- 1Molecular Immunology, Faculty of Health Sciences, Brandenburg University of Technology (BTU) Cottbus-Senftenberg, Senftenberg, Germany
- 2Faculty of Environment and Natural Sciences, Brandenburg University of Technology (BTU) Cottbus-Senftenberg, Senftenberg, Germany
Aging is associated with immunosenescence, a decline in immune functions, but also with inflammaging, a chronic, low-grade inflammation, contributing to immunosenescence. Monocytes and macrophages belong to the innate immune system and aging has a profound impact on these cells, leading to functional changes and most importantly, to the secretion of pro-inflammatory cytokines and thereby contributing to inflammaging. Rheumatoid arthritis (RA) is an autoimmune disease and age is an important risk factor for developing RA. RA is associated with the early development of age-related co-morbidities like cardiovascular manifestations and osteoporosis. The immune system of RA patients shows signs of premature aging like age-inappropriate increased production of myeloid cells, accelerated telomeric erosion, and the uncontrolled production of pro-inflammatory cytokines. In this review we discuss the influence of aging on monocytes and macrophages during healthy aging and premature aging in rheumatoid arthritis.
Introduction
The immune system is the body’s defense against diseases and infection. As individuals age, the immune system undergoes changes that gradually impair its ability to defend against diseases, infections and other challenges; a process called immunosenescence.
Cellular senescence, stem cell exhaustion, genomic instability, telomere attrition, epigenetic alterations, loss of proteostasis, altered cellular communication, dysregulated nutrient-sensing, and mitochondrial dysfunction have been described as hallmarks of aging (1). Senescent cells secrete a variety of mediators (growth factors, proteases, chemokines, cytokines), termed the senescence-associated secretory phenotype (SASP), that affect surrounding cells and tissues (2).
In addition to immunosenescence, aging is accompanied by a chronic, low-grade inflammation. This process is called inflammaging (3). Both the innate and the adaptive immune system are altered by aging. While the innate immune system becomes more active during aging, the adaptive immune system tends to become more inactive. This leads to a decreased ability to resolve infections and establish immunological memory, as well as an increased risk of age-related diseases (3, 4).
Rheumatoid arthritis (RA) is an autoimmune disease characterized by systemic chronic inflammation, chronic infiltration of immune cells in the synovial membrane, and joint destruction. Age is considered an important risk factor for RA (5, 6) and the immune system is prematurely aged (7), making it an important model to study the molecular mechanisms of an aging immune system. RA patients have increased systemic levels of pro-inflammatory cytokines such as IL-6 and TNFα (5). Monocytes and macrophages are the main producers of TNFα and IL-6, and these cytokines are targets of the most successful therapies in RA, demonstrating that monocytes and macrophages are important players in the pathogenesis of RA. Circulating monocytes are recruited into the RA synovium via chemotaxis and this recruitment is an important factor for causing synovial inflammation (8). Monocytes also express increased cellular surface antigens, chemokine receptors, produce inflammatory cytokines and promote cartilage and bone destruction in RA (9). These immune responses contribute to RA pathogenesis. Thus, understanding the role of monocytes and how they are influenced by aging in RA can provide insight into potential therapeutic targets to modulate monocyte function or block inflammatory signals.
In this review we summarize the findings on the effects of healthy aging and premature aging in RA on the phenotype and function of monocytes and macrophages. We will focus thereby on monocytopoiesis and monocyte subpopulations, telomere length and epigenetic changes, functional consequences like cytokine production, phagocytosis, and respiratory burst, and finally on monocyte metabolism.
Monocytopoiesis
Monocytes develop from hematopoietic stem cells (HSCs). These multipotent stem cells are located in the bone marrow and have the potential to differentiate into all blood cell types. In the myeloid lineage, HSCs then differentiate into common myeloid progenitors (CMPs), granulocyte-monocyte progenitors (GMPs) and ultimately culminate in the development of granulocytes and monocytes (10). Aging leads to changes in the differentiation of HSCs into the various blood cells (11), which results in the increased production of myeloid cells, in a process called “myeloid skewing” (12–15).
Aged healthy adults have an increased monocyte count in the peripheral blood. Reitsema et al. reported a monocyte count of 0.56 x 109/L in healthy older individuals in comparison to 0.41 x 109/L in young individuals (16). Similar results were reported by others (17–21). Seidler et al. observed no difference in the monocyte count (22). The discrepancies seen might depend on the different recruitment strategies of old healthy individuals and the different age spans in the cohorts. The time point of the phlebotomy might also play role in the discrepancies between the studies, especially the metabolic state and physical activity. Snodgrass et al. showed a decreased fasting monocyte count in older individuals that increased after consuming a high-fat meal (23). In addition, exercise is known to mobilize monocytes, however, the effect on the monocyte count is mostly short-term (24). In healthy mice, an increased monocyte count was observed in the bone marrow, the spleen, and the peripheral blood of old mice (15, 19, 25, 26). Aging in rats also leads to monocytosis in the peripheral blood (27).
Bone marrow progenitor cells of RA patients show an accelerated differentiation into CD14+ cells compared to control cells (28). In line with this study, Smijanovic et al. showed that the transcriptomes of bone marrow monocytes of RA patients indicate an accelerated monocytopoiesis (29). This increased production of monocytes in the bone marrow leads to an increased monocyte count in the peripheral blood and increased monocyte frequencies in the mononuclear peripheral blood cells (30–34). Klimek et al. showed that the increase in absolute monocyte numbers is more pronounced in RA patients with a high disease activity (33), and Coulthard et al. observed increased monocyte numbers in both early (<12 months disease duration) and late RA (>12 months disease duration) (34). Murine arthritis models show increased myeloid precursors in the bone marrow and peripheral blood, and increased monocyte numbers in the spleen and peripheral blood (35, 36).
In addition to bone marrow hematopoiesis, extramedullary hematopoiesis in the spleen has been reported in adults (37). Hematopoietic stem and progenitor cells (HSPCs) are able to migrate from the bone marrow via the peripheral blood into the spleen and increase myelopoiesis during infectious or inflammatory conditions (38, 39). The so-called “emergency hematopoiesis” plays a role in acute and chronic sterile inflammation (38, 40, 41). Not much is known about extramedullary hematopoiesis in the spleen during aging. Loukov et al. showed that extramedullary hematopoiesis is increased in aged mice, resulting in increased monocyte numbers (42). They also found that splenic monopoiesis is driven by TNF, suggesting that the increased extramedullary monopoiesis is caused by the chronic, low-level inflammation that occurs with age. Extramedullary hematopoiesis in the spleen has also been reported in murine arthritis models (35, 43, 44), however, studies in humans are missing.
It is known that inflammatory conditions lead to an enhanced production and release of myeloid cells from the bone marrow (45). So it’s not surprising that in both RA and aging an increased monocytopoiesis and increased numbers of monocytes in the peripheral blood are observed. TNFα and IL-1β have a profound influence on the bone marrow, already in low-grade inflammation (46, 47). Neutralization of TNFα by therapeutic antibodies led to a reduction of monocyte numbers in the peripheral blood of RA patients (48, 49), further demonstrating the role of TNFα in bone marrow mobilization.
Monocyte subpopulations
Circulating human blood monocytes are not a homogenous cell population but can be divided into classical monocytes (CM, CD14++/CD16-), intermediate monocytes (IM, CD14++/CD16+), and non-classical monocytes (NCM, CD14+/CD16++), where CMs represent approximately 90%, IMs 5%, and NCMs 5% of peripheral blood monocytes (50). In mice, CMs are Ly-6C++/CD43+, IMs Ly-6C++/CD43++, and NCMs Ly-6C+/CD43++ (50).
Classical monocytes are released from the bone marrow and circulate in the bloodstream for approximately 24 hours (51). A similar lifespan was reported for Ly-6C++ monocytes in mice (52). CMs then differentiate into intermediate monocytes, however, most of the CMs leave the circulation or die. The CMs leave the circulation to enter various organs to replenish the monocyte-derived macrophage pool or migrate to sites of inflammation (53–55). Intermediate monocytes then circulate for approximately four days and all differentiate into non-classical monocytes. The NCMs circulate for approximately seven days, the Ly-6C+ monocytes in mice for two days (51, 52). They are known to patrol the endothelium (56, 57). All three monocyte subpopulations are able to produce pro- and anti-inflammatory cytokines. However, there are discrepancies in the literature in regard to comparisons between the subpopulations, most likely explained by different isolation strategies (57–61).
In humans, there seems to be an age-dependent dynamic in the prevalence of the three subpopulations as early as birth. However, it is important to note that monocytes in neonates originate from both fetal and adult HSCs (62), and this might influence monocyte subpopulation frequencies. Hegge et al. compared the percentage of the three subpopulations on total monocytes between healthy term neonates (mean gestational age 39 weeks) to young adults (mean age 24 years), and found a decrease of CMs and an increase of IMs in the neonates (63). Wisgrill et al. compared cord blood from premature infants (mean gestational age 29 weeks), term newborns (mean gestational age 39 weeks), and peripheral blood from healthy adults (mean age 25 years) (64). The absolute count of CMs was found to be increased in term neonates compared to preterm neonates. IMs were decreased in term neonates in comparison to preterm neonates and also in adults in comparison to both term and preterm neonates. In contrast, Sohlberg et al. and Murphy et al. observed no difference between cord blood from term newborns and blood of healthy adults, however, both did not differentiate between three subpopulations but only between CMs and IMs combined and NCMs (65, 66).
Most of the studies focus on monocytes in the peripheral blood of adults after the neonatal-to-adult hematopoiesis transition. Several studies showed that the NCM subpopulation and the IM subpopulation expand with age in adults, both in frequency and in absolute numbers (17, 67–69). In addition, several other studies reported an expansion of the NCM subset. Seidler et al. observed both an increase in NCM frequency as well as an increase in absolute numbers of NCMs in old individuals in comparison to young individuals (22). Wang et al. found an increase in NCM and a decrease in CM frequencies in old individuals compared to young individuals (70). They also observed a positive correlation of the IM frequency with age in old individuals, however, there was no significant difference between the two age groups when compared directly. Ong et al. found the absolute number of all three subpopulations increased with age (61). Mohanty et al. analyzed IMs and NCMs together, and the frequency of this combined subset was increased in older individuals, whereas CMs decreased (71).
In addition to an increase in absolute monocyte numbers, RA patients also have an expansion of the intermediate monocyte subset. Early studies reported an increase in the frequency and absolute number of CD16+ monocytes in RA patients, however, the monocytes were only classified into CMs and CD16+ monocytes (IMs and NCMs together) (72, 73). Cooper et al. also did not use the CM, IM, and NCM classification, but compared NCMs and CD14++ monocytes (CMs and IMs together) between healthy donors and RA patients (74). They observed no difference in the NCM frequency, but an increased CD16 expression in long-standing RA vs. healthy donors in the CD14++ population, most likely representing IMs. Using the CM, IM, and NCM classification, we and other groups observed an expansion of the IM subset in RA (33, 34, 58, 75–79). IMs are also the predominant monocyte subpopulation in the synovial fluid of RA patients (29, 76). While the expansion of IMs in RA is well documented, the data on NCMs are less clear. Several studies observed no difference (58, 74, 76, 79), some reported a decrease (29, 33, 80), and others an increase (34, 75). Overall, all the studies did not observe an age correlation, while in healthy adults it was shown that the NCM and IM subset expands with age, as described earlier. This suggests that there might be a premature shift in the RA patient subpopulations, at least with the expansion of the IM subset. On the other hand, most RA patient cohorts have a mean age of approximately 50 years, and although some cohorts also include younger patients, more cohorts with young RA patients are needed to analyze the influence of age on monocyte subpopulations. Disease duration seems to play no role in the expansion of monocyte subsets as the changes are already present in early RA (34, 58).
There is one other noteworthy monocyte subpopulation that is part of the classical monocyte subset, namely CD56+ monocytes (81). CD56+ monocytes were first described by Sconocchia et al. in 2005 (82). We found that the frequency of CD56+ monocytes strongly increases with age (83). Young RA patients (20-39 years old) have an increased frequency of CD56+ monocytes compared to age-matched healthy adults (83), suggesting that the monocytes of RA patients are prematurely aged. The frequency of CD56+ monocytes declined during therapeutic TNFα blockade and the decrease was also associated with a better response to the treatment. CD56+ monocytes are also expanded in obesity and SARS-CoV-2, both diseases associated with immunosenescence (81, 84, 85). Dutt et al. also showed a correlation of CD56+ monocyte frequency and age in SARS-CoV-2 patients (84).
Telomere length
Aging is associated with telomere shortening, and Hearps et al. showed that classical monocytes and CD16+ monocytes from older individuals had shorter telomeres than monocytes from young individuals (67). Spyridopoulos et al. observed an age-dependent telomere attrition of 30 base pairs per year in monocytes (86). Hochstrasser et al. also reported a strong negative correlation between age and telomere length (87). Bone marrow-derived macrophages from old mice had shorter telomeres than from young mice (88).
Circulating monocytes do not show signs of proliferation and active cell cycle (51). This suggests that telomere shortening occurs before the monocytes enter the circulation and is already present in the myeloid precursors. Spyridopoulos et al. showed that there was a strong correlation of telomere length in monocytes, granulocytes, and CD34+ peripheral blood progenitor cells (86). Telomere length is maintained by telomerase, a reverse transcriptase able to lengthen telomeres. When telomerase components were deleted, bone marrow-derived macrophages had shorter telomeres and an inflammatory phenotype with increased oxidative stress and hyperactivation of the NLRP3 inflammasome (89).
