Introduction
Sepsis is a life-threatening multi-organ dysfunction syndrome (MODS) associated with dysregulated body responses to infection and is the leading cause of death in intensive care units (1–3). In 2017, a total of 48.9 million cases of sepsis and 11 million sepsis-related deaths were recorded worldwide, the latter accounting for approximately 20% of global deaths (4–6). Despite decades of research, substantial breakthroughs in specific treatments have yet to be achieved. High incidence and mortality rates, coupled with limited non-specific treatment options, render sepsis a significant challenge in the medical field and cause substantial social and economic burdens.
Aggressive early sepsis identification and treatment initiatives are essential to reducing mortality in patients with sepsis. Traditional sepsis treatments primarily focus on infection control (7–9), hemodynamic stability (10–12), and the maintenance of organ function (13–15). However, these traditional treatment strategies often show limited efficacy. Such therapeutic failure may be attributed to various factors, including the development of drug resistance (16, 17), pharmacokinetic alterations induced by sepsis (18), and an inability to address the underlying immune dysregulation (19). Rapidly advancing nanotechnology has unlocked a new stage in sepsis treatment. Nanotechnology is mainly based on the processes of designing, synthesizing, and utilizing nanomaterials (NMs) within the size range of 1–100 nm (20, 21). NMs exhibit unique properties, such as high surface area, tunable dimensions, and the capability to target specific cells or pathogens, enabling them to interact directly with infectious agents or modulate immune responses (22–24). These advantages make NMs particularly effective in pathogen removal, drug delivery, and inflammation reduction, thereby offering promising solutions in areas where traditional therapies fall short (22–24). However, it must be noted that while the prospects of NMs in sepsis treatment are encouraging, their application to date remains mainly focused on antimicrobial and anti-inflammatory responses, somewhat neglecting the complex immunopathological mechanisms of sepsis. From an immunological perspective, sepsis is currently considered to display stages of excessive inflammation and immunosuppression, which dynamically coexist or alternate (25, 26). The occurrence of immunosuppression is increasingly recognized as a key factor in immune dysfunction and sepsis mortality (27, 28). Therefore, we propose that the use of NMs in sepsis treatment should be expanded from an emphasis on anti-inflammatory effects to include a focus on reversing immunosuppression and thus restoring the body’s immune homeostasis.
Key role of immune dysregulation in the progression of sepsis
To date, there is no gold standard for defining sepsis, as the pathogenesis of this disease involves multiple pathological mechanisms. The gradually increasing understanding of the mechanisms of sepsis has brought with it three successive and significant revisions in the definition of sepsis. Initially, sepsis was defined as a systemic inflammatory response syndrome (SIRS) caused by infection (29, 30). In 2016, new guidelines redefined sepsis as a life-threatening organ dysfunction caused by a dysregulated host response to infection (1). The latest definition of sepsis emphasizes the importance of host immune dysregulation caused by infection, which goes beyond the possibility of direct death from the infection itself (31–33).
The development of immune dysregulation during sepsis is typically divided into two closely related but distinct stages: early systemic inflammatory response and later immunosuppression (25, 26). In the early stages of infection, pathogens enter the body and trigger the activation of the innate immune system through pathogen-associated molecular patterns (PAMPs) (34–36). This response is intended to eliminate pathogens and protect body tissues from further damage. However, in some cases, such as when the infection load is too heavy or the individual’s immune system response is abnormal, the immune response cannot return to homeostasis. The inflammatory response is then further amplified and produces a series of damage-associated molecular patterns (DAMPs) in response to tissue damage and cell death (37, 38). In this process, excessive pro-inflammatory cascades lead to a large accumulation of cytokines (39, 40) and trigger many pathological events, such as complement activation (41, 42), coagulation dysfunction (43, 44), and exhaustion and metabolic reprogramming of immune cells (45–47). These phenomena exacerbate immune dysregulation and cause immunosuppression, which in turn leads to a reduction in infection clearance and makes the individual more susceptible to secondary infections, ultimately worsening the outcome of sepsis.
It is worth noting that these two stages do not strictly appear in chronological order. In fact, they often coexist during the course of sepsis, and which stage predominates may depend on multiple host and pathogen factors (48, 49). Host factors include the individual’s genetic background, age, baseline health status, and comorbidities, while pathogen factors include type, virulence, and load. Growing evidence suggests that immunosuppression is a major cause of sepsis-related mortality, with approximately 70% of clinical deaths in septic patients occurring during this phase (25, 26). Despite significant progress in understanding the immune mechanisms of sepsis, the coexistence and alternation of excessive inflammation and severe immunosuppression add significant complexity to its treatment. For instance, excessive inflammation can promote immunosuppression by inducing immune cell exhaustion and death. Therefore, early anti-inflammatory therapy is essential to mitigate immunosuppression. However, poorly timed or excessive anti-inflammatory treatment can also exacerbate immunosuppression. Hence, achieving a balance between controlling inflammation and preventing immunosuppression is crucial for optimizing therapeutic strategies in sepsis management.
Current NMs-based treatments for sepsis
NMs have revolutionized the therapeutic approach to sepsis by utilizing a diverse array of materials, including inorganic and organic substances, of both natural and synthetic origins, or even innovative combinations thereof (22–24). This diversity allows for tailored solutions targeting the complex pathophysiology of sepsis. Given the breadth and depth of studies on this topic, it is not feasible to cover all research comprehensively. Therefore, Table 1 illustrates representative examples of nanoparticles employed in sepsis treatment, categorized by their specific mechanisms of action.
The anti-septic efficacy of NMs can be broadly categorized based on four key mechanisms. Firstly, NMs serve as highly efficient delivery systems for anti-septic drugs, increasing drug efficacy and reducing systemic side effects. This targeted drug delivery is crucial in sepsis, where rapid and localized intervention is often required. Secondly, certain NMs can directly eradicate pathogens or neutralize toxins produced by these pathogens. This pathogen-specific mechanism of action is particularly valuable in the early stages of sepsis, where the pathogen burden is high. Thirdly, NMs can modulate the host’s immune response to attenuate the overwhelming inflammatory reactions typical of sepsis. By interacting with immune cells or acting as scavengers for inflammatory cytokines, they prevent the progression to severe systemic inflammation and multi-organ failure. Lastly, NMs can be engineered for combination therapy, simultaneously providing antimicrobial and anti-inflammatory effects. This dual functionality ensures a comprehensive approach to sepsis management, addressing both the underlying infection and the detrimental host immune response.
Improving drug delivery efficiency
NMs provide a multifaceted approach to enhancing the delivery of therapeutic agents, particularly for treating sepsis. Their unique sizes and properties offer significant advantages in various aspects of drug delivery. By encapsulating drugs, they protect these agents from enzymatic degradation and harsh physiological conditions, thereby improving stability and bioavailability until the drugs reach the infection site (50). For instance, liposomal nanoparticles can safeguard antibiotics from premature breakdown in the bloodstream, preserving their potency and allowing for reduced dosing frequencies (51–53). Additionally, NMs can be engineered for intelligent, controlled, and sustained release. They respond to specific environmental stimuli characteristic of infection sites—such as pH changes (54, 55), bacterial enzyme concentrations (56, 57), or reactive oxygen species (ROS) variations (58, 59)—to release their payload precisely when needed. The functionalization of these NMs enables targeted delivery to specific cells or tissues by attaching ligands, antibodies, or peptides to their surfaces. This strategy concentrates therapeutic agents at the site of infection while minimizing adverse effects and reducing the development of drug resistance. Moreover, their ability to overcome biological barriers, such as penetrating bacterial biofilms and accessing intracellular pathogens, facilitates the delivery of antibiotics directly to otherwise inaccessible infection sites (60, 61). Collectively, these capabilities of NMs significantly enhance therapeutic efficacy and safety, ultimately leading to improved treatment outcomes.
