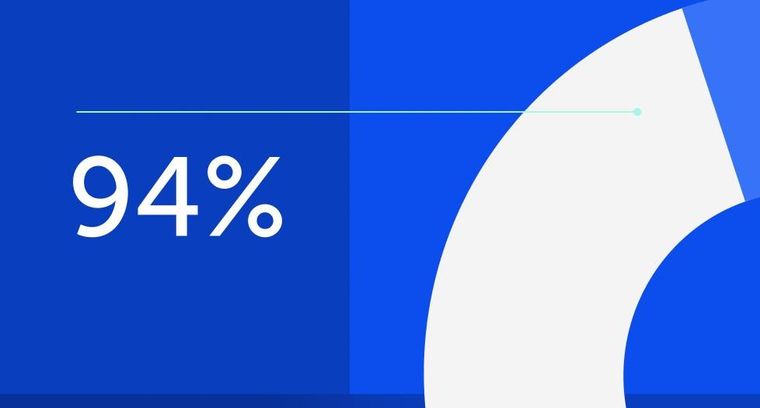
94% of researchers rate our articles as excellent or good
Learn more about the work of our research integrity team to safeguard the quality of each article we publish.
Find out more
REVIEW article
Front. Immunol., 15 November 2024
Sec. Cancer Immunity and Immunotherapy
Volume 15 - 2024 | https://doi.org/10.3389/fimmu.2024.1499700
This article is part of the Research TopicDecoding Tumor Plasticity: Integrative Analysis of Epigenetic Regulation and Microenvironmental AdaptationView all 11 articles
Retinoblastoma is the most common type of eye tumor in infants and children. Current treatments for retinoblastoma include intravenous chemotherapy, intra-arterial chemotherapy, intravitreal chemotherapy, cryotherapy, radiotherapy, and surgery. However, these treatments come accompanied by adverse effects such as the toxic side effects of chemotherapeutic drugs, post-operative complications including blindness after surgery, or other complications caused by radiotherapy. Immunotherapy is more promising for its low toxicity on normal cells and effectively improves the quality of life of patients. Disialoganglioside (GD2), a sphingolipid expressed on the surface of retinoblastoma, is a potential therapeutic target for retinoblastoma. We summarized immunotherapeutic approaches for both preclinical studies and clinical trials of GD2. An anti-GD2 monoclonal antibody (Dinutuximab), which has been approved for the treatment of high-risk neuroblastomas, has shown promising efficacy in improving patients’ prognosis. Additionally, chimeric antigen receptors (CAR)-T therapy, GD2 vaccines and nanoparticles are also potential therapeutics. Finally, we discuss the prospects and current limitations of these immunotherapeutic approaches for treating retinoblastoma, as well as how to address these problems.
Retinoblastoma (RB) is the most common type of intraocular malignancy in infancy and childhood, usually caused by a mutation in the retinoblastoma 1 (RB1) gene on chromosome 13, which occurs in one or both eyes (1). The survival rate of children with retinoblastoma varies in countries with different income levels, depending on early diagnosis, staging, and treatment options. In high-income countries, the 3-year survival rate can reach more than 99%. In contrast, in low-income countries, many children present to the clinic with disseminated lesions and some with distant metastases, resulting in a 3-year survival rate of less than 58% (2, 3). It is not satisfactory that there are essentially no treatment options available for children with disseminated retinoblastoma (4). Therefore, new therapeutic approaches are desperately needed for both intraocular and extraocular illnesses.
The blood-retinal barrier (BRB), which prevents the interchange of macromolecules between the retina and the circulatory system, is considered to keep retinoblastoma separate from blood cells, in contrast to the majority of malignancies that frequently interact with the vascular system (5, 6). Immunohistochemistry of retinoblastoma and immunocytochemical analysis of tumor-infiltrating vitreous samples detected immune cells but not in normal retinal tissue or normal vitreous samples, suggesting that destruction of the BRB by retinoblastoma cells promotes immune cell infiltration and disrupts immune homeostasis (7).
