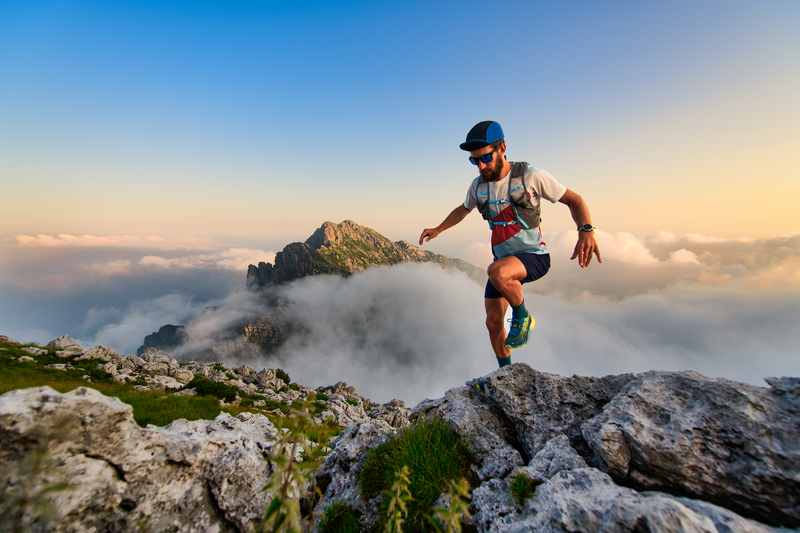
94% of researchers rate our articles as excellent or good
Learn more about the work of our research integrity team to safeguard the quality of each article we publish.
Find out more
MINI REVIEW article
Front. Immunol. , 22 January 2025
Sec. Cancer Immunity and Immunotherapy
Volume 16 - 2025 | https://doi.org/10.3389/fimmu.2025.1541467
This article is part of the Research Topic Harnessing Big Data for Precision Medicine: Revolutionizing Diagnosis and Treatment Strategies View all 33 articles
Glioblastoma (GBM) cells leverage complex endogenous and environmental regulatory mechanisms to drive proliferation, invasion, and metastasis. Tumor immune evasion, facilitated by a multifactorial network, poses a significant challenge to effective therapy, as evidenced by the limited clinical benefits of monotherapies, highlighting the adaptive nature of immune evasion. This review explores glioblastoma’s immune evasion mechanisms, the role of ICIs in the tumor microenvironment, and recent clinical advancements, offering theoretical insights and directions for monotherapy and combination therapy in glioblastoma management.
Glioblastoma, the most common primary tumor of the central nervous system (CNS), originate from glial cells and account for over 80% of brain tumors. According to the World Health Organization (WHO), gliomas are categorized into four grades, with grade IV, known as glioblastoma, comprising 60–70% of all gliomas. GBM, the most prevalent primary malignant CNS tumor, manifests with symptoms such as progressive neurological deficits, headaches, nausea, vomiting, cognitive impairment, and seizures, depending on tumor stage and location. The incidence of GBM is approximately 3.21 cases per 100,000 individuals, with prevalence increasing with age and a median diagnosis age of 65 years. Males are more commonly affected than females, with a male-to-female ratio of 1.28:1 (1).
Despite advancements in surgical resection, radiotherapy, and temozolomide (TMZ)-based chemotherapy, GBM prognosis remains poor, with a median survival of 15 months and a five-year survival rate of 9.8% (2, 3). Challenges include its high migratory and invasive properties, preventing complete resection, and frequent localized recurrence near resected tissue, leading to patient mortality (4). Anti-angiogenic agents like bevacizumab and tumor-treating fields (TTF) have shown limited success in prolonging survival, emphasizing the need for innovative therapies (5, 6). Immunotherapy, a rapidly advancing field, aims to enhance immune responses to suppress tumor progression (7–12). However, GBM’s unique immune evasion mechanisms present significant obstacles. Immunotherapeutic approaches, including immune checkpoint inhibitors (ICIs), cytokine-based therapies, dendritic cell vaccines, oncolytic virotherapy, CAR-T cell therapy, and tumor-associated macrophage modulation, have shown promise. ICIs, successful in cancers like melanoma and non-small cell lung cancer, remain in early-stage research for GBM due to the CNS’s unique anatomy and immune microenvironment (13–17).
The CNS has traditionally been considered immune-privileged due to the blood-brain barrier (BBB), lack of lymphatic drainage, and limited immune cell presence, which restrict immune functions (18). However, recent studies have challenged this view, showing that glioma progression and inflammation increase BBB permeability, allowing immune cells and biomolecules to access the CNS (19–22). This disruption facilitates cytokine accumulation, exacerbating the immunosuppressive tumor microenvironment and reducing immunotherapy efficacy (23). Additionally, the discovery of functional lymphatic vessels in dural sinuses and the APC-like function of microglia and astrocytes has highlighted CNS-peripheral immune interactions (24, 25). Tumor-specific lymphocytes infiltrate the CNS through the BBB and choroid plexus, exerting cytotoxic effects on gliomas (24, 26, 27). Advances in nano-molecules and immune-targeting drugs have improved BBB penetration, enhancing intracranial tumor therapies (28). These findings advance glioma immunotherapy strategies.
GBM is characterized by its potent immune evasion capabilities, shaped by the brain’s unique immunological environment and a complex tumor microenvironment (TME). TME includes peripheral immune cells such as myeloid-derived suppressor cells (MDSCs) (29), natural killer (NK) cells, macrophages (30), neutrophils, CD4+ helper T cells (Th), CD8+ cytotoxic T lymphocytes (CTLs) (31), and regulatory T cells (Tregs), which influence tumor progression, recurrence, and resistance by modulating inflammatory responses (32). These immune cells are controlled by complex signaling networks, transforming into collaborators of tumor immune evasion and impairing immune surveillance (Figure 1).
GBM immune evasion mechanisms include: (I) downregulation of MHC-I molecules on tumor and antigen-presenting cells, limiting immune recognition; (II) activation of immune checkpoints like CTLA-4, PD-1/PD-L1, and LAG-3, suppressing immune responses; (III) secretion of immunosuppressive biomolecules such as TGF-β, IL-10, prostaglandin E2, and VEGF, creating a suppressive cytokine milieu; and (IV) infiltration of immunosuppressive cells like Tregs, M2-polarized tumor-associated macrophages (TAMs), and MDSCs, enhancing immunosuppression (33). These mechanisms are interdependent, amplifying immunosuppression, such as Tregs secreting TGF-β to suppress dendritic cell maturation and expressing CTLA-4 to inhibit effector T cell activation (34, 35). T-cell exhaustion also plays a critical role in GBM progression. This phenomenon, marked by the loss of effector functions and sustained expression of inhibitory receptors (e.g., PD-1, TIM-3, LAG-3), is induced by chronic antigen exposure and the immunosuppressive TME (36). Engagement of PD-1 with PD-L1 on tumor cells inhibits T-cell receptor (TCR) signaling, reducing cytokine production and cytotoxicity. Similarly, interactions between TIM-3 and galectin-9, and LAG-3 with MHC class II molecules, further suppress T-cell activity and contribute to exhaustion. The simultaneous expression of multiple immune checkpoints creates an environment that promotes tumor immune evasion and supports GBM growth, limiting the effectiveness of immunotherapies and contributing to GBM’s resilience against immune clearance.