Telomere length is associated with the risk of RA (90, 91), but most data are from T cells (92). So far, we can only speculate on telomere length in RA monocytes and macrophages. Li et al. showed that the expression of MRE11A is decreased in monocytes of RA patients (93). MRE11A is a double-strand-break repair nuclease and a decreased expression leads to telomere damage in CD4+ T cells in RA. It is also known that circulating CD34+ HSCs from RA patients have a decreased telomere length but an increased telomerase activity (92, 94). The regulation of telomerase seems to be cell-type specific in the hematopoietic system in RA and independent of disease activity, at least in naïve CD4 T cells (92). In aging, it has been shown that an increased inflammatory load is associated with increased odds for a short leukocyte telomere length (95) and inflammation can cause telomere attrition (96), but it is not clear if cytokines are the cause or the result of shortened telomere length and vice versa. It has been shown that persistent DNA damage in senescent cells initiated the secretion of inflammatory cytokines like IL-6 (97). However, more research focusing on telomere length in monocytes and macrophages in aging and RA could provide further insight into the interplay between inflammation and telomere length as the cells are one of the major producers of these cytokines.
Epigenetic changes
Epigenetics is the structural adaptation of chromosomes that do not alter underlying DNA sequence but influence genetic function (98). Common types of epigenetic changes include DNA methylation and histone modification and can have long term effects on an individual’s health. DNA methylation patterns play a part in the determination of immune cell fate and immune responses (99). Aging leads to changes in the DNA methylation patterns, and sites with hyper-methylation and hypo-methylation have been described (100–103).
Reynolds et al. identified genes that showed an age-associated expression in monocytes, and in some of those genes the CpG methylation state mediated the association (102, 104). The described age-related methylation sites are located in enhancers linked to the expression of antigen processing and presentation genes and tend to be hypomethylated, suggesting a change in antigen presentation during aging. Saare et al. detected several CpG sites with differential methylation between age groups in monocytes (105), and they also found hypermethylation of CpG sites at the ELOVL2 and FHL2 gene loci. These have been previously reported to be associated with age (100). Other groups also observed age-related changes in DNA methylation in monocytes (106–109).
There have been several monocyte methylome alterations described in RA. Rodríguez-Ubreva et al. reported that the change in the methylome correlated with the disease activity score DAS28, and that the methylome of RA patients in remission resembled the methylome of healthy donors (110). They also showed that pro-inflammatory cytokines can induce the methylation pattern linked to the high DAS28 score. Mok et al. showed the hypomethylation of CYP2E1 and DUSP22 promoters in monocytes of active RA patients with erosive disease (111). Other groups also described a distinct DNA methylation pattern in monocytes of RA patients (112, 113).
The above studies show that in recent years epigenetic changes in monocytes have emerged as features of aging and RA. Currently there is insufficient data available on its functional consequences, interaction of various epigenetic modifications and their exact role in RA and aging. More insight could lead to a better predicament of therapeutic responses and opens the possibility of personalized anti-TNF treatment as demonstrated recently in a study on the PBMC methylome in RA patients using machine learning models (114). More research is also needed on the role of aging in the epigenetic modifications in monocytes observed in RA.
Cytokines
Aging is associated with elevated concentrations of pro-inflammatory cytokines, like TNFα, IL-6, and IL-1β, in the serum (70, 115–117). There is a plethora of studies focusing on the cytokine response of monocytes and macrophages during aging and we will focus on one hand on the spontaneous cytokine secretion of resting monocytes and macrophages, and on the other hand on the cytokine response to bacterial challenges. Finally, we will discuss RA monocytes and macrophages.
Spontaneous cytokine secretion of monocytes and macrophages
Álvarez-Rodríguez et al. reported that age is positively correlated with the intracellular expression of TNFα, IL-6, and IL-1β in circulating monocytes (117), whereas O’Mahony et al. found no difference (118). Cao et al. and Puchta et al. also did not observe a difference in the TNFα, IL-6, and IL-1β expression of monocytes in young and old individuals (17, 119). Hearps et al. however, reported an increased TNFα expression in classical, intermediate, and non-classical monocytes of older individuals (67). Pence et al. showed a decreased IL-6 gene expression in older individuals (120), corroborating the findings of Mohanty et al. who observed a decrease in IL-6+ monocytes (71). In mice, unstimulated splenic macrophages, thioglycollate-elicited peritoneal macrophages, or bone marrow-derived macrophages from young and old mice did not differ in their spontaneous TNFα, IL-6, and IL-1β secretion (121–125).
The results on pro-inflammatory cytokine secretion or expression in resting monocytes and macrophages are inconclusive. Different analysis methods (intracellular cytokine staining, ELISA of cytokines in the supernatant) and detection limits of the expected low, spontaneous monocyte cytokine secretion might play a role.
Induced cytokine secretion of monocytes and macrophages
Cao et al. reported a higher frequency of TNFα+ monocytes, a decreased frequency of IL-6+ monocytes, and an unchanged frequency of IL-1β+ monocytes in response to stimulation with bacterial lipopolysaccharide (LPS) in older individuals (17). Puchta et al. analyzed the TNFα and IL-6 response of classical, intermediate, and non-classical monocytes of old and young individuals. They found an increased TNFα and IL-6 expression in intermediate monocytes of older individuals in response to LPS and no difference in the other two subpopulations (119). In addition, the group analyzed the LPS-induced TNFα and IL-6 secretion of isolated monocytes and observed an increased response from older individuals. An increased TNFα expression was also observed by Hearps et al. in intermediate monocytes and non-classical monocytes (67). Wang et al. analyzed the intracellular expression of IL-6 and found an increased LPS-induced IL-6 expression in all three monocyte subpopulations from aged donors (60-70 years) vs. young donors (21–30) (70). However, Pence et al. showed a decreased IL-6 gene expression in response to LPS in older individuals (120). We analyzed CD56+ monocytes, a monocyte subset that expands with age, and found that they express more TNFα in response to LPS than CD56- monocytes (83).
The findings on an increased TLR4 cytokine response is not limited to monocytes. Bouchlaka et al. differentiated peripheral blood mononuclear cells (PBMCs) into macrophages and stimulated them with LPS. They found an increased TNFα and IL-6 secretion in response to LPS in macrophages differentiated from older individuals compared to young individuals (123). Gather et al. differentiated monocytes from young and old individuals towards M1 and found no difference in TNFα and IL-6 mRNA and TNFα protein expression (126).
Whereas there is a trend towards a stronger pro-inflammatory cytokine response in TLR4 activation in monocytes and also macrophages, the response to other TLR ligands seems to be attenuated. Van Duin et al. showed that the frequency of TNFα+ and IL-6+ monocytes is diminished in older individuals when the monocytes were activated with Pam3CSK4, a TLR1/2 heterodimer ligand (127). However, they observed no difference when the monocytes were activated with lipoteichoic acid (TLR2/6 heterodimer ligand), LPS (TLR4 ligand), or flagellin (TLR5 ligand). Nyugen et al. activated whole blood with Pam3CSK4 and analyzed the frequency of TNFα+ and IL-6+ monocytes (68). IL-6+ monocytes were decreased in all three monocyte subsets, TNFα+ monocytes were decreased in intermediate and non-classical monocytes in older individuals.
LPS-stimulated thioglycollate (TG)-elicited peritoneal macrophages from old mice produced less TNFα and IL-6 than those from young mice (121). The same group also showed that activation of splenic macrophages from old mice with LPS or zymosan led to a diminished TNFα and IL-6 production (122, 128). Fallah et al. made similar observations. They reported that splenic macrophages produced less TNFα in response to LPS or killed S. pneumoniae (129). Gomez et al. and Chelvarajan et al. also described a decreased LPS-induced TNFα, IL-6, and IL-1β secretion in splenic macrophages from old mice (130, 131). Beharka et al. observed no difference in the LPS-induced IL-6 secretion of peritoneal macrophages between young and old mice (125). Chen and Bradley found a decreased TNFα and IL-6 secretion in resident and complete Freund’s adjuvant-elicited peritoneal macrophages from old mice, but an increased TNFα and IL-6 secretion in TG-elicited peritoneal macrophages (132). Cecílio et al. showed that resident and TG-elicited peritoneal macrophages from old mice have a decreased TNFα response (133).
The overall findings on decreased cytokine secretion are not limited to TLR4 ligands but also found with other TLR ligands. Alveolar macrophages from mature mice (10-12 months) and old mice (19-21 months) had a decreased TNFα response to ethanol-killed pneumococci and purified pneumococcal cell wall (134), and purified CD11b+ peritoneal macrophages a decreased TNFα response to zymosan (135).
The data on murine bone marrow-derived macrophages (BMDM) from old mice are less clear. Several studies reported an increased TNFα and IL-6 secretion in response to LPS (123, 133, 136, 137) Kang et al. reported an increased production of IL-1β in BMDMs from old mice in response to activation with Staphylococcus aureus (89). Zhao et al., however, found a decreased LPS-induced IL-6 secretion (138), whereas Mahbub et al. observed no difference in the TNFα, IL-6, and IL-1β secretion between old and young mice (128). Ramirez et al. also did not find an age effect on the LPS-induced IL-1β secretion, however, they observed a decreased response to an inflammasome activation with LPS and ATP (139). BMDMs activated with Porphyromonas gingivalis from older mice also showed a decreased TNFα response (140).
Published data on the anti-inflammatory cytokine IL-10 and monocytes or macrophages are scarce. Cao et al. did not observe a difference in the IL-10 expression of monocytes in young and old individuals (17). They also reported an unchanged frequency of IL-10+ monocytes in response to stimulation with LPS in older individuals. Mohanty et al. found an increased frequency of IL-10+ monocytes in older individuals (71). Boehmer et al. showed that splenic macrophages of old mice produce more IL-10 than young mice in response to LPS as well as zymosan (122), whereas Cecílio et al. observed no effect on IL-10 in LPS-activated murine BMDM (133).
While an increased TLR4-induced pro-inflammatory cytokine response was most often observed in monocytes, the cytokine response was diminished in tissue macrophages. The age effect on bone marrow-derived macrophages is also less clear than on tissue macrophages from mice. Mahbub et al. already speculated that the surrounding aging microenvironment has an effect on macrophage development (128). Gomez et al. reported similar findings (141). Inflammaging and inflammatory cytokines and chemokines secreted by senescent cells (the senescence-associated secretory phenotype, SASP) have a profound influence on surrounding cells. Gather et al. showed that age has no effect on monocyte-derived macrophages, but that an aged microenvironment in co-culture experiments with aged dermal fibroblasts drives a more pro-inflammatory macrophage phenotype (126). The aged microenvironment also drives the accumulation and activation of M1-like CD38+ macrophages in adipose tissue and liver during aging (142, 143). McQuattie-Pimentel et al. also showed that age-related changes in alveolar macrophages are defined by their microenvironment in the lung (144). Chambers et al. described the recruitment of inflammatory monocytes by senescent fibroblasts in the human skin (145).
Cytokine secretion of monocytes and macrophages in RA
Pro-inflammatory cytokines play an important role in the pathogenesis of RA, as demonstrated by the successful therapies using TNFα and IL-6 neutralizing agents (146). We have shown that monocytes of RA patients show signs of premature aging. They express membrane TNFα and spontaneously secrete IL-1β, which leads to an increased survival of the monocytes (147). Paoletti et al. also found an increased expression of membrane TNFα on RA monocytes (148). It has also been demonstrated that the intermediate monocyte subpopulation is predominantly expanded in the synovial fluid of RA patients (29, 76) and this subpopulation is associated with aging as discussed above and characterized by a high IL-1β secretion (58, 149). Single-cell RNA sequencing of RA synovial tissue led to the identification of a IL1B+ pro-inflammatory monocyte subset which was possibly shaped by the local microenvironment (150). Transcriptomic analysis of classical monocytes revealed that the expression of IL6 was higher in erosive disease than in non-erosive disease in premenopausal women (median age 39 vs. 37 years) (151).
We and others have shown that monocytes of treated RA patients and healthy controls have a comparable cytokine response to LPS (147, 152, 153). However, Leirisalo-Repo et al. observed an increased TNFα secretion in LPS-activated monocytes of untreated patients with early RA (152). This is in contrast to a study by Liou (154). They reported a normal cytokine response to LPS in monocytes from untreated RA patients. We also found no difference in the IL-1β secretion in treated and untreated RA patients following monocyte activation with LPS, however, we observed a lower IL-18 response in monocytes of untreated RA patients compared to healthy controls (153). The inflammasome activation with LPS and calcium led to an increased IL-1β and IL-18 secretion in monocytes from RA patients compared to healthy controls (153).
Phagocytosis
Aging is associated with an increased susceptibility to infections. Phagocytosis of pathogens and apoptotic cells is one of the key functions of monocytes and macrophages and plays a crucial role in immune defense.
Human monocytes and macrophages
Published data on the effect of aging on human monocyte phagocytosis are conflicting. Hearps et al. report an impaired phagocytosis of Escherichia coli by human monocytes from old adults (median age 72) compared to monocytes from young adults (median age 28) (67) whereas several older publications describe no effect or a non-significant trend towards impaired phagocytosis in old individuals (155, 156). Mege et al. studied the phagocytosis of opsonized zymosan, immunoglobulin-coated sheep red cells and glutaraldehyde-treated sheep red cells (155). They observed an impaired phagocytosis of opsonized zymosan and immunoglobulin-coated sheep red cells by human monocytes from old adults (mean age 76) compared to monocytes from young adults (mean age 36) but this trend did not reach statistical significance (155). Gardner et al. found no difference in the phagocytosis and killing of Candida albicans in monocytes from healthy young controls (<35 years) and hospitalized patients >60 years and <35 years of age (156). Unrelated to an infectious setting, Bliederhaeuser et al. report an impaired phagocytosis of exosome-associated and free alpha-synuclein oligomers by human monocytes from old adults (median age 66) compared to monocytes from young adults (median age 24) (157). A recent study showed that monocytes and monocyte-derived macrophages from old adults (mean age 60.5) have an impaired phagocytosis of opsonized beads or Staphylococcus aureus in comparison to young individuals (mean age 23.7) (158).
Murine monocytes and macrophages
Studies of aged monocytes and macrophages in mice and rats are as conflicting as in humans. The phagocytosis of latex beads by peritoneal macrophages was decreased in aged mice (85 weeks) compared to young mice (15 weeks) (159), whereas the phagocytosis of Klebsiella pneumoniae was increased in alveolar macrophages from old rats (18 month) compared to young rats (6 month) (160). The phagocytosis of apoptotic cells by thioglycolate-elicited peritoneal macrophages, resident peritoneal macrophages and bone marrow-derived macrophages was found to be decreased in aged mice (161–163).
