- Department of Biological Sciences, California State University San Marcos, San Marcos, CA, United States
Voltage-gated ion channels (VGICs) respond to changes in membrane potential (Vm) and typically exhibit fast kinetic properties. They play an important role in signal detection and propagation in excitable tissues. In contrast, the role of VGICs in non-excitable tissues like epithelia is less studied and less clear. Studies in epithelia of vertebrates and invertebrates demonstrate wide expression of VGICs in epithelia of animals. Recently, VGICs have emerged as regulators of ion transport in the Malpighian tubules (MTs) and other osmoregulatory organs of insects. This mini-review aims to concisely summarize which VGICs have been implicated in the regulation of ion transport in the osmoregulatory epithelia of insects to date, and highlight select groups for further study. We have also speculated on the roles VGICs may potentially play in regulating processes connected directly to ion transport in insects (e.g., acid-base balance, desiccation, thermal tolerance). This review is not meant to be exhaustive but should rather serve as a thought-provoking collection of select existing highlights on VGICs, and to emphasize how understudied this mechanism of ion transport regulation is in insect epithelia.
1 Introduction
Insects are the most diverse group of animals with approximately 1 million species that span all geographical, aquatic, and terrestrial environments. In order to overcome their physiological disadvantage of having a large surface-to-volume ratio, insects have evolved and conserved a diverse range of systemic osmoregulatory functions to maintain internal homeostatic conditions (1, 2) that protect against unfavorable environmental challenges (3). Many insects face frequent and rapid salt-and-water imbalance due to their environmental ion availability changes, feeding habits, alterations in acid-base balance, desiccation, changes in environmental temperatures, and even buoyancy.
For instance, a blood-feeding mosquito must get rid of extra water ingested with a blood meal rapidly to address the post-prandial salt-and-water imbalance. A caterpillar eating ~5 times its own body weight in food daily must excrete gargantuan amounts of metabolic wastes and plant-based xenobiotics and be able to adjust ion transport within minutes when necessary. To add insult to injury, the only way for a caterpillar to digest plant-based food and tap into the nutritional potential of plants is to raise midgut pH to ~11 to dissociate plant proteins from tannins (4). Some insects (e.g., beetles) possess a sophisticated “desiccation tolerant” physiological adaptation to cope with intermittent or evaporative water loss by accumulating and storing water in the haemolymph (2, 5, 6). Many insects face rapid and drastic changes in the temperature of their environment. Chill-susceptible insects experience chill injury or chill coma with exposure to unfavorable thermal conditions when key osmoregulatory and active ion transport mechanisms decline, disturb membrane polarization and ion balance, and negatively impact energy budgets as temperatures decrease (1, 7, 8). Chaoborus midge larvae control buoyancy by manipulating pH levels using ion-transporting endothelia adjacent to the air-sac’s pH-sensitive protein resilin, which encourages the passive diffusion of gases across the endothelium that covers air-sac (9). All of these processes involve epithelia/endothelia that may benefit from employing fast ion transport-regulating mechanisms like the one discussed in this review.
Epithelial tissues simultaneously serve as: (i) a barrier between internal and external environment, and (ii) as a conduit for selective exchange of ions, nutrients, and wastes. Integral membrane proteins, like ion pumps, channels, aquaporins, and transporter are embedded in the membrane and form complexes that regulate Vm (10, 11) and provide a transmembrane pathway for ions and fluid (12).
Ion channels can be activated by temperature, osmolarity, ligand binding, mechanical change in the cell membrane, pH, neuroendocrine signals, and Vm (13–15) and display a spectrum of ion selectivity (Na+, K+, Cl, and Ca2+) (16). For the purpose of this review, voltage-gated ion channels (VGICs) are broadly defined as channels belonging to the voltage-gated ion channel superfamily regardless of whether they’re actually activated by changes in Vm, which is considered on a case-by-case basis. The primary function of VGICs is to generate action potentials in excitable tissues in response to changes in the cell Vm (17–23). Since the development of patch-clamp electrophysiology, protein purification and molecular and biochemical techniques, >250 types of ion channels have been identified (24, 25).
Interestingly, expression of many CaV, KV, NaV, and non-selective cation permeable VGICs has been reported specifically in epithelia of animals ranging from early divergent Placozoans to vertebrates (e.g., 26) (See Table 1). The genesis of the study of how VGICs regulate ion transport in insect epithelia traces itself to a recent collection of studies that investigate the topic in the Malpighian tubules (MTs) of lepidopterans. Analogous to the mammalian kidney, the MTs of insects, together with the hindgut, serve as the primary osmoregulatory and excretory organs carefully balancing the uptake and recycling of vital ions and fluid while efficiently excreting ingested xenobiotics/toxins, and metabolic wastes (50).
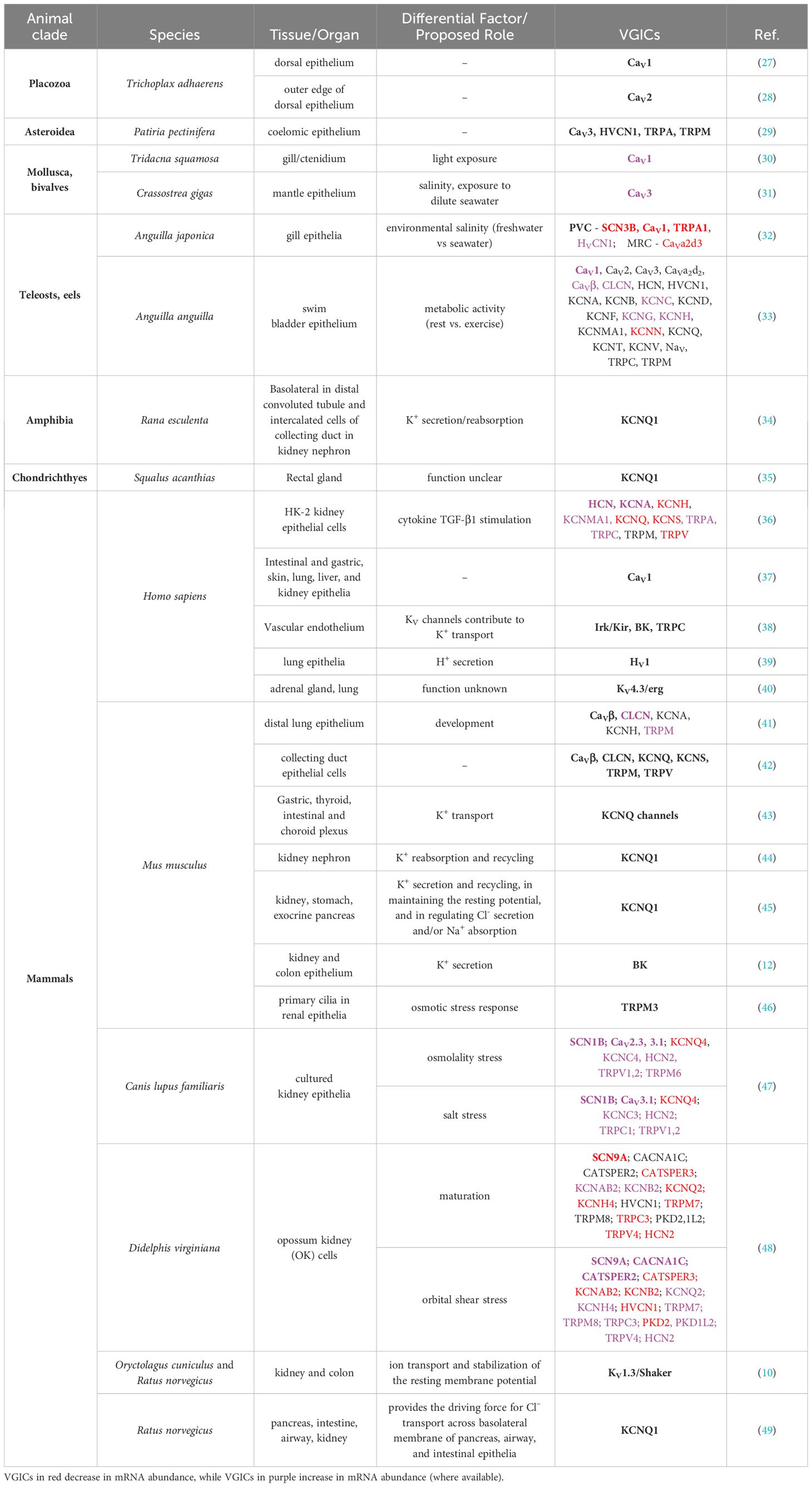
Table 1 Voltage-gated ion channels (VGICs) expressed in non-insect epithelia with potential roles they may play noted (where available).
