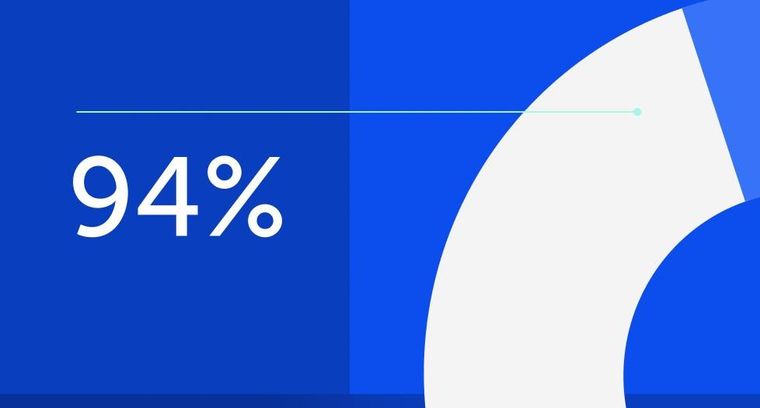
94% of researchers rate our articles as excellent or good
Learn more about the work of our research integrity team to safeguard the quality of each article we publish.
Find out more
REVIEW article
Front. Immunol., 29 January 2025
Sec. Cancer Immunity and Immunotherapy
Volume 16 - 2025 | https://doi.org/10.3389/fimmu.2025.1531246
This article is part of the Research TopicPost-Translational Modifications (PTMs) in Human Cancer: Pharmacological Insights and Therapeutic OpportunitiesView all 11 articles
Succinylation represents an emerging class of post-translational modifications (PTMs), characterized by the enzymatic or non-enzymatic transfer of a negatively charged four-carbon succinyl group to the ϵ-amino group of lysine residues, mediated by succinyl-coenzyme A. Recent studies have highlighted the involvement of succinylation in various diseases, particularly cancer progression. Sirtuin 5 (SIRT5), a member of the sirtuin family, has been extensively studied for its robust desuccinylase activity, alongside its deacetylase function. To date, only a limited number of SIRT5 substrates have been identified. These substrates mediate diverse physiological processes such as glucose oxidation, fatty acid oxidation, ammonia detoxification, reactive oxygen species scavenging, anti-apoptosis, and inflammatory responses. The regulation of these activities can occur through either the same enzymatic activity acting on different substrates or distinct enzymatic activities targeting the same substrate. Aberrant expression of SIRT5 has been closely linked to tumorigenesis and disease progression; however, its role remains controversial. SIRT5 exhibits dual functionalities: it can promote tumor proliferation, metastasis, drug resistance, and metabolic reprogramming, thereby acting as an oncogene; conversely, it can also inhibit tumor cell growth and induce apoptosis, functioning as a tumor suppressor gene. This review aims to provide a comprehensive overview of the current research status of SIRT5. We discuss its structural characteristics and regulatory mechanisms, compare its functions with other sirtuin family members, and elucidate the mechanisms regulating SIRT5 activity. Specifically, we focus on the role of succinylation modification mediated by SIRT5 in tumor progression, highlighting how desuccinylation by SIRT5 modulates tumor development and delineating the underlying mechanisms involved.
Cancer continues to pose a significant global health challenge. According to recent data, the countries with the highest incidence of new cancer cases include China, the United States, India, Japan, Germany, Brazil, Russia, France, the United Kingdom, and Italy (1–3). Emerging research underscores the critical role of PTMs in tumor progression (4, 5). a member of the sirtuin family, was initially characterized as a mitochondrial deacetylase but has since been recognized for its diverse roles in PTMs, particularly desuccinylation (6). Located on chromosome locus 6p23, SIRT5 is an NAD-dependent deacetylase that contains a Zn²+ binding domain and a Rossmann fold domain, which together form the substrate binding site and the NAD+ binding site (6). Compared to other sirtuins, SIRT5 features a larger lysine acyl-binding pocket, resulting in relatively weaker deacetylase activity but robust desuccinylase activity (7). SIRT5 stands out among sirtuins due to its unique ability to remove succinyl groups from lysine residues, significantly influencing protein function and cellular metabolism (8). The biological significance of desuccinylation has garnered increasing attention, especially in the context of cancer. By modulating the succinylation status of key metabolic enzymes, SIRT5 affects various cellular processes, including glycolysis, mitochondrial function, and cell proliferation (9–11). This modulation is crucial because succinylation can either activate or inhibit enzymes involved in these pathways, thereby impacting tumorigenesis and cancer progression.
PTMs encompass a diverse array of chemical alterations where modifying groups covalently bind to substrate proteins, thereby altering their physiological properties such as activity, cellular localization, stability, and interactions with other proteins, ultimately influencing their function (12). Advances in proteomics have led to the identification of various PTM types, including acetylation, propionylation, methylation, butyrylation, succinylation, crotonylation, malonylation, ubiquitination, and 2-hydroxyisobutyrylation. As proteomics continues to advance, an increasing number of studies are reporting the involvement of PTMs in cancer development and progression (13, 14). Among these PTMs, succinylation has garnered significant attention in recent years for its role in tumorigenesis. Succinylation involves the reversible and dynamic covalent attachment of a succinyl group (-COCH2-CH2-COOH), donated by succinyl-CoA, to amino acid residues, predominantly lysine, within substrate proteins (15). This process is evolutionarily conserved and plays a critical role in numerous biological processes. Aberrant lysine succinylation has been shown to significantly impact metabolic pathways, gene transcription, DNA damage responses, and protein folding, stability, and functionality (16). Although several studies have documented the involvement of succinylation in various physiological and pathological processes (17, 18), including tumor biology (19), elucidating the regulatory mechanisms underlying succinylation in cancer can provide novel insights for prevention and therapeutic strategies.
In summary, the research surrounding SIRT5 and its desuccinylation activity underscores its pivotal role in cancer biology. By modulating the succinylation status of key metabolic enzymes and interacting with tumor suppressor pathways, SIRT5 exerts multifaceted influences on tumorigenesis. The ongoing elucidation of SIRT5’s functions and mechanisms may pave the way for novel therapeutic approaches that target metabolic pathways in cancer treatment, potentially improving outcomes for patients with malignancies characterized by dysregulated metabolic processes (20, 21). Therefore, this review aims to provide a comprehensive overview of the current research status of SIRT5. Specifically, it seeks to elucidate the role of SIRT5-mediated succinylation modification in tumors, thereby establishing a theoretical foundation for understanding the mechanisms of tumor development. Additionally, this review offers new perspectives for tumor therapy and drug development, highlighting the potential of targeting SIRT5 and succinylation as innovative strategies for combating cancer.
The human SIRT5 gene, located at chromosome locus 6p23, encodes two protein isoforms comprising 310 and 299 amino acids, respectively. Predominantly localized within the mitochondria, with minor presence in the cytoplasm, SIRT5 exhibits a complex structural architecture. The protein consists of 14 β-strands and 9 α-helices that form both the zinc-binding domain and the Rossmann fold domain, thereby creating the substrate and NAD+ binding sites (6). Within the substrate binding site, three hydrophobic residues—phenylalanine-223 (Phe223), leucine-227 (Leu227), and valine-254 (Val254)—form the entrance for acyl-lysine groups. Two non-hydrophobic residues, tyrosine-102 (Tyr102) and arginine-105 (Arg105), specifically recognize the negatively charged acyl-lysine structure. Additionally, alanine-86 (Ala86) contributes to the formation of a larger lysine acyl-binding pocket in SIRT5 (22). These structural features confer SIRT5’s preference for short-chain carboxylates, such as malonyl, succinyl, and glutaryl groups, over acetyl groups (23). Consequently, the catalytic efficiency of SIRT5 for desuccinylation, demalonylation, and deglutarylation activities is approximately 1000-fold higher than its deacetylase activity (24).
SIRT5 exhibits widespread expression across various organs, including the brain, heart, liver, kidneys, muscles, and testes, with predominant localization within mitochondria; however, it is also detectable in the cytoplasm and nucleus (25). In mammals, SIRT5 functions as a primary regulator of lysine desuccinylation. In mouse liver and embryonic fibroblasts, a comprehensive proteomic analysis identified 2,565 succinylation sites across 779 proteins. Upon SIRT5 gene knockout, over 90% of these sites demonstrated increased succinylation levels, primarily affecting proteins involved in the tricarboxylic acid (TCA) cycle and fatty acid metabolism (26). Consistent with these findings, Rardin et al. (27) reported that in the absence of SIRT5, 386 succinylation sites across 140 proteins in mouse liver mitochondria exhibited enhanced succinylation. These succinylated proteins predominantly participate in energy metabolism, β-oxidation, and ketone body production. Recent studies have further elucidated the role of SIRT5 in cardiac tissues, identifying key targets that suggest SIRT5-mediated deglutarylation may play a crucial role in maintaining cardiac energy metabolism (28, 29). This evidence underscores the importance of SIRT5 in regulating metabolic pathways critical for cellular function and homeostasis.
Despite the identification of numerous SIRT5 substrates, including a variety of metabolic enzymes, research into its desuccinylase, demalonylase, and deglutarylase activities is still in its early stages. This relative paucity of research can be attributed to several factors. Firstly, the discovery of SIRT5’s non-acetylation-related enzymatic activities is relatively recent compared to its deacetylase function, which has been extensively studied over the years. The novel nature of these modifications means that specific tools and methodologies for their detection and study are still being developed, refined, and disseminated within the scientific community. Secondly, the complexity of succinylation, malonylation, and glutarylation as PTMs poses additional challenges. These PTMs occur at lower abundances than acetylation and require highly sensitive and specific analytical techniques, such as mass spectrometry coupled with enrichment strategies, for reliable detection and quantification. The technical hurdles associated with studying these modifications have likely slowed progress in this area. Furthermore, the functional significance of these PTMs is not yet fully understood, which may lead to a lack of targeted research efforts. While it is clear that they play critical roles in cellular metabolism and other biological processes, the exact mechanisms by which they influence protein function and cellular physiology remain to be elucidated. As the importance of these modifications becomes more apparent, interest and investment in this field are expected to increase, driving further discoveries. Continued advancements in technology, along with growing awareness of the importance of these PTMs, are likely to facilitate deeper exploration and understanding of SIRT5’s role in regulating these modifications and their implications for health and disease. In summary, while significant strides have been made in identifying SIRT5 substrates, the full spectrum of its enzymatic activities, particularly those related to desuccinylation, demalonylation, and deglutarylation, remains to be thoroughly investigated. Addressing these knowledge gaps will be crucial for advancing our understanding of SIRT5’s functions and developing potential therapeutic targets for diseases characterized by dysregulated metabolic processes.
In addition to SIRT5, the sirtuin family encompasses proteins with distinct characteristics and structures. The sirtuins constitute a highly conserved family of proteins, comprising seven members in mammals (SIRT1-7), which regulate various metabolic and stress response pathways (30). Specifically, SIRT1 and SIRT2 are predominantly localized in the cytoplasm, while SIRT3, SIRT4, and SIRT5 reside within mitochondria, and SIRT6 and SIRT7 are found in the nucleus (31). These proteins play pivotal roles in genomic stability, cell cycle regulation, metabolism, aging, and disease development (32). Sirtuins possess NAD+-dependent deacetylase activity (SIRT1, SIRT2, SIRT3, SIRT5, SIRT6, and SIRT7) or mono-ADP-ribosyltransferase activity (SIRT4 and SIRT6). Each sirtuin exhibits distinct enzymatic activities, biological functions, and subcellular localizations, which contribute to their diverse roles in cancer biology (Table 1).