Direct pathogen clearance
Some NMs exhibit inherent antimicrobial properties, offering a promising approach for combating bacterial infections, particularly those caused by multidrug-resistant strains (62, 63). Among these, silver nanoparticles (AgNPs) have been extensively researched for their ability to eradicate a broad spectrum of bacteria through various mechanisms. These include the disruption of bacterial cell walls and membranes, leading to structural damage and increased permeability (64, 65); the promotion of ROS production, which induces oxidative stress and damages vital cellular components (66, 67); and the interference with bacterial DNA and RNA replication, thereby hindering cell proliferation and survival (68, 69). Similarly, gold nanoparticles (AuNPs) and zinc nanoparticles (ZnNPs) also demonstrate intrinsic antimicrobial activity by penetrating bacterial cells and disrupting metabolic processes, resulting in bacterial death (70, 71). Moreover, some NMs serve as catalysts that enhance the effects of external antimicrobial agents or stimuli. For instance, magnetic nanoparticles can be activated under exogenous electromagnetic stimulation, generating localized heating (hyperthermia) or mechanical vibrations that disrupt bacterial cell structures (72, 73). This targeted antimicrobial therapy minimizes damage to surrounding healthy tissues while reducing systemic side effects. Furthermore, surface modification of NMs can significantly improve their interaction with bacterial cells. Functionalizing nanoparticles with specific ligands or antibodies increases their binding affinity to bacterial targets, thereby enhancing selectivity and efficacy (74, 75). Collectively, these advances underscore the potential of NMs as effective agents in the fight against bacterial infections.
Inhibition of excessive inflammatory response
NMs can also effectively inhibit excessive inflammatory responses by clearing ROS, adsorbing (neutralizing) excessive inflammatory mediators in the body, and targeting immune cells. During the development of sepsis, excessive inflammation causes a large amount of ROS to be produced in cells; these include H2O2, hydroxyl radicals, and superoxide anions (76). Excessive ROS will cause severe oxidative stress in cells, leading to cell death and consequent release of a large number of DAMPs (37, 38). These are further recognized by pattern recognition receptors (PRRs), forming a vicious cycle of inflammation amplification. Suitably modified NMs, such as tungsten disulfide nanoparticles, mesoporous selenium nanoparticles, and manganese dioxide nanoparticles, demonstrate excellent ROS clearance efficiency and bioavailability, which can effectively inhibit the inflammatory response in sepsis (77, 78). The development of excessive inflammation in sepsis is mediated by various inflammatory mediators, which may derive from pathogens (such as endotoxins) (79) or from the body itself (including free nucleic acids, histones, and pro-inflammatory cytokines) (80). Some NMs, such as chitosan, cationic polymers, and biomimetic nanoparticles, have the capability to scavenge toxins from pathogens or inflammatory mediators produced by the body, thereby inhibiting the progression of excessive inflammation (81, 82). In addition, the use of NMs to target immune cells and block overactive immune responses can also achieve effective control of inflammation (83, 84).
Combination therapy
The pathophysiology of sepsis is intricate, and relying solely on antimicrobial or anti-inflammatory therapies may be insufficient for effective management. Consequently, numerous researchers have investigated combination therapies that integrate both antimicrobial and anti-inflammatory strategies. One promising approach involves the use of nanomaterials as carriers to co-deliver antibacterial and anti-inflammatory agents, thereby enhancing therapeutic efficacy (85–89). For instance, Zhang et al. developed polymeric nanoparticles capable of efficiently releasing the antibiotic ciprofloxacin and the anti-inflammatory agent TPCA-1, which resulted in a survival rate of up to 90% in septic mice (89). Additionally, treatment strategies can employ inherently antimicrobial nanomaterials as carriers for anti-inflammatory drugs or modify these materials to exhibit dual antimicrobial and anti-inflammatory properties (90–92). Such dual-function nanomaterials offer a versatile platform for addressing the multifaceted nature of sepsis by simultaneously targeting infection and inflammation. These innovative combination therapies not only improve survival outcomes in experimental models but also hold potential for translation into clinical applications, representing a significant advancement in the treatment of sepsis.
Challenges in the use of NMs for sepsis treatment
NMs are increasingly recognized as a promising medium for antibacterial and anti-inflammatory applications. However, several significant limitations persist in their application in sepsis treatment. The primary issue is that current strategies focus predominantly on suppressing hyperinflammation, with insufficient attention being paid to modulating the immunosuppressive phase. Several points should be noted in this regard.
Firstly, sepsis, as a heterogeneous disease syndrome, does not simply follow a strict sequence of systemic inflammatory response and immunosuppressive phases. In many cases, these two phases may overlap, with dominance depending on various factors related to the host and pathogen (25, 26). Given the complexity of sepsis pathogenesis, distinguishing these two phases to determine the optimal timing for NM treatment is a challenging task. Implementing aggressive anti-inflammatory treatment at an inappropriate time could conceivably exacerbate a sepsis condition already in the immunosuppressive phase. Such misdirected treatment may suppress essential immune functions, heightening the risk of secondary infections and worsening patient outcomes. Therefore, a nuanced understanding of the patient’s immunological status is crucial for the effective application of NM therapies.
Secondly, it is well known that the surge of inflammatory molecules is a necessary condition for the immune system to combat invading pathogens (93, 94). Although NMs possess potent anti-inflammatory properties, excessive suppression of the inflammatory response could lead to earlier and more intense immunosuppression, making the host more susceptible to secondary infections. In fact, in some drug trials, the mortality rate of sepsis patients was shown to increase due to excessive suppression of the inflammatory response (95). Similarly, while intracellular ROS can promote inflammation and potentially cause various cellular dysfunctions, an appropriate level of ROS generation is beneficial for pathogen clearance (96, 97). In contrast, large-scale clinical trials have demonstrated that supplementation with classical antioxidants (such as vitamins C and E) has limited efficacy and may even increase the risk of sepsis (11, 98). Similarly, while the strategy of controlling sepsis through the adsorption of inflammatory mediators or endotoxins by NMs seems theoretically feasible, currently available treatments have not demonstrated a reduction in mortality rates in clinical practice (11). Some literature suggests that suppressing pro-inflammatory cytokines during infection does not provide protective effects against sepsis (99–101). Notably, several trials have reported an increased mortality rate in patients with Gram-positive bacterial infections following anti-endotoxin treatment (102).
Thirdly, the application of NMs in sepsis therapy presents a paradoxical challenge due to their potential to invoke significant pro-inflammatory responses. The introduction of NMs into biological systems can activate immune cells, leading to the excessive release of pro-inflammatory cytokines and chemokines (103–105). This hyperinflammatory state not only results in direct tissue damage but also exacerbates the subsequent immunosuppressive phase characteristic of sepsis. Such overactivation depletes immune resources, compromising the body’s ability to combat secondary infections and leading to more severe immune dysfunction (103–105). Additionally, the physicochemical attributes of NMs—such as size, shape, surface charge, and composition—critically influence their biocompatibility and immunogenicity. Accumulation in vital organs may provoke cytotoxic effects and oxidative stress, further impairing organ function (106–108). Therefore, a comprehensive understanding of these factors is essential to mitigate adverse effects.