Linehan et al. showed that the tissue microenvironment might play a role in age-related defects in phagocytosis (164). They reported a decreased phagocytosis of fluorescent particles by peritoneal macrophages from old mice (15-20 month) compared to young mice (8-12 weeks), whereas bone marrow-derived macrophages and bone marrow monocytes showed no age-related impairment of phagocytosis (164). Interestingly, peritoneal macrophages derived from young mice showed impaired phagocytosis when injected into the peritoneum of old mice (164). This suggests that microenvironmental factors in the aged peritoneum cause age-related defects and Linehan et al. identified IL-10 as one of the factors (164).
Rheumatoid arthritis
Several older studies reported no difference in phagocytosis of bacteria between RA patients and healthy controls (165–167). Steven et al. observed increased phagocytosis of opsonized Staphylococcus aureus and Proteus mirabilis by monocytes from RA patients compared to age-matched controls (168). The phagocytosis rate correlated positively with age when patients and controls were analyzed combined, and the phagocytosis rate of RA patients remained higher than in controls in the 30-60 age group (168). More recent studies also did not focus on age. Fragoulis et al. observed no difference in the phagocytosis of secondary necrotic cell remnants in RA patients and controls (169) and Tas et al. found no difference in the phagocytosis of apoptotic cells in monocyte-derived macrophages from RA patients and controls (170).
Interestingly, Lee et al. reported that activated platelet-induced CD16 (also known as FcγRIII) on classical monocytes participates in increased antibody-dependent cellular phagocytosis (171). The monocytes then resemble intermediate monocytes, a monocyte subpopulation expanded in the blood and synovial fluid of RA patients (58, 76) and reported to be expanded in older individuals (17, 23).
Respiratory burst and reactive oxygen species
As described earlier, immune functions such as phagocytosis decline with age. Phagocytosis is accompanied by a sudden increase in the activation of oxidative metabolism known as the respiratory burst, which is responsible for killing phagocytosed pathogens, and mediated by the NADPH-oxidase (172). The enzyme catalyzes the formation of superoxide by transferring one electron to oxygen from NADPH (173). Other prominent reactive oxygen species (ROS) are hydrogen peroxide and hydroxyl radical. ROS are not only produced in response to pathogens or pathogen-associated molecular patterns by the NADPH-oxidase, ROS are also formed during mitochondrial oxidative phosphorylation and by the 5-lipoxygenase (173).
ROS and respiratory burst
Aging leads to a decrease in ROS produced during the respiratory burst in monocytes and macrophages. Alvarez et al. have shown that the production of ROS decreases with age in rat peritoneal macrophages (174, 175). Phorbol Myristate Acetate (PMA)-activated 12-month-old rat macrophages showed a lower production of superoxide ions compared to 3-month-old rats. Macrophages from 24-month-old rats had a further reduction in ROS production compared to both 12-month-old and 3-month-old rats. Activation of macrophages with N-formylmethionyl-leucylphenylalanine (fMLP) or concanavalin A did not show a decrease in ROS production between 3 and 12-month-old rats, however, a significant reduction in 24-month-old rats compared to both 3 and 12-month-old rats was observed (175).
The PMA-induced superoxide generation in human monocytes is negatively correlated with (176) and the PMA-induced capacity to produce superoxide ions is reduced in aged subjects (65-75 years old) compared to young (25-35 years old) and mature (45-55 years old) (177). Lower ROS production was also reported in alveolar macrophages. Ganguly et al. measured the production of superoxide in alveolar macrophages from female Fisher-344 rats stimulated by opsonised zymosan and found that aged rats showed lower ROS production (178). One limitation of this study was the use of aged breeder rats which have been shown to exhibit a lower magnitude of respiratory burst compared to young and aged virgins (179). Esposito et al. compared alveolar macrophages obtained from virgin adult and aged female rats and observed that normal senescent mice released larger quantities of superoxide when activated by either PMA or zymosan (180), contrary to previous findings.
ROS accumulation in aging monocytes and macrophages
In contrast to the ROS produced in activated monocytes and macrophages, the general ROS content in resting monocytes and macrophages seems to increase with age. Sebastián et al. showed an increased ROS content in BMDM from old mice compared to young mice (88). Jacinto et al. reported an increased ROS content in circulating monocytes from aged mice (181). Their study focused primarily on the apoE-/- mouse model of spontaneous atherosclerosis, but they also observed a significant increase in ROS content in monocytes from 18-month-old mice compared to 2-month-old wild-type mice.
Saare et al. reported similar findings in human monocytes (105). Oxidative stress due to ROS production was determined by measuring the fluorescence of oxidized products of chloromethyl derivative of 2’,7’-dichlorodihydrofluorescein diacetate (CM-H2DCFDA) in monocytes isolated from young (mean age 29.6 years) and old (mean age 79.4 years) donors (105). Unstimulated monocytes from older donors showed a twofold increase in ROS production, while LPS-stimulated monocytes showed a smaller but still significant increase. Higher cellular levels of ROS are associated with DNA damage (182), and Saare et al. observed that double-strand breaks were 1.5 times higher in the old cohort by analysing the percentage of monocytes showing a γH2AX signal (105).
Wang et al. also reported an increased ROS content (measured with DCFH-DA) in all three monocyte subpopulations (CM, IM, NCM) from elderly individuals (60-70 years) compared with those from young persons (21-30 years) (70). Hayashi et al. observed a correlation of monocyte ROS content (measured with dichlorofluorescein and hydroethidine) and age in combined exposed and unexposed Hiroshima atomic bomb survivors (65-95 years) (183). The monocyte ROS content also correlated with the radiation dose implying that atomic bomb radiation accelerates immunological aging. In our study on CD56+ monocytes, a monocyte subpopulation associated with aging, we found increased ROS content (measured with dihydrorhodamine 123) in CD56+ monocytes compared to CD56- monocytes (83).
ROS and rheumatoid arthritis
Monocytes in RA are under high oxidative stress compared to healthy controls as shown by Ostrakhovitch and Afanas’ev (184). Oxygen radical production of RA monocytes and healthy controls were measured by chemiluminescence with luminol or lucigenin. PMA-stimulated RA monocytes showed 2.7 times more oxygen radical production compared to controls. Monocytes from RA patients also showed an increase in NADPH oxidase activity compared to healthy controls. In addition, oxygen radical production was decreased in monocytes from RA patients in the presence of mitochondrial inhibitors rotenone and antimycin A, while normal cells were unaffected, suggesting that mitochondrial superoxide production is another source of oxygen radicals in RA monocytes.
Other studies also show that superoxide anion production is increased in monocytes from RA patients compared to healthy controls. Repo et al. showed that monocytes from untreated, early RA patients showed significantly higher Luminol-enhanced chemiluminescence to stimulation by FMLP, PMA or opsonised zymosan particles compared to healthy controls (185). Hurst et al. observed an increased superoxide anion production in RA monocytes stimulated with IgG-treated zymosan or serum-treated zymosan (186, 187). No difference in basal rates of monocyte superoxide anion production was observed between controls and RA patients. Mateen et al. showed that RA patients have an increased ROS formation compared to age-matched controls. They stained ROS with 2’, 7’-dichlorofluorescein-diacetate (DCFH-DA) to observe the intracellular ROS production (188). Nitric oxide (NO), an important component of inflammatory oxidative burst, was also shown to be 1.56 times higher in monocytes isolated from the blood of RA patients compared to healthy individuals (189).
Monocyte metabolism
The bioenergetic status of monocytes and macrophages determines cellular functions, with pro-inflammatory macrophages relying on glycolysis and the pentose phosphate pathway and anti-inflammatory macrophages relying on oxidative phosphorylation and fatty acid oxidation (FAO) (190). Monocytes and macrophages can undergo metabolic reprogramming when challenged with a stimulus and switch from an aerobic profile to anaerobic glycolysis in a process called the Warburg effect. Aging impairs oxidative phosphorylation and FAO pathways and this shift might contribute to inflammaging (191, 192).
Glycolysis and oxidative phosphorylation
Maoldomhnaigh et al. compared macrophages differentiated from monocytes from adult blood (aged 18-69 years) and cord blood (term neonates) (193). Both MDMs responded with a rapid increase in glycolysis when activated with LPS, but only the MDMs from adults showed a decrease in oxidative phosphorylation.
Wang et al. reported an increased glycolysis, impaired oxidative phosphorylation, and mitochondrial dysfunction in all three monocyte subsets in aged adults (70). These changes were most prominent in non-classical monocytes, and the authors speculated that the increased glycolysis is a result of the mitochondrial dysfunction to meet the demand for energy metabolism.
Reduced cellular respiratory capacity with age has been attributed to dysfunctional mitochondria (194). Saare et al. also reported lower mitochondrial membrane potential despite higher mitochondrial mass in aged subjects (105). The oxygen consumption rate (OCR), basal and maximal of monocytes from young (mean age 29.6 years) and old (mean age 82.3 years) individuals were measured to calculate the spare respiratory capacity (SRC) which was significantly lower in the older group. Pence and Yarbro also reported impaired mitochondrial respiratory activity in classical monocytes from old individuals using flow cytometry (120). Their monocyte isolation strategy included CD16 depletion to only focus on classical monocytes. Both studies showed that basal respiration remains largely unaffected by age but the maximal respiratory capacity is reduced in monocytes of older individuals which leads to lower SRC.
Additionally, gene expression studies in monocytes of young (average age 35.7) and old individuals (average age 71.6 years) also highlighted the downregulation of oxidative phosphorylation genes PLA2G4B and ALOX15B in older individuals, while PDK4 which inhibits pyruvate dehydrogenase complex was highly upregulated. This correlates to a shift of glucose metabolism from oxidative phosphorylation to lactate production in monocytes of older individuals (195). Interestingly, the NADPH oxidase, the enzyme involved in respiratory burst, is not affected by age (177).
However, Pence and Yarbro did not find conclusive evidence of increased glycolysis in LPS-stimulated aged monocytes ex-vivo (196). The microenvironment plays a significant role in age-related changes which could be a reason for such an observation. As the authors point out, there could be several epigenetic modifications and alterations in sirtuin activity that could be linked to metabolism.
FAO and lipid metabolism
There is an increase in circulating fatty acids in older adults since aging leads to the redistribution of body fat from subcutaneous to visceral, the latter being less efficient in the storage of fatty acids (197). Additionally, Wang et al. reported decreased FAO and increased presence of lipid droplets (LD) in aged monocytes of mice and humans (198). They also observed that PPAR-α expression was downregulated in monocytes of aged humans (>60 years old) and subsequently were able to prove that this caused the FAO decrease and LD increase by downregulating PPAR-α in young monocytes to similar effects. This also affects monocyte polarisation as the accumulation of LD in aged monocytes leads to a pro-inflammatory phenotype.
NAD+ metabolism
As an important regulator of metabolism, NAD+ is thought to also undergo changes during aging. Endogenous NAD+ is depleted during aging which eventually disrupts cellular metabolism (199). NAD+ can be obtained by several pathways, the two most commonly studied ones being de novo biosynthesis pathway, also called kynurenine pathway (KP), or the salvage pathway through nicotinamide precursors. Clement et al. showed that the NAD+ precursors remain stable with age, while NAD+ levels are reduced (200). This suggests that the decline in NAD+ might be caused by an increase in the activity of NAD-consuming enzymes. It is currently not clear which pathway is primarily responsible for providing NAD+ in macrophages as previous reports show conflicting results based on the characteristics of the macrophage studied. Liu et al. showed that de novo synthesis majorly occurs in the liver (201). However, Minhas et al. showed that decreased cellular NAD+ can also be replenished by macrophages via de novo synthesis (202). Disruption of the salvage pathway using a NAMPT inhibitor in human monocyte-derived macrophages (MDMs) demonstrated a marked increase in KP enzymes and metabolites. They also confirmed that basal de novo NAD+ synthesis accounts for 40% of total NAD+, and almost all when the salvage pathway is blocked by the NAMPT inhibitor FK866. Furthermore, they also showed that loss of the KP disrupts mitochondrial respiration by inhibiting KP enzymes IDO1 and QPRT. Peritoneal macrophages from IDO1- or QPRT-deficient mice showed reduced cellular NAD+ amounts compared to wild type controls, along with suppressed oxygen consumption and increased glycolysis. This effect was reversed on supplementation with kynurenine. Similarly, human MDMs also showed a decreased NAD+ and mitochondrial respiration when IDO1 and QPRT were inhibited. Additionally, loss of QPRT activity also elevated lactate, pentose phosphate pathway intermediates and pro-inflammatory TCA intermediates that favour IL-1β and IL-6 production. Analysis of QPRT expression in human MDMs derived from young (<35 years old) and older (>65 years old) individuals showed a significant decline in older macrophages, the older macrophages having a metabolite profile similar to that observed in LPS-stimulated macrophages. Suppression of NAD+ synthesis in aged mice indicated that the KP derived NAD+ is critical in sustaining the cellular concentration. The age-associated loss of this pathway likely contributes to the proinflammatory shift of macrophages with age.
Macrophages after inflammation, however, seem to be dependent on the salvage pathway for NAD+. Cameron et al. observed that murine BMDM showed reduced NAD+ levels when stimulated by LPS compared to resting M0 macrophages or IL-4-stimulated macrophages (203). NAMPT inhibition by FK866 treatment induced dose-dependent cell death in LPS-stimulated macrophages while the viability of M0 and IL-4 macrophages was affected to a lesser extent. Inflammatory cytokines such as TNFα, IL-1β, IFNγ induce the rate limiting enzyme for the salvage pathway, NAMPT. The authors reported that this is necessary to counter the rapid NAD+ depletion on LPS activation. As mentioned earlier, these cytokines also increase with age which likely enables the macrophages to keep up with the demand due to an increase in NAD+ consuming enzyme activities resulting from glyceraldehyde-3-phosphate dehydrogenase activity and Warburg metabolism.