For instance, SIRT1, an NAD+-dependent histone deacetylase belonging to the sirtuin family, has been closely associated with tumor development (33). It influences a wide array of processes, including cellular senescence (34), DNA replication (35), cell growth, metabolism (36), and tumor progression (37). Studies indicate that SIRT1 impacts the onset and progression of various cancers, such as colorectal, prostate, breast, and murine lung cancers, sarcomas, and lymphomas (38–40). Given its high expression in certain tumor tissues and its inhibition of several tumor suppressor genes like FOXO1, p73, and WRN (41–43), SIRT1 is believed to promote tumorigenesis. SIRT1 primarily modulates transcription factors, histones, and other non-histone substrates through deacetylation, thereby affecting gene expression patterns (35, 37). In contrast, SIRT5 exhibits a broader range of actions, encompassing not only deacetylation but also desuccinylation and demalonylation activities (27). While both SIRT1 and SIRT5 are involved in metabolic regulation, SIRT1 is more closely associated with nutrient-sensing signaling pathways, whereas SIRT5 focuses on the direct regulation of metabolic enzymes. This distinction highlights the specialized roles of each sirtuin in cellular metabolism and underscores the importance of understanding their individual contributions to cancer biology.
SIRT2, predominantly localized in the cytoplasm but also present in mitochondria and nuclei, deacetylates a variety of endogenous substrates, playing a significant role in multiple physiological and pathological processes. These include cancer cell proliferation, cell cycle regulation, apoptosis, genomic integrity, cellular metabolism, infection, and inflammation (44–46). Notably, SIRT2 exhibits both oncogenic and tumor-suppressive functions across different cancer types, indicating context-specific roles in cancer progression (47). While both SIRT5 and SIRT2 possess deacetylase activity, their distinct subcellular distributions determine their primary functions. The mitochondrial localization of SIRT5 positions it as a key regulator of metabolic processes, particularly in energy production and metabolite conversion (48). In contrast, SIRT2’s presence in the cytoplasm involves it more prominently in processes such as cytoskeletal dynamics, cell division, and signaling pathways (49).
SIRT3 is a critical mitochondrial deacetylase that plays an essential role in regulating protein acetylation levels, maintaining mitochondrial integrity, and modulating energy metabolism (50, 51). Hyper-acetylation, frequently observed in tumors, contributes to cancer survival by altering protein function. SIRT3 counteracts this hyper-modification, thereby modulating tumor progression (52). Moreover, SIRT3 can reprogram metabolism, significantly impacting tumor initiation and progression (53). However, its dual nature, exhibiting both pro- and anti-tumorigenic effects, complicates its targeting for therapeutic purposes (54, 55). Both SIRT3 and SIRT5 are principal sirtuin members within the mitochondria, each contributing uniquely to mitochondrial function. SIRT3 is renowned for its antioxidant effects and metabolic control, enhancing mitochondrial efficiency and reducing oxidative stress (56). It acts primarily as a deacetylase, normalizing hyper-acetylation and supporting mitochondrial health. Conversely, SIRT5 exhibits a broader range of demodification activities within the mitochondria. It plays a crucial role in fatty acid oxidation (28) and amino acid metabolism (57), including the regulation of arginase II activity via desuccinylation, which influences the urea cycle (58).
SIRT4 significantly inhibits glutamine metabolism by ADP-ribosylating glutamate dehydrogenase, thereby limiting the supply of energy and materials required for rapid proliferation in tumor cells (59, 60). This effect has been confirmed across various cancer types, including breast cancer (61), colorectal cancer (62), esophageal squamous cell carcinoma (63), and thyroid cancer (64). The consensus that SIRT4 suppresses tumor development through inhibition of glutamine metabolism suggests its potential as a novel biomarker and therapeutic target for malignancies. While both SIRT5 and SIRT4 act within mitochondria, they exhibit distinct functional orientations and mechanisms. SIRT5 primarily modulates metabolic enzyme activity through its unique demodification activities, whereas SIRT4 affects metabolic processes by regulating signaling pathways. Specifically, SIRT5’s enzymatic actions are more directly involved in the regulation of metabolic enzyme activity, while SIRT4 plays a more significant role in signal transduction and metabolic network regulation.
SIRT6 is predominantly localized in the nucleus and possesses two key enzymatic activities: NAD+-dependent deacetylase and mono-ADP-ribosyltransferase. These activities are integral to SIRT6’s functions (65). Studies have shown that, acting as a deacetylase for histone H3 lysine 9 (H3K9), SIRT6 controls the expression of various glycolytic genes, particularly by co-repressing the transcription factor hypoxia-inducible factor 1α (HIF-1α), thus inhibiting tumor progression (66). Moreover, overexpression of SIRT6 can induce apoptosis in fibrosarcoma and human cervical cancer cell lines via its mono-ADP-ribosyltransferase activity without affecting normal cells (67). Conversely, SIRT6 has also been shown to enhance cytokine secretion and cell motility, and increase drug resistance by hyperactivating calcium channels, playing a pro-oncogenic role (68). This dual action of SIRT6 appears to depend on tissue context, spatiotemporal distribution of various factors, and different stages of tumorigenesis. Despite sharing deacetylase activity, SIRT5 and SIRT6 differ significantly in their biological functions due to their distinct subcellular localizations. SIRT5 operates mainly within the mitochondria, influencing metabolic pathways and energy conversion, whereas SIRT6 is active in the nucleus, participating in DNA repair and gene expression regulation. This disparity underscores the Sirtuin family’s capability to perform diverse functions within the cell, contributing to cellular health and adaptation to environmental changes.
SIRT7, primarily localized in the nucleolus, has recently been identified as possessing deacetylase activity towards specific substrates, thereby influencing cellular life activities through various pathways (69). Overexpression of SIRT7 has been observed in several human malignancies, including hepatocellular carcinoma (70), breast cancer (71), thyroid cancer (72), gastric cancer (73), and others. Its expression levels correlate with clinical-pathological features and patient prognosis, underscoring its potential role in tumor biology. The oncogenic effects of SIRT7 are closely linked to its deacetylation activity, which primarily influences gene expression regulation. Unlike SIRT7, which predominantly affects transcriptional regulation, SIRT5 plays a crucial role in cellular energy metabolism. The mitochondrial localization of SIRT5 establishes it as a key regulator of metabolic enzyme activity, whereas the nuclear role of SIRT7 positions it as an important participant in gene expression modulation. These two sirtuin members influence cellular health through distinct mechanisms: SIRT5 by modulating metabolic pathways and SIRT7 by regulating gene expression.
In summary, while all members of the sirtuin family depend on NAD+ and can influence metabolic processes, each member exhibits specific cellular localization and functional characteristics. SIRT5 stands out for its importance in non-conventional lysine modifications, such as desuccinylation, which are less common among other sirtuin members. Additionally, the unique role of SIRT5 in mitochondrial metabolism, particularly in fatty acid oxidation, highlights its significance in cellular energy production and tumor progression.
Protein PTMs represent a vast array of biochemical alterations that modulate protein function. To date, over 600 types of PTMs have been identified, including methylation, phosphorylation, ubiquitination, acetylation, succinylation, and lactylation (113). Among these, succinylation involves the enzymatic or non-enzymatic addition of a succinyl group to the ϵ-amino group of lysine residues within proteins, representing a reversible modification that can significantly influence protein spatial conformation, activity, stability, and intracellular localization (114). This acylation process can occur either non-enzymatically or enzymatically (16). Enzymatic succinylation primarily relies on succinyltransferases, which are analogous to histone acetyltransferases (HATs), facilitating the transfer of a succinyl group from succinyl-CoA to target protein lysine residues. This process is highly specific, allowing for precise regulation of protein function (115, 116) (Figure 1). In contrast, non-enzymatic succinylation occurs dynamically and widely in response to changes in the cellular metabolic environment, such as during metabolic or oxidative stress conditions. Under these circumstances, succinyl groups can directly bind to lysine residues through spontaneous chemical reactions. The dynamic equilibrium of this non-enzymatic modification is influenced by various factors, including the concentration of succinyl donors, intracellular pH levels, and redox status (116, 117).
Understanding the dynamic equilibrium of succinylation is critical for elucidating its role under both physiological and pathological conditions. Under normal physiological conditions, appropriate regulation of succinylation levels helps maintain cellular metabolic homeostasis and function. Conversely, in pathological states, aberrant increases or decreases in succinylation can lead to cellular dysfunction, thereby promoting disease progression. For instance, in cancer cells, succinylation influences cell proliferation and apoptosis by modulating the activity of key metabolic enzymes (116, 118). Moreover, succinylation is closely associated with the development and progression of various metabolic diseases, including liver metabolism disorders and diabetes. The main factors affecting the dynamic balance of succinylation include cellular metabolic status, environmental conditions (such as temperature and pH), and cellular signaling pathways (119, 120). Therefore, delving deeper into the regulatory mechanisms governing succinylation’s dynamic balance could provide new insights and potential therapeutic targets for preventing and treating related diseases.
Lysine succinylation is a prevalent PTM observed in both eukaryotic and prokaryotic cells (121), with its role in eukaryotic cells being closely associated with cancer progression. Within the nucleus of eukaryotic cells, lysine succinylation has been identified at specific histone sites, including H1.3K65, H1.3K86, H2BK109, H2BK117, H3K79, H3K120, and H3K122 (122). Additionally, non-histone proteins such as HMGB2K114, HMGB1K127, HMGB1K114, and HMGB1K43 also undergo succinylation (123). In the cytoplasm, lysine succinylation affects a wide array of metabolic enzymes and regulatory proteins. These include glycolytic enzymes and those involved in the TCA cycle, such as PDK3, IDH2, ACO2, DLAT, PDHA1, PITRM1, GOT2, MDH2, IDH3B, and SDHA. Enzymes involved in fatty acid metabolism, like ACAA2, HSD17B10, ETFa, HADHB, and HADHA, are also subject to this PTM. Proteins participating in ketone body metabolism, such as OXCT1 and ACAT1, and those related to reactive oxygen species (ROS) scavenging, including SOD, PRX, and GPX, exhibit succinylation as well (124). Thus, in eukaryotes, the dynamic regulation of protein succinylation and desuccinylation modulates various cellular processes, including metabolism, transcriptional regulation, and DNA damage repair. These processes are essential for maintaining normal cellular functions and are intimately linked with the occurrence and development of tumors.
Succinyl-coenzyme A (succinyl-CoA) serves as the primary donor of succinyl groups, primarily derived from the mitochondrial TCA cycle through the oxidative decarboxylation of α-ketoglutarate. Beyond glucose metabolism, the catabolism of amino acids such as methionine, threonine, valine, and isoleucine can also generate succinyl-CoA. Additionally, short-chain fatty acid ω-oxidation products, including hydroxy fatty acids, can be converted into succinyl-CoA. For instance, fibroblasts have been shown to utilize fatty acids as an effective source of succinyl-CoA under conditions where glucose and pyruvate are absent from the culture medium (125). The intracellular concentration of succinyl-CoA directly influences the level of succinylation modification (126), indicating that alterations in metabolic pathways can lead to corresponding changes in protein succinylation.
In the realm of energy metabolism, succinylation plays a pivotal role in modulating the activity of key metabolic enzymes. Studies have demonstrated that succinylation can influence the function of TCA cycle enzymes, thereby affecting overall mitochondrial energy production. This modification is particularly relevant in conditions characterized by mitochondrial dysfunction, such as heart failure and ischemic stroke. In models of heart failure, altered succinylation patterns have been linked to impaired oxidative phosphorylation capacity and dysregulated energy metabolism, highlighting the potential of targeting succinylation pathways for therapeutic interventions (127). The dynamic regulation of succinylation, mediated by enzymes such as SIRT5, which desuccinylates metabolic enzymes, underscores the complexity of metabolic regulation and its impact on cellular energy homeostasis (17). SIRT5’s role in controlling succinylation levels further emphasizes the intricate balance required for maintaining optimal metabolic function.
Oxidative stress represents another critical domain where succinylation exerts its regulatory influence. Accumulation of ROS can lead to oxidative damage, which is implicated in various diseases, including neurodegenerative disorders and cardiovascular diseases. Recent evidence suggests that succinylation can modulate ROS generation by influencing the stability and activity of antioxidant enzymes. For instance, succinylation of specific proteins involved in the antioxidant response can either enhance or inhibit their activity, thereby shaping the cellular response to oxidative stress (128). This regulatory mechanism is particularly significant in the context of aging and neuroinflammation, where increased succinylation levels have been associated with a senescence phenotype in microglia, suggesting a potential link between succinylation and age-related neurodegenerative processes (129).