Fourthly, it is crucial to recognize that the development of sepsis is often associated with dysbiosis of the host’s microbiota. Clinical studies have demonstrated that patients experiencing microbiota imbalance, particularly following hospital interventions like antibiotic treatments, have a significantly increased risk of developing sepsis and septic shock within 90 days (109, 110). Dysbiosis disrupts the delicate equilibrium of commensal microorganisms that play a vital role in immune system modulation. This disruption can impair gut barrier function, leading to translocation of pathogens into the bloodstream and triggering systemic inflammation (111, 112). Moreover, dysbiosis may promote immunosuppression by altering the maturation and function of immune cells, rendering the host more susceptible to infections (113). Although some NMs possess broad-spectrum antibacterial properties, their use could inadvertently exacerbate host dysbiosis. The non-selective elimination of beneficial microbiota might further compromise the immune system, negatively impacting sepsis treatment outcomes. Therefore, while designing nanomaterial-based therapies, it is imperative to consider their effects on the host microbiota.
Fifthly, it is imperative to consider that the efficacy of NMs in sepsis treatment may be significantly modulated by individual host differences. As previously noted, the progression of the inflammatory response and subsequent immunosuppressive phases in sepsis is profoundly influenced by host-specific factors. Notably, the trajectory of the immunosuppressive phase exhibits substantial heterogeneity among different patient populations, including adults, the elderly, infants, and individuals with underlying conditions such as diabetes or cancer (114–120). These comorbidities and age-related changes can alter immune function, affecting both the innate and adaptive immune responses. Consequently, the therapeutic effectiveness and safety profile of NMs may vary widely across these groups. Most preclinical studies investigating the anti-sepsis effects of NMs employ healthy adult animal models, which do not adequately represent the clinical diversity encountered in practice (121–123). This discrepancy inevitably leads to a significant gap between experimental outcomes and real-world applicability. To bridge this gap, it is essential to incorporate diverse animal models that mimic the immunological states of different patient populations. Moreover, personalized medicine strategies should be considered to tailor NM treatments according to individual host characteristics, thereby optimizing therapeutic efficacy and minimizing adverse effects.
Lastly, it must be acknowledged that environmental pollution, both extracorporeal and intracorporeal, could significantly impact NMs. Before entering the host body, numerous potential pollutants present in the environment can easily adsorb onto the surface of NMs. For example, LPS is a heat-resistant and widely distributed potential pollutant. Current methods for its detection are easily subject to interference by various factors, making it difficult to exclude in nanomaterial experiments (124, 125). The mechanisms by which LPS modulates immune responses are complex and could exacerbate inflammation or induce immunosuppression, thereby masking the true effects of NMs. Moreover, once NMs are introduced into the host, they can rapidly interact with a myriad of biomolecules—including proteins, nucleic acids, lipids, and metabolites—to form a “protein corona” (126). This dynamic biointerface can alter the physicochemical properties and immunogenicity of NMs, potentially promoting immune imbalance within the host. Therefore, it is crucial to consider both environmental and biological factors that may influence NM behavior, and to develop rigorous purification and characterization protocols to mitigate these effects in clinical applications.
NMs for dynamic immunomodulation in sepsis: a paradigm shift in treatment
The pathogenesis of sepsis is characterized by a complex and dynamic interplay between inflammation and immunosuppression, a duality that renders simplistic anti-bacterial and anti-inflammatory approaches insufficient. Effective therapeutic intervention necessitates a nuanced strategy focused on precise immune modulation, tailored to the evolving phases of the disease. During the predominantly inflammatory phase, anti-inflammatory strategies should be prioritized. Conversely, during the subsequent immunosuppressive phase, effective immune reconstitution becomes paramount. This transition highlights the need for adaptive therapies that can dynamically respond to the shifting immunological landscape. The immunosuppressive phase of sepsis is marked by widespread immune cell dysfunction, leading to systemic immune paralysis. This includes a shift from M1 to M2 macrophage polarization, T cell exhaustion, increased regulatory T cell (Treg) counts, elevated immature neutrophil populations, enhanced dendritic cell apoptosis, and impaired natural killer (NK) cell function (45–47). While current NM-based therapies for sepsis largely focus on anti-bacterial and anti-inflammatory effects, their substantial potential for immune reconstitution remains largely unexplored. The ability of NMs to specifically target and modulate diverse immune cell subsets, a capability extensively demonstrated in the field of oncology, suggests a promising therapeutic strategy for sepsis treatment. Integrating the established immune-activating properties of NMs, well-characterized in anti-cancer applications (127–130), with their inherent anti-inflammatory capabilities, presents a transformative therapeutic paradigm for sepsis management. As depicted in Figure 1, this strategy envisions a dynamic therapeutic shift between anti-inflammatory and immune-reconstitutive modalities, adapting to the evolving needs of the patient throughout the course of the disease. However, realizing this vision requires the development of robust, real-time monitoring tools capable of accurately assessing the individual patient’s disease stage and immunological profile. Furthermore, comprehensive safety assessments, meticulously evaluating potential toxicities and accounting for inter-individual variability based on age, comorbidities, and genetic background, are critical for the successful clinical translation of such NMs. This rigorous approach will be essential to ensure both efficacy and safety in this complex disease setting.
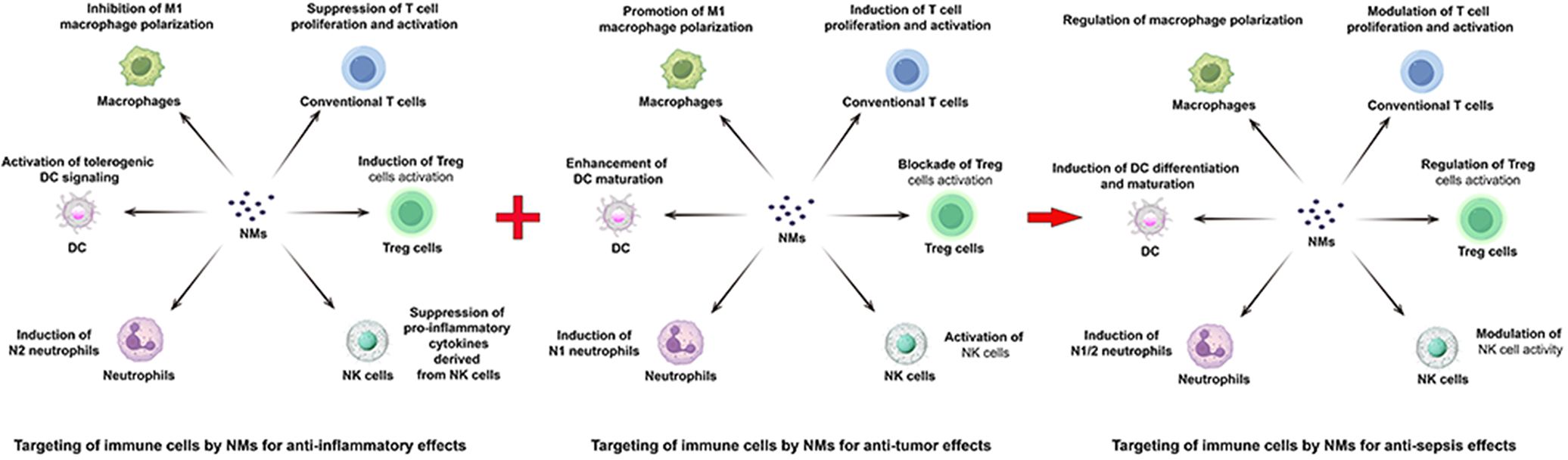
Figure 1. Mechanistic representation of NM-mediated targeted immunomodulation in sepsis. NMs leverage their combined anti-inflammatory and immune-activating properties, mirroring strategies employed in tumor, to address the biphasic nature of sepsis. In the early inflammatory phase, NMs suppress inflammation. As the disease progresses into the immunosuppressive phase, NMs promote immune reconstitution. This dynamic interplay, depicted centrally, represents a novel therapeutic paradigm for sepsis treatment, integrating the established anti-inflammatory and immune-reactivating capabilities of NMs for targeted immune cell modulation.