Metabolic reprogramming in rheumatoid arthritis
Several studies on RA monocytes and macrophages show a pro-inflammatory metabolic profile. Metabolomic profiling showed an increased presence of lactic acid, citrate and succinate in the synovial fluid of RA (204). Shime et al. showed that lactic acid promotes pro-inflammatory pathway (205). Succinate too is known to be induced on LPS activation, as a result of the metabolic shift from oxidative phosphorylation to glycolysis during inflammation leading to its accumulation as a TCA cycle intermediate. These suggest that the macrophages in the synovial fluid might show a pro-inflammatory phenotype. Michopoulos et al. showed that itaconic acid is a marker of RA using the Tg197 mouse model which correlates with findings from Lampropoulou et al. who reported that itaconate is involved in the regulation of succinate levels in LPS-stimulated mouse bone marrow-derived macrophages (206, 207).
Yoon et al. described that Solute carrier family 7 member 5 (SLC7A5) is increased in RA patients (208). LPS-stimulated human peripheral monocytes from RA patients showed a 5.85-fold increase in SLC7A5 expression compared to healthy controls. LPS-mediated expression was also observed in human macrophages. They further reported the role of SLC7A5 in being involved in the glycolytic shift and production of pro-inflammatory cytokines such as IL-1β. McGarry et al. (30) showed that RA monocytes have increased mitochondrial respiration and enhanced glycolysis after activation with LPS. Blockade of glucose consumption resulted in an inhibition of the release of pro-inflammatory mediators. They also reported that RA monocytes have an increased number of mitochondria and an increased mitochondrial respiration already ex vivo without any stimulation. These above studies show that monocytes in RA tend to have a pro-inflammatory metabolic profile, and thus RA patients exhibit signs of premature aging.
Conclusion
Monocytes and macrophages as part of the innate immune system are markedly influenced by aging. The myeloid biased hematopoiesis leads to increased numbers of monocytes in the peripheral blood, and the monocyte subpopulations are shifted towards intermediate and non-classical monocytes during healthy aging as well as in rheumatoid arthritis. Monocyte and macrophage functions like phagocytosis and cytokine production are affected by aging. The findings on healthy aging of monocytes and macrophages are summarized in Figure 1 and aging of monocytes and macrophages in RA in Figure 2. However, further research is needed as there is still inconclusive data on the functional consequences of healthy aging and premature aging in RA on monocytes and macrophages.
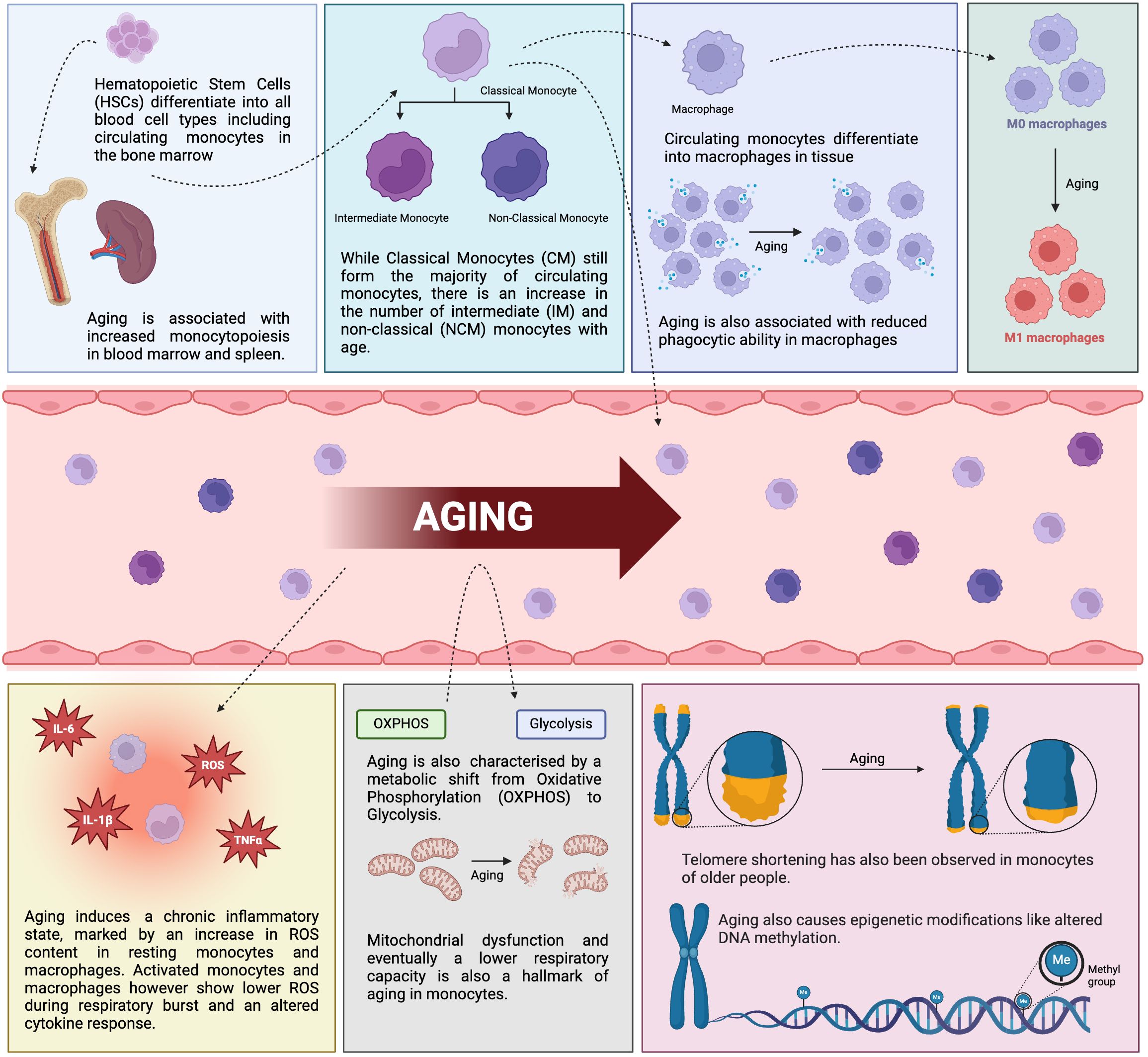
Figure 1. Impact of healthy aging on monocytes and macrophages. Schematic diagram displaying the consequence of aging on the phenotype and functions of monocytes and macrophages in healthy individuals. IL-6, interleukin-6, TNFα, tumor necrosis factor α, IL-1β, interleukin-1β, ROS, reactive oxygen species (created in BioRender.com).
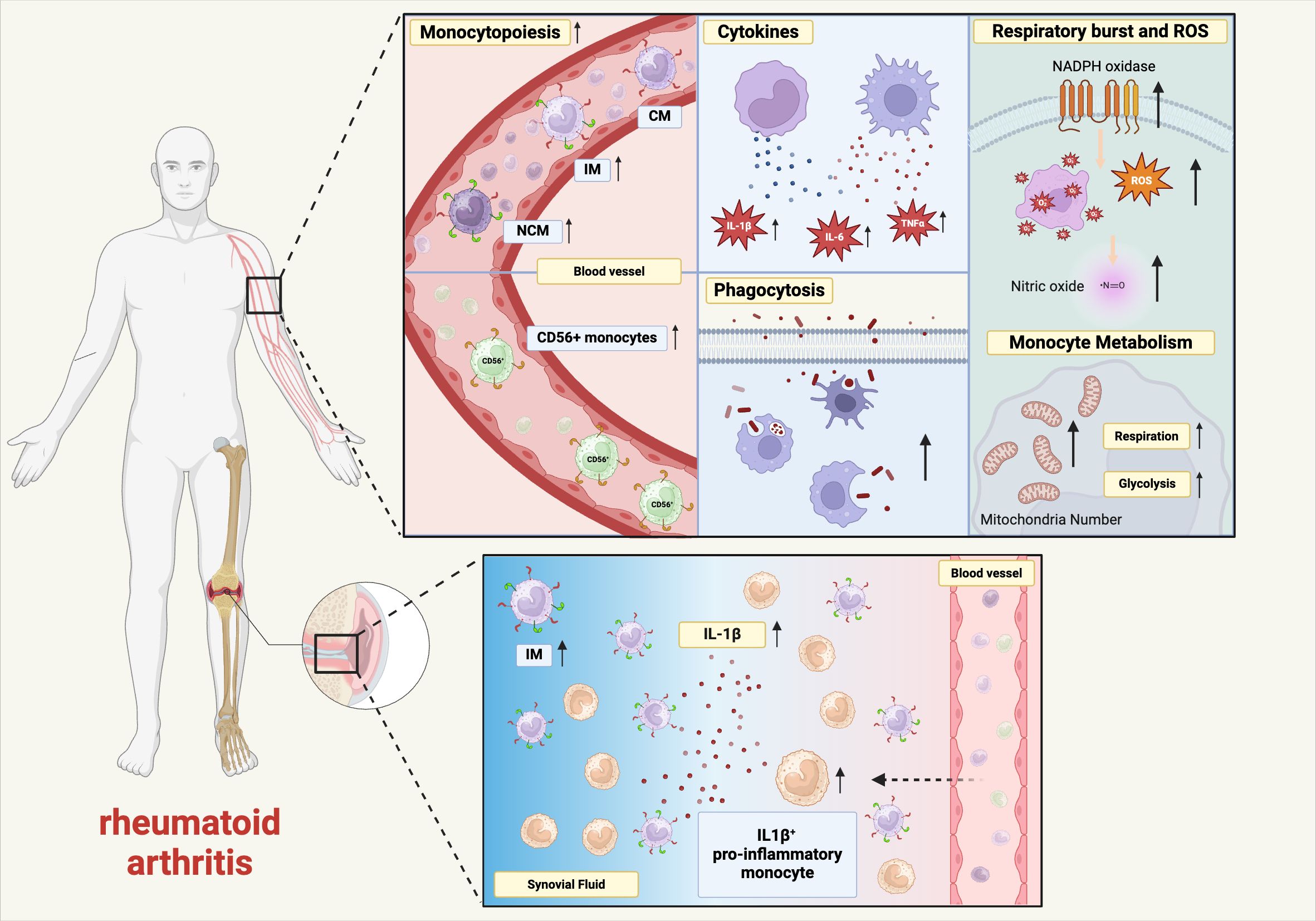
Figure 2. Premature aging in monocytes and macrophages in rheumatoid arthritis. Schematic diagram showing the effect of RA on age-related changes in monocyte and macrophage phenotypes and functions. IL-6, interleukin-6, TNFα, tumor necrosis factor α, IL-1β, interleukin-1β, ROS, reactive oxygen species, CM, classical monocytes, IM, intermediate monocytes, NCM, non-classical monocytes, NADPH, nicotinamide adenine dinucleotide phosphate (created in BioRender.com).
Author contributions
SB: Writing – original draft, Writing – review & editing. YU: Writing – original draft, Writing – review & editing. MR: Conceptualization, Writing – original draft, Writing – review & editing.
Funding
The author(s) declare that no financial support was received for the research and/or publication of this article.
Conflict of interest
The authors declare that the research was conducted in the absence of any commercial or financial relationships that could be construed as a potential conflict of interest.
Generative AI statement
The author(s) declare that no Generative AI was used in the creation of this manuscript.
Publisher’s note
All claims expressed in this article are solely those of the authors and do not necessarily represent those of their affiliated organizations, or those of the publisher, the editors and the reviewers. Any product that may be evaluated in this article, or claim that may be made by its manufacturer, is not guaranteed or endorsed by the publisher.