Exogenous factors such as prolonged use of cytotoxic chemotherapy and immunosuppressive drugs, particularly glucocorticoids, exacerbate immune deficiencies in GBM patients, especially the elderly, leading to passive systemic immune suppression (37). However, studies in mouse models suggest that the CNS’s unique immune features may alleviate some glucocorticoid-induced effects on local tumor immunotherapy, offering insights into optimizing treatments, though human validation is needed (38). These immunological insights highlight the potential for targeted immunotherapies to reverse immunosuppression and induce durable anti-tumor immune responses, offering promising avenues for improving GBM treatment outcomes.
The human immune system maintains equilibrium by activating immune cells through antigen and co-stimulatory signals while terminating responses via co-inhibitory receptors to prevent tissue damage. Tumor cells exploit these immune checkpoints (ICs) to evade surveillance, a key mechanism of immune escape (39). IC inhibitors, targeting these pathways, modulate immune cells in the tumor microenvironment rather than acting directly on tumor cells, making them critical tools in cancer immunotherapy with broad clinical applications (40).
CTLA-4 is expressed on activated T cells and Tregs that suppresses T cell activation by binding to CD80/CD86 and enhances Treg activity in the tumor microenvironment. Preclinical glioma studies show that CTLA-4 inhibitors promote CD4+ T cell proliferation and reduce Treg/CD4+ T cell ratios, correlating CTLA-4 expression with poor prognosis in glioblastoma (41, 42). Approved by the FDA for melanoma, ipilimumab and tremelimumab are under investigation for GBM. A Phase III trial (NCT02017717) comparing Ipilimumab and Nivolumab to bevacizumab reported partial responses and disease stabilization but was suspended due to subsequent failures (43, 44). Retrospective data links low CTLA-4 expression to improved survival (45). Interestingly, CTLA-4 blockade loses efficacy in CD4+ T cell-depleted mice, suggesting its anti-tumor effects rely on CD4+ T cell-mediated modulation of dendritic cells and microglia, offering new insights into its role in GBM treatment (46).
Programmed cell death protein 1 (PD-1) and its ligands, PD-L1/PD-L2, suppress T cell activation and cytokine production, marking a key mechanism of immune evasion. PD-1 expression in GBM correlates with T cell exhaustion, reduced IFN-γ production, and poor survival (47–50). Tumor PD-L1 expression inhibits T cell activity and promotes resistance to cytotoxicity, accelerating glioblastoma progression (51, 52). Wild-type IDH glioblastomas exhibit higher PD-L1 levels than mutant forms, further linking the pathway to tumor grade and immune modulation (53, 54).
FDA-approved PD-1 inhibitors, such as Nivolumab and Pembrolizumab, have demonstrated efficacy in various cancers. In GBM, neoadjuvant PD-1 blockade enhances survival and local immune responses, though the microenvironment remains dominated by immunosuppressive myeloid cells (55, 56). Among 61 clinical trials, a completed study (NCT02337491) combining Pembrolizumab and Bevacizumab improved progression-free survival but increased toxicity (Table 1). Another (NCT02550249) revealed modest survival benefits with neoadjuvant Nivolumab but significantly boosted immune responses (57, 58). Trials on PD-L1 inhibitors like Avelumab, Durvalumab, and Atezolizumab continue, with most results pending, emphasizing the need for further optimization in this domain.
TIGIT and CD96 are critical co-inhibitory receptors in tumor immune regulation. CD96, part of the immunoglobulin superfamily, is a promising immunotherapy target due to its regulatory roles in NK and CD8+ T cell activity and influence on NK cell adhesion and migration. CD96 mitigates immune reactivation after PD-1/PD-L1 blockade, with elevated expression correlating with aggressive molecular phenotypes like IDH wild-type and mesenchymal subtypes. In GBM, CD96 co-expressed with PD-1 on CD8+ T cells indirectly promote tumor growth via enhanced IFN-γ secretion. Combined targeting of CD96, PD-1, and TIGIT has shown significant antitumor efficacy (59). Bioinformatics analyses reveal high CD96 mRNA expression correlates with invasiveness and poor prognosis in IDH wild-type GBM, underscoring its role as an independent prognostic factor (60). Similarly, TIGIT facilitates immune evasion by competing with CD115 for CD226 binding, while its ligand, CD155, overexpressed in GBM, enhances tumor migration and invasion. Monotherapy targeting TIGIT shows limited efficacy, but combining anti-TIGIT with anti-PD-1 significantly improves survival and immune response in GBM models (61). The TIGIT/CD155 axis also correlates with lower survival in lower-grade gliomas, making it a promising therapeutic target for GBM (61).
TIM-3, an inhibitory checkpoint protein, marks T cell dysfunction in cancer and is highly expressed in tumor-infiltrating lymphocytes. It binds ligands such as galectin-9 (Gal-9), HMGB1, and CEACAM1, with the TIM-3/Gal-9 interaction suppressing Th1 responses and promoting Treg development. In GBM and IDH wild-type gliomas, TIM-3 is highly expressed and correlates with poor prognosis (62). TIM-3 knockout enhances NK cell cytotoxicity against GBM, supporting dual checkpoint blockade strategies for therapy (63). TIM-3 also contributes to chemoresistance in high-grade GBM, as silencing TIM-3 expression sensitizes tumor cells to temozolomide while inducing apoptosis (64). Dual TIM-3 and PD-1 blockade restores T cell function and demonstrates superior antitumor efficacy in preclinical models, with several anti-TIM-3 antibodies currently in clinical trials (65). These findings highlight TIM-3 as a critical target for immunotherapy in high-grade GBM.
Lymphocyte activation gene-3 (LAG-3) is a type I transmembrane protein structurally similar to CD4 and functions as an inhibitory co-receptor critical in autoimmune diseases, tumor immunity, and anti-infective immunity. As a second-generation immune checkpoint inhibitor target, LAG-3 represents a promising therapeutic direction after PD-1. It is minimally expressed on resting T cells but significantly upregulated on CD4 and CD8 T cells upon antigen stimulation and is closely tied to Treg homeostasis and function. LAG-3 often co-expresses with PD-1 on exhausted T cells, making it a key target in immunotherapy, particularly in combination with PD-1/PD-L1 inhibitors. As an early marker of T-cell exhaustion, LAG-3 inhibits T-cell activation by disrupting CD4-MHC II interactions and intracellular signaling (66) and directly suppresses CD8 T-cell functions.