Current treatments for retinoblastoma include intravenous chemotherapy, intra-arterial chemotherapy, intravitreal chemotherapy, cryotherapy, radiotherapy, and surgery (8, 9). Intravenous chemotherapy not only controls the tumor, but also plays a role in preventing distant metastases (10). However, it is difficult to avoid complications such as alopecia and ototoxicity with intravenous chemotherapy (8, 11). In contrast, intra-arterial chemotherapy can lead to a higher concentration of locally delivered chemotherapeutic drugs and better control of ocular tumors (12). Although intra-arterial chemotherapy also results in reduced systemic complications, it causes complications localized to the eye including periorbital edema, periocular congestion, and vitreous hemorrhage (13). Intravitreal chemotherapy also directly delivers chemotherapeutic drugs to the tumor lesion, which plays a very good therapeutic effect on patients with intravitreal dissemination (8). Cryotherapy as a local treatment is usually combined with intravenous chemotherapy or intra-arterial chemotherapy. However, this local treatment can cause choroidal retinal scarring and even lead to vision loss (8). Radiation therapy is now generally considered as a treatment of last resort, which is related to its complication rate, as external radiation radiotherapy (EBRT) can cause cataracts, orbital hypoplasia, and in severe cases, secondary tumors (14). Some advanced patients must undergo surgical removal to prevent metastasis of the tumor, but postoperatively, patients may face physical effects such as postoperative hemorrhage, infection, and psychological effects such as anxiety and depression (15). With the advent of systemic chemotherapy, the use of external beam radiation therapy has been gradually relinquished. Although novel methods for delivering chemotherapeutic agents to tumor sites are now available, the toxicity associated with chemotherapy remains a significant concern. Thus, there is an urgent need to develop new targeted therapies that can preserve patients’ vision and improve their overall quality of life (16).
In recent years, targets related to RB immunotherapy include GD2 (17), PD-1 (18), B7H3 (19), EpCAM (20), SYK (21). With high density in tumor cells and limited expression in normal tissues, GD2 is ideally suited as a target for cancer therapy, and has been ranked by the US National Cancer Institute as one of the most promising anticancer therapies accordingly (22). Malignant transformations are commonly accompanied by alterations in cell surface glycosylation. GD2 has been identified as a potential serum marker for retinoblastoma since as early as 1993 (23). GD2 has served as an effective target on human retinoblastoma cell lines (17). This review focuses on ganglioside GD2 as a therapeutic target for retinoblastoma and the therapeutic approaches being investigated.
GD2 is enmeshed with its ceramide tail (fatty acid-linked sphingosine) in the cell membrane (24, 25). The sugar moiety, which is made up of galactose (Gal), N-acetylgalactosamine (GalNAc), and glucose (Glc; connected to ceramide), is exposed to the extracellular milieu and has antigenic properties that promote mutual recognition and adhesion between cells (25, 26). Gal is formed by two more sialic acid residues (N-acetylneuraminic acid, NeuAc), which also give GD2 a negative charge (25). Gangliosides first synthesize ceramide in the endoplasmic reticulum (27), which is subsequently transferred to the Golgi apparatus via ceramide transfer protein or vesicles (28, 29) and glycosylated under the action of glycosyltransferase and sialyltransferase (30). The glycosylated and sialylated ceramides are then transferred to the plasma membrane via the cytosolic action of the Golgi apparatus by the transfer of vesicles (Figure 1) (31). Ceramide binds to glucose in the presence of UDP-glucose-ceramide-glucosyltransferase, resulting in glucosylceramide (GlcCer). The GlcCer combines with galactose in the presence of lactosylceramide synthetase to produce lactosylceramide (LacCer), which then combines with salivary acid to form GM3. In the presence of GD3 synthetase, GM3 continues to bind to salivary acid to produce GD3, which then binds to N-acetylgalactosamine (GalNAc) to produce GD2 (Figure 1) (32). Sialic acids can be further O-acetylated (33), allowing the O-acetylated derivative of GD2, O-acetyl-GD2, to gain attention as a novel antigen targeting GD2-positive cancers (34). O-acetyl-GD2 is coexpressed with GD2 in a variety of tumor cells including lung carcinoma, melanoma, osteosarcoma, brain tumors, and neuroblastoma (35, 36).