Conclusion and prospects
In summary, despite the tremendous potential of NMs in sepsis treatment, current application strategies primarily focus on suppressing hyperinflammation. This limited approach may neglect the modulation of the immunosuppressive phase of sepsis, potentially exacerbating the disease course. To overcome the existing limitations of NMs in sepsis treatment, we anticipate the following trends in future developments. Firstly, given the significant differences among sepsis patients in terms of infection source, degree and stage of immune response, age, gender, and comorbidities, the importance of personalized treatment should be emphasized. The design and application of future NM treatment strategies need to consider each patient’s specific status and needs, achieving a “precision medicine” approach. Secondly, the disease course of sepsis typically includes an excessive inflammatory response phase followed by an immunosuppressive phase. Therefore, an ideal treatment strategy should be able to adaptively respond to these two phases, exerting anti-inflammatory effects during hyperinflammation and activating immune responses to restore immune balance during immunosuppression. Future research on NMs may focus on developing nanotherapeutic systems with this “smart” response capability. Lastly, the precise detection of molecular biomarkers by NMs constitutes a unique advantage of these materials and a crucial step towards personalized treatment. By integrating diagnosis, treatment, and monitoring into a single nano-platform, it is possible to track a patient’s immunopathological status in real time and adjust treatment strategies promptly. This integrated diagnostic-therapeutic nano-system not only provides more precise and efficient treatment plans for patients but also helps evaluate treatment effects, which is crucial for controlling the development and prognosis of sepsis. Overall, NMs offer many new perspectives and tools for sepsis treatment, warranting further exploration and development.
Author contributions
ZW: Writing – original draft. PW: Writing – review & editing.
Funding
The authors declare financial support was received for the research, authorship, and/or publication of this article. This work was supported by the Science and Technology Support Program of Guizhou Province ((2020)4Y191).
Acknowledgments
The authors would like to express their gratitude to Figdraw (www.figdraw.com) developed by HOME for Researchers (www.home-for-researchers.com) for the assistance for the NM-mediated targeted immunomodulation illustration (ID No. IOYTOeaabe).
Conflict of interest
The authors declare that the research was conducted in the absence of any commercial or financial relationships that could be construed as a potential conflict of interest.
Publisher’s note
All claims expressed in this article are solely those of the authors and do not necessarily represent those of their affiliated organizations, or those of the publisher, the editors and the reviewers. Any product that may be evaluated in this article, or claim that may be made by its manufacturer, is not guaranteed or endorsed by the publisher.
References
1. Singer M, Deutschman CS, Seymour CW, Shankar-Hari M, Annane D, Bauer M, et al. The third international consensus definitions for sepsis and septic shock (Sepsis-3). JAMA. (2016) 315:801–10. doi: 10.1001/jama.2016.0287
2. Fleischmann C, Scherag A, Adhikari NK, Hartog CS, Tsaganos T, Schlattmann P, et al. Assessment of global incidence and mortality of hospital-treated sepsis. current estimates and limitations. Am J Respir Crit Care Med. (2016) 193:259–72. doi: 10.1164/rccm.201504-0781OC
3. Coopersmith CM, De Backer D, Deutschman CS, Ferrer R, Lat I, MaChado FR, et al. Surviving sepsis campaign: research priorities for sepsis and septic shock. Crit Care Med. (2018) 46:1400–26. doi: 10.1007/s00134-018-5175-z
4. Seymour CW, Kennedy JN, Wang S, Chang CH, Elliott CF, Xu Z, et al. Derivation, validation, and potential treatment implications of novel clinical phenotypes for sepsis. JAMA. (2019) 321:2003–17. doi: 10.1001/jama.2019.5791
5. Gavelli F, Castello LM, Avanzi GC. Management of sepsis and septic shock in the emergency department. Intern Emerg Med. (2021) 16:1649–61. doi: 10.1007/s11739-021-02735-7
6. Rudd KE, Johnson SC, Agesa KM, Shackelford KA, Tsoi D, Kievlan DR, et al. Global, regional, and national sepsis incidence and mortality, 1990–2017: analysis for the Global Burden of Disease Study. Lancet. (2020) 395:200–11. doi: 10.1016/S0140-6736(19)32989-7
7. Sterling SA, Miller WR, Pryor J, Puskarich MA, Jones AE. The impact of timing of antibiotics on outcomes in severe sepsis and septic shock: a systematic review and meta-analysis. Crit Care Med. (2015) 43:1907–15. doi: 10.1097/CCM.0000000000001142
8. Im Y, Kang D, Ko RE, Lee YJ, Lim SY, Park S, et al. Time-to-antibiotics and clinical outcomes in patients with sepsis and septic shock: a prospective nationwide multicenter cohort study. Crit Care. (2022) 26:19. doi: 10.1186/s13054-021-03883-0
9. Ruddel H, Thomas-Ruddel DO, Reinhart K, Bach F, Gerlach H, Lindner M, et al. Adverse effects of delayed antimicrobial treatment and surgical source control in adults with sepsis: results of a planned secondary analysis of a cluster-randomized controlled trial. Crit Care. (2022) 26:51. doi: 10.1186/s13054-022-03901-9
10. Vincent JL. Fluid management in the critically ill. Kidney Int. (2019) 96:52–7. doi: 10.1016/j.kint.2018.11.047
11. Evans L, Rhodes A, Alhazzani W, Antonelli M, Coopersmith CM, French C, et al. Surviving sepsis campaign: international guidelines for management of sepsis and septic shock 2021. Intensive Care Med. (2021) 47:1181–247. doi: 10.1007/s00134-021-06506-y
12. Chowdhury AH, Cox EF, Francis ST, Lobo DN. A randomized, controlled, double-blind crossover study on the effects of 2-L infusions of 0.9% saline and plasma-lyte(R) 148 on renal blood flow velocity and renal cortical tissue perfusion in healthy volunteers. Ann Surg. (2012) 256:18–24. doi: 10.1097/SLA.0b013e318256be72
13. Cariou A, Vinsonneau C, Dhainaut JF. Adjunctive therapies in sepsis: an evidence-based review. Crit Care Med. (2004) 32:S562–70. doi: 10.1097/01.ccm.0000142910.01076.a5