One phenomenon is common to all insect MTs – they must secrete ions into their lumen to osmotically draw water in, and drive excretion and osmoregulation (see Figure 1) (51). Evolving approximately 350 million years ago, the highly conserved two-cell-type structure of insect MT epithelium, comprised of stellate cells (SC) and principal cells (PCs), employ physiologically distinctive cell-specific transepithelial cation and anion transporting mechanisms (2). Although, the MTs of Drosophila melanogaster have been used as the insect model of study for human renal pathologies (52, 53), there are substantial differences in complexity of the insect MTs between different clades of insects (54), highlighting the need for clade-specific investigation of ion transport mechanisms in osmoregulatory epithelia (see Figure 1A). For instance, how PCs and SCs transport ions does not translate between insect clades, and in many cases blind ends of MTs are either closely associated with the hindgut or are embedded into a specialized structure (15, 55, 56).
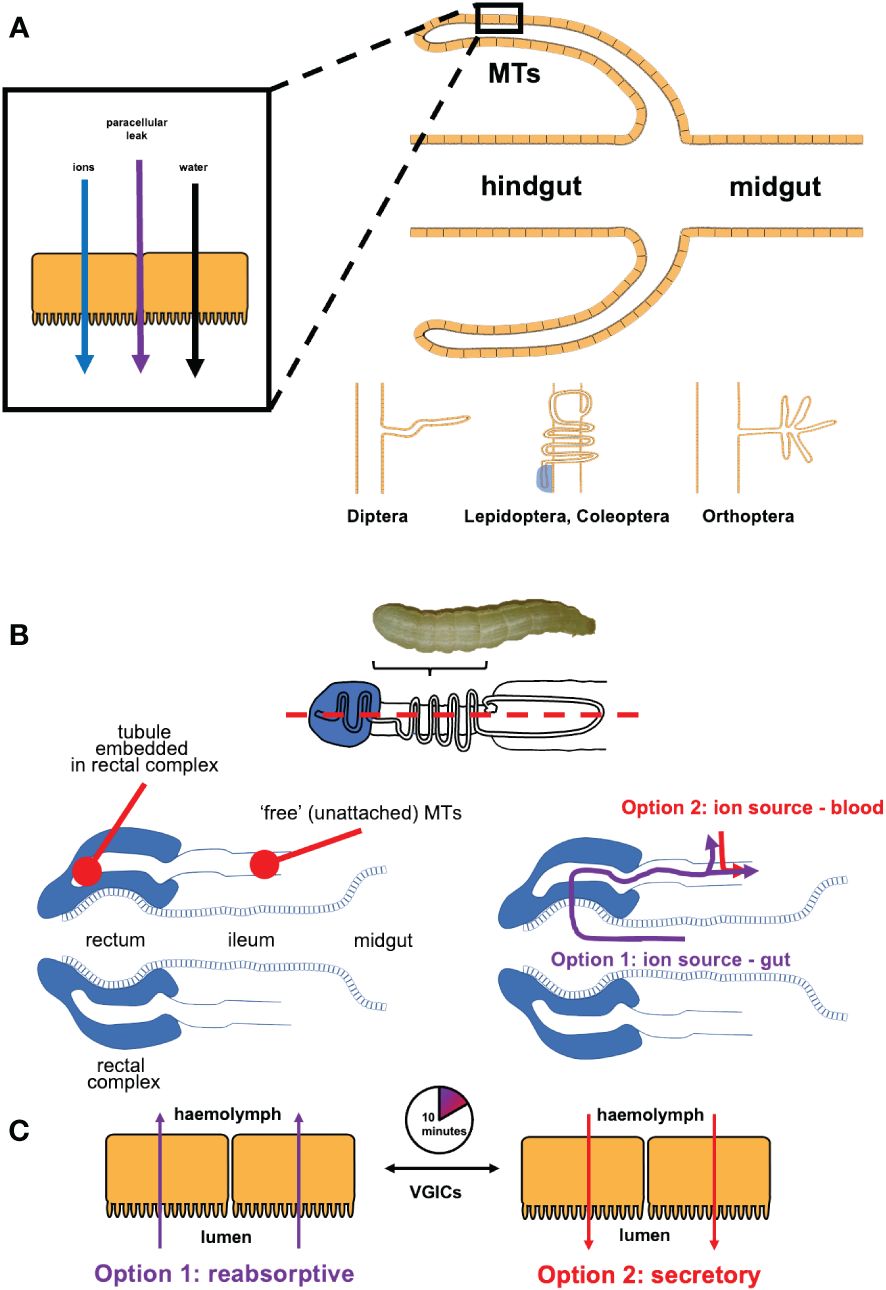
Figure 1 (A) Malpighian tubules (MTs) are blind outpouchings between the midgut and the hindgut of an insect - they secrete fluid by secreting ions into the lumen, and allowing water to follow by osmosis, with a paracellular junction leak component. Structure and functional adaptation depends on the insect clade. (B) In the larval lepidopterans, the distal end of each tubule is embedded into the rectal complex (in longitudinal section, dashed blue line, top panel). Each embedded tubule connects to the ‘free’ (unattached) region, suspended in the hemolymph and closely juxtaposed to the gut. Larvae have two options as a source of ions and water for secreting fluid in their MTs. The reabsorptive option (in purple) sources ions and water from the gut by way of the blind embedded tubules when the caterpillars are well fed. Some ions reabsorbed from the gut are transferred into the hemolymph across the unattached MTs. The secretory option (in red) sources ions and water from the larva’s hemolymph, when dietary ions and/or water are unavailable or are in short supply (e.g., postprandially or during molting). (C) The switchover between reabsorption and secretion takes as little as 10 min.
In lepidopteran larvae, the distal end of MT is embedded into a specialized structure, the rectal complex (see Figure 1B). This allows the larvae to extract ions and water from their diet that they use to secrete fluid in the MTs. The remainder of the tubule (not embedded into the rectal complex) reabsorbs ions. However, when the gut is empty (e.g., during moulting or cessation of feeding), ions and water cannot be procured from the gut. A downstream region called the distal ileac plexus rapidly switches from ion reabsorption to ion secretion to enable osmotic secretion of fluid into the MTs, and to ensure that MTs function is not interrupted. This process is regulated in part by VGICs, which likely contribute to how rapidly (~10 min) the switch takes place in the MTs (Figure 1C). Given profound differences in the structure and function of MTs in insect clades, this begged the question of whether VGICs may be present in the MTs of other insects? Perhaps, even in other osmoregulatory epithelia of insects?
In addition to the MTs and the hindgut, many insects employ epithelia/endothelia that either play a direct role in osmoregulation (e.g., anal papillae of mosquitoes (e.g., 57, 58), collophore epithelia of springtails (e.g., 59)), or employ ion transport that is tied to other functions - e.g., gas bladder endothelia that regulate buoyancy in midge larvae (e.g., 9), blood-brain barrier endothelia that maintain the ion content of neuron-bathing fluid (e.g., 60). Caution must be used, however in the study of whether VGICs regulate ion transport in many of these organs, as excitable tissues layers (nerves, muscles) may confound results obtained in whole-organ studies.
Lastly, MTs epithelia play important roles in the immune response, oxidative stress, and response to xenobiotics and toxins (61). A speedy response to any of these using fast-acting VGICs would surely be of benefit to insects.