In GBM, LAG-3 expression is associated with pathological subtypes, predominantly observed in high-grade GBM but absent in WHO grade II-III GBM. Preclinical studies showed that both LAG-3 inhibitor monotherapy and combination therapy with PD-1 inhibitors significantly prolonged survival in GBM mouse models. In human GBM, LAG-3 is mainly found on tumor-infiltrating lymphocytes (TILs) and perivascular lymphocytes (67). Despite promising preclinical data, the therapeutic implications in GBM remain uncertain, with clinical trials on the LAG-3 inhibitor BMS-986016 ongoing but unpublished.
Indoleamine 2,3-dioxygenase (IDO), a tryptophan-metabolizing enzyme, plays a key role in glioma immune evasion by depleting tryptophan, essential for T-cell function, and promoting Treg infiltration (68, 69), This dual mechanism suppresses effector T-cell activity and facilitates glioma progression, with studies linking higher IDO expression to increased tumor malignancy and worse prognosis (69, 70). IDO inhibitors, including indoximod and PF-06840003, are under clinical investigation, with three trials currently registered. While one trial is completed, results are pending. Preliminary data suggest potential for IDO-targeted glioblastoma therapies, but further studies are needed to confirm their clinical efficacy and mechanisms.
While monotherapy with immune checkpoint inhibitors (ICIs) demonstrates limited efficacy in gliomas, combination strategies show significant promise. Ferroptosis, for example, plays a crucial role in reshaping the immunosuppressive microenvironment. The combination of ferrostatin-1 and anti-PD-L1 antibodies significantly prolonged survival, reduced tumor volume, and enhanced T-cell-mediated antitumor activity in GBM mouse models (71). Similarly, targeting PD-L1 expression, linked to glioblastoma cellular metabolism, with hexokinase inhibition and anti-PD-1 therapy significantly reduced immune evasion (72). Neutralizing IL-8 has been proposed as an adjunct to anti-PD-L1 therapy, offering further therapeutic potential (73). Approved ICIs include two anti-PD-1 antibodies (nivolumab and pembrolizumab) and three anti-PD-L1 antibodies (atezolizumab, avelumab, durvalumab) for solid tumors. Among glioblastoma patients, nivolumab has shown survival benefits in primary cases but limited efficacy in recurrent gliomas (58).
Dual immune checkpoint blockade holds potential for glioma therapy optimization. The FDA-approved combination of nivolumab and ipilimumab for hepatocellular carcinoma has shown promising preclinical results in glioma models, where CTLA-4 and PD-1 blockade significantly extended survival, with 74% of mice achieving long-term responses (74, 75). Combining CTLA-4 blockade with IL-12 enhanced TH1 polarization, achieving complete remission in glioma-bearing mice (76). Despite some durable responses, clinical efficacy remains constrained by immune-related adverse events, affecting 90% of patients and causing high-grade toxicities, particularly with high-dose ipilimumab. Only 20% of patients achieved stable disease at 12 weeks, with most discontinuing treatment due to progression or toxicity. A median follow-up revealed over half succumbed to the disease within 27.5 months (77), emphasizing the need for refined patient selection to improve outcomes and minimize adverse effects.
IC molecules, including PD-1, TIM-3, and LAG-3, regulate immune responses and are often co-expressed in various tumors, contributing to T-cell exhaustion and impaired function. Studies show that dual blockade of IC molecules restores T-cell function more effectively than single-agent inhibition, underscoring the synergistic potential of combination therapies (67, 78–81). For example, PD-1 and IDO induce CTLA-4 expression in Tregs, while CTLA-4 binding to CD80/CD86 upregulates IDO, highlighting interactions between pathways (82–84). Clinical trials increasingly adopt multi-ICI combinations to counteract T-cell dysfunction, though their efficacy in gliomas awaits further validation (82–84).
Conventional cancer treatments—surgical resection, chemotherapy, and radiotherapy—often leave residual tumor cells that can lead to relapse and metastasis due to their resistance and extensive infiltration into surrounding tissues. To address this, recent strategies integrate immune checkpoint (IC) inhibitors with these traditional modalities, enhancing therapeutic efficacy through synergistic mechanisms. For instance, in brainstem glioma patients, the combination of the PD-1 inhibitor nivolumab with repeated radiotherapy has significantly improved overall survival, likely due to radiation-induced tumor antigen release that promotes immune cell infiltration into the tumor microenvironment (85, 86). Similarly, in murine glioma models, the co-administration of temozolomide with PD-1 or IDO inhibitors has markedly extended survival and inhibited tumor growth compared to monotherapy (87, 88). Despite these promising outcomes, further research is necessary to determine optimal dosing schedules and to evaluate potential additive toxicities when integrating IC inhibitors with conventional treatments.
Additionally, combining IC inhibitors with anti-angiogenic therapies represents a pivotal advancement in cancer treatment. Tumor-induced aberrant angiogenesis, primarily driven by pro-angiogenic factors like VEGF, creates a hypoxic microenvironment that suppresses anti-tumor immune responses and facilitates pro-tumor inflammatory cell infiltration, thereby limiting the efficacy of IC inhibitors (89). Preclinical studies have demonstrated that VEGF-blocking antibodies can downregulate multiple IC molecules, revealing the interplay between angiogenesis and immune suppression. The development of anti-angiogenic agents such as recombinant human endostatin has shown enhanced outcomes when used alongside PD-1 inhibitors in tumor models (90–92). Clinical trials are currently exploring combinations of IC inhibitors with anti-angiogenic drugs like bevacizumab, adatinib, axitinib, and endostar, which have shown preliminary efficacy in various solid tumors, including renal cell carcinoma, breast cancer, non-small cell lung cancer, and hepatocellular carcinoma. However, their effectiveness in glioma patients remains to be fully elucidated, necessitating further investigation to optimize these combination therapies for maximal clinical benefit.
Advancements in ICB therapy now encompass innovative approaches beyond traditional blocking antibodies. MicroRNAs (miRNAs) (93), such as miR-138 and miR-34a, have emerged as promising modulators of IC expression (94, 95). For instance, miR-138 suppresses CTLA-4 and PD-1 in T cells, while miR-34a downregulates PD-L1, enhancing anti-tumor immunity (96, 97). Additionally, targeting upstream regulators like PTEN and CDK5, which influence IC expression, can potentiate the efficacy of IC inhibitors (98, 99). Integrating these strategies with conventional treatments, such as chemotherapy and radiotherapy, may overcome resistance mechanisms and improve therapeutic outcomes. For example, combining miR-34a mimics with temozolomide has demonstrated synergistic effects in preclinical models, resulting in increased apoptosis and reduced tumor growth.