Succinylation also plays a substantial role in regulating gene expression. This modification can alter chromatin structure and transcription factor activity, thereby influencing the transcription of genes involved in metabolism, stress response, and other critical cellular functions. Notably, succinylation of histones has been implicated in the regulation of gene expression patterns associated with tumorigenesis, indicating that this PTM could serve as a potential therapeutic target in cancer (130). Moreover, the interplay between succinylation and other PTMs, such as acetylation and phosphorylation, adds an additional layer of complexity to the regulatory networks governing gene expression and cellular responses to environmental cues (131).
In summary, succinylation emerges as a crucial post-translational modification that intricately links energy metabolism, oxidative stress response, and gene expression regulation. Ongoing research into the mechanisms and effects of succinylation continues to unveil its significance in maintaining cellular homeostasis and its potential implications in various diseases. As our understanding of succinylation deepens, it holds promise as a target for therapeutic interventions aimed at modulating metabolic disorders, oxidative stress-related conditions, and cancer (17).
Succinylation modification plays an essential role in various biological processes, and the regulatory mechanism of SIRT5 on succinylation has garnered increasing attention. Advances in the study of the deacylase SIRT5 have confirmed its dual capabilities: not only does it function as a deacetylase but it also exhibits potent desuccinylase activity (27). This versatility positions SIRT5 as a key regulator of cellular metabolism and other critical biological functions.
The enzymatic process by which SIRT5 catalyzes protein desuccinylation involves several critical steps that reflect the general mechanism of NAD+-dependent deacylation enzymes. Initially, SIRT5 must recognize and bind to the succinylated protein substrate. This binding typically occurs through the identification of specific sequence motifs or structural features on the substrate, particularly those harboring succinylated lysine residues. Upon binding, SIRT5 utilizes NAD+ as a covalent catalyst. In this context, NAD+ serves both as an electron donor and as a component that generates a covalent intermediate (ADP-ribose) during the reaction—a step essential for deacylation. The active site of SIRT5 contains a conserved cysteine residue that forms a covalent bond with the succinyl group of the succinyl-lysine residue. During this interaction, NAD+ is converted into nicotinamide and released from the complex. Subsequently, SIRT5 undergoes a series of chemical rearrangements to transfer the succinyl group from the substrate to the cysteine residue within the enzyme’s active site, forming a succinyl-enzyme intermediate. Finally, a water molecule attacks the succinyl group within the succinyl-enzyme intermediate, leading to the cleavage of the succinyl group. This results in the release of free succinate and the restoration of the unmodified state of the protein substrate. Once the reaction is complete, the desuccinylated protein is released from SIRT5, and the enzyme is reset to engage with another succinylated substrate, continuing the desuccinylation cycle (132). Through these catalytic steps, SIRT5 effectively removes succinyl groups from proteins, restoring the original state of lysine residues or altering their chemical environment. This action influences protein function, stability, or interactions with other molecules. Such deacylation is crucial for cellular metabolism, signaling pathways [such as NF-κB and IRF signaling (133), Notch and β-catenin signaling (134)], and adaptation to environmental changes.
The desuccinylation process mediated by SIRT5 is vital for regulating various cellular functions, including metabolism and stress responses. By modulating the succinylation state of proteins, SIRT5 can influence metabolic pathways, transcriptional activities, and other cellular processes, thereby playing a significant role in health and disease, including cancer development and progression.
In addition to the well-characterized SIRT5 and SIRT7, recent studies have identified new proteins that exhibit desuccinylase activity. Notably, Jialun Li et al. (135) reported that histone desuccinylation is predominantly catalyzed by class I histone deacetylases (HDAC1/2/3). Inhibition or depletion of HDAC1/2/3 resulted in a significant increase in global histone succinylation levels, while ectopic expression of these enzymes—but not their deacetylase-inactive mutants—reduced global histone succinylation. Furthermore, in vitro assays demonstrated robust histone desuccinylase activity for class I HDAC1/2/3 complexes. These findings establish that class I HDAC1/2/3, rather than SIRT family proteins, are the principal histone desuccinylases, particularly important for promoter histone desuccinylation. The understanding of desuccinylation mechanisms in microorganisms remains in its infancy due to the paucity of identified specific desuccinylases. CobB, a known Sir2-like bacterial lysine deacetylase, was recently identified as the first prokaryotic enzyme with desuccinylation activity (136). The characterization of CobB as a bifunctional enzyme capable of both lysine desuccinylation and deacetylation suggests that eukaryotic Kac-regulatory enzymes may possess enzymatic activities on various lysine acylations with distinct structures. Additionally, in the model soil bacterium Streptomyces coelicolor, a sirtuin-like protein named ScCobB2 was biochemically characterized as a divergent desuccinylase. Comparative LC-MS/MS analysis of the ΔScCobB2 mutant versus wild-type succinylome revealed a total of 673 unique succinylated sites, with 470 sites quantified across 317 proteins. Further quantitative analysis indicated that at least 114 proteins involved in two major pathways—protein biosynthesis and carbon metabolism—are markedly hypersuccinylated in ΔScCobB2 cells (137). We conducted an analysis of the protein domains of these desuccinylases. Figure 2 illustrates the specific sites regulated by SIRT5 and SIRT7 in the context of succinylation modification.
In summary, while SIRT5 is well-characterized as a desuccinylase with distinctive features, the existence and characteristics of other desuccinylases remain speculative without further research. SIRT5 stands out due to its defined role in mitochondrial function and its dependence on NAD+, distinguishing it from potential non-sirtuin desuccinylases that might operate through different mechanisms and within distinct cellular contexts. Future investigations into the diversity and specificity of desuccinylases will be crucial for elucidating their roles in cellular regulation and disease pathogenesis.
Given the unique properties and functions of SIRT5 discussed previously, this section delves into the primary factors that influence its activity. As an NAD+-dependent deacylase, the intracellular concentration of NAD+ is crucial for SIRT5’s functionality. NAD+ serves dual roles: it acts as a cofactor essential for SIRT5’s desuccinylation reaction and operates as a rate-limiting factor for enzyme activity. The catalytic mechanism of SIRT5 requires NAD+ as a cofactor. During catalysis, NAD+ is consumed, generating nicotinamide and an ADP-ribose moiety. This process is pivotal for forming a covalent intermediate necessary for the desuccinylation reaction. The active site of SIRT5 contains a conserved cysteine residue that forms a covalent bond with the succinyl group of succinyl-lysine. Simultaneously, the ADP-ribose portion of NAD+ transiently forms a covalent complex with the enzyme, facilitating subsequent hydrolysis steps (8). Variations in intracellular NAD+ levels directly impact SIRT5 activity. Higher NAD+ levels enhance SIRT5’s desuccinylase activity by providing sufficient cofactor support for the catalytic reaction, while decreased NAD+ levels reduce SIRT5 activity due to insufficient cofactor availability (138). The cellular metabolic state, particularly the energy status, significantly influences NAD+ levels (139). For instance, under conditions of fasting or caloric restriction, NAD+ levels increase, potentially enhancing SIRT5 activity (140). Conversely, in states characterized by high-fat diets or obesity, NAD+ levels may decrease, leading to reduced SIRT5 activity (141). The ratio of NAD+ to reduced nicotinamide adenine dinucleotide (NADH) also plays a critical role in determining SIRT5 activity. A higher NAD+/NADH ratio generally promotes SIRT5 activity, indicating greater availability of NAD+ as a cofactor, whereas a lower ratio can inhibit SIRT5 activity (121). During stress responses, such as oxidative stress or hypoxia, cells experience fluctuations in NAD+ levels, which directly affect SIRT5 activity and consequently influence the cellular response to these stress conditions (142, 143). In certain disease states, including diabetes and cardiovascular diseases, alterations in NAD+ levels can indirectly impact SIRT5 activity and its regulatory role in cellular metabolism and signaling (142, 144). Changes in cellular metabolic status directly influence the supply of NAD+, thereby affecting SIRT5 activity.
Understanding the impact of NAD+ levels on SIRT5 activity can provide insights into the enzyme’s role in cellular physiology and offer potential intervention strategies for related diseases, including cancer.
Succinylation modifications have been implicated in various malignancies, including lung cancer, melanoma, hepatocellular carcinoma, osteosarcoma, neurologic malignancies, renal cell carcinoma, thyroid cancer, and colorectal cancer (119, 145, 146). However, the role of succinylation in tumor progression is contingent upon the specific succinylation-modified genes, which can exert either tumor-suppressive or oncogenic effects. SIRT5, identified as the latest desuccinylation gene, acts as a double-edged sword in tumorigenesis. By modulating the expression of different target genes, SIRT5 can either inhibit or promote tumor development. The specific regulatory effects of SIRT5 on different cancer cells behaviors were shown in Table 2, Figure 3.
Studies have demonstrated that the succinylation of superoxide dismutase 1 (SOD1) promotes cancer cell proliferation; however, SIRT5 can reverse this effect by mediating desuccinylation and thereby restoring SOD1 enzyme activity. Lung cancer cells with mutations at the succinylation sites of SOD1 exhibit significantly reduced proliferation rates, indicating the tumor-suppressive role of SIRT5 (154). Clark et al. (155) found that mutant isocitrate dehydrogenase 1 (IDH1) converts α-ketoglutarate (α-KG) into R-2-hydroxyglutarate (R-2-HG), an α-KG analog that elevates succinyl-CoA levels, leading to abnormal succinylation of mitochondrial proteins and promoting cancer cell growth while impairing apoptosis. However, ectopic expression of SIRT5 reverses the metabolic defects and apoptotic resistance in IDH1-mutated glioma cells, impairing their growth both in vitro and in vivo (156). In hepatocellular carcinoma (HCC), SIRT5 also exerts a tumor-suppressive function. Expression of SIRT5 is lower in primary liver cancer tissues compared to normal tissues. Aberrant activation of acyl-CoA oxidase 1 (ACOX1), which is involved in H2O2 generation, leads to DNA oxidative damage and impaired liver function, contributing to HCC onset. SIRT5 can inhibit ACOX1 through desuccinylation, thereby reducing H2O2 levels and mitigating oxidative stress (97). In gastric cancer, Lu et al. (151) discovered that SIRT5 expression is significantly decreased in human gastric cancer tissues. Functional analyses indicated that overexpression of SIRT5 can inhibit gastric cancer cell growth both in vitro and in vivo by arresting the cell cycle at the G1/S phase and suppressing migration and invasion via modulation of epithelial-mesenchymal transition (EMT). Further analysis revealed that the tumor-suppressive effect of SIRT5 in gastric cancer is associated with the regulation of 2-oxoglutarate dehydrogenase (OGDH) expression. SIRT5-mediated desuccinylation of OGDH inhibits the OGDH complex’s activity, leading to reduced mitochondrial membrane potential, decreased ATP production, increased ROS levels, and altered NADP/NADPH ratios, ultimately suppressing gastric cancer progression. In prostate cancer, SIRT5 expression is significantly reduced, and a correlation between decreased SIRT5 levels and reduced patient survival has been established. Quantitative global succinylation profiling in prostate cancer revealed a significant increase in the succinylation of lysine 118 (K118su) of lactate dehydrogenase A (LDHA), enhancing LDH activity and exacerbating tumor progression. Overexpression of SIRT5 reduces LDHA-K118 succinylation, inhibiting the migration and invasion of prostate cancer cells and alleviating disease progression (157). Beyond these examples, SIRT5 has also been found to inhibit gastric cancer invasion by catalyzing the desuccinylation of S100A10 protein (158), and it can desuccinylate the K280 site of serine hydroxymethyltransferase 2 (SHMT2) protein, thereby inhibiting osteosarcoma development (159). Therefore, SIRT5 can inhibit tumor cell growth through interfering with multiple pathways.