2 Voltage-gated Ca2+ channels
CaV channels are selectively permeable to Ca2+ and open in response to membrane depolarization (62, 63). In the osmoregulatory epithelia of insects, CaV1 has been detected in the MTs of Drosophila melanogaster, and implicated in intracellular signaling, directional Ca2+ transport and the regulation of diuresis (64). Likewise, CaV1 was shown regulate epithelial ion transport in the MTs of larval Trichoplusia ni (65), and larval and adult Aedes aegypti (58). Additionally, the same study has localized CaV1 in hindgut epithelium of the blood-fed adults of Aedes aegypti, where it may play a role in the regulation of post-prandial diuresis. The function of other CaV channels in insect epithelia remains unclear. In most animals studied to date, CaV channels demonstrate conserved biophysical properties across clades, and CaV2 has different properties from CaV1 and CaV3 (66). The use of isoform-specific inhibitors may help discern the roles of other CaV3 isoforms in the MT of insects. Typical Vm of epithelial cells in MTs varies depending on the insect clade and osmoregulatory status of the animal (e.g., post-prandial, ion-rich diet). The use of high- and low-voltage activated CaV channels may provide an additional link between the regulation of ion transport and Ca2+ signaling. Ca2+ as a second messenger whose role is to maintain Ca2+ stores and concentration, a powerful activator of potassium transport mechanisms (14).
In addition, as Ca2+ is a general second messenger, there is potential for CaV channels to be involved in the regulation of epithelial processes other than ion transport, such as acid-base balance, desiccation and thermal tolerance, buoyancy control, and regulation of blood-brain barrier.
3 Voltage-gated K+ channels
KV channels belong to a diverse superfamily of proteins (67, 68) distinguished by their K+ selectivity (21), and their role in K+ transport, recycling, and intracellular signaling (69–71). Within the K+ channel superfamily, KV channels and at least 9 of their subfamilies are widely expressed in the membranes of excitable and non-excitable tissues (10, 72). KV channels can also be activated by Na+ or Ca2+, can be inwardly rectifying or delayed rectifying, and display fast or slow kinetics of opening and closing (68, 73–76). KV channels (KV1-KV4) named Shaker/KV1.3, Shab/KV2, Shaw/Kv3.1, and Shal/KV4, based on Drosophila studies, vary in biophysical properties (77–82).
Inward-rectifying potassium (Kir) channels received their name from the fact that the inward flow of K+ into the cell at any given voltage is greater than the outflow at same voltage but opposite in polarity (83). Kir channels play a role in K+ secretion into MTs of the yellow fever mosquito Aedes aegypti, the fruit fly Drosophila melanogaster, and larval lepidopteran Trichoplusia ni (84). In Drosophila, three Kir homologues irk1, irk2 and irk3 are involved in PC-based K+ secretion (85, 86). In the MTs of yellow fever mosquito Aedes aegypti, transepithelial secretion of K+ is regulated by Kir1 located in the SC’s basolateral membrane and by Kir2B in the basolateral membrane of PC’s (87, 88). Together Kir1 and Kir2B are credited with conducting 66% of total transepithelial K+ secretion in Aedes MTs. Additionally, Kir1 has been shown to play a role in K+ secretion in the basolateral membrane of principal cells of a larval lepidopteran T. ni (55). Notably, there are pronounced differences in cellular localization of Kir isoforms in the MTs of different insects but have been detected in the MTs of larval lepidopterans using transcriptomic approaches (56, 89).
KCNQ/KV7.1 are VGICs found in excitable and non-excitable cells that are sensitive to extracellular [K+], providing constant repolarizing force that controls Vm (21). Interestingly, the membrane protein Potassium Voltage-gated Channel Subfamily E Regulatory Unit 3 (KCNE3) regulates the function of K+ channel KCNQ1 channel by co-assembling with its ɑ subunits preventing channel closure and converting it into a voltage-independent channel (90), while remaining constitutively active and open (43) at a negative voltage that would typically close the channel (91, 92). In insect epithelia, KCNQ1 was found to be enriched specifically in Drosophila MTs, although its function in the renal tissue has not been investigated to date (93). Recently, several studies detected KCNQ channels in MTs of larval lepidopteran T. ni (89, 94). KCNQ1 has also been detected in the MTs of larval dipteran Ae. aegypti where this channel may contribute to K+ secretion in the MTs of brackish water (BW) larvae, helping the larvae rid themselves of extra K+ and preventing K+ loading with BW exposure. KCNQ1 was also found in anal papillae of larval Ae. aegypti, where it may play a role in uptake of environmental K+ by the AP of larvae in freshwater (FW), aiding larvae in retention of hemolymph K+ in the face of diffusional K+ loss to FW (58).
In addition to being directly involved in ion transport in osmoregulatory epithelia, KV channels may be involved in response to extracellular hyperkalemia observed in chill-injured insects (e.g., 95). Low levels of K+ are essential for the proper function of the brain in insects (96), and potential use of KV channels by the blood-brain barrier endothelia (especially in K+-feeding herbivores) may be advantageous for rapid rebalancing of K+ between body compartments.
4 Voltage-gated Na+ channels
The ability to rapidly regulate natriuresis may be advantageous to certain insects – e.g., the female mosquito can rapidly increase diuresis to efficiently remove excess Na+ post ingestion of a blood meal (97). In blood-feeders, the electrochemical potential of Na+ is what drives Na+-rich fluid secretion (98). Transcripts encoding NaV channels like Para and Nalcn have been detected in the MTs of larval lepidopterans T. ni (89, 94) and Helicoverpa armigera (99) as well as that both MTs and anal papillae of larval dipteran Ae. aegypti (58). Previous pharmacological studies suggested that apical Na+ channels regulated Na+ uptake in anal papillae (57, 100) and Na+ channels present in the MTs of Ae. aegypti transport Na+ from haemolymph to lumen via voltage gradients created by V-type H+ ATPase (88, 101, 102). A recent study demonstrated that Nalcn is present in the water-facing membrane of anal papillae in the larval Ae. aegypti (58). Although a significant number of neuropeptide toxins specific to NaV channels have been identified among vertebrates, insect NaV channels are remarkably different, which may prove mechanistic study of their function in insect epithelia rather challenging (103).
Na+ balance is especially important to plant-feeding insects as all excitable tissues require Na+ for producing action potentials, but it can be quite low in the diet, necessitating bizarre behaviors like puddling in adult moths aimed at supplementation of low dietary Na+ with that acquired from mud puddles of vertebrate urine. Thus, in addition potentially contributing to epithelial Na+ transport NaV channels could be used by blood-brain endothelia to quickly rebalance Na+ between body compartments.
5 Cation-selective VGICs
5.1 Transient receptor potential channels
TRP channels are cation-permeable (Na+, K+, Ca2+) voltage-dependent channels (104). TRP channels are activated through a range of gating mechanisms including Vm depolarization (105). TRPA channels carry out functions in many excitable and non-excitable tissues like those found in Drosophila gut epithelia (106, 107). Several families of TRP channels have been reported in the osmoregulatory epithelia of in insects, including insect-specific Painless and Pyrexia TRPA channels. TRPA channels have been detected in MTs of larval T. ni (89), Pieris rapae (108) and Bactrocera dorsalis (109). TRPA1, TRP/Painless and TRP/Pyrexia are more permeable to K+ than to Na+, with well-established roles in nociception and thermotaxis in excitable tissues of insects (110–112). Six TRPA channels have been detected in MTs and AP of larval Ae. aegypti and mRNA abundance of every channel was altered as a result of BW exposure in MTs or anal papillae (58). TRP channels may provide an additional link between epithelial Vm, ion transport, Ca2+ signaling, and activation of other VGICs in insect MTs.
In addition to playing a role in the regulation of epithelial ion transport, TRP channels like Painless may serve as peripheral thermal sensors since they have been shown to respond to increased temperatures in vitro (i.e., regardless of the context of which tissue they’re expressed in) (113). Attuning the function of osmoregulatory epithelia to changes in environmental temperature may be particularly advantageous for these small ectothermic animals.