References
1. López-Otín C, Blasco MA, Partridge L, Serrano M, Kroemer G. The hallmarks of aging. Cell. (2013) 153:1194–217. doi: 10.1016/j.cell.2013.05.039
2. Freund A, Orjalo AV, Desprez PY, Campisi J. Inflammatory networks during cellular senescence: causes and consequences. Trends Mol Med. (2010) 16:238–46. doi: 10.1016/j.molmed.2010.03.003
3. Franceschi C, Bonafè M, Valensin S, Olivieri F, De Luca M, Ottaviani E, et al. Inflamm-aging. An evolutionary perspective on immunosenescence. Ann N Y Acad Sci. (2000) 908:244–54. doi: 10.1111/j.1749-6632.2000.tb06651.x
4. Liu Z, Liang Q, Ren Y, Guo C, Ge X, Wang L, et al. Immunosenescence: molecular mechanisms and diseases. Signal Transduct Target Ther. (2023) 8:1–16. doi: 10.1038/s41392-023-01451-2
5. Smolen JS, Aletaha D, Barton A, Burmester GR, Emery P, Firestein GS, et al. Rheumatoid arthritis. Nat Rev Dis Primer. (2018) 4:1–23. doi: 10.1038/nrdp.2018.1
6. Boots AMH, Maier AB, Stinissen P, Masson P, Lories RJ, De Keyser F. The influence of ageing on the development and management of rheumatoid arthritis. Nat Rev Rheumatol. (2013) 9:604–13. doi: 10.1038/nrrheum.2013.92
7. Weyand CM, Goronzy JJ. Aging of the immune system. Mechanisms and therapeutic targets. Ann Am Thorac Soc. (2016) 13 Suppl 5:S422–8. doi: 10.1513/AnnalsATS.201602-095AW
8. Torsteinsdóttir I, Arvidson NG, Hällgren R, Håkansson L. Monocyte activation in rheumatoid arthritis (RA): increased integrin, Fcγ and complement receptor expression and the effect of glucocorticoids. Clin Exp Immunol. (1999) 115:554–60. doi: 10.1046/j.1365-2249.1999.00817.x
9. Kinne RW, Bräuer R, Stuhlmüller B, Palombo-Kinne E, Burmester GR. Macrophages in rheumatoid arthritis. Arthritis Res Ther. (2000) 2:189. doi: 10.1186/ar86
10. Akashi K, Traver D, Miyamoto T, Weissman IL. A clonogenic common myeloid progenitor that gives rise to all myeloid lineages. Nature. (2000) 404:193–7. doi: 10.1038/35004599
11. Latchney SE, Calvi LM. The aging hematopoietic stem cell niche: Phenotypic and functional changes and mechanisms that contribute to hematopoietic aging. Semin Hematol. (2017) 54:25–32. doi: 10.1053/j.seminhematol.2016.10.001
12. Sudo K, Ema H, Morita Y, Nakauchi H. Age-associated characteristics of murine hematopoietic stem cells. J Exp Med. (2000) 192:1273–80. doi: 10.1084/jem.192.9.1273
13. Ho YH, del Toro R, Rivera-Torres J, Rak J, Korn C, García-García A, et al. Remodeling of bone marrow hematopoietic stem cell niches promotes myeloid cell expansion during premature or physiological aging. Cell Stem Cell. (2019) 25:407–18.e6. doi: 10.1016/j.stem.2019.06.007
14. Ergen AV, Boles NC, Goodell MA. Rantes/Ccl5 influences hematopoietic stem cell subtypes and causes myeloid skewing. Blood. (2012) 119:2500–9. doi: 10.1182/blood-2011-11-391730
15. Pioli PD, Casero D, Montecino-Rodriguez E, Morrison SL, Dorshkind K. Plasma cells are obligate effectors of enhanced myelopoiesis in aging bone marrow. Immunity. (2019) 51:351–66.e6. doi: 10.1016/j.immuni.2019.06.006
16. Reitsema RD, Kumawat AK, Hesselink BC, Van Baarle D, Van Sleen Y. Effects of ageing and frailty on circulating monocyte and dendritic cell subsets. NPJ Aging. (2024) 10:17. doi: 10.1038/s41514-024-00144-6
17. Cao Y, Fan Y, Li F, Hao Y, Kong Y, Chen C, et al. Phenotypic and functional alterations of monocyte subsets with aging. Immun Ageing A. (2022) 19:63. doi: 10.1186/s12979-022-00321-9
18. Waterhouse DF, Cahill RA, Sheehan F, McCreery C. Prediction of calculated future cardiovascular disease by monocyte count in an asymptomatic population. Vasc Health Risk Manage. (2008) 4:177–87. doi: 10.2147/VHRM.S2240
19. Barman PK, Shin JE, Lewis SA, Kang S, Wu D, Wang Y, et al. Production of MHCII-expressing classical monocytes increases during aging in mice and humans. Aging Cell. (2022) 21:e13701. doi: 10.1111/acel.v21.10
20. Costantini A, Viola N, Berretta A, Galeazzi R, Matacchione G, Sabbatinelli J, et al. Age-related M1/M2 phenotype changes in circulating monocytes from healthy/unhealthy individuals. Aging. (2018) 10:1268–80. doi: 10.18632/aging.101465
21. Della Bella S, Bierti L, Presicce P, Arienti R, Valenti M, Saresella M, et al. Peripheral blood dendritic cells and monocytes are differently regulated in the elderly. Clin Immunol Orlando Fla. (2007) 122:220–8. doi: 10.1016/j.clim.2006.09.012
22. Seidler S, Zimmermann HW, Bartneck M, Trautwein C, Tacke F. Age-dependent alterations of monocyte subsets and monocyte-related chemokine pathways in healthy adults. BMC Immunol. (2010) 11:30. doi: 10.1186/1471-2172-11-30
23. Snodgrass RG, Jiang X, Stephensen CB. Monocyte subsets display age-dependent alterations at fasting and undergo non-age-dependent changes following consumption of a meal. Immun Ageing. (2022) 19:41. doi: 10.1186/s12979-022-00297-6
24. Peake JM, Neubauer O, Walsh NP, Simpson RJ. Recovery of the immune system after exercise. J Appl Physiol Bethesda Md. (1985) 122:1077–87. doi: 10.1152/japplphysiol.00622.2016
25. Duong L, Pixley FJ, Nelson DJ, Jackaman C. Aging leads to increased monocytes and macrophages with altered CSF-1 receptor expression and earlier tumor-associated macrophage expansion in murine mesothelioma. Front Aging. (2022) 3:848925. doi: 10.3389/fragi.2022.848925
26. Strohacker K, Breslin WL, Carpenter KC, McFarlin BK. Aged mice have increased inflammatory monocyte concentration and altered expression of cell-surface functional receptors. J Biosci. (2012) 37:55–62. doi: 10.1007/s12038-011-9169-z
27. Martinez L, Gomez C, Vazquez-Padron RI. Age-related changes in monocytes exacerbate neointimal hyperplasia after vascular injury. Oncotarget. (2015) 6:17054–64. doi: 10.18632/oncotarget.v6i19
28. Hirohata S, Yanagida T, Itoh K, Nakamura H, Yoshino S, Tomita T, et al. Accelerated generation of CD14+ monocyte-lineage cells from the bone marrow of rheumatoid arthritis patients. Arthritis Rheumatol. (1996) 39:836–43. doi: 10.1002/art.1780390517
29. Smiljanovic B, Radzikowska A, Kuca-Warnawin E, Kurowska W, Grün JR, Stuhlmüller B, et al. Monocyte alterations in rheumatoid arthritis are dominated by preterm release from bone marrow and prominent triggering in the joint. Ann Rheum Dis. (2018) 77:300–8. doi: 10.1136/annrheumdis-2017-211649
30. McGarry T, Hanlon MM, Marzaioli V, Cunningham CC, Krishna V, Murray K, et al. Rheumatoid arthritis CD14+ monocytes display metabolic and inflammatory dysfunction, a phenotype that precedes clinical manifestation of disease. Clin Transl Immunol. (2021) 10:e1237. doi: 10.1002/cti2.v10.1
31. Buchan GS, Palmer DG, Gibbins BL. The response of human peripheral blood mononuclear phagocytes to rheumatoid arthritis. J Leukoc Biol. (1985) 37:221–30. doi: 10.1002/jlb.37.2.221
32. Hamilton JA. Rheumatoid arthritis: opposing actions of haemopoietic growth factors and slow-acting anti-rheumatic drugs. Lancet. (1993) 342:536–9. doi: 10.1016/0140-6736(93)91653-4
33. Klimek E, Mikołajczyk T, Sulicka J, Kwaśny-Krochin B, Korkosz M, Osmenda G, et al. Blood monocyte subsets and selected cardiovascular risk markers in rheumatoid arthritis of short duration in relation to disease activity. BioMed Res Int. (2014) 2014:736853. doi: 10.1155/2014/736853
34. Coulthard LR, Geiler J, Mathews RJ, Church LD, Dickie LJ, Cooper DL, et al. Differential effects of infliximab on absolute circulating blood leucocyte counts of innate immune cells in early and late rheumatoid arthritis patients. Clin Exp Immunol. (2012) 170:36–46. doi: 10.1111/j.1365-2249.2012.04626.x
35. Dragoljevic D, Kraakman MJ, Nagareddy PR, Ngo D, Shihata W, Kammoun HL, et al. Defective cholesterol metabolism in haematopoietic stem cells promotes monocyte-driven atherosclerosis in rheumatoid arthritis. Eur Heart J. (2018) 39:2158–67. doi: 10.1093/eurheartj/ehy119
36. Cook AD, Turner AL, Braine EL, Pobjoy J, Lenzo JC, Hamilton JA. Regulation of systemic and local myeloid cell subpopulations by bone marrow cell-derived granulocyte-macrophage colony-stimulating factor in experimental inflammatory arthritis. Arthritis Rheumatol. (2011) 63:2340–51. doi: 10.1002/art.30354
37. Bronte V, Pittet MJ. The spleen in local and systemic regulation of immunity. Immunity. (2013) 39:806–18. doi: 10.1016/j.immuni.2013.10.010
38. Leuschner F, Rauch PJ, Ueno T, Gorbatov R, Marinelli B, Lee WW, et al. Rapid monocyte kinetics in acute myocardial infarction are sustained by extramedullary monocytopoiesis. J Exp Med. (2012) 209:123–37. doi: 10.1084/jem.20111009
39. Robbins CS, Chudnovskiy A, Rauch PJ, Figueiredo JL, Iwamoto Y, Gorbatov R, et al. Extramedullary hematopoiesis generates ly-6Chigh monocytes that infiltrate atherosclerotic lesions. Circulation. (2012) 125:364–74. doi: 10.1161/CIRCULATIONAHA.111.061986
40. Dutta P, Courties G, Wei Y, Leuschner F, Gorbatov R, Robbins C, et al. Myocardial infarction accelerates atherosclerosis. Nature. (2012) 487:325. doi: 10.1038/nature11260
41. Coppin E, Florentin J, Vasamsetti SB, Arunkumar A, Sembrat J, Rojas M, et al. Splenic hematopoietic stem cells display a pre-activated phenotype. Immunol Cell Biol. (2018) 96(7):772–784. doi: 10.1111/imcb.12035
42. Loukov D, Naidoo A, Puchta A, Marin JLA, Bowdish DME. Tumor necrosis factor drives increased splenic monopoiesis in old mice. J Leukoc Biol. (2016) 100:121–9. doi: 10.1189/jlb.3MA0915-433RR
43. Hernandez G, Mills TS, Rabe JL, Chavez JS, Kuldanek S, Kirkpatrick G, et al. Pro-inflammatory cytokine blockade attenuates myeloid expansion in a murine model of rheumatoid arthritis. Haematologica. (2020) 105:585–97. doi: 10.3324/haematol.2018.197210
44. Ma YD, Park C, Zhao H, Oduro KA, Tu X, Long F, et al. Defects in osteoblast function but no changes in long-term repopulating potential of hematopoietic stem cells in a mouse chronic inflammatory arthritis model. Blood. (2009) 114:4402–10. doi: 10.1182/blood-2008-12-196311
45. Hamilton JA. Colony-stimulating factors in inflammation and autoimmunity. Nat Rev Immunol. (2008) 8:533–44. doi: 10.1038/nri2356
46. Caiado F, Manz MG. IL-1 in aging and pathologies of hematopoietic stem cells. Blood. (2024) 144:368–77. doi: 10.1182/blood.2023023105
47. Yamashita M, Passegué E. TNF-α Coordinates hematopoietic stem cell survival and myeloid regeneration. Cell Stem Cell. (2019) 25:357–72.e7. doi: 10.1016/j.stem.2019.05.019
48. Lorenz HM, Antoni C, Valerius T, Repp R, Grünke M, Schwerdtner N, et al. In vivo blockade of TNF-alpha by intravenous infusion of a chimeric monoclonal TNF-alpha antibody in patients with rheumatoid arthritis. Short term cellular and molecular effects. J Immunol. (1996) 156(4):1646–53.