Recent studies have also identified SIRT5 as an oncogenic promoter through its involvement in various pathways across different malignancies. Teng et al. (147) discovered that mitochondrial malic enzyme 2 (ME2) is highly expressed in colorectal cancer (CRC) tissues, and knockdown of ME2 inhibits CRC cell proliferation. Further analysis revealed that overexpressed ME2 undergoes SIRT5-mediated desuccinylation. Deprivation of glutamine directly enhances the interaction between SIRT5 and ME2, promoting desuccinylation of ME2 at lysine 346 and thereby activating ME2 enzyme activity. This activation leads to increased cellular proliferation and tumor growth. In breast cancer, SIRT5 expression is significantly elevated, and knockout of SIRT5 can induce oxidative stress by increasing the succinylation of IDH2, leading to apoptosis in tumor tissues and inhibiting tumor growth (160). In RCC, SIRT5 interacts with subunit A of the succinate dehydrogenase complex (SDHA). Knockout of SIRT5 results in increased succinylation and expression levels of SDHA. Elevated SIRT5 expression has been observed in RCC cells and tissues, and SIRT5 knockout inhibits cancer cell proliferation. These findings suggest that SIRT5 promotes the occurrence and development of RCC by inhibiting SDHA succinylation (161). Thus, elevated SIRT5 expression has been observed in various cancers and correlates with poor patient prognosis.
Given the contrasting oncogenic and tumor-suppressive effects of SIRT5, it is evident that the specific role of SIRT5 depends on its key target genes and the type of tumor. Additionally, studies have found that the function of SIRT5 also depends on whether it performs desuccinylation or deacetylation (6). Currently, research on SIRT5 presents many unresolved questions. The investigation into SIRT5-mediated desuccinylation activity is still in its infancy, and the relationships and mechanisms between SIRT5 and multiple cancers require further exploration to provide guidance for future cancer treatments.
The role of SIRT5-mediated desuccinylation in tumor progression underscores its potential as a therapeutic target for cancer treatment. In non-small cell lung cancer (NSCLC), quercetin has been shown to bind to SIRT5, thereby regulating SIRT5-mediated desuccinylation of PI3K. This interaction inhibits PI3K/AKT phosphorylation, subsequently blocking homologous recombination and non-homologous end-joining repair processes, leading to mitotic mutations and apoptosis, and ultimately alleviating NSCLC progression (162). In colorectal cancer (CRC), Zhang et al. (163) reported that extracellular vesicles isolated from Lactobacillus plantarum can inhibit SIRT5 expression, thus modulating the desuccinylation level of p53. This regulation leads to inhibition of CRC cell proliferation and glycolysis, effectively suppressing the in vivo growth of tumor tissues. Apart from pharmacological interventions, recent studies have identified upstream regulatory genes involved in tumor progression that affect SIRT5 expression. In hepatocellular carcinoma (HCC), Bai et al. (164) found that solute carrier family 25 member 20 (SLC25A51), a newly identified mammalian mitochondrial NAD+ transporter, is upregulated in human HCC specimens and cell lines. Further analysis revealed that SLC25A51 activates SIRT5 expression, promoting a metabolic shift from oxidative phosphorylation to glycolysis—a key mechanism driving tumor progression. Knockout of SLC25A51 reduces SIRT5 expression, thereby mitigating HCC progression. However, current research predominantly focuses on the oncogenic role of SIRT5, with limited information on enhancing its expression to exert tumor-suppressive effects. In gastric cancer, Tang et al. (165) demonstrated that SIRT5 expression is regulated by cyclin-dependent kinase 2 (CDK2). Typically, CDK2 exacerbates tumor progression by regulating cell cycle progression and DNA damage response. Conversely, knockout of CDK2 can inhibit malignant proliferation and aerobic glycolysis of cancer cells by increasing SIRT5 expression, revealing a novel role for SIRT5 as a tumor suppressor regulated by upstream genes in cancer.
The development of specific SIRT5 regulators has emerged as a promising approach in clinical cancer therapy. The information of specific SIRT5 regulators was showed in Table 3. Deng et al. (166) identified ϵ-N-thioglutaryl-lysine derivatives as potent inhibitors of SIRT5, with photo-crosslinking derivative 8 exhibiting the strongest inhibitory effect. Kinetic analysis revealed that these derivatives inhibit SIRT5 by competing with lysine substrates. Co-crystal structure analysis demonstrated that photo-crosslinking derivative 8 binds to SIRT5 via hydrogen bonds and electrostatic interactions with specific residues, occupying the lysine substrate binding site and potentially reacting with NAD+ to form a stable thio-intermediate. This structural insight provides valuable information for the design of drug-like inhibitors and cross-linking chemical probes for SIRT5-related research. Additionally, Jiang et al. (167) designed six N-terminal-to-side-chain cyclic tripeptides and evaluated their efficacy through in vitro deacetylase inhibition assays and proteolytic stability tests. Among these compounds, cyclic tripeptide 10 exhibited strong inhibition of SIRT5-mediated desuccinylation reactions and demonstrated superior proteolytic stability against SIRT5. Compared to previously reported potent and selective SIRT5 inhibitors, cyclic tripeptide 10 represents a novel modular scaffold, offering a new avenue for discovering improved SIRT5 inhibitors that could serve as chemical or pharmacological probes and potential treatments for tumors characterized by upregulated SIRT5-mediated desuccinylase activity. Regarding SIRT5 activators, MC3138, a selective SIRT5 activator, mimicked the effects of SIRT5 overexpression-mediated deacetylation and desuccinylation in pancreatic cancer cells, leading to reduced levels of metabolites such as glutamine and glutamate (168). Given that SIRT5 expression is downregulated in human and mouse pancreatic ductal adenocarcinomas, the application of MC3138 in pancreatic tumors showed inhibitory effects on proliferation. Combination treatment with gemcitabine may represent a therapeutic strategy for this type of cancer (169). Therefore, modulating SIRT5 expression appears to be an effective means of alleviating tumor progression. The ongoing development of small molecule inhibitors or activators of SIRT5 offers new strategies for future cancer treatments. As our understanding of SIRT5 functions deepens, its potential as a therapeutic target for cancer treatment becomes increasingly evident.
Despite the promising role of SIRT5 in cancer therapy, several challenges and future research directions must be addressed. A primary challenge is the need for a deeper understanding of the molecular mechanisms by which SIRT5 exerts its effects on tumor biology. Although SIRT5 has been implicated in various pathways, its specific interactions and regulatory networks within different cancer types remain inadequately defined. Future studies should focus on elucidating these mechanisms, potentially utilizing advanced genomic and proteomic approaches to comprehensively map SIRT5 interactions within the tumor microenvironment (170). Moreover, the development of specific SIRT5 inhibitors or modulators is critical for translating these findings into clinical applications. This includes investigating the potential side effects and off-target effects of such therapies, as well as assessing their efficacy when used in combination with existing cancer treatments. It will also be important to explore dose-response relationships and pharmacokinetic properties to ensure optimal therapeutic outcomes. Additionally, exploring the role of SIRT5 in immune modulation could open new avenues for immunotherapy, particularly in cancers that exhibit resistance to current therapies (171). Understanding how SIRT5 influences immune cell function and tumor-immune interactions may provide insights into novel therapeutic strategies that combine SIRT5 modulation with immunotherapeutic approaches. As research progresses, addressing these challenges will be essential for harnessing the full therapeutic potential of SIRT5 in oncology. The integration of multi-disciplinary approaches, including systems biology, computational modeling, and translational research, will be crucial for overcoming the complexities associated with SIRT5’s multifaceted roles in cancer. Addressing these issues will not only enhance our understanding of SIRT5’s biological functions but also pave the way for innovative cancer therapies targeting this enzyme.
SIRT5, functioning primarily as a desuccinylase, exhibits significant regulatory roles in tumor biology. Advances in proteomics have led to the recognition that SIRT5 is not merely a deacetylase but increasingly serves as a critical desuccinylase involved in modulating multiple metabolic pathways, including glycolysis, the TCA cycle, fatty acid metabolism, and ROS scavenging. SIRT5 plays a pivotal role in cellular energy metabolism and homeostasis, with its dysregulation being implicated in various types of cancer. These findings underscore the central importance of SIRT5 in tumor metabolic reprogramming, suggesting that both SIRT5 itself and the succinylation modifications it regulates could serve as promising targets for the development of novel anticancer therapies. However, the specific functions of SIRT5 in different types of tumors remain to be further elucidated, particularly given its dual nature as a potential tumor suppressor in some contexts and a tumor promoter in others. Consequently, the application of SIRT5 as a therapeutic target necessitates personalized research approaches tailored to specific tumor types and microenvironments. Future studies should focus on delineating the precise mechanisms by which SIRT5 exerts its effects, considering the complex interplay between SIRT5 activity, metabolic alterations, and tumor progression. By addressing these challenges, researchers can harness the therapeutic potential of SIRT5 to develop more effective and targeted anticancer strategies.
ZK: Writing – original draft, Writing – review & editing. KS: Conceptualization, Investigation, Software, Writing – review & editing. LW: Data curation, Investigation, Software, Writing – review & editing. HX: Data curation, Methodology, Software, Writing – review & editing. XP: Data curation, Methodology, Supervision, Writing – review & editing. ZQ: Formal Analysis, Methodology, Supervision, Writing – review & editing. YW: Formal Analysis, Methodology, Supervision, Writing – review & editing. TL: Formal Analysis, Project administration, Validation, Writing – review & editing. XZ: Formal Analysis, Project administration, Validation, Writing – review & editing. YS: Funding acquisition, Resources, Visualization, Writing – review & editing.
The author(s) declare that financial support was received for the research, authorship, and/or publication of this article. This work was supported by grants from the following sources: the National Natural Science Foundation of China (82370096, 82172728), Respiratory Disease -Jiangsu Province Medical Key Discipline Construction Unit (JSDW202208), Nanjing Science and Technology Project (YKK22220), and supported by Science Foundation of Kangda College of Nanjing Medical University (KD2023KYJJ224).
The authors declare that the research was conducted in the absence of any commercial or financial relationships that could be construed as a potential conflict of interest.
The author(s) declare that no Generative AI was used in the creation of this manuscript.
All claims expressed in this article are solely those of the authors and do not necessarily represent those of their affiliated organizations, or those of the publisher, the editors and the reviewers. Any product that may be evaluated in this article, or claim that may be made by its manufacturer, is not guaranteed or endorsed by the publisher.
