiol. (2014) 69:49–55. doi: 10.1016/j.jinsphys.2014.05.026
111. Wheeler MM, Ament SA, Rodriguez-Zas SL, Robinson GE. Brain gene expression changes elicited by peripheral vitellogenin knockdown in the honey bee: Vitellogenin RNAi and bee brain transcriptome. Insect Mol Biol. (2013) 22:562–73. doi: 10.1111/imb.12043
112. Ament SA, Corona M, Pollock HS, Robinson GE. Insulin signaling is involved in the regulation of worker division of labor in honey bee colonies. Proc Natl Acad Sci. (2008) 105:4226–31. doi: 10.1073/pnas.0800630105
113. Ihle KE, Mutti NS, Kaftanoglu O, Amdam GV. Insulin receptor substrate gene knockdown accelerates behavioural maturation and shortens lifespan in honeybee workers. Insects. (2019) 10:390. doi: 10.3390/insects10110390
114. Zayed A, Robinson GE. Understanding the relationship between brain gene expression and social behavior: lessons from the honey bee. Annu Rev Genet. (2012) 46:591–615. doi: 10.1146/annurev-genet-110711-155517
115. Kohlmeier P, Alleman AR, Libbrecht R, Foitzik S, Feldmeyer B. Gene expression is more strongly associated with behavioural specialization than with age or fertility in ant workers. Mol Ecol. (2019) 28:658–70. doi: 10.1111/mec.14971
116. Unnikrishnan S, Shah A, Bais D, Suryanarayanan A, Brockmann A. Conserved hormonal and molecular mechanisms underlying behavioural maturation in open- and cavity-nesting honey bees [Preprint]. Mol Biol. (2021). doi: 10.1101/2021.03.25.436783
117. Caminer MA, Libbrecht R, Majoe M, Ho DV, Baumann P, Foitzik S. Task-specific odorant receptor expression in worker antennae indicates that sensory filters regulate division of labor in ants. Commun Biol. (2023) 6:1004. doi: 10.1038/s42003-023-05273-4
118. Woodard SH, Bloch GM, Band MR, Robinson GE. Molecular heterochrony and the evolution of sociality in bumblebees (Bombus terrestris). Proc R Soc B: Biol Sci. (2014) 281:20132419. doi: 10.1098/rspb.2013.2419
119. Qiu H-L, Zhao C-Y, He Y-R. On the molecular basis of division of labor in solenopsis invicta (Hymenoptera: formicidae) workers: RNA-seq analysis. J Insect Sci. (2017) 17(2):48. doi: 10.1093/jisesa/iex002
120. Araujo NDS, Arias MC. Gene expression and epigenetics reveal species-specific mechanisms acting upon common molecular pathways in the evolution of task division in bees. Sci Rep. (2021) 11:3654. doi: 10.1038/s41598-020-75432-8
121. Xu H-J, Zhang C-X. Insulin receptors and wing dimorphism in rice planthoppers. Philos Trans R Soc B: Biol Sci. (2017) 372(1713):20150489. doi: 10.1098/rstb.2015.0489
122. Fawcett MM, Parks MC, Tibbetts AE, Swart JS, Richards EM, Vanegas JC, et al. Manipulation of insulin signaling phenocopies evolution of a host-associated polyphenism. Nat Commun. (2018) 9:1699. doi: 10.1038/s41467-018-04102-1
123. Smýkal V, Pivarči M, Provazník J, Bazalová O, Jedlička P, Lukšan O, et al. Complex evolution of insect insulin receptors and homologous decoy receptors, and functional significance of their multiplicity. Mol Biol Evol. (2020) 37:1775–89. doi: 10.1093/molbev/msaa048
124. Xu H-J, Xue J, Lu B, Zhang X-C, Zhuo J-C, He S-F, et al. Two insulin receptors determine alternative wing morphs in planthoppers. Nature. (2015) 519:464–7. doi: 10.1038/nature14286
125. Ding Y-J, Li G-Y, Xu C-D, Wu Y, Zhou Z-S, Wang S-G, et al. Regulatory functions of nilaparvata lugens GSK-3 in energy and chitin metabolism. Front Physiol. (2020) 11:518876. doi: 10.3389/fphys.2020.518876
126. Papadopoulou D, Bianchi MW, Bourouis M. Functional studies of shaggy/glycogen synthase kinase 3 phosphorylation sites in drosophila melanogaster. Mol. Cell. Biol. (2008) 24(11):4909–19.