Compared to traditional therapies, these emerging strategies offer enhanced specificity and the ability to target multiple oncogenic pathways simultaneously, reducing the likelihood of resistance and minimizing collateral damage to healthy tissues. Furthermore, personalized treatment regimens based on miRNA profiles and upstream regulator status can tailor therapies to individual tumor characteristics, improving efficacy and reducing adverse effects. This integration not only complements existing modalities but also addresses the molecular mechanisms underlying glioblastoma’s immune evasion, providing a more robust and sustained anti-tumor response (100).
Tumor cells enhance their proliferative, invasive, and metastatic capabilities through complex endogenous and environmental regulatory mechanisms, with immune evasion being a multifactorial process involving extensive regulatory networks. Monotherapies targeting single pathways, such as PD-1 inhibitors like Nivolumab or Pembrolizumab, have shown limited success in prolonging progression-free survival in recurrent high-grade gliomas. This underscores the adaptability and diversity of tumor immune evasion strategies, indicating that single immune checkpoint blockade is often insufficient.
Consequently, multi-target combination therapies have become a focal point in cancer immunotherapy. These multidimensional approaches integrate multiple immune regulatory mechanisms to effectively counteract the intricate immune evasion networks employed by tumors. Strategies such as combining immune checkpoint inhibitors with metabolic regulation targets, utilizing gene editing technologies to modulate immune cell functions, and applying single-cell multi-omics to analyze immune microenvironment changes post-treatment hold significant promise. These emerging combination therapies are poised to enhance therapeutic efficacy and offer breakthrough options for treating aggressive cancers, including high-grade gliomas.
HS: Writing – original draft. YP: Writing – original draft. YW: Writing – original draft. XZ: Writing – original draft, Writing – review & editing.
The author(s) declare that financial support was received for the research, authorship, and/or publication of this article. This study was supported by the research project of the Science and Technology Bureau of Yongchuan District, Chongqing (Grant No. 2023yc-jckx20058): Experimental Study on the Regulation of Malignant Biological Characteristics of High-Grade Glioma Cells by MiR-325-3p.
All authors thank Yongchuan Hospital of Chongqing Medical University and the First Affiliated Hospital of Gannan Medical University for their support.
The authors declare that the research was conducted in the absence of any commercial or financial relationships that could be construed as a potential conflict of interest.
The author(s) declare that no Generative AI was used in the creation of this manuscript.
All claims expressed in this article are solely those of the authors and do not necessarily represent those of their affiliated organizations, or those of the publisher, the editors and the reviewers. Any product that may be evaluated in this article, or claim that may be made by its manufacturer, is not guaranteed or endorsed by the publisher.
1. Tan AC, Ashley DM, Lopez GY, Malinzak M, Friedman HS, Khasraw M. Management of glioblastoma: State of the art and future directions. CA Cancer J Clin. (2020) 70:299–312. doi: 10.3322/caac.21613
2. Stupp R, Hegi ME, Mason WP, van den Bent MJ, Taphoorn MJ, Janzer RC, et al. Effects of radiotherapy with concomitant and adjuvant temozolomide versus radiotherapy alone on survival in glioblastoma in a randomised phase III study: 5-year analysis of the EORTC-NCIC trial. Lancet Oncol. (2009) 10:459–66. doi: 10.1016/S1470-2045(09)70025-7
3. Tran B, Rosenthal MA. Survival comparison between glioblastoma multiforme and other incurable cancers. J Clin Neurosci. (2010) 17:417–21. doi: 10.1016/j.jocn.2009.09.004
4. Wen J, Chen W, Zhu Y, Zhang P. Clinical features associated with the efficacy of chemotherapy in patients with glioblastoma (GBM): a surveillance, epidemiology, and end results (SEER) analysis. BMC Cancer. (2021) 21:81. doi: 10.1186/s12885-021-07800-0
5. Stupp R, Wong ET, Kanner AA, Steinberg D, Engelhard H, Heidecke V, et al. NovoTTF-100A versus physician’s choice chemotherapy in recurrent glioblastoma: a randomised phase III trial of a novel treatment modality. Eur J Cancer. (2012) 48:2192–202. doi: 10.1016/j.ejca.2012.04.011
6. Friedman HS, Prados MD, Wen PY, Mikkelsen T, Schiff D, Abrey LE, et al. Bevacizumab alone and in combination with irinotecan in recurrent glioblastoma. J Clin Oncol. (2023) 41:4945–52. doi: 10.1200/JCO.22.02772
7. Wang Y, Ma L, He J, Gu H, Zhu H. Identification of cancer stem cell-related genes through single cells and machine learning for predicting prostate cancer prognosis and immunotherapy. Front Immunol. (2024) 15:1464698. doi: 10.3389/fimmu.2024.1464698
8. Sun Z, Wang J, Fan Z, Yang Y, Meng X, Ma Z, et al. Investigating the prognostic role of lncRNAs associated with disulfidptosis-related genes in clear cell renal cell carcinoma. J Gene Med. (2024) 26:e3608. doi: 10.1002/jgm.v26.1
9. Li C, Wirth U, Schardey J, Ehrlich-Treuenstatt VV, Bazhin AV, Werner J, et al. An immune-related gene prognostic index for predicting prognosis in patients with colorectal cancer. Front Immunol. (2023) 14:1156488. doi: 10.3389/fimmu.2023.1156488