1. Siegel RL, Miller KD, Wagle NS, Jemal A. Cancer statistics, 2023. Ca: A Cancer J Clin. (2023) 73:17–48. doi: 10.3322/caac.21763
2. Wang Y, Yan Q, Fan C, Mo Y, Wang Y, Li X, et al. Overview and countermeasures of cancer burden in China. Sci China Life Sci. (2023) 66:2515–26. doi: 10.1007/s11427-022-2240-6
3. Bray F, Laversanne M, Sung H, Ferlay J, Siegel RL, Soerjomataram I, et al. Global cancer statistics 2022: globocan estimates of incidence and mortality worldwide for 36 cancers in 185 countries. Ca: A Cancer J Clin. (2024) 74:229–63. doi: 10.3322/caac.21834
4. Pan S, Chen R. Pathological implication of protein post-translational modifications in cancer. Mol Aspects Med. (2022) 86:101097. doi: 10.1016/j.mam.2022.101097
5. Li Y, Zhang R, Hei H. Advances in post-translational modifications of proteins and cancer immunotherapy. Front Immunol. (2023) 14:1229397. doi: 10.3389/fimmu.2023.1229397
6. Wang Y, Chen H, Zha X. Overview of sirt5 as a potential therapeutic target: structure, function and inhibitors. Eur J Med Chem. (2022) 236:114363. doi: 10.1016/j.ejmech.2022.114363
7. Ren Z, Dong X, Guan L, Yang L, Liu C, Cai X, et al. Sirt5-mediated lysine desuccinylation regulates oxidative stress adaptation in magnaporthe oryzae during host intracellular infection. New Phytol. (2024) 242:1257–74. doi: 10.1111/nph.v242.3
8. Du J, Zhou Y, Su X, Yu JJ, Khan S, Jiang H, et al. Sirt5 is a nad-dependent protein lysine demalonylase and desuccinylase. Science. (2011) 334:806–09. doi: 10.1126/science.1207861
9. Mao J, Wang D, Wang D, Wu Q, Shang Q, Gao C, et al. Sirt5-related desuccinylation modification of aifm1 protects against compression-induced intervertebral disc degeneration by regulating mitochondrial homeostasis. Exp Mol Med. (2023) 55:253–68. doi: 10.1038/s12276-023-00928-y
10. Xiao Z, Lv T, Hou P, Manaenko A, Liu Y, Jin Y, et al. Sirtuin 5-mediated lysine desuccinylation protects mitochondrial metabolism following subarachnoid hemorrhage in mice. Stroke (1970). (2021) 52(12):4043–53. doi: 10.1161/STROKEAHA.121.034850
11. Chang X, Zhang Q, Huang Y, Liu J, Wang Y, Guan X, et al. Quercetin inhibits necroptosis in cardiomyocytes after ischemia-reperfusion via dna-pkcs-sirt5-orchestrated mitochondrial quality control. Phytother Res. (2024) 38:2496–517. doi: 10.1002/ptr.v38.5
12. Millán-Zambrano G, Burton A, Bannister AJ, Schneider R. Histone post-translational modifications - cause and consequence of genome function. Nat Rev Genet. (2022) 23(9):563–80. doi: 10.1038/s41576-022-00468-7
13. Müller S, Bley N, Busch B, Glaß M, Lederer M, Misiak C, et al. The oncofetal rna-binding protein igf2bp1 is a druggable, post-transcriptional super-enhancer of e2f-driven gene expression in cancer. Nucleic Acids Res. (2020) 48:8576–90. doi: 10.1093/nar/gkaa653
14. Holstein E, Dittmann A, Kaariainen A, Pesola V, Koivunen J, Pihlajaniemi T, et al. The burden of post-translational modification (ptm)-disrupting mutations in the tumor matrisome. Cancers (Basel). (2021) 13(5):1081. doi: 10.3390/cancers13051081
15. Hou X, Chen Y, Li X, Gu X, Dong W, Shi J, et al. Protein succinylation: regulating metabolism and beyond. Front Nutr (Lausanne). (2024) 11:1336057. doi: 10.3389/fnut.2024.1336057
16. Sreedhar A, Wiese EK, Hitosugi T. Enzymatic and metabolic regulation of lysine succinylation. Genes Dis. (2020) 7:166–71. doi: 10.1016/j.gendis.2019.09.011
17. Lian J, Liu W, Hu Q, Zhang X. Succinylation modification: a potential therapeutic target in stroke. Neural Regener Res. (2024) 19:781–87. doi: 10.4103/1673-5374.382229
18. Su Z, Li J, Lin J, Li Z, Che Y, Zhang Z, et al. Tnf-alpha-induced kat2a impedes bmmsc quiescence by mediating succinylation of the mitophagy-related protein vcp. Adv Sci (Weinh). (2024) 11(10):e2303388. doi: 10.1002/advs.202303388
19. Liu Z, Wang R, Wang Y, Duan Y, Zhan H. Targeting succinylation-mediated metabolic reprogramming as a potential approach for cancer therapy. BioMed Pharmacother. (2023) 168:115713. doi: 10.1016/j.biopha.2023.115713
20. Hu J, Chen J, Hou Q, Xu X, Ren J, Ma L, et al. Core-predominant gut fungus kazachstania slooffiae promotes intestinal epithelial glycolysis via lysine desuccinylation in pigs. Microbiome. (2023) 11:31. doi: 10.1186/s40168-023-01468-3
21. Wang L, Tao Y, Zhai J, Xue M, Zheng C, Hu H. The emerging roles of ac4c acetylation “writer” nat10 in tumorigenesis: a comprehensive review. Int J Biol Macromol. (2024) 254:127789. doi: 10.1016/j.ijbiomac.2023.127789
22. Yang L, Ma X, He Y, Yuan C, Chen Q, Li G, et al. Sirtuin 5: a review of structure, known inhibitors and clues for developing new inhibitors. Sci China Life Sci. (2017) 60:249–56. doi: 10.1007/s11427-016-0060-7
23. Hirschey MD, Zhao Y. Metabolic regulation by lysine malonylation, succinylation, and glutarylation. Mol Cell Proteomics. (2015) 14:2308–15. doi: 10.1074/mcp.R114.046664
24. Roessler C, Tuting C, Meleshin M, Steegborn C, Schutkowski M. A novel continuous assay for the deacylase sirtuin 5 and other deacetylases. J Med Chem. (2015) 58:7217–23. doi: 10.1021/acs.jmedchem.5b00293
25. Du Y, Hu H, Hua C, Du K, Wei T. Tissue distribution, subcellular localization, and enzymatic activity analysis of human sirt5 isoforms. Biochem Biophys Res Commun. (2018) 503:763–69. doi: 10.1016/j.bbrc.2018.06.073
26. Hershberger KA, Abraham DM, Martin AS, Mao L, Liu J, Gu H, et al. Sirtuin 5 is required for mouse survival in response to cardiac pressure overload. J Biol Chem. (2017) 292(48):19767–81. doi: 10.1074/jbc.M117.809897
27. Rardin MJ, He W, Nishida Y, Newman JC, Carrico C, Danielson SR, et al. Sirt5 regulates the mitochondrial lysine succinylome and metabolic networks. Cell Metab. (2013) 18:920–33. doi: 10.1016/j.cmet.2013.11.013
28. Wu M, Tan J, Cao Z, Cai Y, Huang Z, Chen Z, et al. Sirt5 improves cardiomyocytes fatty acid metabolism and ameliorates cardiac lipotoxicity in diabetic cardiomyopathy via cpt2 de-succinylation. Redox Biol. (2024) 73:103184. doi: 10.1016/j.redox.2024.103184
29. Sadhukhan S, Liu X, Ryu D, Nelson OD, Stupinski JA, Li Z, et al. Metabolomics-assisted proteomics identifies succinylation and sirt5 as important regulators of cardiac function. Proc Natl Acad Sci U S A. (2016) 113:4320–25. doi: 10.1073/pnas.1519858113
30. Ungurianu A, Zanfirescu A, Margină D. Sirtuins, resveratrol and the intertwining cellular pathways connecting them. Ageing Res Rev. (2023) 88:101936. doi: 10.1016/j.arr.2023.101936
31. Osborne B, Bentley NL, Montgomery MK, Turner N. The role of mitochondrial sirtuins in health and disease. Free Radical Biol Med. (2016) 100:164–74. doi: 10.1016/j.freeradbiomed.2016.04.197
32. Gertz M, Steegborn C. Using mitochondrial sirtuins as drug targets: disease implications and available compounds. Cell Mol Life Sciences: Cmls. (2016) 73:2871–96. doi: 10.1007/s00018-016-2180-7
33. Zhu X, Su Q, Xie H, Song L, Yang F, Zhang D, et al. Sirt1 deacetylates wee1 and sensitizes cancer cells to wee1 inhibition. Nat Chem Biol. (2023) 19:585–95. doi: 10.1038/s41589-022-01240-y
34. Wang X, Wang J, Zhao X, Wu H, Li J, Cheng Y, et al. Mettl3-mediated m6a modification of sirt1 mrna inhibits progression of endometriosis by cellular senescence enhancing. J Transl Med. (2023) 21:407. doi: 10.1186/s12967-023-04209-0
35. Rasti G, Becker M, Vazquez BN, Espinosa-Alcantud M, Fernández-Duran I, Gámez-García A, et al. Sirt1 regulates dna damage signaling through the pp4 phosphatase complex. Nucleic Acids Res. (2023) 51:6754–69. doi: 10.1093/nar/gkad504
36. Shen S, Shen M, Kuang L, Yang K, Wu S, Liu X, et al. Sirt1/srebps-mediated regulation of lipid metabolism. Pharmacol Res. (2024) 199:107037. doi: 10.1016/j.phrs.2023.107037
37. Wang X, Jiang Y, Ye W, Shao C, Xie J, Li X. Sirt1 promotes the progression and chemoresistance of colorectal cancer through the p53/mir-101/kpna3 axis. Cancer Biol Ther. (2023) 24:2235770. doi: 10.1080/15384047.2023.2235770
38. Wei Z, Xia J, Li J, Cai J, Shan J, Zhang C, et al. Sirt1 promotes glucolipid metabolic conversion to facilitate tumor development in colorectal carcinoma. Int J Biol Sci. (2023) 19:1925–40. doi: 10.7150/ijbs.76704
39. Dilmac S, Kuscu N, Caner A, Yildirim S, Yoldas B, Farooqi AA, et al. Sirt1/foxo signaling pathway in breast cancer progression and metastasis. Int J Mol Sci. (2022) 23:10227. doi: 10.3390/ijms231810227
40. Bialopiotrowicz-Data E, Noyszewska-Kania M, Jablonska E, Sewastianik T, Komar D, Debek S, et al. Sirt1 and hsp90alpha feed-forward circuit safeguards chromosome segregation integrity in diffuse large b cell lymphomas. Cell Death Dis. (2023) 14(10):667. doi: 10.1038/s41419-023-06186-0
41. Choi HK, Cho KB, Phuong NT, Han CY, Han HK, Hien TT, et al. Sirt1-mediated foxo1 deacetylation is essential for multidrug resistance-associated protein 2 expression in tamoxifen-resistant breast cancer cells. Mol Pharm. (2013) 10:2517–27. doi: 10.1021/mp400287p
42. Dai JM, Wang ZY, Sun DC, Lin RX, Wang SQ. Sirt1 interacts with p73 and suppresses p73-dependent transcriptional activity. J Cell Physiol. (2007) 210:161–66. doi: 10.1002/jcp.v210:1
43. Li K, Casta A, Wang R, Lozada E, Fan W, Kane S, et al. Regulation of wrn protein cellular localization and enzymatic activities by sirt1-mediated deacetylation. J Biol Chem. (2008) 283:7590–98. doi: 10.1074/jbc.M709707200
44. Yang X, Chang H, Tatekoshi Y, Mahmoodzadeh A, Balibegloo M, Najafi Z, et al. Sirt2 inhibition protects against cardiac hypertrophy and ischemic injury. Elife. (2023) 12(20):e85571. doi: 10.7554/eLife.85571
45. Sola-Sevilla N, Mesa-Lombardo A, Aleixo M, Expósito S, Diaz-Perdigón T, Azqueta A, et al. Sirt2 inhibition rescues neurodegenerative pathology but increases systemic inflammation in a transgenic mouse model of alzheimer’s disease. J Neuroimmune Pharmacol. (2023) 18:529–50. doi: 10.1007/s11481-023-10084-9
46. Wang M, Zhang Y, Komaniecki GP, Lu X, Cao J, Zhang M, et al. Golgi stress induces sirt2 to counteract shigella infection via defatty-acylation. Nat Commun. (2022) 13:4494. doi: 10.1038/s41467-022-32227-x