127. Pooraiiouby R, Sharma A, Beard J, Reyes J, Nuss A, Gulia-Nuss M. Nutritional Quality during Development Alters Insulin-Like Peptides’ Expression and Physiology of the Adult Yellow Fever Mosquito, Aedes aEgypti. Insects. (2018) 9:110. doi: 10.3390/insects9030110
128. Snell-Rood EC, Moczek AP. Insulin signaling as a mechanism underlying developmental plasticity: the role of FOXO in a nutritional polyphenism. PloS One. (2012) 7:e34857. doi: 10.1371/journal.pone.0034857
129. Okada Y, Katsuki M, Okamoto N, Fujioka H, Okada K. A specific type of insulin-like peptide regulates the conditional growth of a beetle weapon. PloS Biol. (2019) 17:e3000541. doi: 10.1371/journal.pbio.3000541
130. Emlen DJ, Warren IA, Johns A, Dworkin I, Lavine LC. A mechanism of extreme growth and reliable signaling in sexually selected ornaments and weapons. Science. (2012) 337:860–4. doi: 10.1126/science.1224286
131. Lavine LC, Hahn LL, Warren IA, Garczynski SF, Dworkin I, Emlen DJ. Cloning and characterization of an mRNA encoding an insulin receptor from the horned scarab beetle onthophagus nigriventris (Coleoptera: scarabaeidae). Arch Insect Biochem Physiol. (2013) 82:43–57. doi: 10.1002/Arch.21072
132. Zera AJ. Juvenile Hormone and the endocrine regulation of wing polymorphism in insects: New insights from circadian and functional-genomic studies in Gryllus crickets. Physiol Entomol. (2016) 41:313–26. doi: 10.1111/phen.12166
133. Zera AJ, Vellichirammal NN, Brisson JA. Diurnal and developmental differences in gene expression between adult dispersing and flightless morphs of the wing polymorphic cricket, Gryllus firmus: Implications for life-history evolution. J Insect Physiol. (2018) 107:233–43. doi: 10.1371/journal.pgen.1009312
134. Shegelski VA, Evenden ML, Huber DPW, Sperling FAH. Identification of genes and gene expression associated with dispersal capacity in the mountain pine beetle, Dendroctonus ponderosae Hopkins (Coleoptera: Curculionidae). PeerJ. (2021) 9:e12382. doi: 10.7717/peerj.12382
135. Beckers OM, Kijimoto T, Moczek AP. Doublesex alters aggressiveness as a function of social context and sex in the polyphenic beetle Onthophagus taurus. Anim Behav. (2017) 132:261–9. doi: 10.1016/j.anbehav.2017.08.011
136. Newsom KD, Moczek AP, Schwab DB. Serotonin differentially affects morph-specific behavior in divergent populations of a horned beetle. Behav. Ecol. (2020) 31(2):352–60.