5.2 Hyperpolarization-activated cyclic nucleotide-gated channels
Whereas most VGICs open in response to depolarization, a stand-out group of VGICs is activated by hyperpolarization - the HCN channels, which are permeable to both Na+ and K+, and are additionally activated by cyclic nucleotides, where the latter overrides dependence on Vm changes (114). In osmoregulatory epithelia of insects, the HCN channels have been detected in the MTs of larval lepidopteran T. ni (57, 58, 88, 89, 94) and when HCN1 channels are blocked in MTs, ion transport switches direction from K+ secretion to K+ reabsorption (89). Cyclic nucleotides are known to alter fluid secretion in the MTs of larval and adult insects (e.g., 115–117). HCN channels can provide an additional link between direct activation of ion transport and second messenger-based hormone action.
6 Select unstudied VGICs detected in osmoregulatory epithelia of multiple insects
Transcriptomic studies on osmoregulatory epithelia of lepidopterans and dipterans uncovered the presence of many more VGICs (e.g., HV, ClV, BK, KCNA, KCNC, KCNS, TRP M, TRP V channels), expression of which has not been confirmed using direct molecular, protein-based, or pharmacological approaches to date (26, 58, 65, 89, 94). Whether these VGICs play a role in the regulation of ion transport in insect epithelia remains unexplored. HV and ClV channels may play a role in acid-base balance regulation since acid H+ transport can contribute to acid equivalents, and Cl- is often transported by epithelia in exchange for HCO3- – to the best of our knowledge, these have not been examined in osmoregulatory epithelia of insects to date. TRP channels may serve as peripheral sensors of allelochemicals and xenobiotics in herbivores as many TRPs are activated by noxious plant-based chemicals, as well as peripheral temperature sensors (see above). Many TRP channels are volume-sensing and mechanosensitive (118), both of which would offer insect epithelia an additional mechanism for fine-tuning their ion transport rapidly.
7 Discussion
7.1 Current gaps in research – what remains to be explored
7.1.1 Are VGICs connected with mechanosensation in epithelia?
The role of VGICs in excitable tissues of animals has been well established (37). In insect epithelia however, VGICs seem to play an integral role in the regulation of ion transport and of Vm yet studies on this topic remain largely scarce.
A likely advantage of the presence of VGICs in animal epithelia is the ability to quickly respond to changing ion concentrations. In the MTs of insects, this may be relayed via mechanosensation of fluid flow and hydrostatic pressure, which may result from alternating bouts of diuresis and anti-diuresis, changing epithelial cell volume by applying pressure to the cells against the basal lamina that encases the MT epithelia. Mechanosensitive ion channels found in insect epithelia (e.g., 58, 89, 94) can detect mechanical changes in the epithelial cell membrane caused by changes in fluid flow, hydrostatic pressure and cell volume, and open resulting in changes in Vm and setting off intracellular second messengers (cAMP, Ca2+) cascades (119). VGICs have been shown to respond transcriptionally to mechanical stress at least in some epithelia and can be used to amplify this signal (see Table 1). An instance of this has been described in intestinal epithelia, where TRPM5 channel triggers membrane depolarization in response to nutrient levels and opening of CaV channels amplifies the Ca2+ signal.
7.1.2 How are VGICs activated in insect epithelia?
Mechanisms of VGIC activation in insect epithelia remain largely unexplored. Peptide toxins extracted from metazoan venoms that target the specific subtypes of animal VGICs may prove to be a useful pharmacological tool to gaining a better understanding of insect VGICs (103). Do VGICs respond directly to the changes in epithelial cell Vm resulting from altered intracellular and extracellular ion concentrations? Are VGICs activated by other upstream mechanisms (e.g., mechanosensitive and/or ligand-gated ion channels)? When luminal fluid flow decreases, cell volume may increase, activating mechanosensation and recruiting additional directional ion transport. MTs of insects have recently been shown to react to changes in hydrostatic pressure (120). Ligand-gated ion channels have similarly been detected in the MTs of multiple insect clades (e.g., 121, 122) and epithelial Vm can change in response to stimulation of neurotransmitters and endocrine ligands. VGIC can also be used to amplify activation of ligand-gated ion channels.
7.1.3 Do VGICs remain voltage-gated in epithelia?
Voltage sensitivity varies between the different types of VGICs with KV, NaV, and CaV displaying high sensitivity, activating and opening following membrane depolarization (43), and TRP channels demonstrate comparatively low voltage sensitivity (16). It is widely accepted that the biophysical properties of VGICs, although conserved in the same channel across many taxa, may be modified by channel subunit assembly for VGICs that are made up of multiple subunits. For instance, KV ɑ subunit complexes can also co-assemble with β subunits of KV channels creating isoforms with different biophysical properties (19, 21, 22, 72, 90, 123).
8 Conclusions
It has been decades since the first VGIC was reported in animal epithelia. Recent studies in osmoregulatory epithelia of insects point to an abundance of VGICs, several of which have already been demonstrated to regulate ion transport. Further study of the roles specific VGICs play in the regulation of ion transport in insect epithelia may prove a fruitful ground for basic knowledge needed for instance to design better targeted integrated pest management strategies.
Important questions remain about the role of VGICs in the osmoregulatory epithelia of insects. Do VGICs demonstrate the same ion selectivity in epithelia as they do in excitable tissues? Do VGICs directly contribute to directional ion transport? Do VGICs establish the driving force for ion transport? Do VGICs establish/set Vm in insect epithelia? Do VGICs assemblages in insect epithelia differ based on external salinity, dietary ion availability, and ions used to drive diuresis in MTs (e.g., K+-eating herbivores vs Na+-eating omnivores/carnivores/puddlers)? Are VGICs used to regulate processes connected to the osmoregulatory function in insects – e.g., acid-base balance, desiccation, thermal tolerance, buoyancy control, regulation of blood-brain barrier?
Author contributions
JD: Conceptualization, Writing – original draft, Writing – review & editing. DK: Conceptualization, Data curation, Formal analysis, Funding acquisition, Investigation, Methodology, Supervision, Visualization, Writing – original draft, Writing – review & editing.
Funding
The author(s) declare financial support was received for the research, authorship, and/or publication of this article. JD was supported by the National Institutes of Health/National Institute of General Medical Sciences B2PhD program under award number T32GM146604. The majority of the work was supported by the startup and internal funds provided by California State University to DK.
Conflict of interest
The authors declare that the research was conducted in the absence of any commercial or financial relationships that could be construed as a potential conflict of interest.
Publisher’s note
All claims expressed in this article are solely those of the authors and do not necessarily represent those of their affiliated organizations, or those of the publisher, the editors and the reviewers. Any product that may be evaluated in this article, or claim that may be made by its manufacturer, is not guaranteed or endorsed by the publisher.