14. Martin-Loeches I, Levy MM, Artigas A. Management of severe sepsis: advances, challenges, and current status. Drug Des Devel Ther. (2015) 9:2079–88. doi: 10.2147/DDDT.S78757
15. Morton B, Stolbrink M, Kagima W, Rylance J, Mortimer K. The early recognition and management of sepsis in sub-saharan african adults: A systematic review and meta-analysis. Int J Environ Res Public Health. (2018) 15:2017. doi: 10.3390/ijerph15092017
16. de la Fuente-Nunez C, Cesaro A, Hancock REW. Antibiotic failure: Beyond antimicrobial resistance. Drug Resist Update. (2023) 71:101012. doi: 10.1016/j.drup.2023.101012
17. Einav S, Leone M, Martin-Loeches I. Sepsis and antibiotics: When should we deploy a parachute? Int J Antimicrob Agents. (2023) 61:106732. doi: 10.1016/j.ijantimicag.2023.106732
18. Sanz Codina M, Zeitlinger M. Clinical pharmacokinetics of antimicrobials in obese patients: A practical approach for dose adjustment. Clin Pharmacokinet. (2022) 61:593–617. doi: 10.1007/s40262-021-01102-1
19. Delano MJ, Ward PA. Sepsis-induced immune dysfunction: can immune therapies reduce mortality? J Clin Invest. (2016) 126:23–31. doi: 10.1172/JCI82224
20. Santamaria A. Historical overview of nanotechnology and nanotoxicology. Methods Mol Biol. (2012) 926:1–12. doi: 10.1007/978-1-62703-002-1_1
21. Bayda S, Adeel M, Tuccinardi T, Cordani M, Rizzolio F. The history of nanoscience and nanotechnology: From chemical-physical applications to nanomedicine. Molecules. (2020) 25:112. doi: 10.3390/molecules25010112
22. Pant A, Mackraj I, Govender T. Advances in sepsis diagnosis and management: A paradigm shift towards nanotechnology. J BioMed Sci. (2021) 28:6. doi: 10.1186/s12929-020-00702-6
23. Shen Y, Zhang Y, Gao ZF, Ye Y, Wu Q, Chen HY, et al. Recent advances in nanotechnology for simultaneous identification of multiple pathogenic bacteria. Nano Today. (2021) 38:101121. doi: 10.1016/j.nantod.2021.101121
24. Chen Y, Gao Y, Chen Y, Liu L, Mo A, Peng Q. Nanomaterials-based photothermal therapy and its potentials in antibacterial treatment. J Control Release. (2020) 328:251–62. doi: 10.1016/j.jconrel.2020.08.055
25. Hotchkiss RS, Monneret G, Payen D. Sepsis-induced immunosuppression: from cellular dysfunctions to immunotherapy. Nat Rev Immunol. (2013) 13:862–74. doi: 10.1038/nri3552
26. Xiao W, Mindrinos MN, Seok J, Cuschieri J, Cuenca AG, Gao H, et al. A genomic storm in critically injured humans. J Exp Med. (2011) 208:2581–90. doi: 10.1084/jem.20111354
27. Boomer JS, To K, Chang KC, Takasu O, Osborne DF, Walton AH, et al. Immunosuppression in patients who die of sepsis and multiple organ failure. JAMA. (2011) 306:2594–605. doi: 10.1001/jama.2011.1829
28. Daviaud F, Grimaldi D, Dechartres A, Charpentier J, Geri G, Marin N, et al. Timing and causes of death in septic shock. Ann Intensive Care. (2015) 5:16. doi: 10.1186/s13613-015-0058-8
29. Bone RC, Balk RA, Cerra FB, Dellinger RP, Fein AM, Knaus WA, et al. Definitions for sepsis and organ failure and guidelines for the use of innovative therapies in sepsis. The ACCP/SCCM Consensus Conference Committee. American College of Chest Physicians/Society of Critical Care Medicine. Chest. (1992) 101:1644–55. doi: 10.1378/chest.101.6.1644
30. Levy MM, Fink MP, Marshall JC, Abraham E, Angus D, Cook D, et al. 2001 SCCM/ESICM/ACCP/ATS/SIS international sepsis definitions conference. Intensive Care Med. (2003) 29:530–8. doi: 10.1007/s00134-003-1662-x
31. Shankar-Hari M, Phillips GS, Levy ML, Seymour CW, Liu VX, Deutschman CS, et al. Developing a new definition and assessing new clinical criteria for septic shock: for the third international consensus definitions for sepsis and septic shock (sepsis-3). JAMA. (2016) 315:775–87. doi: 10.1001/jama.2016.0289
32. Seymour CW, Liu VX, Iwashyna TJ, Brunkhorst FM, Rea TD, Scherag A, et al. Assessment of clinical criteria for sepsis: for the third international consensus definitions for sepsis and septic shock (sepsis-3). JAMA. (2016) 315:762–74. doi: 10.1001/jama.2016.0288
33. Rhodes A, Evans LE, Alhazzani W, Levy MM, Antonelli M, Ferrer R, et al. Surviving sepsis campaign: international guidelines for management of sepsis and septic shock: 2016. Intensive Care Med. (2017) 43:304–77. doi: 10.1007/s00134-017-4683-6
34. Akira S. Pathogen recognition by innate immunity and its signaling. Proc Jpn Acad Ser B Phys Biol Sci. (2009) 85:143–56. doi: 10.2183/pjab.85.143
35. Iwasaki A, Medzhitov R. Toll-like receptor control of the adaptive immune responses. Nat Immunol. (2004) 5:987–95. doi: 10.1038/ni1112
36. Kieser KJ, Kagan JC. Multi-receptor detection of individual bacterial products by the innate immune system. Nat Rev Immunol. (2017) 17:376–90. doi: 10.1038/nri.2017.25
37. Darden DB, Kelly LS, Fenner BP, Moldawer LL, Mohr AM, Efron PA. Dysregulated immunity and immunotherapy after sepsis. J Clin Med. (2021) 10:1742. doi: 10.3390/jcm10081742
38. Huber-Lang M, Lambris JD, Ward PA. Innate immune responses to trauma. Nat Immunol. (2018) 19:327–41. doi: 10.1038/s41590-018-0064-8
39. Kany S, Vollrath JT, Relja B. Cytokines in inflammatory disease. Int J Mol Sci. (2019) 20:6008. doi: 10.3390/ijms20236008
40. Wiersinga WJ, Leopold SJ, Cranendonk DR, van der Poll T. Host innate immune responses to sepsis. Virulence. (2014) 5:36–44. doi: 10.4161/viru.25436
41. Fattahi F, Kalbitz M, Malan EA, Abe E, Jajou L, Huber-Lang MS, et al. Complement-induced activation of MAPKs and Akt during sepsis: role in cardiac dysfunction. FASEB J. (2017) 31:4129–39. doi: 10.1096/fj.201700140R
42. Keshari RS, Silasi R, Popescu NI, Patel MM, Chaaban H, Lupu C, et al. Escherichia coliInhibition of complement C5 protects against organ failure and reduces mortality in a baboon model of sepsis. Proc Natl Acad Sci. (2017) 114:E6390–9. doi: 10.1073/pnas.1706818114
43. Levi M, van der Poll T. Coagulation and sepsis. Thromb Res. (2017) 149:38–44. doi: 10.1016/j.thromres.2016.11.007
44. Iba T, Levi M, Levy JH. Sepsis-induced coagulopathy and disseminated intravascular coagulation. Semin Thromb Hemost. (2020) 46:89–95. doi: 10.1055/s-0039-1694995
45. O’Neill LA, Kishton RJ, Rathmell J. A guide to immunometabolism for immunologists. Nat Rev Immunol. (2016) 16:553–65. doi: 10.1038/nri.2016.70