137. Schwab DB, Newsom KD, Moczek AP. Serotonin signaling suppresses the nutrition-responsive induction of an alternate male morph in horn polyphenic beetles. J Exp Zoology Part A: Ecol Integr Physiol. (2020) 333:660–9. doi: 10.1002/jez.2413
138. Yuan Y, Wang Y, Ye W, Yuan E, Di J, Chen X, et al. Functional evaluation of the insulin/insulin-like growth factor signaling pathway in determination of wing polyphenism in pea aphid. Insect Sci. (2023) 30:816–28. doi: 10.1111/1744-7917.13121
139. Stamps JA, Frankenhuis WE. Bayesian models of development. Trends Ecol Evol. (2016) 31:260–8. doi: 10.1016/j.tree.2016.01.012
140. Rittschof CC, Hughes KA. Advancing behavioural genomics by considering timescale. Nat Commun. (2018) 9:489. doi: 10.1038/s41467-018-02971-0
141. Li S, Huang X, McNeill MR, Liu W, Tu X, Ma J, et al. Dietary stress from plant secondary metabolites contributes to grasshopper (Oedaleus asiaticus) migration or plague by regulating insect insulin-like signaling pathway. Front Physiol. (2019) 10:531. doi: 10.3389/fphys.2019.00531
142. Wang S, Yang H, Hu Y, Zhang C, Fan D. Multi-omics reveals the effect of population density on the phenotype, transcriptome and metabolome of mythimna separata. Insects. (2023) 14:68. doi: 10.3390/insects14010068
143. Koyama T, Mendes CC, Mirth CK. Mechanisms regulating nutrition-dependent developmental plasticity through organ-specific effects in insects. Front Physiol. (2013) 4:263. doi: 10.3389/fphys.2013.00263
144. Kremer LPM, Korb J, Bornberg-Bauer E. Reconstructed evolution of insulin receptors in insects reveals duplications in early insects and cockroaches. J Exp Zoology Part B: Mol Dev Evol. (2018) 330:305–11. doi: 10.1002/jez.b.22809
145. Rybczynski R, Bell SC, Gilbert LI. Activation of an extracellular signal-regulated kinase (ERK) by the insect prothoracicotropic hormone. Mol Cell Endocrinol. (2001) 184:1–11. doi: 10.1016/S0303-7207(01)00664-5
146. Bednářová A, Kodrík D, Krishnan N. Knockdown of adipokinetic hormone synthesis increases susceptibility to oxidative stress in Drosophila—A role for dFoxO? Comp Biochem Physiol Part C: Toxicol Pharmacol. (2015) 171:8–14. doi: 10.1016/j.cbpc.2015.03.006
147. Mury FB, Lugon MD, Da Fonseca RN, Silva JR, Berni M, Araujo HM, et al. Glycogen Synthase Kinase-3 is involved in glycogen metabolism control and embryogenesis of Rhodnius prolixus. Parasitology. (2016) 143:1569–79. doi: 10.1017/S0031182016001487
148. Hunt JH, Wolschin F, Henshaw MT, Newman TC, Toth AL, Amdam GV. Differential gene expression and protein abundance evince ontogenetic bias toward castes in a primitively eusocial wasp. PloS One. (2010) 5:e10674. doi: 10.1371/journal.pone.0010674
149. Libbrecht R, Corona M, Wende F, Azevedo DO, Serrão JE, Keller L. Interplay between insulin signaling, juvenile hormone, and vitellogenin regulates maternal effects on polyphenism in ants. Proc Natl Acad Sci. (2013) 110:11050–5. doi: 10.1073/pnas.1221781110
150. Wang Z-J, Peng Y-Q, Compton SG, Yang D-R. Reproductive strategies of two forms of flightless males in a non-pollinating fig wasp under partial local mate competition. Ecol Entomol. (2010) 35:691–7. doi: 10.1111/j.1365-2311.2010.01228.x
151. Cook JM, Bean D. Cryptic male dimorphism and fighting in a fig wasp. Anim Behav. (2006) 71:1095–101. doi: 10.1016/j.anbehav.2005.07.027
Keywords: nutrition, developmental plasticity, fat body, foraging, fecundity, mating, social insect, genetic tool kit
Citation: Weger AA and Rittschof CC (2024) The diverse roles of insulin signaling in insect behavior. Front. Insect Sci. 4:1360320. doi: 10.3389/finsc.2024.1360320
Received: 22 December 2023; Accepted: 21 March 2024;
Published: 04 April 2024.
Edited by:
Peter M. Piermarini, The Ohio State University, United StatesReviewed by:
Mark R. Brown, University of Georgia, United StatesMonika Gulia-Nuss, University of Nevada, Reno, United States
Copyright © 2024 Weger and Rittschof. This is an open-access article distributed under the terms of the Creative Commons Attribution License (CC BY). The use, distribution or reproduction in other forums is permitted, provided the original author(s) and the copyright owner(s) are credited and that the original publication in this journal is cited, in accordance with accepted academic practice. No use, distribution or reproduction is permitted which does not comply with these terms.
*Correspondence: Clare C. Rittschof, clare.rittschof@uky.edu