49. Gengenbacher M, Sebald HJ, Villiger PM, Hofstetter W, Seitz M. Infliximab inhibits bone resorption by circulating osteoclast precursor cells in patients with rheumatoid arthritis and ankylosing spondylitis. Ann Rheum Dis. (2008) 67:620–4. doi: 10.1136/ard.2007.076711
50. Ziegler-Heitbrock L, Ancuta P, Crowe S, Dalod M, Grau V, Hart DN, et al. Nomenclature of monocytes and dendritic cells in blood. Blood. (2010) 116:e74–80. doi: 10.1182/blood-2010-02-258558
51. Patel AA, Zhang Y, Fullerton JN, Boelen L, Rongvaux A, Maini AA, et al. The fate and lifespan of human monocyte subsets in steady state and systemic inflammation. J Exp Med. (2017) 214(7):1913–23. doi: 10.1084/jem.20170355
52. Yona S, Kim KW, Wolf Y, Mildner A, Varol D, Breker M, et al. Fate mapping reveals origins and dynamics of monocytes and tissue macrophages under homeostasis. Immunity. (2013) 38:79–91. doi: 10.1016/j.immuni.2012.12.001
53. Bain CC, Bravo-Blas A, Scott CL, Perdiguero EG, Geissmann F, Henri S, et al. Constant replenishment from circulating monocytes maintains the macrophage pool in the intestine of adult mice. Nat Immunol. (2014) 15:929–37. doi: 10.1038/ni.2967
54. Tamoutounour S, Guilliams M, Montanana Sanchis F, Liu H, Terhorst D, Malosse C, et al. Origins and functional specialization of macrophages and of conventional and monocyte-derived dendritic cells in mouse skin. Immunity. (2013) 39:925–38. doi: 10.1016/j.immuni.2013.10.004
55. Mildner A, Yona S, Jung S. A close encounter of the third kind: monocyte-derived cells. Adv Immunol. (2013) 120:69–103. doi: 10.1016/B978-0-12-417028-5.00003-X
56. Auffray C, Fogg D, Garfa M, Elain G, Join-Lambert O, Kayal S, et al. Monitoring of blood vessels and tissues by a population of monocytes with patrolling behavior. Science. (2007) 317:666–70. doi: 10.1126/science.1142883
57. Cros J, Cagnard N, Woollard K, Patey N, Zhang SY, Senechal B, et al. Human CD14dim monocytes patrol and sense nucleic acids and viruses via TLR7 and TLR8 receptors. Immunity. (2010) 33:375–86. doi: 10.1016/j.immuni.2010.08.012
58. Rossol M, Kraus S, Pierer M, Baerwald C, Wagner U. The CD14(bright) CD16+ monocyte subset is expanded in rheumatoid arthritis and promotes expansion of the Th17 cell population. Arthritis Rheumatol. (2012) 64:671–7. doi: 10.1002/art.33418
59. Wong KL, Tai JJY, Wong WC, Han H, Sem X, Yeap WH, et al. Gene expression profiling reveals the defining features of the classical, intermediate, and nonclassical human monocyte subsets. Blood. (2011) 118:e16–31. doi: 10.1182/blood-2010-12-326355
60. Skrzeczyńska-Moncznik J, Bzowska M, Loseke S, Grage-Griebenow E, Zembala M, Pryjma J. Peripheral blood CD14high CD16+ monocytes are main producers of IL-10. Scand J Immunol. (2008) 67:152–9. doi: 10.1111/j.1365-3083.2007.02051.x
61. Ong SM, Hadadi E, Dang TM, Yeap WH, Tan CTY, Ng TP, et al. The pro-inflammatory phenotype of the human non-classical monocyte subset is attributed to senescence. Cell Death Dis. (2018) 9:266. doi: 10.1038/s41419-018-0327-1
62. Mack R, Zhang L, Breslin P, Zhang J. The fetal-to-adult hematopoietic stem cell transition and its role in childhood hematopoietic Malignancies. Stem Cell Rev Rep. (2021) 17:2059–80. doi: 10.1007/s12015-021-10230-x
63. Hegge I, Niepel F, Lange A, Vogelgesang A, Heckmann M, Ruhnau J. Functional analysis of granulocyte and monocyte subpopulations in neonates. Mol Cell Pediatr. (2019) 6:5. doi: 10.1186/s40348-019-0092-y
64. Wisgrill L, Groschopf A, Herndl E, Sadeghi K, Spittler A, Berger A, et al. Reduced TNF-α response in preterm neonates is associated with impaired nonclassic monocyte function. J Leukoc Biol. (2016) 100:607–12. doi: 10.1189/jlb.4A0116-001RR
65. Sohlberg E, Saghafian-Hedengren S, Bremme K, Sverremark-Ekström E. Cord blood monocyte subsets are similar to adult and show potent peptidoglycan-stimulated cytokine responses. Immunology. (2011) 133:41–50. doi: 10.1111/j.1365-2567.2011.03407.x
66. Murphy FJ, Reen DJ. Differential expression of function-related antigens on newborn and adult monocyte subpopulations. Immunology. (1996) 89:587–91. doi: 10.1046/j.1365-2567.1996.d01-788.x
67. Hearps AC, Martin GE, Angelovich TA, Cheng WJ, Maisa A, Landay AL, et al. Aging is associated with chronic innate immune activation and dysregulation of monocyte phenotype and function. Aging Cell. (2012) 11:867–75. doi: 10.1111/j.1474-9726.2012.00851.x
68. Nyugen J, Agrawal S, Gollapudi S, Gupta S. Impaired functions of peripheral blood monocyte subpopulations in aged humans. J Clin Immunol. (2010) 30:806–13. doi: 10.1007/s10875-010-9448-8
69. Sadeghi HM, Schnelle JF, Thoma JK, Nishanian P, Fahey JL. Phenotypic and functional characteristics of circulating monocytes of elderly persons. Exp Gerontol. (1999) 34:959–70. doi: 10.1016/S0531-5565(99)00065-0
70. Wang C, Cheng Y, Li B, Qiu X, Hu H, Zhang X, et al. Transcriptional characteristics and functional validation of three monocyte subsets during aging. Immun Ageing A. (2023) 20:50. doi: 10.1186/s12979-023-00377-1
71. Mohanty S, Joshi SR, Ueda I, Wilson J, Blevins TP, Siconolfi B, et al. Prolonged proinflammatory cytokine production in monocytes modulated by interleukin 10 after influenza vaccination in older adults. J Infect Dis. (2015) 211:1174–84. doi: 10.1093/infdis/jiu573
72. Kawanaka N, Yamamura M, Aita T, Morita Y, Okamoto A, Kawashima M, et al. CD14+,CD16+ blood monocytes and joint inflammation in rheumatoid arthritis. Arthritis Rheumatol. (2002) 46:2578–86. doi: 10.1002/art.v46:10
73. Iwahashi M, Yamamura M, Aita T, Okamoto A, Ueno A, Ogawa N, et al. Expression of Toll-like receptor 2 on CD16+ blood monocytes and synovial tissue macrophages in rheumatoid arthritis. Arthritis Rheumatol. (2004) 50:1457–67. doi: 10.1002/art.20219
74. Cooper DL, Martin SG, Robinson JI, Mackie SL, Charles CJ, Nam J, et al. FcγRIIIa expression on monocytes in rheumatoid arthritis: role in immune-complex stimulated TNF production and non-response to methotrexate therapy. PloS One. (2012) 7:e28918. doi: 10.1371/journal.pone.0028918
75. Weldon AJ, Moldovan I, Cabling MG, Hernandez EA, Hsu S, Gonzalez J, et al. Surface APRIL is elevated on myeloid cells and is associated with disease activity in patients with rheumatoid arthritis. J Rheumatol. (2015) 42:749–59. doi: 10.3899/jrheum.140630
76. Yoon BR, Yoo SJ, Choi YH, Chung YH, Kim J, Yoo IS, et al. Functional phenotype of synovial monocytes modulating inflammatory T-cell responses in rheumatoid arthritis (RA). PLoS One. (2014) 9:e109775. doi: 10.1371/journal.pone.0109775
77. Tsukamoto M, Seta N, Yoshimoto K, Suzuki K, Yamaoka K, Takeuchi T. CD14(bright)CD16+ intermediate monocytes are induced by interleukin-10 and positively correlate with disease activity in rheumatoid arthritis. Arthritis Res Ther. (2017) 19:28. doi: 10.1186/s13075-016-1216-6
78. Tsukamoto M, Suzuki K, Seta N, Takeuchi T. Increased circulating CD14brightCD16+ intermediate monocytes are regulated by TNF-α and IL-6 axis in accordance with disease activity in patients with rheumatoid arthritis. Clin Exp Rheumatol. (2018) 36:540–4.
79. Talmon M, Percio M, Obeng JA, Ruffinatti FA, Sola D, Sainaghi PP, et al. Transcriptomic profile comparison of monocytes from rheumatoid arthritis patients in treatment with methotrexate, anti-TNFa, abatacept or tocilizumab. PLoS One. (2023) 18:e0282564. doi: 10.1371/journal.pone.0282564
80. Binvignat M, Miao BY, Wibrand C, Yang MM, Rychkov D, Flynn E, et al. Single-cell RNA-Seq analysis reveals cell subsets and gene signatures associated with rheumatoid arthritis disease activity. JCI Insight. (2024) 9(16):e178499. doi: 10.1172/jci.insight.178499
81. Friedrich K, Sommer M, Strobel S, Thrum S, Blüher M, Wagner U, et al. Perturbation of the monocyte compartment in human obesity. Front Immunol. (2019) 10:1874. doi: 10.3389/fimmu.2019.01874
82. Sconocchia G, Keyvanfar K, El Ouriaghli F, Grube M, Rezvani K, Fujiwara H, et al. Phenotype and function of a CD56+ peripheral blood monocyte. Leukemia. (2005) 19:69–76. doi: 10.1038/sj.leu.2403550
83. Krasselt M, Baerwald C, Wagner U, Rossol M. CD56+ monocytes have a dysregulated cytokine response to lipopolysaccharide and accumulate in rheumatoid arthritis and immunosenescence. Arthritis Res Ther. (2013) 15:R139. doi: 10.1186/ar4321
84. Dutt TS, LaVergne SM, Webb TL, Baxter BA, Stromberg S, McFann K, et al. Comprehensive immune profiling reveals CD56+ Monocytes and CD31+ Endothelial cells are increased in severe COVID-19 disease. J Immunol. (2022) 208:685–96. doi: 10.4049/jimmunol.2100830
85. Campana S, De Pasquale C, Sidoti Migliore G, Pezzino G, Cavaliere R, Venanzi Rullo E, et al. Cutting edge: hyperinflammatory monocytes expressing CD56 abound in severe COVID-19 patients. J Immunol. (2022) 209:655–9. doi: 10.4049/jimmunol.2200021
86. Spyridopoulos I, Hoffmann J, Aicher A, Brümmendorf TH, Doerr HW, Zeiher AM, et al. Accelerated telomere shortening in leukocyte subpopulations of patients with coronary heart disease. Circulation. (2009) 120:1364–72. doi: 10.1161/CIRCULATIONAHA.109.854299
87. Hochstrasser T, Marksteiner J, Humpel C. Telomere length is age-dependent and reduced in monocytes of Alzheimer patients. Exp Gerontol. (2012) 47:160–3. doi: 10.1016/j.exger.2011.11.012
88. Sebastián C, Herrero C, Serra M, Lloberas J, Blasco MA, Celada A. Telomere shortening and oxidative stress in aged macrophages results in impaired STAT5a phosphorylation. J Immunol Baltim Md. (1950) 183:2356–64. doi: 10.4049/jimmunol.0901131
89. Kang Y, Zhang H, Zhao Y, Wang Y, Wang W, He Y, et al. Telomere dysfunction disturbs macrophage mitochondrial metabolism and the NLRP3 inflammasome through the PGC-1α/TNFAIP3 axis. Cell Rep. (2018) 22:3493–506. doi: 10.1016/j.celrep.2018.02.071
90. Zeng Z, Zhang W, Qian Y, Huang H, Wu DJH, He Z, et al. Association of telomere length with risk of rheumatoid arthritis: a meta-analysis and Mendelian randomization. Rheumatol Oxf Engl. (2020) 59:940–7. doi: 10.1093/rheumatology/kez524
91. Lee YH, Bae SC. Association between shortened telomere length and rheumatoid arthritis. Z Für Rheumatol. (2018) 77:160–7. doi: 10.1007/s00393-016-0209-9
92. Fujii H, Shao L, Colmegna I, Goronzy JJ, Weyand CM. Telomerase insufficiency in rheumatoid arthritis. Proc Natl Acad Sci. (2009) 106:4360–5. doi: 10.1073/pnas.0811332106
93. Li Y, Shen Y, Hohensinner P, Ju J, Wen Z, Goodman SB, et al. Deficient activity of the nuclease MRE11A induces T cell aging and promotes arthritogenic effector functions in patients with rheumatoid arthritis. Immunity. (2016) 45:903–16. doi: 10.1016/j.immuni.2016.09.013
94. Colmegna I, Diaz-Borjon A, Fujii H, Schaefer L, Goronzy JJ, Weyand CM. Defective proliferative capacity and accelerated telomeric loss of hematopoietic progenitor cells in rheumatoid arthritis. Arthritis Rheumatol. (2008) 58:990–1000. doi: 10.1002/art.23287
95. O’Donovan A, Pantell MS, Puterman E, Dhabhar FS, Blackburn EH, Yaffe K, et al. Cumulative inflammatory load is associated with short leukocyte telomere length in the Health, Aging and Body Composition Study. PloS One. (2011) 6:e19687. doi: 10.1371/journal.pone.0019687
96. Jurk D, Wilson C, Passos JF, Oakley F, Correia-Melo C, Greaves L, et al. Chronic inflammation induces telomere dysfunction and accelerates ageing in mice. Nat Commun. (2014) 5:4172. doi: 10.1038/ncomms5172
97. Rodier F, Coppé JP, Patil CK, Hoeijmakers WAM, Muñoz DP, Raza SR, et al. Persistent DNA damage signalling triggers senescence-associated inflammatory cytokine secretion. Nat Cell Biol. (2009) 11:973–9. doi: 10.1038/ncb1909
99. Zhao M, Wang Z, Yung S, Lu Q. Epigenetic dynamics in immunity and autoimmunity. Int J Biochem Cell Biol. (2015) 67:65–74. doi: 10.1016/j.biocel.2015.05.022
100. Garagnani P, Bacalini MG, Pirazzini C, Gori D, Giuliani C, Mari D, et al. Methylation of ELOVL2 gene as a new epigenetic marker of age. Aging Cell. (2012) 11:1132–4. doi: 10.1111/acel.2012.11.issue-6
101. Horvath S. DNA methylation age of human tissues and cell types. Genome Biol. (2013) 14:R115. doi: 10.1186/gb-2013-14-10-r115
102. Reynolds LM, Taylor JR, Ding J, Lohman K, Johnson C, Siscovick D, et al. Age-related variations in the methylome associated with gene expression in human monocytes and T cells. Nat Commun. (2014) 5:5366. doi: 10.1038/ncomms6366