References
1. Overgaard J, MacMillan HA. The integrative physiology of insect chill tolerance. Annu Rev Physiol. (2017) 79:187–208. doi: 10.1146/annurev-physiol-022516-034142
2. Halberg KV, Denholm B. Mechanisms of systemic osmoregulation in insects. Annu Rev Entomol. (2024) 69:415–38. doi: 10.1146/annurev-ento-040323-021222
3. Huang J-H, Jing X, Douglas AE. The multi-tasking gut epithelium of insects. Insect Biochem Mol Biol. (2015) 67:15–20. doi: 10.1016/j.ibmb.2015.05.004
4. Berenbaum M. Adaptive significance of midgut pH in larval Lepidoptera. Am Nat. (1980) 115:138–46. doi: 10.1086/283551
5. Wang C-Y, Bong L-J, Neoh K-B. Adult paederus fuscipes (Coleoptera: staphylinidae) beetles overcome water loss with increased total body water content, energy metabolite storage, and reduced cuticular permeability: age, sex-specific, and mating status effects on desiccation. Environ Entomology. (2019) 48:911–22. doi: 10.1093/ee/nvz065
7. Orr SE, Buchwalter DB. It’s all about the fluxes: Temperature influences ion transport and toxicity in aquatic insects. Aquat Toxicol. (2020) 221:105405. doi: 10.1016/j.aquatox.2020.105405
8. Overgaard J, Gerber L, Andersen MK. Osmoregulatory capacity at low temperature is critical for insect cold tolerance. Curr Opin Insect Sci. (2021) 47:38–45. doi: 10.1016/j.cois.2021.02.015
9. McKenzie EKG, Kwan GT, Tresguerres M. Matthews PGD. A pH-powered mechanochemical engine regulates the buoyancy of Chaoborus midge larvae. Curr Biol. (2022) 32:927–933.e5. doi: 10.1016/j.cub.2022.01.018
10. Grunnet M, Rasmussen HB, Hay-Schmidt A, Klaerke DA. The voltage-gated potassium channel subunit, Kv1.3, is expressed in epithelia. Biochim Biophys Acta (BBA) - Biomembranes. (2003) 1616:85–94. doi: 10.1016/S0005-2736(03)00198-6
11. Mathew LG, Campbell EM, Yool AJ, Fabrick JA. Identification and characterization of functional aquaporin water channel protein from alimentary tract of whitefly, Bemisia tabaci. Insect Biochem Mol Biol. (2011) 41:178–90. doi: 10.1016/j.ibmb.2010.12.002
12. Morera FJ, Saravia J, Pontigo JP, Vargas-Chacoff L, Contreras GF, Pupo A, et al. Voltage-dependent BK and Hv1 channels expressed in non-excitable tissues: New therapeutics opportunities as targets in human diseases. Pharmacol Res. (2015) 101:56–64. doi: 10.1016/j.phrs.2015.08.011
13. Begenisich T, Melvin JE. Regulation of chloride channels in secretory epithelia. J Membrane Biol. (1998) 163:77–85. doi: 10.1007/s002329900372
14. Van Den Brink GR, Bloemers SM, Van Den Blink B, Tertoolen LG, Van Deventer SJ, Peppelenbosch MP. Study of calcium signaling in non-excitable cells. Microsc Res Tech. (1999) 46:418–33. doi: 10.1002/(SICI)1097-0029(19990915)46:6<418::AID-JEMT9>3.0.CO;2-0
15. Koyama T, Naseem MT, Kolosov D, Vo CT, Mahon D, Jakobsen ASS, et al. A unique Malpighian tubule architecture in Tribolium castaneum informs the evolutionary origins of systemic osmoregulation in beetles. Proc Natl Acad Sci USA. (2021) 118:e2023314118. doi: 10.1073/pnas.2023314118
16. Beyder A, Farrugia G. Targeting ion channels for the treatment of gastrointestinal motility disorders. Therap Adv Gastroenterol. (2012) 5:5–21. doi: 10.1177/1756283X11415892
17. Hodgkin AL, Huxley AF. The components of membrane conductance in the giant axon of Loligo. J Physiol. (1952) 116:473–96. doi: 10.1113/jphysiol.1952.sp004718
18. Catterall WA. Voltage-gated calcium channels. Cold Spring Harbor Perspect Biol. (2011) 3:a003947–a003947. doi: 10.1101/cshperspect.a003947
19. Jan LY, Jan YN. Voltage-gated potassium channels and the diversity of electrical signalling. J Physiol. (2012) 590:2591–9. doi: 10.1113/jphysiol.2011.224212
20. Zhang J, Chen X, Xue Y, Gamper N, Zhang X. Beyond voltage-gated ion channels: Voltage-operated membrane proteins and cellular processes. J Cell Physiol. (2018) 233:6377–85. doi: 10.1002/jcp.26555
21. Abbott GW. KCNQs: ligand- and voltage-gated potassium channels. Front Physiol. (2020) 11:583. doi: 10.3389/fphys.2020.00583
22. Rems L, Kasimova MA, Testa I, Delemotte L. Pulsed electric fields can create pores in the voltage sensors of voltage-gated ion channels. Biophys J. (2020) 119:190–205. doi: 10.1016/j.bpj.2020.05.030
23. Solé L, Roig SR, Vallejo-Gracia A, Serrano-Albarrás A, Martínez-Mármol R, Tamkun MM, et al. The carboxy terminal domain of Kv1.3 regulates functional interactions with the KCNE4 subunit. J Cell Sci. (2016) 129:4265–77. doi: 10.1242/jcs.191650
24. Neher E, Sakmann B. Single-channel currents recorded from membrane of denervated frog muscle fibres. Nature. (1976) 260:799–802. doi: 10.1038/260799a0
25. Chen AY, Brooks BR, Damjanovic A. Ion channel selectivity through ion-modulated changes of selectivity filter p k a values. Proc Natl Acad Sci USA (2023) 120:e2220343120. doi: 10.1073/pnas.2220343120
26. Kapoor D, Khan A, O’Donnell MJ, Kolosov D. Novel mechanisms of epithelial ion transport: insights from the cryptonephridial system of lepidopteran larvae. Curr Opin Insect Sci. (2021) 47:53–61. doi: 10.1016/j.cois.2021.04.001
27. Gauberg J, Elkhatib W, Smith CL, Singh A, Senatore A. Divergent Ca2+/calmodulin feedback regulation of CaV1 and CaV2 voltage-gated calcium channels evolved in the common ancestor of placozoa and bilateria. J Biol Chem (2022) 298:101741.
28. Gauberg J, Abdallah S, Elkhatib W, Harracksingh AN, Piekut T, Stanley EF, et al. Conserved biophysical features of the CaV2 presynaptic Ca2+channel homologue from the early-diverging animal Trichoplax adhaerens. J Biol Chem. (2020) 295:18553–78. doi: 10.1074/jbc.RA120.015725
29. Kim CH, Go HJ, Oh HY, Jo YH, Elphick MR, Park NG. Transcriptomics reveals tissue/organ-specific differences in gene expression in the starfish Patiria pectinifera. Mar Genomics. (2018) 37:92–6. doi: 10.1016/j.margen.2017.08.011
30. Cao-Pham AH, Hiong KC, Boo MV, Choo CYL, Wong WP, Chew SF, et al. Calcium absorption in the fluted giant clam, tridacna squamosa, may involve a homolog of voltage-gated calcium channel subunit α1 (CACNA1) that has an apical localization and displays light-enhanced protein expression in the ctenidium. J Comp Physiol B. (2019) 189:693–706. doi: 10.1007/s00360-019-01238-4
31. Sillanpää JK, Cardoso JCDR, Félix RC, Anjos L, Power DM, Sundell K. Dilution of seawater affects the Ca2+ transport in the outer mantle epithelium of crassostrea gigas. Front Physiol (2020) 11:1. doi: 10.3389/fphys.2020.00001
32. Lai KP, Li J-W, Je G, Chan T-F, Tse WKF, Wong CKC. Transcriptomic analysis reveals specific osmoregulatory adaptive responses in gill mitochondria-rich cells and pavement cells of the japanese eel. BMC Genom (2015) 16:1. doi: 10.1186/s12864-015-2271-0
33. Schneebauer G, Lindemann C, Drechsel V, Marohn L, Wysujack K, Santidrian E, et al. Swimming under elevated hydrostatic pressure increases glycolytic activity in gas gland cells of the European eel. PloS One. (2020) 15:e0239627. doi: 10.1371/journal.pone.0239627
34. Cirović S, Marković-Lipkovski J, Todorović J, Nesović-Ostojić J, Jović M, Ilić S, et al. Differential expression of KCNQ1 K+ channel in tubular cells of frog kidney. Eur J Histochem. (2010) 54:e7. doi: 10.4081/ejh.2010.e7
35. Bleich M, Warth R, Thiele I, Greger R. Three types of K+ channels in the basolateral membrane of the shark rectal gland tubule. Kidney Blood Press Res. (1998) 21:81.