46. Pearce EL, Pearce EJ. Metabolic pathways in immune cell activation and quiescence. Immunity. (2013) 38:633–43. doi: 10.1016/j.immuni.2013.04.005
47. Weinberg SE, Sena LA, Chandel NS. Mitochondria in the regulation of innate and adaptive immunity. Immunity. (2015) 42:406–17. doi: 10.1016/j.immuni.2015.02.002
48. Gentile LF, Cuenca AG, Efron PA, Ang D, Bihorac A, McKinley BA, et al. Persistent inflammation and immunosuppression: A common syndrome and new horizon for surgical intensive care. J Trauma Acute Care Surg. (2012) 72:1491–501. doi: 10.1097/TA.0b013e318256e000
49. van der Poll T, van de Veerdonk FL, Scicluna BP, Netea MG. The immunopathology of sepsis and potential therapeutic targets. Nat Rev Immunol. (2017) 17:407–20. doi: 10.1038/nri.2017.36
50. Haroon HB, Hunter AC, Farhangrazi ZS, Moghimi SM. A brief history of long circulating nanoparticles. Adv Drug Delivery Rev. (2022) 188:114396. doi: 10.1016/j.addr.2022.114396
51. Bhise K, Sau S, Kebriaei R, Rice SA, Stamper KC, Alsaab HO, et al. Liposomal vancomycin as an alternative therapy against methicillin-resistant Staphylococcus aureus. Materials. (2018) 11:1245. doi: 10.3390/ma11071245
52. Filipczak N, Pan J, Yalamarty SSK, Torchilin VP. Recent advancements in liposome technology. Adv Drug Delivery Rev. (2020) 156:4–22. doi: 10.1016/j.addr.2020.06.022
53. Jeong M, Lee Y, Park J, Jung H, Lee H. Lipid nanoparticles (LNPs) for in vivo RNA delivery and their breakthrough technology for future applications. Adv Drug Delivery Rev. (2023) 200:114990. doi: 10.1016/j.addr.2023.114990
54. Su M, Yang B, Xi M, Qiang C, Yin Z. Therapeutic effect of pH-responsive dexamethasone prodrug nanoparticles on acute lung injury. J Drug Delivery Sci Technol. (2021) 66:102738. doi: 10.1016/j.jddst.2021.102738
55. Yu Z, Ma L, Ye S, Li G, Zhang M. Construction of an environmentally friendly octenylsuccinic anhydride modified pH-sensitive chitosan nanoparticle drug delivery system to alleviate inflammation and oxidative stress. Carbohydr Polym. (2020) 236:115972. doi: 10.1016/j.carbpol.2020.115972
56. Mejías JC, Roy K. In-vitro and in-vivo characterization of a multi-stage enzyme-responsive nanoparticle-in-microgel pulmonary drug delivery system. J Control Release. (2019) 316:393–403. doi: 10.1016/j.jconrel.2019.09.012
57. Mejías JC, Forrest OA, Margaroli C, Frey Rubio DA, Viera L, Li J, et al. Neutrophil-targeted, protease-activated pulmonary drug delivery blocks airway and systemic inflammation. JCI Insight. (2019) 4:e131468. doi: 10.1172/jci.insight.131468
58. Zhai Z, Ouyang W, Yao Y, Zhang Y, Zhang H, Xu F, et al. Dexamethasone-loaded ROS-responsive poly(thioketal) nanoparticles suppress inflammation and oxidative stress of acute lung injury. Bioact Mater. (2022) 14:430–42. doi: 10.1016/j.bioactmat.2022.01.047
59. Muhammad W, Zhu J, Zhai Z, Xie J, Zhou J, Feng X, et al. ROS-responsive polymer nanoparticles with enhanced loading of dexamethasone effectively modulate the lung injury microenvironment. Acta Biomater. (2022) 148:258–70. doi: 10.1016/j.actbio.2022.06.024
60. Darabpour E, Kashef N, Mashayekhan S. Chitosan nanoparticles enhance the efficiency of methylene blue-mediated antimicrobial photodynamic inactivation of bacterial biofilms: An in vitro study. Photodiagn Photodyn Ther. (2016) 14:211–7. doi: 10.1016/j.pdpdt.2016.04.009
61. Bose RJC, Tharmalingam N, Garcia Marques FJ, Sukumar UK, Natarajan A, Zeng Y, et al. Reconstructed apoptotic bodies as targeted “nano decoys” to treat intracellular bacterial infections within macrophages and cancer cells. ACS Nano. (2020) 14:5818–35. doi: 10.1021/acsnano.0c00921
62. Makabenta JMV, Nabawy A, Li CH, Schmidt-Malan S, Patel R, Rotello VM. Nanomaterial-based therapeutics for antibiotic-resistant bacterial infections. Nat Rev Microbiol. (2021) 19:23–36. doi: 10.1038/s41579-020-0420-1
63. Gao W, Zhang L. Nanomaterials arising amid antibiotic resistance. Nat Rev Microbiol. (2021) 19:5–6. doi: 10.1038/s41579-020-00469-5
64. Gomaa EZ. Silver nanoparticles as an antimicrobial agent: A case study on Staphylococcus aureus and Escherichia coli as models for Gram-positive and Gram-negative bacteria. J Gen Appl Microbiol. (2017) 63:36–43. doi: 10.2323/jgam.2016.07.004
65. Abalkhil TA, Alharbi SA, Salmen SH, Wainwright M. Bactericidal activity of biosynthesized silver nanoparticles against human pathogenic bacteria. Biotechnol Biotechnol Equip. (2017) 31:411–7. doi: 10.1080/13102818.2016.1267594
66. Gurunathan S, Han JW, Kwon DN, Kim JH. Enhanced antibacterial and anti-biofilm activities of silver nanoparticles against Gram-negative and Gram-positive bacteria. Nanoscale Res Lett. (2014) 9:1–17. doi: 10.1186/1556-276X-9-373
67. Das B, Dash SK, Mandal D, Ghosh T, Chattopadhyay S, Tripathy S, et al. Green synthesized silver nanoparticles destroy multidrug resistant bacteria via reactive oxygen species mediated membrane damage. Arab J Chem. (2017) 10:862–76. doi: 10.1016/j.arabjc.2015.08.008
68. Hsueh YH, Lin KS, Ke WJ, Hsieh CT, Chiang CL, Tzou DY, et al. The antimicrobial properties of silver nanoparticles in Bacillus subtilis are mediated by released Ag+ ions. PloS One. (2015) 10:e0144306. doi: 10.1371/journal.pone.0144306
69. Bondarenko O, Ivask A, Käkinen A, Kurvet I. Kahru A. Particle-cell contact enhances antibacterial activity of silver nanoparticles. PloS One. (2013) 8:e64060. doi: 10.1371/journal.pone.0064060
70. Timoszyk A, Grochowalska R. Mechanism and antibacterial activity of gold nanoparticles (AuNPs) functionalized with natural compounds from plants. Pharmaceutics. (2022) 14:2599. doi: 10.3390/pharmaceutics14122599
71. Wei Y, Wang J, Wu S, Zhou R, Zhang K, Zhang Z, et al. Nanomaterial-based zinc ion interference therapy to combat bacterial infections. Front Immunol. (2022) 13:899992. doi: 10.3389/fimmu.2022.899992
72. de Toledo LAS, Rosseto HC, Bruschi ML. Iron oxide magnetic nanoparticles as antimicrobials for therapeutics. Pharm Dev Technol. (2017) 23:316–23. doi: 10.1080/10837450.2017.1337793
73. Jabalera Y, Montalban-Lopez M, Vinuesa-Rodriguez JJ, Iglesias GR, Maqueda M, Jimenez-Lopez C. Antibacterial directed chemotherapy using AS-48 peptide immobilized on biomimetic magnetic nanoparticles combined with magnetic hyperthermia. Int J Biol Macromol. (2021) 189:206–13. doi: 10.1016/j.ijbiomac.2021.08.110