10. Wang Y, Wang J, He J, Ji B, Pang Z, Wang J, et al. Comprehensive analysis of PRPF19 immune infiltrates, DNA methylation, senescence-associated secretory phenotype and ceRNA network in bladder cancer. Front Immunol. (2023) 14:1289198. doi: 10.3389/fimmu.2023.1289198
11. Liu T, Li C, Zhang J, Hu H, Li C. Unveiling efferocytosis-related signatures through the integration of single-cell analysis and machine learning: a predictive framework for prognosis and immunotherapy response in hepatocellular carcinoma. Front Immunol. (2023) 14:1237350. doi: 10.3389/fimmu.2023.1237350
12. Wang Y, He J, Zhao Q, Bo J, Zhou Y, Sun H, et al. Evaluating the predictive value of angiogenesis-related genes for prognosis and immunotherapy response in prostate adenocarcinoma using machine learning and experimental approaches. Front Immunol. (2024) 15:1416914. doi: 10.3389/fimmu.2024.1416914
13. Hodi FS, O’Day SJ, McDermott DF, Weber RW, Sosman JA, Haanen JB, et al. Improved survival with ipilimumab in patients with metastatic melanoma. N Engl J Med. (2010) 363:711–23. doi: 10.1056/NEJMoa1003466
14. Brahmer J, Reckamp KL, Baas P, Crino L, Eberhardt WE, Poddubskaya E, et al. Nivolumab versus docetaxel in advanced squamous-cell non-small-cell lung cancer. N Engl J Med. (2015) 373:123–35. doi: 10.1056/NEJMoa1504627
15. Motzer RJ, Escudier B, McDermott DF, George S, Hammers HJ, Srinivas S, et al. Nivolumab versus everolimus in advanced renal-cell carcinoma. N Engl J Med. (2015) 373:1803–13. doi: 10.1056/NEJMoa1510665
16. Balar AV, Galsky MD, Rosenberg JE, Powles T, Petrylak DP, Bellmunt J, et al. Atezolizumab as first-line treatment in cisplatin-ineligible patients with locally advanced and metastatic urothelial carcinoma: a single-arm, multicentre, phase 2 trial. Lancet. (2017) 389:67–76. doi: 10.1016/S0140-6736(16)32455-2
17. Zhu C, Sun Z, Wang J, Meng X, Ma Z, Guo R, et al. Exploring oncogenes for renal clear cell carcinoma based on G protein-coupled receptor-associated genes. Discovery Oncol. (2023) 14:182. doi: 10.1007/s12672-023-00795-z
18. Sehgal A, Berger MS. Basic concepts of immunology and neuroimmunology. Neurosurg Focus. (2000) 9:e1. doi: 10.3171/foc.2000.9.6.2
19. Becher B, Bechmann I, Greter M. Antigen presentation in autoimmunity and CNS inflammation: how T lymphocytes recognize the brain. J Mol Med (Berl). (2006) 84:532–43. doi: 10.1007/s00109-006-0065-1
20. Alghamri MS, McClellan BL, Hartlage CS, Haase S, Faisal SM, Thalla R, et al. Targeting neuroinflammation in brain cancer: uncovering mechanisms, pharmacological targets, and neuropharmaceutical developments. Front Pharmacol. (2021) 12:680021. doi: 10.3389/fphar.2021.680021
21. Conq J, Joudiou N, Preat V, Gallez B. Exploring the impact of irradiation on glioblastoma blood-brain-barrier permeability: insights from dynamic-contrast-enhanced-MRI and histological analysis. Biomedicines. (2024) 12:1091. doi: 10.3390/biomedicines12051091
22. Pinkiewicz M, Pinkiewicz M, Walecki J, Zaczynski A, Zawadzki M. Breaking barriers in neuro-oncology: A scoping literature review on invasive and non-invasive techniques for blood-brain barrier disruption. Cancers (Basel). (2024) 16:236. doi: 10.3390/cancers16010236
23. Franzese O. Tumor microenvironment drives the cross-talk between co-stimulatory and inhibitory molecules in tumor-infiltrating lymphocytes: implications for optimizing immunotherapy outcomes. Int J Mol Sci. (2024) 25:12848. doi: 10.3390/ijms252312848
24. Louveau A, Smirnov I, Keyes TJ, Eccles JD, Rouhani SJ, Peske JD, et al. Structural and functional features of central nervous system lymphatic vessels. Nature. (2015) 523:337–41. doi: 10.1038/nature14432
25. Prins RM, Soto H, Konkankit V, Odesa SK, Eskin A, Yong WH, et al. Gene expression profile correlates with T-cell infiltration and relative survival in glioblastoma patients vaccinated with dendritic cell immunotherapy. Clin Cancer Res. (2011) 17:1603–15. doi: 10.1158/1078-0432.CCR-10-2563
26. He G, Lu T, Lu B, Xiao D, Yin J, Liu X, et al. Perivascular and perineural extension of formed and soluble blood elements in an intracerebral hemorrhage rat model. Brain Res. (2012) 1451:10–8. doi: 10.1016/j.brainres.2012.02.069
27. Ransohoff RM, Engelhardt B. The anatomical and cellular basis of immune surveillance in the central nervous system. Nat Rev Immunol. (2012) 12:623–35. doi: 10.1038/nri3265
28. Galstyan A, Markman JL, Shatalova ES, Chiechi A, Korman AJ, Patil R, et al. Blood-brain barrier permeable nano immunoconjugates induce local immune responses for glioma therapy. Nat Commun. (2019) 10:3850. doi: 10.1038/s41467-019-11719-3
29. Deng Y, Shi M, Yi L, Naveed Khan M, Xia Z, Li X. Eliminating a barrier: Aiming at VISTA, reversing MDSC-mediated T cell suppression in the tumor microenvironment. Heliyon. (2024) 10:e37060. doi: 10.1016/j.heliyon.2024.e37060
30. Zhai X, Zhang H, Xia Z, Liu M, Du G, Jiang Z, et al. Oxytocin alleviates liver fibrosis via hepatic macrophages. JHEP Rep. (2024) 6:101032. doi: 10.1016/j.jhepr.2024.101032
31. Xia Z, Chen S, He M, Li B, Deng Y, Yi L, et al. Editorial: Targeting metabolism to activate T cells and enhance the efficacy of checkpoint blockade immunotherapy in solid tumors. Front Immunol. (2023) 14:1247178. doi: 10.3389/fimmu.2023.1247178
32. Gieryng A, Pszczolkowska D, Walentynowicz KA, Rajan WD, Kaminska B. Immune microenvironment of gliomas. Lab Invest. (2017) 97:498–518. doi: 10.1038/labinvest.2017.19
33. Nduom EK, Weller M, Heimberger AB. Immunosuppressive mechanisms in glioblastoma. Neuro Oncol. (2015) 17 Suppl 7:vii9–vii14. doi: 10.1093/neuonc/nov151