36. Brennan EP, Morine MJ, Walsh DW, Roxburgh SA, Lindenmeyer MT, Brazil DP, et al. Next-generation sequencing identifies TGF-β1-associated gene expression profiles in renal epithelial cells reiterated in human diabetic nephropathy. Biochim Et Biophys Acta (BBA) - Mol Basis Dis. (2012) 1822:589–99. doi: 10.1016/j.bbadis.2012.01.008
37. Pitt GS, Matsui M, Cao C. Voltage-gated calcium channels in nonexcitable tissues. Annu Rev Physiol. (2021) 83:183–203. doi: 10.1146/annurev-physiol-031620-091043
38. Nilius B, Droogmans G. Ion channels and their functional role in vascular endothelium. Physiol Rev. (2001) 81:1415–59. doi: 10.1152/physrev.2001.81.4.1415
39. Fischer H. Function of proton channels in lung epithelia. Wiley Interdiscip Rev Membr Transp Signal. (2012) 1:247–58. doi: 10.1002/wmts.17
40. Shi W, Wymore RS, Wang H-S, Pan Z, Cohen IS, McKinnon D, et al. Identification of two nervous system-specific members of the erg potassium channel gene family. J Neurosci. (1997) 17:9423–32. doi: 10.1523/JNEUROSCI.17-24-09423.1997
41. Treutlein B, Brownfield DG, Wu AR, Neff NF, Mantalas GL, Espinoza FH, et al. Reconstructing lineage hierarchies of the distal lung epithelium using single-cell RNA-seq. Nature. (2014) 509:371–5. doi: 10.1038/nature13173
42. Chen L, Clark JZ, Nelson JW, Kaissling B, Ellison DH, Knepper MA. Renal-tubule epithelial cell nomenclature for single-cell RNA-sequencing studies. J Am Soc Nephrol. (2019) 30:1358–64. doi: 10.1681/ASN.2019040415
43. Abbott GW. KCNE1 and KCNE3: The yin and yang of voltage-gated K+ channel regulation. Gene. (2016) 576:1–13. doi: 10.1016/j.gene.2015.09.059
44. Hamilton KL, Devor DC. Basolateral membrane K+ channels in renal epithelial cells. Am J Physiol Renal Physiol. (2012) 302:F1069–81. doi: 10.1152/ajprenal.00646.2011
45. Demolombe S, Franco D, De Boer P, Kuperschmidt S, Roden D, Pereon Y, et al. Differential expression of KvLQT1 and its regulator IsK in mouse epithelia. Am J Physiology-Cell Physiol. (2001) 280:C359–72. doi: 10.1152/ajpcell.2001.280.2.C359
46. Siroky BJ, Kleene NK, Kleene SJ, Varnell CD, Comer RG, Liu J, et al. Primary cilia regulate the osmotic stress response of renal epithelial cells through TRPM3. Am J Physiology-Renal Physiol. (2017) 312:F791–805. doi: 10.1152/ajprenal.00465.2015
47. Rasmussen RN, Christensen KV, Holm R, Nielsen CU. Transcriptome analysis identifies activated signaling pathways and regulated ABC transporters and solute carriers after hyperosmotic stress in renal MDCK i cells. Genom (2019) 111:1557–65. doi: 10.1016/j.ygeno.2018.10.014
48. Park HJ, Fan Z, Bai Y, Ren Q, Rbaibi Y, Long KR, et al. Transcriptional programs driving shear stress-induced differentiation of kidney proximal tubule cells in culture. Front Physiol (2020) 11:587358. doi: 10.3389/fphys.2020.587358
49. Bleich M, Warth R. The very small-conductance K+ channel KVLQT1 and epithelial function. Pflugers Arch - Eur J Physiol. (2000) 440:202–6. doi: 10.1007/s004240000257
50. Ramsay J. The rectal complex in the larvae of Lepidoptera. Phil Trans R Soc Lond B. (1976) 274:203–26. doi: 10.1098/rstb.1976.0043
51. O’Donnell M. Insect excretory mechanisms. In: Advances in insect physiology. London, UK: Elsevier. (2008) p. 1–122.
52. Dow JAT, Maddrell SHP, Görtz A, Skaer NJV, Brogan S, Kaiser K. The malpighianC tubules of drosophila melanogaster : A novel phenotype for studies of fluid secretion and its control. J Exp Biol. (1994) 197:421–8. doi: 10.1242/jeb.197.1.421
53. Millet-Boureima C, Porras Marroquin J, Gamberi C. Modeling renal disease “On the fly”. BioMed Res Int. (2018) 2018:1–13. doi: 10.1155/2018/5697436
54. Phillips J. Comparative physiology of insect renal function. Am J Physiology-Regulatory Integr Comp Physiol. (1981) 241:R241–57. doi: 10.1152/ajpregu.1981.241.5.R241
55. Kolosov D, Piermarini PM, O'Donnell MJ. Malpighian tubules of Trichoplusia ni: recycling ions via gap junctions and switching between secretion and reabsorption of Na+ and K+ in the distal ileac plexus. J Exp Biol. (2018) 221:jeb172296. doi: 10.1242/jeb.172296
56. Kolosov D, O'Donnell MJ. Chapter Five - The Malpighian tubules and cryptonephric complex in lepidopteran larvae. In: Jurenka R, editor. Advances in insect physiology, vol. 56. London, UK: Academic Press (2019). p. 165–202.
57. Durant AC, Grieco Guardian E, Kolosov D, Donini A. The transcriptome of anal papillae of Aedes aEgypti reveals their importance in xenobiotic detoxification and adds significant knowledge on ion, water and ammonia transport mechanisms. J Insect Physiol. (2021) 132:104269. doi: 10.1016/j.jinsphys.2021.104269
58. Farrell S, Dates J, Ramirez N, Hausknecht-Buss H, Kolosov D. Voltage-gated ion channels are expressed in the Malpighian tubules and anal papillae of the yellow fever mosquito ( Aedes aEgypti ), and may regulate ion transport during salt and water imbalance. J Exp Biol. (2024) 227:jeb246486. doi: 10.1242/jeb.246486
59. Konopová B, Kolosov D, O'Donnell MJ. Water and ion transport across the eversible vesicles in the collophore of the springtail Orchesella cincta. J Exp Biol. (2019) 222:jeb200691. doi: 10.1242/jeb.200691
60. Carlson SD, Juang JL, Hilgers SL, Garment MB. Blood barriers of the insect. Annu Rev Entomol. (2000) 45:151–74. doi: 10.1146/annurev.ento.45.1.151
61. Davies SA, Overend G, Sebastian S, Cundall M, Cabrero P, Dow JA, et al. Immune and stress response 'cross-talk' in the Drosophila Malpighian tubule. J Insect Physiol. (2012) 58:488–97. doi: 10.1016/j.jinsphys.2012.01.008
62. Catterall WA. Structure and regulation of voltage-gated Ca 2+ Channels. Annu Rev Cell Dev Biol. (2000) 16:521–55. doi: 10.1146/annurev.cellbio.16.1.521
63. Cens T, Rousset M, Leyris J, Fesquet P, Charnet P. Voltage- and calcium-dependent inactivation in high voltage-gated Ca2+ channels. Prog Biophysics Mol Biol. (2006) 90:104–17. doi: 10.1016/j.pbiomolbio.2005.05.013
64. MacPherson MR, Pollock VP, Broderick KE, Kean L, O’Connell FC, Dow JAT. Davies SA. L-type calcium channels regulate epithelial fluid transport in Drosophila melanogaster. Am J Physiology-Cell Physiol. (2001) 280:C394–407. doi: 10.1152/ajpcell.2001.280.2.C394
65. Kolosov D, Leonard EM, O’Donnell MJ. Voltage-gated calcium channels regulate K+ transport in the Malpighian tubules of the larval cabbage looper, Trichoplusia ni. J Insect Physiol. (2021) 131:104230. doi: 10.1016/j.jinsphys.2021.104230
66. Senatore A, Raiss H, Le P. Physiology and evolution of voltage-gated calcium channels in early diverging animal phyla: cnidaria, placozoa, porifera and ctenophora. Front Physiol. (2016) 7:481. doi: 10.3389/fphys.2016.00481