74. Satishkumar R, Vertegel AA. Antibody-directed targeting of lysostaphin adsorbed onto polylactide nanoparticles increases its antimicrobial activity against S. aureus in vitro. Nanotechnology. (2011) 22:505103. doi: 10.1088/0957-4484/22/50/505103
75. Ontiveros-Robles JA, Villanueva-Flores F, Juarez-Moreno K, Simakov A, Vazquez-Duhalt R. Antibody-functionalized copper oxide nanoparticles with targeted antibacterial activity. ChemistryOpen. (2023) 12:e202200241. doi: 10.1002/open.202200241
76. Kumar S, Saxena J, Srivastava VK, Kaushik S, Singh H, Abo-El-Sooud K, et al. The interplay of oxidative stress and ROS scavenging: Antioxidants as a therapeutic potential in sepsis. Vaccines. (2022) 10:1575. doi: 10.3390/vaccines10101575
77. Yim D, Lee DE, So Y, Choi C, Son W, Jang K, et al. Sustainable nanosheet antioxidants for sepsis therapy via scavenging intracellular reactive oxygen and nitrogen species. ACS Nano. (2020) 14:10324–36. doi: 10.1021/acsnano.0c03807
78. Rajendrakumar SK, Revuri V, Samidurai M, Mohapatra A, Lee JH, Ganesan P, et al. Peroxidase-mimicking nanoassembly mitigates lipopolysaccharide-induced endotoxemia and cognitive damage in the brain by impeding inflammatory signaling in macrophages. Nano Lett. (2018) 18:6417–26. doi: 10.1021/acs.nanolett.8b02785
79. Gabarin RS, Li M, Zimmel PA, Marshall JC, Li Y, Zhang H. Intracellular and extracellular lipopolysaccharide signaling in sepsis: Avenues for novel therapeutic strategies. J Innate Immun. (2021) 13:323–32. doi: 10.1159/000515740
80. Gollomp K, Sarkar A, Harikumar S, Seeholzer SH, Arepally GM, Hudock K, et al. Fc-modified HIT-like monoclonal antibody as a novel treatment for sepsis. Blood. (2020) 135:743–54. doi: 10.1182/blood.2019002329
81. Dawulieti J, Sun M, Zhao Y, Shao D, Yan H, Lao YH, et al. Treatment of severe sepsis with nanoparticulate cell-free DNA scavengers. Sci Adv. (2020) 6:eaay7148. doi: 10.1126/sciadv.aay7148
82. Yuk SA, Kim H, Abutaleb NS, Dieterly AM, Taha MS, Tsifansky MD, et al. Nanocapsules modify membrane interaction of polymyxin B to enable safe systemic therapy of Gram-negative sepsis. Sci Adv. (2021) 7:eabj1577. doi: 10.1126/sciadv.abj1577
83. Song C, Xu J, Gao C, Zhang W, Fang X, Shang Y. Nanomaterials targeting macrophages in sepsis: A promising approach for sepsis management. Front Immunol. (2021) 13:1026173. doi: 10.3389/fimmu.2022.1026173
84. Chen G, Yang F, Fan S, Jin H, Liao K, Li X, et al. Immunomodulatory roles of selenium nanoparticles: Novel arts for potential immunotherapy strategy development. Front Immunol. (2022) 13:956181. doi: 10.3389/fimmu.2022.956181
85. Wei B, Ma Y. Synergistic effect of GF9 and streptomycin on relieving gram-negative bacteria-induced sepsis. Front Bioeng Biotechnol. (2022) 10:973588. doi: 10.3389/fbioe.2022.973588
86. Handa M, Sharma A, Verma RK, Shukla R. Polycaprolactone based nano-carrier for co-administration of moxifloxacin and rutin and its in-vitro evaluation for sepsis. J Drug Delivery Sci Tec. (2019) 54:101286. doi: 10.1016/j.jddst.2019.101286
87. Hou X, Zhang X, Zhao W, Zeng C, Deng B, McComb DW, et al. Vitamin lipid nanoparticles enable adoptive macrophage transfer for the treatment of multidrug-resistant bacterial sepsis. Nat Nanotechnol. (2020) 151:41–6. doi: 10.1038/s41565-019-0600-1
88. Yang Y, Ding Y, Fan B, Wang Y, Mao Z, Wang W, et al. Infammationtargeting polymeric nanoparticles deliver sparfoxacin and tacrolimus for combating acute lung sepsis. J Control Release. (2020) 321:463–74. doi: 10.1016/j.jconrel.2020.02.030
89. Zhang CY, Gao J, Wang Z. Bioresponsive nanoparticles targeted to infectious microenvironments for sepsis management. Adv Mater. (2018) 30:1803618. doi: 10.1002/adma.201803618
90. Liu Y, Li Y, Zhou C, Shi L. Recent advances and prospects in nanomaterials for bacterial sepsis management. J Mater Chem B. (2023) 11:10778–92. doi: 10.1021/acsbiomaterials.4c01385
91. Dabbah K, Perelshtein I, Gedanken A, Houri-Haddad Y, Feuerstein O. Effects of a ZnCuO-nanocoated Ti-6Al-4V surface on bacterial and host cells. Materials. (2022) 15:2514. doi: 10.3390/ma15072514
92. Ali SS, Morsy R, El-Zawawy NA, Fareed MF, Bedaiwy MY. Synthesized zinc peroxide nanoparticles (ZnO2-NPs): a novel antimicrobial, anti-elastase, anti-keratinase, and anti-inflammatory approach toward polymicrobial burn wounds. Int J Nanomedicine. (2017) 12:6059–73. doi: 10.2147/IJN.S141201
93. Nedeva C, Menassa J, Puthalakath H. Sepsis: Inflammation is a necessary evil. Front Cell Dev Biol. (2019) 7:108. doi: 10.3389/fcell.2019.00108
94. Menassa J, Nedeva C, Pollock C, Puthalakath H. TLR4: The fall guy in sepsis? Cell Stress. (2020) 4:270–2. doi: 10.15698/cst2020.12.237
95. Marshall JC. Special issue: Sepsis why have clinical trials in sepsis failed? Trends Mol Med. (2014) 20:195–203. doi: 10.1016/j.molmed.2014.01.007
96. Mynasyan H. Phagocytosis and oxycytosis: two arms of human innate immunity. Immunol Res. (2018) 66:I271–80. doi: 10.1007/s12026-018-8988-5
97. Minasyan H. Mechanisms and pathways for the clearance of bacteria from blood circulation in health and disease. Pathophysiology. (2016) 23:61–6. doi: 10.1016/j.pathophys.2016.03.001
98. Lamontagne F, Masse MH, Menard J, Sprague S, Pinto R, Heyland DK, et al. Intravenous vitamin C in adults with sepsis in the intensive care unit. N Engl J Med. (2021) 386:2387–98. doi: 10.1056/NEJMoa2200644
99. Fisher CJ, Agosti JM, Opal SM, Lowry SF, Balk RA, Sadoff JC, et al. Treatment of septic shock with the tumor necrosis factor receptor: Fc fusion protein. The soluble TNF receptor sepsis study group. N Engl J Med. (1996) 334:1697–702. doi: 10.1056/NEJM199606273342603
100. Bernard GR, Vincent JL, Laterre PF, LaRosa SP, Dhainaut JF, Lopez-Rodriguez A, et al. Efficacy and safety of recombinant human activated protein C for severe sepsis. N Engl J Med. (2001) 344:699–709. doi: 10.1056/NEJM200103083441001
101. Opal SM, Laterre PF, Francois B, LaRosa SP, Angus DC, Mira JP, et al. Effect of eritoran, an antagonist of MD2-TLR4, on mortality in patients with severe sepsis: the ACCESS randomized trial. JAMA. (2013) 309:1154–62. doi: 10.1001/jama.2013.2194