34. Platten M, Wick W, Weller M. Malignant glioma biology: role for TGF-beta in growth, motility, angiogenesis, and immune escape. Microsc Res Tech. (2001) 52:401–10. doi: 10.1002/1097-0029(20010215)52:4<401::AID-JEMT1025>3.0.CO;2-C
35. Walker LS, Sansom DM. Confusing signals: recent progress in CTLA-4 biology. Trends Immunol. (2015) 36:63–70. doi: 10.1016/j.it.2014.12.001
36. Xie H, Xi X, Lei T, Liu H, Xia Z. CD8(+) T cell exhaustion in the tumor microenvironment of breast cancer. Front Immunol. (2024) 15:1507283. doi: 10.3389/fimmu.2024.1507283
37. Heimberger AB, Sampson JH. Immunotherapy coming of age: what will it take to make it standard of care for glioblastoma? Neuro Oncol. (2011) 13:3–13. doi: 10.1093/neuonc/noq169
38. Maxwell R, Luksik AS, Garzon-Muvdi T, Hung AL, Kim ES, Wu A, et al. Contrasting impact of corticosteroids on anti-PD-1 immunotherapy efficacy for tumor histologies located within or outside the central nervous system. Oncoimmunology. (2018) 7:e1500108. doi: 10.1080/2162402X.2018.1500108
39. Yang C, Geng H, Yang X, Ji S, Liu Z, Feng H, et al. Targeting the immune privilege of tumor-initiating cells to enhance cancer immunotherapy. Cancer Cell. (2024) 42:2064–81. doi: 10.1016/j.ccell.2024.10.008
40. Tan AC, Heimberger AB, Khasraw M. Immune checkpoint inhibitors in gliomas. Curr Oncol Rep. (2017) 19:23. doi: 10.1007/s11912-017-0586-5
41. Fecci PE, Ochiai H, Mitchell DA, Grossi PM, Sweeney AE, Archer GE, et al. Systemic CTLA-4 blockade ameliorates glioma-induced changes to the CD4+ T cell compartment without affecting regulatory T-cell function. Clin Cancer Res. (2007) 13:2158–67. doi: 10.1158/1078-0432.CCR-06-2070
42. Fong B, Jin R, Wang X, Safaee M, Lisiero DN, Yang I, et al. Monitoring of regulatory T cell frequencies and expression of CTLA-4 on T cells, before and after DC vaccination, can predict survival in GBM patients. PloS One. (2012) 7:e32614. doi: 10.1371/journal.pone.0032614
43. Reardon DA, Sampson JH, Sahebjam S, Lim M, Baehring JM, Vlahovic G, et al. Safety and activity of nivolumab (nivo) monotherapy and nivo in combination with ipilimumab (ipi) in recurrent glioblastoma (GBM): Updated results from checkmate-143. Am Soc Clin Oncol. (2016) 34:15. doi: 10.1200/JCO.2016.34.15_suppl.2014
44. Filley AC, Henriquez M, Dey M. Recurrent glioma clinical trial, CheckMate-143: the game is not over yet. Oncotarget. (2017) 8:91779–94. doi: 10.18632/oncotarget.21586
45. Liu F, Huang J, Liu X, Cheng Q, Luo C, Liu Z. CTLA-4 correlates with immune and clinical characteristics of glioma. Cancer Cell Int. (2020) 20:7. doi: 10.1186/s12935-019-1085-6
46. Chen D, Varanasi SK, Hara T, Traina K, Sun M, McDonald B, et al. CTLA-4 blockade induces a microglia-Th1 cell partnership that stimulates microglia phagocytosis and anti-tumor function in glioblastoma. Immunity. (2023) 56:2086–2104 e2088. doi: 10.1016/j.immuni.2023.07.015
47. Jiang M, Liu M, Liu G, Ma J, Zhang L, Wang S. Advances in the structural characterization of complexes of therapeutic antibodies with PD-1 or PD-L1. MAbs. (2023) 15:2236740. doi: 10.1080/19420862.2023.2236740
48. Wu Y, Chen W, Xu ZP, Gu W. PD-L1 distribution and perspective for cancer immunotherapy-blockade, knockdown, or inhibition. Front Immunol. (2019) 10:2022. doi: 10.3389/fimmu.2019.02022
49. Wei B, Wang L, Zhao X, Du C, Guo Y, Sun Z. The upregulation of programmed death 1 on peripheral blood T cells of glioma is correlated with disease progression. Tumour Biol. (2014) 35:2923–9. doi: 10.1007/s13277-013-1376-9
50. Dubinski D, Wolfer J, Hasselblatt M, Schneider-Hohendorf T, Bogdahn U, Stummer W, et al. CD4+ T effector memory cell dysfunction is associated with the accumulation of granulocytic myeloid-derived suppressor cells in glioblastoma patients. Neuro Oncol. (2016) 18:807–18. doi: 10.1093/neuonc/nov280
51. El Andaloussi A, Lesniak MS. An increase in CD4+CD25+FOXP3+ regulatory T cells in tumor-infiltrating lymphocytes of human glioblastoma multiforme. Neuro Oncol. (2006) 8:234–43. doi: 10.1215/15228517-2006-006
52. Gato-Canas M, Zuazo M, Arasanz H, Ibanez-Vea M, Lorenzo L, Fernandez-Hinojal G, et al. PDL1 signals through conserved sequence motifs to overcome interferon-mediated cytotoxicity. Cell Rep. (2017) 20:1818–29. doi: 10.1016/j.celrep.2017.07.075
53. Liu JN, Kong XS, Huang T, Wang R, Li W, Chen QF. Clinical implications of aberrant PD-1 and CTLA4 expression for cancer immunity and prognosis: A pan-cancer study. Front Immunol. (2020) 11:2048. doi: 10.3389/fimmu.2020.02048
54. Rao G, Latha K, Ott M, Sabbagh A, Marisetty A, Ling X, et al. Anti-PD-1 induces M1 polarization in the glioma microenvironment and exerts therapeutic efficacy in the absence of CD8 cytotoxic T cells. Clin Cancer Res. (2020) 26:4699–712. doi: 10.1158/1078-0432.CCR-19-4110
55. Cloughesy TF, Mochizuki AY, Orpilla JR, Hugo W, Lee AH, Davidson TB, et al. Neoadjuvant anti-PD-1 immunotherapy promotes a survival benefit with intratumoral and systemic immune responses in recurrent glioblastoma. Nat Med. (2019) 25:477–86. doi: 10.1038/s41591-018-0337-7
56. Zhao J, Chen AX, Gartrell RD, Silverman AM, Aparicio L, Chu T, et al. Immune and genomic correlates of response to anti-PD-1 immunotherapy in glioblastoma. Nat Med. (2019) 25:462–9. doi: 10.1038/s41591-019-0349-y
57. Lee AH, Sun L, Mochizuki AY, Reynoso JG, Orpilla J, Chow F, et al. Neoadjuvant PD-1 blockade induces T cell and cDC1 activation but fails to overcome the immunosuppressive tumor associated macrophages in recurrent glioblastoma. Nat Commun. (2021) 12:6938. doi: 10.1038/s41467-021-26940-2