103. Hannum G, Guinney J, Zhao L, Zhang L, Hughes G, Sadda S, et al. Genome-wide methylation profiles reveal quantitative views of human aging rates. Mol Cell. (2013) 2):359–67. doi: 10.1016/j.molcel.2012.10.016
104. Reynolds LM, Ding J, Taylor JR, Lohman K, Soranzo N, de la Fuente A, et al. Transcriptomic profiles of aging in purified human immune cells. BMC Genomics. (2015) 16:333. doi: 10.1186/s12864-015-1522-4
105. Saare M, Tserel L, Haljasmägi L, Taalberg E, Peet N, Eimre M, et al. Monocytes present age-related changes in phospholipid concentration and decreased energy metabolism. Aging Cell. (2020) 19:e13127. doi: 10.1111/acel.13127
106. Tserel L, Limbach M, Saare M, Kisand K, Metspalu A, Milani L, et al. CpG sites associated with NRP1, NRXN2 and miR-29b-2 are hypomethylated in monocytes during ageing. Immun Ageing A. (2014) 11:1. doi: 10.1186/1742-4933-11-1
107. Austin MK, Chen E, Ross KM, McEwen LM, Maclsaac JL, Kobor MS, et al. Early-life socioeconomic disadvantage, not current, predicts accelerated epigenetic aging of monocytes. Psychoneuroendocrinology. (2018) 97:131–4. doi: 10.1016/j.psyneuen.2018.07.007
108. Salpea P, Russanova VR, Hirai TH, Sourlingas TG, Sekeri-Pataryas KE, Romero R, et al. Postnatal development- and age-related changes in DNA-methylation patterns in the human genome. Nucleic Acids Res. (2012) 40:6477–94. doi: 10.1093/nar/gks312
109. Dobbs KR, Embury P, Koech E, Ogolla S, Munga S, Kazura JW, et al. Age-related differences in monocyte DNA methylation and immune function in healthy Kenyan adults and children. Immun Ageing A. (2021) 18:11. doi: 10.1186/s12979-021-00223-2
110. Rodríguez-Ubreva J, de-la-Calle-Fabregat C, Li T, Ciudad L, Ballestar ML, Català-Moll F, et al. Inflammatory cytokines shape a changing DNA methylome in monocytes mirroring disease activity in rheumatoid arthritis. Ann Rheum Dis. (2019) 78:1505–16. doi: 10.1136/annrheumdis-2019-215355
111. Mok A, Rhead B, Holingue C, Shao X, Quach HL, Quach D, et al. Hypomethylation of CYP2E1 and DUSP22 promoters associated with disease activity and erosive disease among rheumatoid arthritis patients. Arthritis Rheumatol Hoboken Nj. (2018) 70:528–36. doi: 10.1002/art.40408
112. Liebold I, Grützkau A, Göckeritz A, Gerl V, Lindquist R, Feist E, et al. Peripheral blood mononuclear cells are hypomethylated in active rheumatoid arthritis and methylation correlates with disease activity. Rheumatology. (2021) 60:1984–95. doi: 10.1093/rheumatology/keaa649
113. Craig G, Kenney H, Nilsson EE, Sadler-Riggleman I, Beck D, Skinner MK. Epigenome association study for DNA methylation biomarkers in buccal and monocyte cells for female rheumatoid arthritis. Sci Rep. (2021) 11:23789. doi: 10.1038/s41598-021-03170-6
114. Tao W, Concepcion AN, Vianen M, Marijnissen ACA, Lafeber FPGJ, Radstake TRDJ, et al. Multiomics and machine learning accurately predict clinical response to adalimumab and etanercept therapy in patients with rheumatoid arthritis. Arthritis Rheumatol Hoboken Nj. (2021) 73:212–22. doi: 10.1002/art.41516
115. Bruunsgaard H, Andersen-Ranberg K, Jeune B, Pedersen AN, Skinhoj P, Pedersen BK. A high plasma concentration of TNF- is associated with dementia in centenarians. J Gerontol A Biol Sci Med Sci. (1999) 54:M357–64. doi: 10.1093/gerona/54.7.M357
116. Paolisso G, Rizzo MR, Mazziotti G, Tagliamonte MR, Gambardella A, Rotondi M, et al. Advancing age and insulin resistance: role of plasma tumor necrosis factor-α. Am J Physiol-Endocrinol Metab. (1998) 275:E294–9. doi: 10.1152/ajpendo.1998.275.2.E294
117. Álvarez-Rodríguez L, López-Hoyos M, Muñoz-Cacho P, Martínez-Taboada VM. Aging is associated with circulating cytokine dysregulation. Cell Immunol. (2012) 273:124–32. doi: 10.1016/j.cellimm.2012.01.001
118. O’Mahony L, Holland J, Jackson J, Feighery C, Hennessy TPJ, Mealy K. Quantitative intracellular cytokine measurement: age-related changes in proinflammatory cytokine production. Clin Exp Immunol. (1998) 113:213–9. doi: 10.1046/j.1365-2249.1998.00641.x
119. Puchta A, Naidoo A, Verschoor CP, Loukov D, Thevaranjan N, Mandur TS, et al. TNF drives monocyte dysfunction with age and results in impaired anti-pneumococcal immunity. PloS Pathog. (2016) 12:e1005368. doi: 10.1371/journal.ppat.1005368
120. Pence BD, Yarbro JR. Aging impairs mitochondrial respiratory capacity in classical monocytes. Exp Gerontol. (2018) 108:112–7. doi: 10.1016/j.exger.2018.04.008
121. Boehmer ED, Goral J, Faunce DE, Kovacs EJ. Age-dependent decrease in Toll-like receptor 4-mediated proinflammatory cytokine production and mitogen-activated protein kinase expression. J Leukoc Biol. (2004) 75:342–9. doi: 10.1189/jlb.0803389
122. Boehmer ED, Meehan MJ, Cutro BT, Kovacs EJ. Aging negatively skews macrophage TLR2- and TLR4-mediated pro-inflammatory responses without affecting the IL-2-stimulated pathway. Mech Ageing Dev. (2005) 126:1305–13. doi: 10.1016/j.mad.2005.07.009
123. Bouchlaka MN, Sckisel GD, Chen M, Mirsoian A, Zamora AE, Maverakis E, et al. Aging predisposes to acute inflammatory induced pathology after tumor immunotherapy. J Exp Med. (2013) 210:2223–37. doi: 10.1084/jem.20131219
124. Lee DY, Lim JS, Cho KA. Differential activation of macrophages based on their environment in advanced age. Chonnam Med J. (2020) 56:12–9. doi: 10.4068/cmj.2020.56.1.12
125. Beharka AA, Meydani M, Wu D, Leka LS, Meydani A, Meydani SN. Interleukin-6 production does not increase with age. J Gerontol Ser A. (2001) 56:B81–8. doi: 10.1093/gerona/56.2.B81
126. Gather L, Nath N, Falckenhayn C, Oterino-Sogo S, Bosch T, Wenck H, et al. Macrophages Are Polarized toward an Inflammatory Phenotype by their Aged Microenvironment in the Human Skin. J Invest Dermatol. (2022) 142:3136–45.e11. doi: 10.1016/j.jid.2022.06.023
127. van Duin D, Mohanty S, Thomas V, Ginter S, Montgomery RR, Fikrig E, et al. Age-associated defect in human TLR-1/2 function. J Immunol Baltim Md. (1950) 178:970–5. doi: 10.4049/jimmunol.178.2.970
128. Mahbub S, Deburghgraeve CR, Kovacs EJ. Advanced age impairs macrophage polarization. J Interferon Cytokine Res. (2012) 32:18–26. doi: 10.1089/jir.2011.0058
129. Fallah MP, Chelvarajan RL, Garvy BA, Bondada S. Role of phosphoinositide 3-kinase-Akt signaling pathway in the age-related cytokine dysregulation in splenic macrophages stimulated via TLR-2 or TLR-4 receptors. Mech Ageing Dev. (2011) 132:274–86. doi: 10.1016/j.mad.2011.05.003
130. Gomez CR, Karavitis J, Palmer JL, Faunce DE, Ramirez L, Nomellini V, et al. Interleukin-6 contributes to age-related alteration of cytokine production by macrophages. Mediators Inflamm. (2010) 2010:475139. doi: 10.1155/2010/475139
131. Chelvarajan RL, Liu Y, Popa D, Getchell ML, Getchell TV, Stromberg AJ, et al. Molecular basis of age-associated cytokine dysregulation in LPS-stimulated macrophages. J Leukoc Biol. (2006) 79:1314–27. doi: 10.1189/jlb.0106024
132. Chen Y, Bradley SF. Aging and eliciting agents: effect on murine peritoneal macrophage monokine bioactivity. Exp Gerontol. (1993) 28:145–59. doi: 10.1016/0531-5565(93)90004-W
133. Cecílio CA, Costa EH, Simioni PU, Gabriel DL, Tamashiro WMSC. Aging alters the production of iNOS, arginase and cytokines in murine macrophages. Braz J Med Biol Res Rev Bras Pesqui Medicas E Biol. (2011) 44:671–81. doi: 10.1590/S0100-879X2011007500067
134. Boyd AR, Shivshankar P, Jiang S, Berton MT, Orihuela CJ. Age-related defects in TLR2 signaling diminish the cytokine response by alveolar macrophages during murine pneumococcal pneumonia. Exp Gerontol. (2012) 47:507–18. doi: 10.1016/j.exger.2012.04.004
135. Murciano C, Yáñez A, O’Connor JE, Gozalbo D, Gil ML. Influence of aging on murine neutrophil and macrophage function against Candida albicans. FEMS Immunol Med Microbiol. (2008) 2):214–21. doi: 10.1111/j.1574-695X.2008.00418.x
136. Thevaranjan N, Puchta A, Schulz C, Naidoo A, Szamosi JC, Verschoor CP, et al. Age-associated microbial dysbiosis promotes intestinal permeability, systemic inflammation, and macrophage dysfunction. Cell Host Microbe. (2017) 21:455–66.e4. doi: 10.1016/j.chom.2017.03.002
137. Gibon E, Loi F, Córdova LA, Pajarinen J, Lin T, Lu L, et al. Aging affects bone marrow macrophage polarization: relevance to bone healing. Regener Eng Transl Med. (2016) 2:98–104. doi: 10.1007/s40883-016-0016-5
138. Zhao H, Roychoudhury J, Doggett TA, Apte RS, Ferguson TA. Age-dependent changes in FasL (CD95L) modulate macrophage function in a model of age-related macular degeneration. Invest Ophthalmol Vis Sci. (2013) 54:5321–31. doi: 10.1167/iovs.13-12122
139. Ramirez A, Rathinam V, Fitzgerald KA, Golenbock DT, Mathew A. Defective pro-IL-1β responses in macrophages from aged mice. Immun Ageing. (2012) 9:27. doi: 10.1186/1742-4933-9-27
140. Shaik-Dasthagirisaheb YB, Kantarci A, Gibson FC. Immune response of macrophages from young and aged mice to the oral pathogenic bacterium Porphyromonas gingivalis. Immun Ageing A. (2010) 7:15. doi: 10.1186/1742-4933-7-15
141. Gómez CR, Acuña-Castillo C, Nishimura S, Pérez V, Escobar A, Salazar-Onfray F, et al. Serum from aged F344 rats conditions the activation of young macrophages. Mech Ageing Dev. (2006) 127:257–63. doi: 10.1016/j.mad.2005.10.002
142. Covarrubias AJ, Kale A, Perrone R, Lopez-Dominguez JA, Pisco AO, Kasler HG, et al. Senescent cells promote tissue NAD+ decline during ageing via the activation of CD38+ macrophages. Nat Metab. (2020) 2:1265–83. doi: 10.1038/s42255-020-00305-3
143. Chini CCS, Peclat TR, Warner GM, Kashyap S, Espindola-Netto JM, de Oliveira GC, et al. CD38 ecto-enzyme in immune cells is induced during aging and regulates NAD+ and NMN levels. Nat Metab. (2020) 2:1284–304. doi: 10.1038/s42255-020-00298-z
144. McQuattie-Pimentel AC, Ren Z, Joshi N, Watanabe S, Stoeger T, Chi M, et al. The lung microenvironment shapes a dysfunctional response of alveolar macrophages in aging. J Clin Invest. (2021) 131:e140299. doi: 10.1172/JCI140299
145. Chambers ES, Vukmanovic-Stejic M, Shih BB, Trahair H, Subramanian P, Devine OP, et al. Recruitment of inflammatory monocytes by senescent fibroblasts inhibits antigen-specific tissue immunity during human aging. Nat Aging. (2021) 1:101–13. doi: 10.1038/s43587-020-00010-6
146. Brennan FM, McInnes IB. Evidence that cytokines play a role in rheumatoid arthritis. J Clin Invest. (2008) 118:3537–45. doi: 10.1172/JCI36389
147. Rossol M, Meusch U, Pierer M, Kaltenhäuser S, Häntzschel H, Hauschildt S, et al. Interaction between transmembrane TNF and TNFR1/2 mediates the activation of monocytes by contact with T cells. J Immunol. (2007) 179(6):4239–48. doi: 10.4049/jimmunol.179.6.4239
148. Paoletti A, Rohmer J, Ly B, Pascaud J, Rivière E, Seror R, et al. Monocyte/macrophage abnormalities specific to rheumatoid arthritis are linked to miR-155 and are differentially modulated by different TNF inhibitors. J Immunol Author Choice. (2019) 203:1766–75. doi: 10.4049/jimmunol.1900386
149. Santos D, Campos TM, Saldanha M, Oliveira SC, Nascimento M, Zamboni DS, et al. IL-1β Production by intermediate monocytes is associated with immunopathology in cutaneous leishmaniasis. J Invest Dermatol. (2018) 138:1107–15. doi: 10.1016/j.jid.2017.11.029
150. Zhang F, Wei K, Slowikowski K, Fonseka CY, Rao DA, Kelly S, et al. Defining inflammatory cell states in rheumatoid arthritis joint synovial tissues by integrating single-cell transcriptomics and mass cytometry. Nat Immunol. (2019) 20:928–42. doi: 10.1038/s41590-019-0378-1
151. Sales LP, Hounkpe BW, Perez MO, Caparbo VF, Domiciano DS, Borba EF, et al. Transcriptomic characterization of classical monocytes highlights the involvement of immuno-inflammation in bone erosion in Rheumatoid Arthritis. Front Immunol. (2023) 14:1251034. doi: 10.3389/fimmu.2023.1251034
152. Leirisalo-Repo M, Paimela L, Jäätteä M, Koskimies S, Repo H. Production of TNF by monocytes of patients with early rheumatoid arthritis is increased. Scand J Rheumatol. (1995) 24:366–71. doi: 10.3109/03009749509095182
153. Jäger E, Murthy S, Schmidt C, Hahn M, Strobel S, Peters A, et al. Calcium-sensing receptor-mediated NLRP3 inflammasome response to calciprotein particles drives inflammation in rheumatoid arthritis. Nat Commun. (2020) 11:4243. doi: 10.1038/s41467-020-17749-6
154. Liou LB. Different monocyte reaction patterns in newly diagnosed, untreated rheumatoid arthritis and lupus patients probably confer disparate C-reactive protein levels. Clin Exp Rheumatol. (2003) 21:437–44.