102. Bone RC, Balk RA, Fein AM, Perl TM, Wenzel RP, Reines HD, et al. A second large controlled clinical study of E5, a monoclonal antibody to endotoxin: results of a prospective, multicenter, randomized, controlled trial. Crit Care Med. (1995) 23(6):994–1006. doi: 10.1097/00003246-199506000-00003
103. Chapekar MS. Tissue engineering: challenges and opportunities. J BioMed Mater Res. (2000) 53:617–20. doi: 10.1002/1097-4636(2000)53:6<617::AID-JBM1>3.0.CO;2-C
104. Padmanabhan J, Kyriakides TR. Nanomaterials, inflammation, and tissue engineering. Wiley Interdiscip Rev Nanomed Nanobiotechnol. (2015) 7:355–70. doi: 10.1002/wnan.1320
105. Erdem JS, Závodná T, Ervik TK, Skare Ø, Hron T, Anmarkrud KH, et al. High aspect ratio nanomaterial-induced macrophage polarization is mediated by changes in miRNA levels. Front Immunol. (2023) 14:1111123. doi: 10.3389/fimmu.2023.1111123
106. Lewinski N, Colvin V, Drezek R. Cytotoxicity of nanoparticles. Small. (2008) 4:26–49. doi: 10.1002/smll.200700595
107. Wang ZY, Wang M, Zeng X, Yue XP, Wei P. Nanomaterial-induced pyroptosis: A cell type-specific perspective. Front Cell Dev Biol. (2024) 11:1322305. doi: 10.3389/fcell.2023.1322305
108. Aljabali AA, Obeid MA, Bashatwah RM, Serrano-Aroca Á, Mishra V, Mishra Y, et al. Nanomaterials and their impact on the immune system. Int J Mol Sci. (2023) 24:2008. doi: 10.3390/ijms24032008
109. Prescott HC, Dickson RP, Rogers MAM, Langa KM, Iwashyna TJ. Hospitalization type and subsequent severe sepsis. Am J Respir Crit Care Med. (2015) 192:581–8. doi: 10.1164/rccm.201503-0483OC
110. Baggs J, Jernigan JA, Halpin AL, Epstein L, Hatfield KM, McDonald LC. Risk of subsequent sepsis within 90 days after a hospital stay by type of antibiotic exposure. Clin Infect Dis. (2018) 66:1004–12. doi: 10.1093/cid/cix947
111. Ni J, Wu GD, Albenberg L, Tomov VT. Gut microbiota and IBD: causation or correlation? Nat Rev Gastroenterol Hepatol. (2017) 14:573–84. doi: 10.1038/nrgastro.2017.88
112. Shan Y, Lee M, Chang EB. The gut microbiome and inflammatory bowel diseases. Annu Rev Med. (2022) 73:455–68. doi: 10.1146/annurev-med-042320-021020
113. Klingensmith NJ, Coopersmith CM. The gut microbiome in sepsis. Surg Infect. (2023) 24:250–7. doi: 10.1089/sur.2022.420
114. Martin GS, Mannino DM, Moss M. The effect of age on the development and outcome of adult sepsis. Crit Care Med. (2006) 34:15–21. doi: 10.1097/01.ccm.0000194535.82812.ba
115. Nasa P, Juneja D, Singh O. Severe sepsis and septic shock in the elderly: an overview. World J Crit Care Med. (2012) 1:23–30. doi: 10.5492/wjccm.v1.i1.23
116. Fox AC, Robertson CM, Belt B, Clark AT, Chang KC, Leathersich AM, et al. Cancer causes increased mortality and is associated with altered apoptosis in murine sepsis. Crit Care Med. (2010) 38:886–93. doi: 10.1097/CCM.0b013e3181c8fdb1
117. Hensley MK, Donnelly JP, Carlton EF, Prescott HC. Epidemiology and outcomes of cancer-related versus non-cancer-related sepsis hospitalizations. Crit Care Med. (2019) 47:1310–6. doi: 10.1097/CCM.0000000000003896
118. Carey IM, Critchley JA, DeWilde S, Harris T, Hosking FJ, Cook DG. Risk of infection in type 1 and type 2 diabetes compared with the general population: a matched cohort study. Diabetes Care. (2018) 41:513–21. doi: 10.2337/dc17-2131
119. Frydrych LM, Fattahi F, He K, Ward PA, Delano MJ. Diabetes and sepsis: risk, recurrence, and ruination. Front Endocrinol. (2017) 8:271. doi: 10.3389/fendo.2017.00271
120. Trevelin SC, Carlos D, Beretta M, da Silva JS, Cunha FQ. Diabetes mellitus and sepsis: a challenging association. Shock. (2017) 47:276–87. doi: 10.1097/SHK.0000000000000778
121. Doi K, Leelahavanichkul A, Yuen PST, Star RA. Animal models of sepsis and sepsis-induced kidney injury. J Clin Invest. (2009) 119:2868–78. doi: 10.1172/jci39421
122. Buras JA, Holzmann B, Sitkovsky M. Animal models of sepsis: setting the stage. Nat Rev Drug Discovery. (2005) 4:854–65. doi: 10.1038/nrd1854
123. Nemzek JA, Hugunin KM, Opp MR. Modeling sepsis in the laboratory: merging sound science with animal well-being. Comp Med. (2008) 58:120–8. doi: 10.1111/j.1751-0813.2008.00260.x
124. Li Y, Italiani P, Casals E, Tran N, Puntes VF. Optimising the use of commercial LAL assays for the analysis of endotoxin contamination in metal colloids and metal oxide nanoparticles. Nanotoxicol. (2015) 9:462–73. doi: 10.3109/17435390.2014.948090
125. Schwarz H, Gornicec J, Neuper T, Parigiani MA, Wallner M, Duschl A, et al. Biological activity of masked endotoxin. Sci Rep. (2017) 7:44750. doi: 10.1038/srep44750
126. Saptarshi SR, Duschl A, Lopata AL. Interaction of nanoparticles with proteins: relation to bio-reactivity of the nanoparticle. J Nanobiotechnol. (2013) 11:26. doi: 10.1186/1477-3155-11-26
127. Lee NK, Kim SN, Park CG. Immune cell targeting nanoparticles: a review. Biomater Res. (2021) 25:44. doi: 10.1186/s40824-021-00246-2
128. Iscaro A, Howard NF, Muthana M. Nanoparticles: Properties and applications in cancer immunotherapy. Curr Pharm Des. (2019) 25:1962–79. doi: 10.2174/1381612825666190708214240
129. Qi FL, Wang MF, Li BZ, Lu ZF, Nie GJ, Li SP. Reversal of the immunosuppressive tumor microenvironment by nanoparticle-based activation of immune-associated cells. Acta Pharmacol Sin. (2020) 41:895–901. doi: 10.1038/s41401-020-0423-5
Keywords: nanomaterials (A), inflammation, immunosuppression, sepsis, bacteria
Citation: Wang Z and Wei P (2024) Beyond anti-inflammatory strategies: addressing immunosuppression with nanomaterials in sepsis treatment. Front. Immunol. 15:1500734. doi: 10.3389/fimmu.2024.1500734
Received: 23 September 2024; Accepted: 28 October 2024;
Published: 18 November 2024.
Edited by:
Lorella Pascolo, Institute for Maternal and Child Health Burlo Garofolo (IRCCS), ItalyReviewed by:
Yu-Fon Chen, National Taitung University, TaiwanCopyright © 2024 Wang and Wei. This is an open-access article distributed under the terms of the Creative Commons Attribution License (CC BY). The use, distribution or reproduction in other forums is permitted, provided the original author(s) and the copyright owner(s) are credited and that the original publication in this journal is cited, in accordance with accepted academic practice. No use, distribution or reproduction is permitted which does not comply with these terms.
*Correspondence: Zhiyong Wang, d2FuZ3poaXlvbmdAem11emguZWR1LmNu; Pei Wei, d2VpcGVpQHptdXpoLmVkdS5jbg==