58. Schalper KA, Rodriguez-Ruiz ME, Diez-Valle R, Lopez-Janeiro A, Porciuncula A, Idoate MA, et al. Neoadjuvant nivolumab modifies the tumor immune microenvironment in resectable glioblastoma. Nat Med. (2019) 25:470–6. doi: 10.1038/s41591-018-0339-5
59. Mittal D, Lepletier A, Madore J, Aguilera AR, Stannard K, Blake SJ, et al. CD96 is an immune checkpoint that regulates CD8(+) T-cell antitumor function. Cancer Immunol Res. (2019) 7:559–71. doi: 10.1158/2326-6066.CIR-18-0637
60. Liu F, Huang J, He F, Ma X, Fan F, Meng M, et al. CD96, a new immune checkpoint, correlates with immune profile and clinical outcome of glioma. Sci Rep. (2020) 10:10768. doi: 10.1038/s41598-020-66806-z
61. Hung AL, Maxwell R, Theodros D, Belcaid Z, Mathios D, Luksik AS, et al. TIGIT and PD-1 dual checkpoint blockade enhances antitumor immunity and survival in GBM. Oncoimmunology. (2018) 7:e1466769. doi: 10.1080/2162402X.2018.1466769
62. Li G, Wang Z, Zhang C, Liu X, Cai J, Wang Z, et al. Molecular and clinical characterization of TIM-3 in glioma through 1,024 samples. Oncoimmunology. (2017) 6:e1328339. doi: 10.1080/2162402X.2017.1328339
63. Morimoto T, Nakazawa T, Matsuda R, Nishimura F, Nakamura M, Yamada S, et al. CRISPR-cas9-mediated TIM3 knockout in human natural killer cells enhances growth inhibitory effects on human glioma cells. Int J Mol Sci. (2021) 22:3489. doi: 10.3390/ijms22073489
64. Zhang J, Zhu ZQ, Li YX, Zhuang QF, Lai Y, Li SF, et al. Therapeutics: Tim-3 expression in glioma cells is associated with drug resistance. J Cancer Res Ther. (2019) 15:882–8. doi: 10.4103/jcrt.JCRT_630_18
65. Liu J, Zhang S, Hu Y, Yang Z, Li J, Liu X, et al. Targeting PD-1 and Tim-3 pathways to reverse CD8 T-cell exhaustion and enhance ex vivo T-cell responses to autologous dendritic/tumor vaccines. J Immunother. (2016) 39:171–80. doi: 10.1097/CJI.0000000000000122
66. Maruhashi T, Okazaki I-m, Sugiura D, Takahashi S, TK M, Shimizu K, et al. LAG-3 inhibits the activation of CD4+ T cells that recognize stable pMHCII through its conformation-dependent recognition of pMHCII. Nat Immunol. (2018) 19:1415–26. doi: 10.1038/s41590-018-0217-9
67. Harris-Bookman S, Mathios D, Martin AM, Xia Y, Kim E, Xu H, et al. Expression of LAG-3 and efficacy of combination treatment with anti-LAG-3 and anti-PD-1 monoclonal antibodies in glioblastoma. Int J Cancer. (2018) 143:3201–8. doi: 10.1002/ijc.31661
68. Prendergast GC, Smith C, Thomas S, Mandik-Nayak L, Laury-Kleintop L, Metz R, et al. Indoleamine 2,3-dioxygenase pathways of pathogenic inflammation and immune escape in cancer. Cancer Immunol Immunother. (2014) 63:721–35. doi: 10.1007/s00262-014-1549-4
69. Wainwright DA, Balyasnikova IV, Chang AL, Ahmed AU, Moon KS, Auffinger B, et al. IDO expression in brain tumors increases the recruitment of regulatory T cells and negatively impacts survival. Clin Cancer Res. (2012) 18:6110–21. doi: 10.1158/1078-0432.CCR-12-2130
70. Mitsuka K, Kawataki T, Satoh E, Asahara T, Horikoshi T, Kinouchi H. Expression of indoleamine 2,3-dioxygenase and correlation with pathological Malignancy in gliomas. Neurosurgery. (2013) 72:1031–8. doi: 10.1227/NEU.0b013e31828cf945
71. Liu T, Zhu C, Chen X, Guan G, Zou C, Shen S, et al. Ferroptosis, as the most enriched programmed cell death process in glioma, induces immunosuppression and immunotherapy resistance. Neuro Oncol. (2022) 24:1113–25. doi: 10.1093/neuonc/noac033
72. Guo D, Tong Y, Jiang X, Meng Y, Jiang H, Du L, et al. Aerobic glycolysis promotes tumor immune evasion by hexokinase2-mediated phosphorylation of IkappaBalpha. Cell Metab. (2022) 34:1312–1324 e1316. doi: 10.1016/j.cmet.2022.08.002
73. Liu H, Zhao Q, Tan L, Wu X, Huang R, Zuo Y, et al. Neutralizing IL-8 potentiates immune checkpoint blockade efficacy for glioma. Cancer Cell. (2023) 41:693–710 e698. doi: 10.1016/j.ccell.2023.03.004
74. Wright K. FDA approves nivolumab plus ipilimumab for the treatment of advanced HCC. Oncol (Williston Park). (2020) 34:693606.
75. Reardon DA, Gokhale PC, Klein SR, Ligon KL, Rodig SJ, Ramkissoon SH, et al. Glioblastoma eradication following immune checkpoint blockade in an orthotopic, immunocompetent model. Cancer Immunol Res. (2016) 4:124–35. doi: 10.1158/2326-6066.CIR-15-0151
76. Vom Berg J, Vrohlings M, Haller S, Haimovici A, Kulig P, Sledzinska A, et al. Intratumoral IL-12 combined with CTLA-4 blockade elicits T cell-mediated glioma rejection. J Exp Med. (2013) 210:2803–11. doi: 10.1084/jem.20130678
77. Omuro A, Vlahovic G, Lim M, Sahebjam S, Baehring J, Cloughesy T, et al. Nivolumab with or without ipilimumab in patients with recurrent glioblastoma: results from exploratory phase I cohorts of CheckMate 143. Neuro Oncol. (2018) 20:674–86. doi: 10.1093/neuonc/nox208
78. Castro MG, Baker GJ, Lowenstein PR. Blocking immunosuppressive checkpoints for glioma therapy: the more the Merrier! Clin Cancer Res. (2014) 20:5147–9. doi: 10.1158/1078-0432.CCR-14-0820
79. Sakuishi K, Apetoh L, Sullivan JM, Blazar BR, Kuchroo VK, Anderson AC. Targeting Tim-3 and PD-1 pathways to reverse T cell exhaustion and restore anti-tumor immunity. J Exp Med. (2010) 207:2187–94. doi: 10.1084/jem.20100643
80. Woo SR, Turnis ME, Goldberg MV, Bankoti J, Selby M, Nirschl CJ, et al. Immune inhibitory molecules LAG-3 and PD-1 synergistically regulate T-cell function to promote tumoral immune escape. Cancer Res. (2012) 72:917–27. doi: 10.1158/0008-5472.CAN-11-1620
81. Fourcade J, Sun Z, Benallaoua M, Guillaume P, Luescher IF, Sander C, et al. Upregulation of Tim-3 and PD-1 expression is associated with tumor antigen-specific CD8+ T cell dysfunction in melanoma patients. J Exp Med. (2010) 207:2175–86. doi: 10.1084/jem.20100637