47. Chen G, Huang P, Hu C. The role of sirt2 in cancer: a novel therapeutic target. Int J Cancer. (2020) 147:3297–304. doi: 10.1002/ijc.v147.12
48. Zhang M, Wu J, Sun R, Tao X, Wang X, Kang Q, et al. Sirt5 deficiency suppresses mitochondrial atp production and promotes ampk activation in response to energy stress. PLoS One. (2019) 14:e211796. doi: 10.1371/journal.pone.0211796
49. Ciarlo E, Heinonen T, Théroude C, Herderschee J, Mombelli M, Lugrin J, et al. Sirtuin 2 deficiency increases bacterial phagocytosis by macrophages and protects from chronic staphylococcal infection. Front Immunol. (2017) 8:1037. doi: 10.3389/fimmu.2017.01037
50. Liu J, Li D, Zhang T, Tong Q, Ye RD, Lin L. Sirt3 protects hepatocytes from oxidative injury by enhancing ros scavenging and mitochondrial integrity. Cell Death Dis. (2017) 8:e3158. doi: 10.1038/cddis.2017.564
51. Gong Y, Tang N, Liu P, Sun Y, Lu S, Liu W, et al. Newcastle disease virus degrades sirt3 via pink1-prkn-dependent mitophagy to reprogram energy metabolism in infected cells. Autophagy. (2022) 18:1503–21. doi: 10.1080/15548627.2021.1990515
52. Chen J, Wang A, Chen Q. Sirt3 and p53 deacetylation in aging and cancer. J Cell Physiol. (2017) 232:2308–11. doi: 10.1002/jcp.v232.9
53. Zhao Q, Zhou J, Li F, Guo S, Zhang L, Li J, et al. The role and therapeutic perspectives of sirtuin 3 in cancer metabolism reprogramming, metastasis, and chemoresistance. Front Oncol. (2022) 12:910963. doi: 10.3389/fonc.2022.910963
54. Liu L, Li Y, Cao D, Qiu S, Li Y, Jiang C, et al. Sirt3 inhibits gallbladder cancer by induction of akt-dependent ferroptosis and blockade of epithelial-mesenchymal transition. Cancer Lett. (2021) 510:93–104. doi: 10.1016/j.canlet.2021.04.007
55. Paku M, Haraguchi N, Takeda M, Fujino S, Ogino T, Takahashi H, et al. Sirt3-mediated sod2 and pgc-1alpha contribute to chemoresistance in colorectal cancer cells. Ann Surg Oncol. (2021) 28:4720–32. doi: 10.1245/s10434-020-09373-x
56. Ge Y, Wu X, Cai Y, Hu Q, Wang J, Zhang S, et al. Correction: fndc5 prevents oxidative stress and neuronal apoptosis after traumatic brain injury through sirt3-dependent regulation of mitochondrial quality control. Cell Death Dis. (2024) 15:524–27. doi: 10.1038/s41419-024-06901-5
57. Rajabi N, Hansen TN, Nielsen AL, Nguyen HT, Baek M, Bolding JE, et al. Investigation of carboxylic acid isosteres and prodrugs for inhibition of the human sirt5 lysine deacylase enzyme. Angew Chem Int Ed Engl. (2022) 61(22):e202115805. doi: 10.1002/anie.202115805
58. Zhang K, Mishra A, Jagannath C. New insight into arginine and tryptophan metabolism in macrophage activation during tuberculosis. Front Immunol. (2024) 15:1363938. doi: 10.3389/fimmu.2024.1363938
59. Jiang W, Ouyang X, Ji Z, Shi W, Wu Y, Yao Q, et al. The pik3ca-e545k-sirt4 signaling axis reduces radiosensitivity by promoting glutamine metabolism in cervical cancer. Cancer Lett. (2023) 556:216064. doi: 10.1016/j.canlet.2023.216064
60. Cai G, Ge Z, Xu Y, Cai L, Sun P, Huang G. Sirt4 functions as a tumor suppressor during prostate cancer by inducing apoptosis and inhibiting glutamine metabolism. Sci Rep. (2022) 12:12208. doi: 10.1038/s41598-022-16610-8
61. Wang L, Zhou H, Wang Y, Cui G, Di LJ. Ctbp maintains cancer cell growth and metabolic homeostasis via regulating sirt4. Cell Death Dis. (2015) 6:e1620. doi: 10.1038/cddis.2014.587
62. Miyo M, Yamamoto H, Konno M, Colvin H, Nishida N, Koseki J, et al. Tumour-suppressive function of sirt4 in human colorectal cancer. Br J Cancer. (2015) 113:492–99. doi: 10.1038/bjc.2015.226
63. Nakahara Y, Yamasaki M, Sawada G, Miyazaki Y, Makino T, Takahashi T, et al. Downregulation of sirt4 expression is associated with poor prognosis in esophageal squamous cell carcinoma. Oncology. (2016) 90:347–55. doi: 10.1159/000445323
64. Chen Z, Lin J, Feng S, Chen X, Huang H, Wang C, et al. Sirt4 inhibits the proliferation, migration, and invasion abilities of thyroid cancer cells by inhibiting glutamine metabolism. Onco Targets Ther. (2019) 12:2397–408. doi: 10.2147/OTT.S189536
65. Chang AR, Ferrer CM, Mostoslavsky R. Sirt6, a mammalian deacylase with multitasking abilities. Physiol Rev. (2020) 100:145–69. doi: 10.1152/physrev.00030.2018
66. You Q, Wang J, Yu Y, Li F, Meng L, Chen M, et al. The histone deacetylase sirt6 promotes glycolysis through the hif-1alpha/hk2 signaling axis and induces erlotinib resistance in non-small cell lung cancer. Apoptosis. (2022) 27:883–98. doi: 10.1007/s10495-022-01751-y
67. Sample KM. Dna repair gene expression is associated with differential prognosis between hpv16 and hpv18 positive cervical cancer patients following radiation therapy. Sci Rep. (2020) 10:2774. doi: 10.1038/s41598-020-59383-8
68. Yang J, Li Y, Zhang Y, Fang X, Chen N, Zhou X, et al. Sirt6 promotes tumorigenesis and drug resistance of diffuse large b-cell lymphoma by mediating pi3k/akt signaling. J Exp Clin Cancer Res. (2020) 39:142. doi: 10.1186/s13046-020-01623-w
69. Raza U, Tang X, Liu Z, Liu B. Sirt7: the seventh key to unlocking the mystery of aging. Physiol Rev. (2024) 104:253–80. doi: 10.1152/physrev.00044.2022
70. Xiang J, Zhang N, Sun H, Su L, Zhang C, Xu H, et al. Disruption of sirt7 increases the efficacy of checkpoint inhibitor via mef2d regulation of programmed cell death 1 ligand 1 in hepatocellular carcinoma cells. Gastroenterology. (2020) 158:664–78. doi: 10.1053/j.gastro.2019.10.025
71. Huo Q, Chen S, Zhuang J, Quan C, Wang Y, Xie N. Sirt7 downregulation promotes breast cancer metastasis via lap2alpha-induced chromosomal instability. Int J Biol Sci. (2023) 19:1528–42. doi: 10.7150/ijbs.75340
72. Li H, Tian Z, Qu Y, Yang Q, Guan H, Shi B, et al. Sirt7 promotes thyroid tumorigenesis through phosphorylation and activation of akt and p70s6k1 via dbc1/sirt1 axis. Oncogene. (2019) 38:345–59. doi: 10.1038/s41388-018-0434-6
73. Yu W, Cui X, Wan Z, Yu Y, Liu X, Jin L. Silencing forkhead box m1 promotes apoptosis and autophagy through sirt7/mtor/igf2 pathway in gastric cancer cells. J Cell Biochem. (2018) 119:9090–98. doi: 10.1002/jcb.v119.11
74. Natani S, Dhople VM, Parveen A, Sruthi KK, Khilar P, Bhukya S, et al. Ampk/sirt1 signaling through p38mapk mediates interleukin-6 induced neuroendocrine differentiation of lncap prostate cancer cells. Biochim Et Biophys Acta Mol Cell Res. (2021) 1868:119085. doi: 10.1016/j.bbamcr.2021.119085
75. Yao Y, Hua Q, Zhou Y, Shen H. Circrna has_circ_0001946 promotes cell growth in lung adenocarcinoma by regulating mir-135a-5p/sirt1 axis and activating wnt/beta-catenin signaling pathway. BioMed Pharmacother. (2019) 111:1367–75. doi: 10.1016/j.biopha.2018.12.120
76. Sasca D, Hahnel PS, Szybinski J, Khawaja K, Kriege O, Pante SV, et al. Sirt1 prevents genotoxic stress-induced p53 activation in acute myeloid leukemia. Blood. (2014) 124:121–33. doi: 10.1182/blood-2013-11-538819
77. Zhang S, Yang Y, Huang S, Deng C, Zhou S, Yang J, et al. Sirt1 inhibits gastric cancer proliferation and metastasis via stat3/mmp-13 signaling. J Cell Physiol. (2019) 234:15395–406. doi: 10.1002/jcp.v234.9
78. Deng A, Ning Q, Zhou L, Liang Y. Sirt2 is an unfavorable prognostic biomarker in patients with acute myeloid leukemia. Sci Rep. (2016) 6:27694. doi: 10.1038/srep27694
79. Zhao N, Guo Y, Liu P, Chen Y, Wang Y. Sirtuin 2 promotes cell stemness and mek/erk signaling pathway while reduces chemosensitivity in endometrial cancer. Arch Gynecol Obstet. (2022) 305:693–701. doi: 10.1007/s00404-021-06216-2
80. Zhou W, Ni TK, Wronski A, Glass B, Skibinski A, Beck A, et al. The sirt2 deacetylase stabilizes slug to control Malignancy of basal-like breast cancer. Cell Rep. (2016) 17:1302–17. doi: 10.1016/j.celrep.2016.10.006
81. Fiskus W, Coothankandaswamy V, Chen J, Ma H, Ha K, Saenz DT, et al. Sirt2 deacetylates and inhibits the peroxidase activity of peroxiredoxin-1 to sensitize breast cancer cells to oxidant stress-inducing agents. Cancer Res. (2016) 76:5467–78. doi: 10.1158/0008-5472.CAN-16-0126
82. Chen J, Chan AW, To KF, Chen W, Zhang Z, Ren J, et al. Sirt2 overexpression in hepatocellular carcinoma mediates epithelial to mesenchymal transition by protein kinase b/glycogen synthase kinase-3beta/beta-catenin signaling. Hepatology. (2013) 57:2287–98. doi: 10.1002/hep.26278
83. Cui Y, Qin L, Wu J, Qu X, Hou C, Sun W, et al. Sirt3 enhances glycolysis and proliferation in sirt3-expressing gastric cancer cells. PloS One. (2015) 10:e129834. doi: 10.1371/journal.pone.0129834
84. Ullah A, Rehman NU, Islam WU, Khan F, Waqas M, Halim SA, et al. Identification of small molecular inhibitors of sirt3 by computational and biochemical approaches a potential target of breast cancer. Sci Rep. (2024) 14:12475. doi: 10.1038/s41598-024-63177-7
85. Liao M, Sun X, Zheng W, Wu M, Wang Y, Yao J, et al. Linc00922 decoys sirt3 to facilitate the metastasis of colorectal cancer through up-regulation the h3k27 crotonylation of ets1 promoter. Mol Cancer. (2023) 22:163. doi: 10.1186/s12943-023-01859-y
86. Li X, Zhang W, Xing Z, Hu S, Zhang G, Wang T, et al. Targeting sirt3 sensitizes glioblastoma to ferroptosis by promoting mitophagy and inhibiting slc7a11. Cell Death Dis. (2024) 15:168. doi: 10.1038/s41419-024-06558-0
87. Xiang XY, Kang JS, Yang XC, Su J, Wu Y, Yan XY, et al. Sirt3 participates in glucose metabolism interruption and apoptosis induced by bh3 mimetic s1 in ovarian cancer cells. Int J Oncol. (2016) 49:773–84. doi: 10.3892/ijo.2016.3552
88. Zhang J, Han L, Yu J, Li H, Li Q. Mir-224 aggravates cancer-associated fibroblast-induced progression of non-small cell lung cancer by modulating a positive loop of the sirt3/ampk/mtor/hif-1alpha axis. Aging (Albany Ny). (2021) 13:10431–49. doi: 10.18632/aging.202803
89. Jin J, Bai L, Wang D, Ding W, Cao Z, Yan P, et al. Sirt3-dependent delactylation of cyclin e2 prevents hepatocellular carcinoma growth. EMBO Rep. (2023) 24:e56052. doi: 10.15252/embr.202256052