67. Alexander SPH, Mathie A, Peters JA, Veale EL, Striessnig J, Kelly E, et al. THE CONCISE GUIDE TO PHARMACOLOGY 2019/20: ion channels. Br J Pharmacol. (2019) 176:S142–S228. doi: 10.1111/bph.14749
68. Varanita T, Angi B, Scattolini V, Szabo I. Kv1.3 K + Channel physiology assessed by genetic and pharmacological modulation. Physiology. (2023) 38:25–41. doi: 10.1152/physiol.00010.2022
69. Schroeder BC, Waldegger S, Fehr S, Bleich M, Warth R, Greger R, et al. A constitutively open potassium channel formed by KCNQ1 and KCNE3. Nature. (2000) 403:196–9. doi: 10.1038/35003200
70. Boonen B, Alpizar Y, Soldano A, Lopez Requena A, Hassan B, Voets T, et al. Broad sensitivity of drosophila melanogaster TRPA1 to noxious chemicals. Biophys J. (2016) 110:283a. doi: 10.1016/j.bpj.2015.11.1535
71. Voros O, Szilagyi O, Balajthy A, Somodi S, Panyi G, Hajdu P. The C-terminal HRET sequence of Kv1.3 regulates gating rather than targeting of Kv1.3 to the plasma membrane. Sci Rep. (2018) 8:5937. doi: 10.1038/s41598-018-24159-8
72. Cidad P, Jiménez-Pérez L, García-Arribas D, Miguel-Velado E, Tajada S, Ruiz-McDavitt C, et al. Kv1.3 channels can modulate cell proliferation during phenotypic switch by an ion-flux independent mechanism. ATVB (2012) 32:1299–307. doi: 10.1161/ATVBAHA.111.242727
73. Goldstein SAN, Bayliss DA, Kim D, Lesage F, Plant LD, Rajan S. International union of pharmacology. LV. Nomenclature and molecular relationships of two-P potassium channels. Pharmacol Rev. (2005) 57:527–40. doi: 10.1124/pr.57.4.12
74. Kubo Y, Adelman JP, Clapham DE, Jan LY, Karschin A, Kurachi Y, et al. International union of pharmacology. LIV. Nomenclature and molecular relationships of inwardly rectifying potassium channels. Pharmacol Rev. (2005) 57:509–26. doi: 10.1124/pr.57.4.11
75. Wei AD, Gutman GA, Aldrich R, Chandy KG, Grissmer S, Wulff H. International union of pharmacology. LII. Nomenclature and molecular relationships of calcium-activated potassium channels. Pharmacol Rev. (2005) 57:463–72. doi: 10.1124/pr.57.4.9
76. Kaczmarek LK, Aldrich RW, Chandy KG, Grissmer S, Wei AD, Wulff H. International union of basic and clinical pharmacology. C. Nomenclature and properties of calcium-activated and sodium-activated potassium channels. Pharmacol Rev. (2017) 69:1–11. doi: 10.1124/pr.116.012864
77. Rashid AJ, Dunn RJ. Sequence diversity of voltage-gated potassium channels in an electric fish. Mol Brain Res. (1998) 54:101–7. doi: 10.1016/S0169-328X(97)00328-8
78. Schwarz TL, Tempel BL, Papazian DM, Jan YN, Jan LY. Multiple potassium–channel components are produced by alternative splicing at the Shaker locus in Drosophila. Nature. (1988) 331:137–42. doi: 10.1038/331137a0
79. Pongs O. Molecular biology of voltage-dependent potassium channels. Physiol Rev. (1992) 72:S69–88. doi: 10.1152/physrev.1992.72.suppl_4.S69
80. Massengill JL, Smith MA, Son DI, O’Dowd DK. Differential expression of K 4-AP currents and kv3.1 potassium channel transcripts in cortical neurons that develop distinct firing phenotypes. J Neurosci. (1997) 17:3136–47. doi: 10.1523/JNEUROSCI.17-09-03136.1997
81. Zhao M-L, Wu C-F. Alterations in frequency coding and activity dependence of excitability in cultured neurons of drosophila memory mutants. J Neurosci. (1997) 17:2187–99. doi: 10.1523/JNEUROSCI.17-06-02187.1997
82. Butler DM, Ono JK, Chang T, McCaman RE, Barish ME. Mouse brain potassium channel ?1 subunit mRNA: Cloning and distribution during development. J Neurobiol. (1998) 34:135–50. doi: 10.1002/(SICI)1097-4695(19980205)34:2<135::AID-NEU4>3.0.CO;2-3
83. Nichols CG, Lopatin AN. INWARD RECTIFIER POTASSIUM CHANNELS. Annu Rev Physiol. (1997) 59:171–91. doi: 10.1146/annurev.physiol.59.1.171
84. Piermarini PM, Denton JS, Swale DR. The molecular physiology and toxicology of inward rectifier potassium channels in insects. Annu Rev Entomol. (2022) 67:125–42. doi: 10.1146/annurev-ento-062121-063338
85. Evans JM, Allan AK, Davies SA, Dow JAT. Sulphonylurea sensitivity and enriched expression implicate inward rectifier K+ channels in Drosophila melanogaster renal function. J Exp Biol. (2005) 208:3771–83. doi: 10.1242/jeb.01829
86. Wu Y, Baum M, Huang C-L, Rodan AR. Two inwardly rectifying potassium channels, Irk1 and Irk2 , play redundant roles in Drosophila renal tubule function. Am J Physiology-Regulatory Integr Comp Physiol. (2015) 309:R747–56. doi: 10.1152/ajpregu.00148.2015
87. Piermarini PM, Dunemann SM, Rouhier MF, Calkins TL, Raphemot R, Denton JS, et al. Localization and role of inward rectifier K+ channels in Malpighian tubules of the yellow fever mosquito Aedes aEgypti. Insect Biochem Mol Biol. (2015) 67:59–73. doi: 10.1016/j.ibmb.2015.06.006
88. Li Y, Piermarini PM, Esquivel CJ, Drumm HE, Schilkey FD, Hansen IA. RNA-Seq comparison of larval and adult malpighian tubules of the yellow fever mosquito aedes aEgypti reveals life stage-specific changes in renal function. Front Physiol. (2017) 8:283. doi: 10.3389/fphys.2017.00283
89. Kolosov D, Donly C, MacMillan H, O’Donnell MJ. Transcriptomic analysis of the Malpighian tubules of Trichoplusia ni: Clues to mechanisms for switching from ion secretion to ion reabsorption in the distal ileac plexus. J Insect Physiol. (2019) 112:73–89. doi: 10.1016/j.jinsphys.2018.12.005
90. Kroncke BM, Van Horn WD, Smith J, Kang C, Welch RC, Song Y, et al. Structural basis for KCNE3 modulation of potassium recycling in epithelia. Sci Adv. (2016) 2:e1501228. doi: 10.1126/sciadv.1501228
91. Barro-Soria R, Perez ME, Larsson HP. KCNE3 acts by promoting voltage sensor activation in KCNQ1. Proc Natl Acad Sci USA. (2015) 112:E7286–E7292. doi: 10.1073/pnas.1516238112
92. Sun J, MacKinnon R. Structural basis of human KCNQ1 modulation and gating. Cell. (2020) 180:340–347.e9. doi: 10.1016/j.cell.2019.12.003
93. Chintapalli VR, Wang J, Herzyk P, Davies SA, Dow JA. Data-mining the FlyAtlas online resource to identify core functional motifs across transporting epithelia. BMC Genomics. (2013) 14:518. doi: 10.1186/1471-2164-14-518
94. Kolosov D, O'Donnell MJ. Malpighian tubules of caterpillars: blending RNAseq and physiology to reveal regional functional diversity and novel epithelial ion transport control mechanisms. J Exp Biol. (2019) 222:jeb211623. doi: 10.1242/jeb.211623
95. Carrington J, Andersen MK, Brzezinski K, MacMillan HA. Hyperkalaemia, not apoptosis, accurately predicts insect chilling injury. Proc Biol Sci. (2020) 287:20201663. doi: 10.1098/rspb.2020.1663
96. Limmer S, Weiler A, Volkenhoff A, Babatz F, Klämbt C. The Drosophila blood-brain barrier: development and function of a glial endothelium. Front Neurosci. (2014) 8:365. doi: 10.3389/fnins.2014.00365