155. Mege JL, Capo C, Michel B, Gastaut JL, Bongrand P. Phagocytic cell function in aged subjects. Neurobiol Aging. (1988) 9:217–20. doi: 10.1016/S0197-4580(88)80054-X
156. Gardner ID, Lim STK, Lawton JWM. Monocyte function in ageing humans. Mech Ageing Dev. (1981) 16:233–9. doi: 10.1016/0047-6374(81)90099-3
157. Bliederhaeuser C, Grozdanov V, Speidel A, Zondler L, Ruf WP, Bayer H, et al. Age-dependent defects of alpha-synuclein oligomer uptake in microglia and monocytes. Acta Neuropathol (Berl). (2016) 131:379–91. doi: 10.1007/s00401-015-1504-2
158. Moss CE, Johnston SA, Kimble JV, Clements M, Codd V, Hamby S, et al. Aging-related defects in macrophage function are driven by MYC and USF1 transcriptional programs. Cell Rep. (2024) 43:114073. doi: 10.1016/j.celrep.2024.114073
159. De La Fuente M. Changes in the macrophage function with aging. Comp Biochem Physiol A Physiol. (1985) 81:935–8. doi: 10.1016/0300-9629(85)90933-8
160. Mancuso P, McNish RW, Peters-Golden M, Brock TG. Evaluation of phagocytosis and arachidonate metabolism by alveolar macrophages and recruited neutrophils from F344xBN rats of different ages. Mech Ageing Dev. (2001) 122:1899–913. doi: 10.1016/S0047-6374(01)00322-0
161. Aprahamian T, Takemura Y, Goukassian D, Walsh K. Ageing is associated with diminished apoptotic cell clearance in vivo. Clin Exp Immunol. (2008) 152:448–55. doi: 10.1111/j.1365-2249.2008.03658.x
162. Kim OH, Kim H, Kang J, Yang D, Kang YH, Lee DH, et al. Impaired phagocytosis of apoptotic cells causes accumulation of bone marrow-derived macrophages in aged mice. BMB Rep. (2017) 50:43–8. doi: 10.5483/BMBRep.2017.50.1.167
163. Takahashi R, Totsuka S, Ishigami A, Kobayashi Y, Nagata K. Attenuated phagocytosis of secondary necrotic neutrophils by macrophages in aged and SMP30 knockout mice. Geriatr Gerontol Int. (2016) 16:135–42. doi: 10.1111/ggi.2016.16.issue-1
164. Linehan E, Dombrowski Y, Snoddy R, Fallon PG, Kissenpfennig A, Fitzgerald DC. Aging impairs peritoneal but not bone marrow-derived macrophage phagocytosis. Aging Cell. (2014) 13:699–708. doi: 10.1111/acel.2014.13.issue-4
165. Bar-Eli M, Ehrenfeld M, Litvin Y, Gallily R. Monocyte function in rheumatoid arthritis. Scand J Rheumatol. (1980) 9:17–23. doi: 10.1080/03009748009098122
166. Wynne KM, Dieppe PA, Scott J, Huskisson EC. Cellular phagocytic studies in rheumatoid arthritis patients treated with levamisole. Ann Rheum Dis. (1981) 40:382–7. doi: 10.1136/ard.40.4.382
167. Hurst NP, Nuki G. Evidence for defect of complement-mediated phagocytosis by monocytes from patients with rheumatoid arthritis and cutaneous vasculitis. Br Med J Clin Res Ed. (1981) 282:2081–3. doi: 10.1136/bmj.282.6282.2081
168. Steven MM, Lennie SE, Sturrock RD, Gemmell CG. Enhanced bacterial phagocytosis by peripheral blood monocytes in rheumatoid arthritis. Ann Rheum Dis. (1984) 43:435–9. doi: 10.1136/ard.43.3.435
169. Fragoulis GE, Vakrakou AG, Papadopoulou A, Germenis A, Kanavakis E, Moutsopoulos HM, et al. Impaired degradation and aberrant phagocytosis of necrotic cell debris in the peripheral blood of patients with primary Sjögren’s syndrome. J Autoimmun. (2015) 56:12–22. doi: 10.1016/j.jaut.2014.08.004
170. Tas SW, Quartier P, Botto M, Fossati-Jimack L. Macrophages from patients with SLE and rheumatoid arthritis have defective adhesion in vitro, while only SLE macrophages have impaired uptake of apoptotic cells. Ann Rheum Dis. (2006) 65:216–21. doi: 10.1136/ard.2005.037143
171. Lee SJ, Yoon BR, Kim HY, Yoo SJ, Kang SW, Lee WW. Activated platelets convert CD14+CD16- into CD14+CD16+ Monocytes with enhanced fcγR-mediated phagocytosis and skewed M2 polarization. Front Immunol. (2020) 11:611133. doi: 10.3389/fimmu.2020.611133
172. Park JB. Phagocytosis induces superoxide formation and apoptosis in macrophages. Exp Mol Med. (2003) 35:325–35. doi: 10.1038/emm.2003.44
173. Novo E, Parola M. Redox mechanisms in hepatic chronic wound healing and fibrogenesis. Fibrogenesis Tissue Repair. (2008) 1:5. doi: 10.1186/1755-1536-1-5
174. Alvarez E, Conde M, MaChado A, Sobrino F, Santa Maria C. Decrease in free-radical production with age in rat peritoneal macrophages. Biochem J. (1995) 312:555–60. doi: 10.1042/bj3120555
175. Alvarez E, María CS, MaChado A. Respiratory burst reaction changes with age in rat peritoneal macrophages. Biochim Biophys Acta BBA - Mol Cell Res. (1993) 1179:247–52. doi: 10.1016/0167-4889(93)90079-5
176. Bruce IN, McNally JA, Rea IM, Bell AL. Age-related changes in non-receptor dependent generation of reactive oxygen species from phagocytes of healthy adults. Mech Ageing Dev. (1997) 94:135–44. doi: 10.1016/S0047-6374(96)01867-2
177. Alvarez E, Santa María C. Influence of the age and sex on respiratory burst of human monocytes. Mech Ageing Dev. (1996) 90:157–61. doi: 10.1016/0047-6374(96)01763-0
178. Ganguly R, Shamblin PB, Craig CP. Microbicidal activity and superoxide production by macrophages in aging rats. Allerg Immunol (Leipz). (1984) 30:225–9.
179. Petrequin PR, Johnson AG. Macrophage activation by adjuvants in aging mice. J Leukoc Biol. (1984) 35:251–63. doi: 10.1002/jlb.35.3.251
180. Esposito AL, Clark CA, Poirier WJ. An assessment of the respiratory burst and bactericidal activity of alveolar macrophages from adult and senescent mice. J Leukoc Biol. (1988) 43:445–54. doi: 10.1002/jlb.43.5.445
181. Jacinto TA, Meireles GS, Dias AT, Aires R, Porto ML, Gava AL, et al. Increased ROS production and DNA damage in monocytes are biomarkers of aging and atherosclerosis. Biol Res. (2018) 51:33. doi: 10.1186/s40659-018-0182-7
182. Davalli P, Mitic T, Caporali A, Lauriola A, D’Arca D. ROS, Cell Senescence, and novel molecular mechanisms in aging and age-related diseases. Oxid Med Cell Longev. (2016) 2016:1–18. doi: 10.1155/2016/3565127
183. Hayashi T, Furukawa K, Morishita Y, Hayashi I, Kato N, Yoshida K, et al. Intracellular reactive oxygen species level in blood cells of atomic bomb survivors is increased due to aging and radiation exposure. Free Radic Biol Med. (2021) 171:126–34. doi: 10.1016/j.freeradbiomed.2021.05.017
184. Ostrakhovitch EA, Afanas’ev IB. Oxidative stress in rheumatoid arthritis leukocytes: suppression by rutin and other antioxidants and chelators 1 1Abbreviations: CL, chemiluminescence; DF, desferrioxamine; DHR, dihydrorhodamine; FA, Fanconi anemia; HBSS, Hanks’ balanced salt solution; NMMA, NG-monomethyl l-arginine; NO, nitric oxide; PMA, 12-O-myristate 13-acetate; PMN, polymorphonuclear; RA, rheumatoid arthritis, and SOD, superoxide dismutase. Biochem Pharmacol. (2001) 62:743–6. doi: 10.1016/S0006-2952(01)00707-9
185. Repo H, Paimela L, Leirisalo-Repo M. Chemiluminescence responses and chemotaxis of monocytes from patients with early rheumatoid arthritis. Scand J Rheumatol. (1996) 25:92–6. doi: 10.3109/03009749609069214
186. Hurst NP, Bessac B, Nuki G. Monocyte superoxide anion production in rheumatoid arthritis: preliminary evidence for enhanced rates of superoxide anion production by monocytes from patients receiving penicillamine, sodium aurothiomalate and corticosteroids. Ann Rheum Dis. (1984) 43:28–33. doi: 10.1136/ard.43.1.28
187. Hurst NP, Bell AL, Nuki G. Studies of the effect of D-penicillamine and sodium aurothiomalate therapy on superoxide anion production by monocytes from patients with rheumatoid arthritis: evidence for in vivo stimulation of monocytes. Ann Rheum Dis. (1986) 45:37–43. doi: 10.1136/ard.45.1.37
188. Mateen S, Moin S, Khan AQ, Zafar A, Fatima N. Increased reactive oxygen species formation and oxidative stress in rheumatoid arthritis. PLoS One. (2016) 11:e0152925. doi: 10.1371/journal.pone.0152925
189. Kundu S, Ghosh P, Datta S, Ghosh A, Chattopadhyay S, Chatterjee M. Oxidative stress as a potential biomarker for determining disease activity in patients with Rheumatoid Arthritis. Free Radic Res. (2012) 46:1482–9. doi: 10.3109/10715762.2012.727991
190. Viola A, Munari F, Sánchez-Rodríguez R, Scolaro T, Castegna A. The metabolic signature of macrophage responses. Front Immunol. (2019) 10:1462. doi: 10.3389/fimmu.2019.01462
191. Lesnefsky EJ, Hoppel CL. Oxidative phosphorylation and aging. Ageing Res Rev. (2006) 5:402–33. doi: 10.1016/j.arr.2006.04.001
192. Kruglov V, Jang IH, Camell CD. Inflammaging and fatty acid oxidation in monocytes and macrophages. Immunometabolism Cobham Surrey. (2024) 6:e00038. doi: 10.1097/IN9.0000000000000038
193. Maoldomhnaigh CÓ, Cox DJ, Phelan JJ, Malone FD, Keane J, Basdeo SA. The Warburg Effect Occurs Rapidly in Stimulated Human Adult but Not Umbilical Cord Blood Derived Macrophages. Front Immunol. (2021) 12:657261. doi: 10.3389/fimmu.2021.657261
194. Bratic A, Larsson NG. The role of mitochondria in aging. J Clin Invest. (2013) 123:951–7. doi: 10.1172/JCI64125
195. Park H, Jeoung NH. Inflammation increases pyruvate dehydrogenase kinase 4 (PDK4) expression via the Jun N-Terminal Kinase (JNK) pathway in C2C12 cells. Biochem Biophys Res Commun. (2016) 469:1049–54. doi: 10.1016/j.bbrc.2015.12.113
196. Pence BD, Yarbro JR. Classical monocytes maintain ex vivo glycolytic metabolism and early but not later inflammatory responses in older adults. Immun Ageing. (2019) 16:3. doi: 10.1186/s12979-019-0143-1
197. Pararasa C, Bailey CJ, Griffiths HR. Ageing, adipose tissue, fatty acids and inflammation. Biogerontology. (2015) 16:235–48. doi: 10.1007/s10522-014-9536-x
198. Wang M, Yan Y, Zhang Z, Yao X, Duan X, Jiang Z, et al. Programmed PPAR-α downregulation induces inflammaging by suppressing fatty acid catabolism in monocytes. iScience. (2021) 24:102766. doi: 10.1016/j.isci.2021.102766
199. Yoshino J, Baur JA, Imai SI. NAD+ Intermediates: the biology and therapeutic potential of NMN and NR. Cell Metab. (2018) 27:513–28. doi: 10.1016/j.cmet.2017.11.002
200. Clement J, Wong M, Poljak A, Sachdev P, Braidy N. The plasma NAD + Metabolome is dysregulated in “Normal” Aging. Rejuvenation Res. (2019) 22:121–30. doi: 10.1089/rej.2018.2077
201. Liu L, Su X, Quinn WJ, Hui S, Krukenberg K, Frederick DW, et al. Quantitative analysis of NAD synthesis-breakdown fluxes. Cell Metab. (2018) 27:1067–80.e5. doi: 10.1016/j.cmet.2018.03.018
202. Minhas PS, Liu L, Moon PK, Joshi AU, Dove C, Mhatre S, et al. Macrophage de novo NAD+ synthesis specifies immune function in aging and inflammation. Nat Immunol. (2019) 20:50–63. doi: 10.1038/s41590-018-0255-3
203. Cameron AM, Castoldi A, Sanin DE, Flachsmann LJ, Field CS, Puleston D, et al. Inflammatory macrophage dependence on NAD+ salvage is a consequence of reactive oxygen species–mediated DNA damage. Nat Immunol. (2019) 20:420–32. doi: 10.1038/s41590-019-0336-y
204. Kim S, Hwang J, Xuan J, Jung YH, Cha HS, Kim KH. Global metabolite profiling of synovial fluid for the specific diagnosis of rheumatoid arthritis from other inflammatory arthritis. PloS One. (2014) 9:e97501. doi: 10.1371/journal.pone.0097501
205. Shime H, Yabu M, Akazawa T, Kodama K, Matsumoto M, Seya T, et al. Tumor-secreted lactic acid promotes IL-23/IL-17 proinflammatory pathway. J Immunol. (2008) 180:7175–83. doi: 10.4049/jimmunol.180.11.7175
206. Michopoulos F, Karagianni N, Whalley NM, Firth MA, Nikolaou C, Wilson ID, et al. Targeted metabolic profiling of the Tg197 mouse model reveals itaconic acid as a marker of rheumatoid arthritis. J Proteome Res. (2016) 15:4579–90. doi: 10.1021/acs.jproteome.6b00654
207. Lampropoulou V, Sergushichev A, Bambouskova M, Nair S, Vincent EE, Loginicheva E, et al. Itaconate links inhibition of succinate dehydrogenase with macrophage metabolic remodeling and regulation of inflammation. Cell Metab. (2016) 24:158–66. doi: 10.1016/j.cmet.2016.06.004
Keywords: monocytes, macrophages, aging, immunosenescence, rheumatoid arthritis, inflammaging, monocyte metabolism
Citation: Basu S, Ulbricht Y and Rossol M (2025) Healthy and premature aging of monocytes and macrophages. Front. Immunol. 16:1506165. doi: 10.3389/fimmu.2025.1506165
Received: 04 October 2024; Accepted: 28 February 2025;
Published: 17 March 2025.
Edited by:
Calogero Caruso, University of Palermo, ItalyReviewed by:
Barbara Molon, University of Padua, ItalyLuciana Cavalheiro Marti, Albert Einstein Israelite Hospital, Brazil
Copyright © 2025 Basu, Ulbricht and Rossol. This is an open-access article distributed under the terms of the Creative Commons Attribution License (CC BY). The use, distribution or reproduction in other forums is permitted, provided the original author(s) and the copyright owner(s) are credited and that the original publication in this journal is cited, in accordance with accepted academic practice. No use, distribution or reproduction is permitted which does not comply with these terms.
*Correspondence: Manuela Rossol, cm9zc29sQGItdHUuZGU=