90. Wang YS, Du L, Liang X, Meng P, Bi L, Wang YL, et al. Sirtuin 4 depletion promotes hepatocellular carcinoma tumorigenesis through regulating adenosine-monophosphate-activated protein kinase alpha/mammalian target of rapamycin axis in mice. Hepatology. (2019) 69:1614–31. doi: 10.1002/hep.30421
91. Sun H, Huang D, Liu G, Jian F, Zhu J, Zhang L. Sirt4 acts as a tumor suppressor in gastric cancer by inhibiting cell proliferation, migration, and invasion. Onco Targets Ther. (2018) 11:3959–68. doi: 10.2147/OTT.S156143
92. Fu L, Dong Q, He J, Wang X, Xing J, Wang E, et al. Sirt4 inhibits Malignancy progression of nsclcs, through mitochondrial dynamics mediated by the erk-drp1 pathway. Oncogene. (2017) 36:2724–36. doi: 10.1038/onc.2016.425
93. Wang C, Piao C, Liu J, Zhang Z, Zhu Y, Kong C. Mammalian sirt4 is a tumor suppressor of clear cell renal cell carcinoma by inhibiting cancer proliferation, migration and invasion. Cancer biomark. (2020) 29:453–62. doi: 10.3233/CBM-191253
94. Shi L, Yan H, An S, Shen M, Jia W, Zhang R, et al. Sirt5-mediated deacetylation of ldhb promotes autophagy and tumorigenesis in colorectal cancer. Mol Oncol. (2019) 13:358–75. doi: 10.1002/mol2.2019.13.issue-2
95. Li Z, Yu DP, Wang N, Tao T, Luo W, Chen H. Sirt5 promotes non-small cell lung cancer progression by reducing fabp4 acetylation level. Neoplasma. (2022) 69:909–17. doi: 10.4149/neo_2022_220107N28
96. Guan J, Jiang X, Gai J, Sun X, Zhao J, Li J, et al. Sirtuin 5 regulates the proliferation, invasion and migration of prostate cancer cells through acetyl-coa acetyltransferase 1. J Cell Mol Med. (2020) 24:14039–49. doi: 10.1111/jcmm.v24.23
97. Chen XF, Tian MX, Sun RQ, Zhang ML, Zhou LS, Jin L, et al. Sirt5 inhibits peroxisomal acox1 to prevent oxidative damage and is downregulated in liver cancer. EMBO Rep. (2018) 19(5):e45124. doi: 10.15252/embr.201745124
98. Han LL, Jia L, Wu F, Huang C. Sirtuin6 (sirt6) promotes the emt of hepatocellular carcinoma by stimulating autophagic degradation of e-cadherin. Mol Cancer Res. (2019) 17:2267–80. doi: 10.1158/1541-7786.MCR-19-0321
99. Han Q, Xie QR, Li F, Cheng Y, Wu T, Zhang Y, et al. Targeted inhibition of sirt6 via engineered exosomes impairs tumorigenesis and metastasis in prostate cancer. Theranostics. (2021) 11:6526–41. doi: 10.7150/thno.53886
100. Becherini P, Caffa I, Piacente F, Damonte P, Vellone VG, Passalacqua M, et al. Sirt6 enhances oxidative phosphorylation in breast cancer and promotes mammary tumorigenesis in mice. Cancer Metab. (2021) 9:6. doi: 10.1186/s40170-021-00240-1
101. Cea M, Cagnetta A, Adamia S, Acharya C, Tai YT, Fulciniti M, et al. Evidence for a role of the histone deacetylase sirt6 in dna damage response of multiple myeloma cells. Blood. (2016) 127:1138–50. doi: 10.1182/blood-2015-06-649970
102. Abbotto E, Miro C, Piacente F, Salis A, Murolo M, Nappi A, et al. Sirt6 pharmacological inhibition delays skin cancer progression in the squamous cell carcinoma. BioMed Pharmacother. (2023) 166:115326. doi: 10.1016/j.biopha.2023.115326
103. Feng J, Yan PF, Zhao HY, Zhang FC, Zhao WH, Feng M. Sirt6 suppresses glioma cell growth via induction of apoptosis, inhibition of oxidative stress and suppression of jak2/stat3 signaling pathway activation. Oncol Rep. (2016) 35:1395–402. doi: 10.3892/or.2015.4477
104. Shang J, Zhu Z, Chen Y, Song J, Huang Y, Song K, et al. Small-molecule activating sirt6 elicits therapeutic effects and synergistically promotes anti-tumor activity of vitamin d(3) in colorectal cancer. Theranostics. (2020) 10:5845–64. doi: 10.7150/thno.44043
105. Zhang G, Liu Z, Qin S, Li K. Decreased expression of sirt6 promotes tumor cell growth correlates closely with poor prognosis of ovarian cancer. Eur J Gynaecol Oncol. (2015) 36(6):629–32.
106. Xiong L, Tan B, Lei X, Zhang B, Li W, Liu D, et al. Sirt6 through pi3k/akt/mtor signaling pathway to enhance radiosensitivity of non-small cell lung cancer and inhibit tumor progression. IUBMB Life. (2021) 73:1092–102. doi: 10.1002/iub.v73.9
107. Zhang ZG, Qin CY. Sirt6 suppresses hepatocellular carcinoma cell growth via inhibiting the extracellular signal−regulated kinase signaling pathway. Mol Med Rep. (2014) 9:882–88. doi: 10.3892/mmr.2013.1879
108. Gu Y, Ding C, Yu T, Liu B, Tang W, Wang Z, et al. Sirt7 promotes hippo/yap activation and cancer cell proliferation in hepatocellular carcinoma via suppressing mst1. Cancer Sci. (2024) 115:1209–23. doi: 10.1111/cas.v115.4
109. Zhang S, Chen P, Huang Z, Hu X, Chen M, Hu S, et al. Sirt7 promotes gastric cancer growth and inhibits apoptosis by epigenetically inhibiting mir-34a. Sci Rep. (2015) 5:9787. doi: 10.1038/srep09787
110. Yu H, Ye W, Wu J, Meng X, Liu R, Ying X, et al. Overexpression of sirt7 exhibits oncogenic property and serves as a prognostic factor in colorectal cancer. Clin Cancer Res. (2014) 20:3434–45. doi: 10.1158/1078-0432.CCR-13-2952
111. Li G, Xu W, Li X, Chen M, Shi Y, Wei M, et al. Oncogenic sirt7 inhibits gata4 transcriptional activity and activates the wnt signaling pathway in ovarian cancer. Gynecol Oncol. (2023) 171:39–48. doi: 10.1016/j.ygyno.2022.12.005
112. Yu J, Yuan S, Song J, Yu S. Usp39 interacts with sirt7 to promote cervical squamous cell carcinoma by modulating autophagy and oxidative stress via foxm1. J Transl Med. (2023) 21:807. doi: 10.1186/s12967-023-04623-4
113. Lee JM, Hammarén HM, Savitski MM, Baek SH. Control of protein stability by post-translational modifications. Nat Commun. (2023) 14:201. doi: 10.1038/s41467-023-35795-8
114. Alleyn M, Breitzig M, Lockey R, Kolliputi N. The dawn of succinylation: a posttranslational modification. Am J Physiology: Cell Physiol. (2018) 314:C228–32. doi: 10.1152/ajpcell.00148.2017
115. Yang G, Yuan Y, Yuan H, Wang J, Yun H, Geng Y, et al. Histone acetyltransferase 1 is a succinyltransferase for histones and non-histones and promotes tumorigenesis. EMBO Rep. (2021) 22:e50967. doi: 10.15252/embr.202050967
116. Liu S, Li R, Sun Y, Lin H, Li H. Protein succinylation, hepatic metabolism, and liver diseases. World J Hepatol. (2024) 16:344–52. doi: 10.4254/wjh.v16.i3.344
117. Katsuta N, Nagai M, Saruwatari K, Nakamura M, Nagai R. Mitochondrial stress and glycoxidation increase with decreased kidney function. J Clin Biochem Nutr. (2023) 72:147–56. doi: 10.3164/jcbn.22-101
118. Zhan QY, Xie LX, Wang C. Promoting critical care system and capacity building in pulmonary and critical care medicine subspecialties. Zhong Hua Yi Xue Za Zhi. (2023) 103(40):3149–51. doi: 10.3760/cma.j.cn112137-20230602-00919
119. Mu R, Ma Z, Lu C, Wang H, Cheng X, Tuo B, et al. Role of succinylation modification in thyroid cancer and breast cancer. Am J Cancer Res. (2021) 11(10):4683–99.
120. Carrico C, Cruz A, Walter M, Meyer J, Wehrfritz C, Shah S, et al. Coenzyme a binding sites induce proximal acylation across protein families. Sci Rep. (2023) 13:5029. doi: 10.1038/s41598-023-31900-5
121. Chen H, Xu H, Potash S, Starkov A, Belousov VV, Bilan DS, et al. Mild metabolic perturbations alter succinylation of mitochondrial proteins. J Neurosci Res. (2017) 95:2244–52. doi: 10.1002/jnr.v95.11
122. Liu J, Shangguan Y, Tang D, Dai Y. Histone succinylation and its function on the nucleosome. J Cell Mol Med. (2021) 25:7101–09. doi: 10.1111/jcmm.v25.15
123. Jing Y, Tian G, Qin X, Liu Z, Li XD. Lysine succinylation on non-histone chromosomal protein hmg-17 (hmgn2) regulates nucleosomal dna accessibility by disrupting the hmgn2-nucleosome association. Rsc Chem Biol. (2021) 2:1257–62. doi: 10.1039/D1CB00070E
124. Smestad J, Erber L, Chen Y, Maher LJ. Chromatin succinylation correlates with active gene expression and is perturbed by defective tca cycle metabolism. Iscience. (2018) 2:63–75. doi: 10.1016/j.isci.2018.03.012
125. Karunanidhi A, Basu S, Zhao XJ, D’Annibale O, Van’T LC, Vockley J, et al. Heptanoic and medium branched-chain fatty acids as anaplerotic treatment for medium chain acyl-coa dehydrogenase deficiency. Mol Genet Metab. (2023) 140:107689. doi: 10.1016/j.ymgme.2023.107689
126. Gut P, Matilainen S, Meyer JG, Pällijeff P, Richard J, Carroll CJ, et al. Sucla2 mutations cause global protein succinylation contributing to the pathomechanism of a hereditary mitochondrial disease. Nat Commun. (2020) 11:5927. doi: 10.1038/s41467-020-19743-4
127. Takada S, Maekawa S, Furihata T, Kakutani N, Setoyama D, Ueda K, et al. Succinyl-coa-based energy metabolism dysfunction in chronic heart failure. Proc Natl Acad Sci - Pnas. (2022) 119:1–2091339177. doi: 10.1073/pnas.2203628119
128. Zhao X, Yang X, Du C, Hao H, Liu S, Liu G, et al. Up-regulated succinylation modifications induce a senescence phenotype in microglia by altering mitochondrial energy metabolism. J Neuroinflammation. (2024) 21:221–96. doi: 10.1186/s12974-024-03284-4
129. Zhao Y, Han Y, Sun Y, Wei Z, Chen J, Niu X, et al. Comprehensive succinylome profiling reveals the pivotal role of lysine succinylation in energy metabolism and quorum sensing of staphylococcus epidermidis. Front Microbiol. (2020) 11:632367. doi: 10.3389/fmicb.2020.632367
130. Lu W, Che X, Qu X, Zheng C, Yang X, Bao B, et al. Succinylation regulators promote clear cell renal cell carcinoma by immune regulation and rna n6-methyladenosine methylation. Front Cell Dev Biol. (2021) 9:622198. doi: 10.3389/fcell.2021.622198
131. Lancaster MS, Kim B, Doud EH, Tate MD, Sharify AD, Gao H, et al. Loss of succinyl-coa synthetase in mouse forebrain results in hypersuccinylation with perturbed neuronal transcription and metabolism. Cell Rep. (2023) 42:113241. doi: 10.1016/j.celrep.2023.113241
132. Hang T, Chen W, Wu M, Zhan L, Wang C, Jia N, et al. Structural insights into the molecular mechanism underlying sirt5-catalyzed desuccinylation of histone peptides. Biochem J. (2019) 476:211–23. doi: 10.1042/BCJ20180745
133. Zhang X, Ling C, Xiong Z, Gong T, Luo S, Liu X, et al. Desuccinylation of tbk1 by sirt5 regulates inflammatory response of macrophages in sepsis. Cell Rep (Cambridge). (2024) 43:115060. doi: 10.1016/j.celrep.2024.115060