97. Pacey EK, O’Donnell MJ. Transport of H+, Na+ and K+ across the posterior midgut of blood-fed mosquitoes (Aedes aEgypti). J Insect Physiol. (2014) 61:42–50. doi: 10.1016/j.jinsphys.2013.12.008
98. Ianowski JP, O’Donnell MJ. Basolateral ion transport mechanisms during fluid secretion by Drosophila Malpighian tubules: Na+ recycling,Na+:K+:2Cl– cotransport and Cl– conductance. J Exp Biol. (2004) 207:2599–609. doi: 10.1242/jeb.01058
99. Yuan Y, Li M, Fan F, Qiu X. Comparative transcriptomic analysis of larval and adult Malpighian tubules from the cotton bollworm Helicoverpa armigera. Insect Sci. (2018) 25:991–1005. doi: 10.1111/1744-7917.12561
100. Duca OD, Nasirian A, Galperin V, Donini A. Pharmacological characterisation of apical Na+ and Cl– transport mechanisms of the anal papillae in the larval mosquito Aedes aEgypti. J Exp Biol. (2011) 214:3992–9. doi: 10.1242/jeb.063719
101. Beyenbach KW, Skaer H, Dow JAT. The developmental, molecular, and transport biology of malpighian tubules. Annu Rev Entomol. (2010) 55:351–74. doi: 10.1146/annurev-ento-112408-085512
102. Beyenbach KW, Piermarini PM. Transcellular and paracellular pathways of transepithelial fluid secretion in Malpighian (renal) tubules of the yellow fever mosquito Aedes aEgypti. Acta Physiologica. (2011) 202:387–407. doi: 10.1111/j.1748-1716.2010.02195.x
103. King GF, Escoubas P, Nicholson GM. Peptide toxins that selectively target insect Na V and Ca V channels. Channels. (2008) 2:100–16. doi: 10.4161/chan.2.2.6022
104. Yue L, Xu H. TRP channels in health and disease at a glance. J Cell Sci. (2021) 134:jcs258372. doi: 10.1242/jcs.258372
105. Venkatachalam K, Montell C. TRP channels. Annu Rev Biochem. (2007) 76:387–417. doi: 10.1146/annurev.biochem.75.103004.142819
106. Van Liefferinge E, Van Noten N, Degroote J, Vrolix G, Van Poucke M, Peelman L, et al. Expression of transient receptor potential ankyrin 1 and transient receptor potential vanilloid 1 in the gut of the peri-weaning pig is strongly dependent on age and intestinal site. Animals. (2020) 10:2417. doi: 10.3390/ani10122417
107. Gong J, Nirala NK, Chen J, Wang F, Gu P, Wen Q, et al. TrpA1 is a shear stress mechanosensing channel regulating intestinal stem cell proliferation in Drosophila. Sci Adv. (2023) 9:eadc9660. doi: 10.1126/sciadv.adc9660
108. Mao F, Lu W, Yang Y, Qiao X, Ye G, Huang J. Identification, characterization and expression analysis of TRP channel genes in the vegetable pest, pieris rapae. Insects. (2020) 11:192. doi: 10.3390/insects11030192
109. Su HA, Bai X, Zeng T, Lu YY, Qi YX. Identification, characterization and expression analysis of transient receptor potential channel genes in the oriental fruit fly, bactrocera dorsalis. BMC Genom (2018) 19:674. doi: 10.1186/s12864-018-5053-7
110. Tracey WD, Wilson RI, Laurent G, Benzer S. painless, a drosophila gene essential for nociception. Cell. (2003) 113:261–73. doi: 10.1016/S0092-8674(03)00272-1
111. Lee Y, Lee Y, Lee J, Bang S, Hyun S, Kang J, et al. Pyrexia is a new thermal transient receptor potential channel endowing tolerance to high temperatures in Drosophila melanogaster. Nat Genet. (2005) 37:305–10. doi: 10.1038/ng1513
112. Corfas RA, Vosshall LB. The cation channel TRPA1 tunes mosquito thermotaxis to host temperatures. eLife. (2015) 4:e11750. doi: 10.7554/eLife.11750
113. Sokabe T, Tominaga M. A temperature-sensitive TRP ion channel, Painless, functions as a noxious heat sensor in fruit flies. Commun Integr Biol. (2009) 2:170–3. doi: 10.4161/cib.7708
114. Wahl-Schott C, Biel M. HCN channels: Structure, cellular regulation and physiological function. Cell Mol Life Sci. (2009) 66:470–94. doi: 10.1007/s00018-008-8525-0
115. Donini A, Patrick ML, Bijelic G, Christensen RJ, Ianowski JP, Rheault MR, et al. Secretion of water and ions by malpighian tubules of larval mosquitoes: effects of diuretic factors, second messengers, and salinity. Physiol Biochem Zoology. (2006) 79:645–55. doi: 10.1086/501059
116. Ruiz-Sanchez E, O'Donnell MJ, Donini A. Secretion of Na(+), K(+) and fluid by the Malpighian (renal) tubule of the larval cabbage looper Trichoplusia ni (Lepidoptera: Noctuidae). J Insect Physiol. (2015) 82:92–8. doi: 10.1016/j.jinsphys.2015.09.007
117. Sajadi F, Curcuruto C, Al Dhaheri A, Paluzzi JV. Anti-diuretic action of a CAPA neuropeptide against a subset of diuretic hormones in the disease vector aedes aEgypti. J Exp Biol. (2018) 221:jeb177089. doi: 10.1242/jeb.177089
118. Plant TD. TRPs in mechanosensing and volume regulation. Handb Exp Pharmacol. (2014) 223:743–66. doi: 10.1007/978-3-319-05161-1_2
119. Jentsch TJ. VRACs and other ion channels and transporters in the regulation of cell volume and beyond. Nat Rev Mol Cell Biol. (2016) 17:293–307. doi: 10.1038/nrm.2016.29
120. Humphreys JM, Teixeira LR, Akella R, He H, Kannangara AR, Sekulski K, et al. Hydrostatic pressure sensing by WNK kinases. MBoC (2023) 34:ar109. doi: 10.1091/mbc.E23-03-0113
121. Feingold D, Knogler L, Starc T, Drapeau P, O’Donnell MJ, Nilson LA, et al. secCl is a cys-loop ion channel necessary for the chloride conductance that mediates hormone-induced fluid secretion in Drosophila. Sci Rep. (2019) 9:7464. doi: 10.1038/s41598-019-42849-9
122. Kolosov D, O'Donnell MJ. Mechanisms and regulation of chloride transport in the malpighian tubules of the larval cabbage looper trichoplusia ni. Insect Biochem Mol Biol (2020) 116:103263. doi: 10.1016/j.ibmb.2019.103263
Keywords: Malpighian tubules, salt and water balance, voltage-gated ion channels, ion transport, excretion
Citation: Dates J and Kolosov D (2024) Voltage-gated ion channels as novel regulators of epithelial ion transport in the osmoregulatory organs of insects. Front. Insect Sci. 4:1385895. doi: 10.3389/finsc.2024.1385895
Received: 14 February 2024; Accepted: 01 May 2024;
Published: 21 May 2024.
Edited by:
Nicholas Teets, University of Kentucky, United StatesReviewed by:
Mads Kuhlmann Andersen, Carleton University, CanadaCopyright © 2024 Dates and Kolosov. This is an open-access article distributed under the terms of the Creative Commons Attribution License (CC BY). The use, distribution or reproduction in other forums is permitted, provided the original author(s) and the copyright owner(s) are credited and that the original publication in this journal is cited, in accordance with accepted academic practice. No use, distribution or reproduction is permitted which does not comply with these terms.
*Correspondence: Dennis Kolosov, ZGtvbG9zb3ZAY3N1c20uZWR1