82. Francisco LM, Salinas VH, Brown KE, Vanguri VK, Freeman GJ, Kuchroo VK, et al. PD-L1 regulates the development, maintenance, and function of induced regulatory T cells. J Exp Med. (2009) 206:3015–29. doi: 10.1084/jem.20090847
83. Mbongue JC, Nicholas DA, Torrez TW, Kim NS, Firek AF, Langridge WH. The role of indoleamine 2, 3-dioxygenase in immune suppression and autoimmunity. Vaccines (Basel). (2015) 3:703–29. doi: 10.3390/vaccines3030703
84. Munn DH, Sharma MD, Mellor AL. Ligation of B7-1/B7-2 by human CD4+ T cells triggers indoleamine 2,3-dioxygenase activity in dendritic cells. J Immunol. (2004) 172:4100–10. doi: 10.4049/jimmunol.172.7.4100
85. Kline C, Liu SJ, Duriseti S, Banerjee A, Nicolaides T, Raber S, et al. Reirradiation and PD-1 inhibition with nivolumab for the treatment of recurrent diffuse intrinsic pontine glioma: a single-institution experience. J Neurooncol. (2018) 140:629–38. doi: 10.1007/s11060-018-2991-5
86. Sharabi AB, Lim M, DeWeese TL, Drake CG. Radiation and checkpoint blockade immunotherapy: radiosensitisation and potential mechanisms of synergy. Lancet Oncol. (2015) 16:e498–509. doi: 10.1016/S1470-2045(15)00007-8
87. Dai B, Qi N, Li J, Zhang G. Temozolomide combined with PD-1 Antibody therapy for mouse orthotopic glioma model. Biochem Biophys Res Commun. (2018) 501:871–6. doi: 10.1016/j.bbrc.2018.05.064
88. Hanihara M, Kawataki T, Oh-Oka K, Mitsuka K, Nakao A, Kinouchi H. Synergistic antitumor effect with indoleamine 2,3-dioxygenase inhibition and temozolomide in a murine glioma model. J Neurosurg. (2016) 124:1594–601. doi: 10.3171/2015.5.JNS141901
89. Yi M, Jiao D, Qin S, Chu Q, Wu K, Li A. Synergistic effect of immune checkpoint blockade and anti-angiogenesis in cancer treatment. Mol Cancer. (2019) 18:60. doi: 10.1186/s12943-019-0974-6
90. Voron T, Colussi O, Marcheteau E, Pernot S, Nizard M, Pointet AL, et al. VEGF-A modulates expression of inhibitory checkpoints on CD8+ T cells in tumors. J Exp Med. (2015) 212:139–48. doi: 10.1084/jem.20140559
91. Tamura R, Tanaka T, Ohara K, Miyake K, Morimoto Y, Yamamoto Y, et al. Persistent restoration to the immunosupportive tumor microenvironment in glioblastoma by bevacizumab. Cancer Sci. (2019) 110:499–508. doi: 10.1111/cas.2019.110.issue-2
92. Zhao Y, Chen G, Chen J, Zhuang L, Du Y, Yu Q, et al. AK112, a novel PD-1/VEGF bispecific antibody, in combination with chemotherapy in patients with advanced non-small cell lung cancer (NSCLC): an open-label, multicenter, phase II trial. EClinicalMedicine. (2023) 62:102106. doi: 10.1016/j.eclinm.2023.102106
93. Zhang X, Zhang P, Cong A, Feng Y, Chi H, Xia Z, et al. Unraveling molecular networks in thymic epithelial tumors: deciphering the unique signatures. Front Immunol. (2023) 14:1264325. doi: 10.3389/fimmu.2023.1264325
94. Gong J, Tang Z, Yu Z, Deng Z, Liu Y, Ren N, et al. miR-138-5p inhibits the growth and invasion of glioma cells by regulating WEE1. Anal Cell Pathol (Amst). (2022) 2022:7809882. doi: 10.1155/2022/7809882
95. Mustafov D, Siddiqui SS, Klena L, Karteris E, Braoudaki M. SV2B/miR-34a/miR-128 axis as prognostic biomarker in glioblastoma multiforme. Sci Rep. (2024) 14:6647. doi: 10.1038/s41598-024-55917-6
96. Wei J, Nduom EK, Kong LY, Hashimoto Y, Xu S, Gabrusiewicz K, et al. MiR-138 exerts anti-glioma efficacy by targeting immune checkpoints. Neuro Oncol. (2016) 18:639–48. doi: 10.1093/neuonc/nov292
97. Wang Y, Wang L. miR-34a attenuates glioma cells progression and chemoresistance via targeting PD-L1. Biotechnol Lett. (2017) 39:1485–92. doi: 10.1007/s10529-017-2397-z
98. Parsa AT, Waldron JS, Panner A, Crane CA, Parney IF, Barry JJ, et al. Loss of tumor suppressor PTEN function increases B7-H1 expression and immunoresistance in glioma. Nat Med. (2007) 13:84–8. doi: 10.1038/nm1517
99. Dorand RD, Nthale J, Myers JT, Barkauskas DS, Avril S, Chirieleison SM, et al. Cdk5 disruption attenuates tumor PD-L1 expression and promotes antitumor immunity. Science. (2016) 353:399–403. doi: 10.1126/science.aae0477
Keywords: glioblastoma, immune evasion, immunotherapy, PD-1, immune checkpoint
Citation: Su H, Peng Y, Wu Y and Zeng X (2025) Overcoming immune evasion with innovative multi-target approaches for glioblastoma. Front. Immunol. 16:1541467. doi: 10.3389/fimmu.2025.1541467
Received: 07 December 2024; Accepted: 02 January 2025;
Published: 22 January 2025.
Edited by:
Dan Liu, Wuhan University, ChinaReviewed by:
Min Miao, Ningbo University, ChinaCopyright © 2025 Su, Peng, Wu and Zeng. This is an open-access article distributed under the terms of the Creative Commons Attribution License (CC BY). The use, distribution or reproduction in other forums is permitted, provided the original author(s) and the copyright owner(s) are credited and that the original publication in this journal is cited, in accordance with accepted academic practice. No use, distribution or reproduction is permitted which does not comply with these terms.
*Correspondence: Xiaoli Zeng, eGx6ZW5nOTE1QGdtdS5lZHUuY24=
Disclaimer: All claims expressed in this article are solely those of the authors and do not necessarily represent those of their affiliated organizations, or those of the publisher, the editors and the reviewers. Any product that may be evaluated in this article or claim that may be made by its manufacturer is not guaranteed or endorsed by the publisher.
Research integrity at Frontiers
Learn more about the work of our research integrity team to safeguard the quality of each article we publish.