134. Ruan J, Zheng J, Zhang X, Chen Z, Sun Y, Jia X. Sirt5 suppresses the trophoblast cell proliferation, invasion, and migration to promote preeclampsia via desuccinylating hoxb3. J Assist Reprod Genet. (2024) 41:2759–70. doi: 10.1007/s10815-024-03223-5
135. Li J, Lu L, Liu L, Ren X, Chen J, Yin X, et al. Hdac1/2/3 are major histone desuccinylases critical for promoter desuccinylation. Cell Discovery. (2023) 9:85. doi: 10.1038/s41421-023-00573-9
136. Colak G, Xie Z, Zhu AY, Dai L, Lu Z, Zhang Y, et al. Identification of lysine succinylation substrates and the succinylation regulatory enzyme cobb in escherichia coli. Mol Cell Proteomics. (2013) 12:3509–20. doi: 10.1074/mcp.M113.031567
137. Zhang H, Li P, Ren S, Cheng Z, Zhao G, Zhao W. Sc cobb2-mediated lysine desuccinylation regulates protein biosynthesis and carbon metabolism in streptomyces coelicolor. Mol Cell Proteomics. (2019) 18:2003. doi: 10.1074/mcp.RA118.001298
138. Maurer B, Rumpf T, Scharfe M, Stolfa DA, Schmitt ML, He W, et al. Inhibitors of the nad(+)-dependent protein desuccinylase and demalonylase sirt5. ACS Med Chem Lett. (2012) 3:1050–53. doi: 10.1021/ml3002709
139. Covarrubias AJ, Perrone R, Grozio A, Verdin E. Nad + metabolism and its roles in cellular processes during ageing. Nat Rev Mol Cell Biol. (2021) 22:119–41. doi: 10.1038/s41580-020-00313-x
140. Yu J, Sadhukhan S, Noriega LG, Moullan N, He B, Weiss RS, et al. Metabolic characterization of a sirt5 deficient mouse model. Sci Rep. (2013) 3:2806. doi: 10.1038/srep02806
141. Jukarainen S, Heinonen S, Rämö JT, Rinnankoski-Tuikka R, Rappou E, Tummers M, et al. Obesity is associated with low nad+/sirt pathway expression in adipose tissue of bmi-discordant monozygotic twins. J Clin Endocrinol Metab. (2016) 101:275–83. doi: 10.1210/jc.2015-3095
142. Liu L, Wang Q, Zhao B, Wu Q, Wang P. Exogenous nicotinamide adenine dinucleotide administration alleviates ischemia/reperfusion-induced oxidative injury in isolated rat hearts via sirt5-sdh-succinate pathway. Eur J Pharmacol. (2019) 858:172520. doi: 10.1016/j.ejphar.2019.172520
143. Morris-Blanco KC, Dave KR, Saul I, Koronowski KB, Stradecki HM, Perez-Pinzon MA. Protein kinase c epsilon promotes cerebral ischemic tolerance via modulation of mitochondrial sirt5. Sci Rep. (2016) 6:29790. doi: 10.1038/srep29790
144. Wang CH, Wei YH. Roles of mitochondrial sirtuins in mitochondrial function, redox homeostasis, insulin resistance and type 2 diabetes. Int J Mol Sci. (2020) 21(15):5266. doi: 10.3390/ijms21155266
145. Shen R, Ruan H, Lin S, Liu B, Song H, Li L, et al. Lysine succinylation, the metabolic bridge between cancer and immunity. Genes Dis. (2023) 10:2470–78. doi: 10.1016/j.gendis.2022.10.028
146. Bezwada D, Brugarolas J. Reporting on fh-deficient renal cell carcinoma using circulating succinylated metabolites. J Clin Invest. (2023) 133:1–03. doi: 10.1172/JCI170195
147. Teng P, Cui K, Yao S, Fei B, Ling F, Li C, et al. Sirt5-mediated me2 desuccinylation promotes cancer growth by enhancing mitochondrial respiration. Cell Death Differ. (2024) 31:65–77. doi: 10.1038/s41418-023-01240-y
148. Greene KS, Lukey MJ, Wang X, Blank B, Druso JE, Lin MJ, et al. Sirt5 stabilizes mitochondrial glutaminase and supports breast cancer tumorigenesis. Proc Natl Acad Sci - Pnas. (2019) 116:26625–32. doi: 10.1073/pnas.1911954116
149. Sun X, Wang S, Gai J, Guan J, Li J, Li Y, et al. Sirt5 promotes cisplatin resistance in ovarian cancer by suppressing dna damage in a ros-dependent manner via regulation of the nrf2/ho-1 pathway. Front Oncol. (2019) 9:754. doi: 10.3389/fonc.2019.00754
150. Meng L, Chen D, Meng G, Lu L, Han C. Dysregulation of the sirt5/idh2 axis contributes to sunitinib resistance in human renal cancer cells. FEBS Open Bio. (2021) 11:921–31. doi: 10.1002/2211-5463.13090
151. Lu X, Yang P, Zhao X, Jiang M, Hu S, Ouyang Y, et al. Ogdh mediates the inhibition of sirt5 on cell proliferation and migration of gastric cancer. Exp Cell Res. (2019) 382:111483. doi: 10.1016/j.yexcr.2019.06.028
152. Choi SY, Jeon JM, Na AY, Kwon OK, Bang IH, Ha YS, et al. Sirt5 directly inhibits the pi3k/akt pathway in prostate cancer cell lines. Cancer Genomics Proteomics. (2022) 19:50–9. doi: 10.21873/cgp.20303
153. Chen X, Xu Z, Zeng S, Wang X, Liu W, Qian L, et al. Sirt5 downregulation is associated with poor prognosis in glioblastoma. Cancer Biomarkers: Section Dis Markers. (2019) 24:449–59. doi: 10.3233/CBM-182197
154. Xiangyun Y, Xiaomin N, Linping G, Yunhua X, Shun L. Desuccinylation of pyruvate kinase m2 by sirt5 contributes to antioxidant response and tumor growth. Oncotarget. (2016) 8:6984–93. doi: 10.18632/oncotarget.14346
155. Clark O, Yen K, Mellinghoff IK. Molecular pathways: isocitrate dehydrogenase mutations in cancer. Clin Cancer Res. (2016) 22:1837–42. doi: 10.1158/1078-0432.CCR-13-1333
156. Fujii T, Khawaja MR, DiNardo CD, Atkins JT, Janku F. Targeting isocitrate dehydrogenase (idh) in cancer. Discovery Med. (2016) 21(117):373.
157. Kwon OK, Bang IH, Choi SY, Jeon JM, Na A, Gao Y, et al. Ldha desuccinylase sirtuin 5 as a novel cancer metastatic stimulator in aggressive prostate cancer. Genomics Proteomics Bioinf. (2023) 21:177–89. doi: 10.1016/j.gpb.2022.02.004
158. Wang C, Zhang C, Li X, Shen J, Xu Y, Shi H, et al. Cpt1a-mediated succinylation of s100a10 increases human gastric cancer invasion. J Cell Mol Med. (2019) 23:293–305. doi: 10.1111/jcmm.2019.23.issue-1
159. Yang X, Wang Z, Li X, Liu B, Liu M, Liu L, et al. Shmt2 desuccinylation by sirt5 drives cancer cell proliferation. Cancer Res. (2018) 78:372–86. doi: 10.1158/0008-5472.CAN-17-1912
160. Abril YLN, Fernandez IR, Hong JY, Chiang Y, Kutateladze DA, Zhao Q, et al. Pharmacological and genetic perturbation establish sirt5 as a promising target in breast cancer. Oncogene. (2021) 40:1644–58. doi: 10.1038/s41388-020-01637-w
161. Ma Y, Qi Y, Wang L, Zheng Z, Zhang Y, Zheng J. Sirt5-mediated sdha desuccinylation promotes clear cell renal cell carcinoma tumorigenesis. Free Radical Biol Med. (2019) 134:458–67. doi: 10.1016/j.freeradbiomed.2019.01.030
162. Zhou B, Yang Y, Pang X, Shi J, Jiang T, Zheng X. Quercetin inhibits dna damage responses to induce apoptosis via sirt5/pi3k/akt pathway in non-small cell lung cancer. BioMed Pharmacother. (2023) 165:115071. doi: 10.1016/j.biopha.2023.115071
163. Zhang J, Huang X, Zhang T, Gu C, Zuo W, Fu L, et al. Antitumorigenic potential of lactobacillus-derived extracellular vesicles: p53 succinylation and glycolytic reprogramming in intestinal epithelial cells via sirt5 modulation. Cell Biol Toxicol. (2024) 40:66. doi: 10.1007/s10565-024-09897-y
164. Bai L, Yang ZX, Ma PF, Liu JS, Wang DS, Yu HC. Overexpression of slc25a51 promotes hepatocellular carcinoma progression by driving aerobic glycolysis through activation of sirt5. Free Radic Biol Med. (2022) 182:11–22. doi: 10.1016/j.freeradbiomed.2022.02.014
165. Tang Z, Li L, Tang Y, Xie D, Wu K, Wei W, et al. Cdk2 positively regulates aerobic glycolysis by suppressing sirt5 in gastric cancer. Cancer Sci. (2018) 109:2590–98. doi: 10.1111/cas.2018.109.issue-8
166. Deng J, Liu ZM, Zhu KR, Cui GL, Liu LX, Yan YH, et al. New epsilon-n-thioglutaryl-lysine derivatives as sirt5 inhibitors: chemical synthesis, kinetic and crystallographic studies. Bioorg Chem. (2023) 135:106487. doi: 10.1016/j.bioorg.2023.106487
167. Jiang Y, Zheng W. Cyclic tripeptide-based potent and selective human sirt5 inhibitors. Med Chem. (2020) 16:358–67. doi: 10.2174/1573406415666190603101937
168. Hu T, Shukla SK, Vernucci E, He C, Wang D, King RJ, et al. Metabolic rewiring by loss of sirt5 promotes kras-induced pancreatic cancer progression. Gastroenterol (New York N.Y 1943). (2021) 161(5):1584–600. doi: 10.1053/j.gastro.2021.06.045
169. Barreca F, Aventaggiato M, Vitiello L, Sansone L, Russo MA, Mai A, et al. Sirt5 activation and inorganic phosphate binding reduce cancer cell vitality by modulating autophagy/mitophagy and ros. Antioxidants (Basel). (2023) 12:1635. doi: 10.3390/antiox12081635
170. Shen H, Ma W, Hu Y, Liu Y, Song Y, Fu L, et al. Mitochondrial sirtuins in cancer: a revisited review from molecular mechanisms to therapeutic strategies. Theranostics. (2024) 14:2993–3013. doi: 10.7150/thno.97320
Keywords: SIRT5, cancer, succinylation, desuccinylase, sirtuin
Citation: Ke Z, Shen K, Wang L, Xu H, Pan X, Qian Z, Wen Y, Lv T, Zhang X and Song Y (2025) Emerging roles of mitochondrial sirtuin SIRT5 in succinylation modification and cancer development. Front. Immunol. 16:1531246. doi: 10.3389/fimmu.2025.1531246
Received: 20 November 2024; Accepted: 13 January 2025;
Published: 29 January 2025.
Edited by:
Lei Huang, University of Massachusetts Medical School, United StatesReviewed by:
Yuliang Tang, Cornell University, United StatesCopyright © 2025 Ke, Shen, Wang, Xu, Pan, Qian, Wen, Lv, Zhang and Song. This is an open-access article distributed under the terms of the Creative Commons Attribution License (CC BY). The use, distribution or reproduction in other forums is permitted, provided the original author(s) and the copyright owner(s) are credited and that the original publication in this journal is cited, in accordance with accepted academic practice. No use, distribution or reproduction is permitted which does not comply with these terms.
*Correspondence: Xiuwei Zhang, emhhbmd4aXV3ZWl5d3lAMTI2LmNvbQ==; Yong Song, eW9uZ19zb25nNjMxMEB5YWhvby5jb20=
†These authors have contributed equally to this work
Disclaimer: All claims expressed in this article are solely those of the authors and do not necessarily represent those of their affiliated organizations, or those of the publisher, the editors and the reviewers. Any product that may be evaluated in this article or claim that may be made by its manufacturer is not guaranteed or endorsed by the publisher.
Research integrity at Frontiers
Learn more about the work of our research integrity team to safeguard the quality of each article we publish.