Figure 1. The biosynthetic process of GD2. Ceramide (Cer) is first synthesised in the endoplasmic reticulum (ER), ① Cer transfers to the inner Golgi (cytoplasmic side) via vesicles or ceramide transfer protein (CERT), ② Cer binds to glucose to produce glucosylceramide (GlcCer), ③ GlcCer is transferred to the cytoplasmic face of the ER via FAPP2 (phosphatidylinositol 4-phosphate adaptor protein), ④ GlcCer is flipped to the luminal side of the ER by ATP-independent flippase, ⑤ GlcCer in the lumen of the ER is translocated to the Golgi via vesicles, ⑥ GlcCer binds to galactose in the Golgi to form lactosylceramide (LacCer), ⑦ LacCer binds to salivary acid (Neu5Ac) to produce GM3, ⑧ GM3 continues to bind to Neu5Ac to generate GD3, ⑨ GD3 binds to N-acetylgalactosamine (GalNAc) to produce GD2, ⑩ GD2 is transferred to the cell membrane via vesicles secreted by the Golgi apparatus.
The catabolism of GD2 begins with the formation of endosomes by endocytosis, which then fuses with lysosomes to form invaginated vesicles. The outer layer of these vesicles carries GD2, which is then degraded when exposed to lysosomal matrix glycohydrolases (37). GD2 removes one salivary acid in the presence of sialidase to form GM2, then removes N-acetylgalactosamine in the presence of hexosaminidase to form GM3 (24). The remaining salivary acid is removed in the presence of SAP-B and sialidase to form LacCer, which is subsequently degraded to ceramide in the sequential presence of β-galactosidase, β-glucosidase, and SAP-C. This is ultimately broken down into sphingomyelin base and fatty acid in the presence of ceramidase and SAP-D (24, 37).
Several glycosyltransferase (GT) genes implicated in GD2 biosynthesis (ST3GAL5, B4GALNT1, and ST8SIA1) were found to be substantially elevated in cancer stem cells (CSCs) by gene expression analysis (38, 39). GD2 expression was decreased by ST8SIA1 knockdown, which changed the phenotype from CSCs to non-CSCs, thereby inhibiting tumor formation in vivo.
Before birth, GD2 is expressed in neural and mesenchymal stem cells (40). Whereas after birth, it is expressed in the central nervous system (41), peripheral nerves (41), dermal melanocytes (41), and mesenchymal stromal cells (42, 43). However, the normal human retina does not exhibit GD2 expression (44, 45). The high expression level of GD2 is associated with reduced apoptosis and enhanced proliferation, adhesion, angiogenesis, migration and invasion of tumor cells in a variety of solid tumors, including retinoblastoma (RB) (23, 45–48), neuroblastoma (NB) (41, 49), breast cancer (BC) (38, 50), bladder cancer (BLCA) (51), small cell lung carcinoma (SCLC) (52, 53), osteosarcoma (OS) (54), ewing sarcoma (ES) (47, 55, 56) and diffuse intrinsic pontine glioma (DIPG) (57). The tumor stage and proliferation of retinoblastoma are both positively correlated with GD2 expression (7). GD2 is also detectable in the serum (23), bone marrow (48), and cerebrospinal fluid (58) of patients with extraocular dissemination.
GD2 activates receptor tyrosine kinase (RTK)-mediated signaling and engages in cell proliferation and migration, angiogenesis, and tumor metastasis (59). GD2 is directly involved in RTK activation, possibly realized by specific interaction of the oligosaccharide portion of GD2 with the RTK receptor (60). The activation of c-Met was found to be associated with GD2 (60, 61), which in turn activated the MEK/Erk and PI3K/Akt signaling pathways, resulting in enhanced cell migration, proliferation, and tumor growth (60). Moreover, carbohydrate interactions between GD2 and c-Met induce constitutive activation of c-Met even in the absence of hepatocyte growth factor (62). Additionally, GD2 is physically associated with integrins on the same cell (61, 63), which are strongly associated with focal adhesion kinase (FAK) both physically and functionally. The formation of a tertiary complex consisting of GD2, integrins, and FAK further generates, and/or maintains the malignant properties of SCLC cells by activating MAPK signaling (63). In addition, associations between GD2 and the FAK/AKT signaling pathway were found in melanoma cells, glioma cells, prostate cancer cells, triple-negative breast cancer cells, and osteosarcoma cells with high GD2 expression (24).
The expression of GD2 is increased when epithelial-mesenchymal transition (EMT) is induced (38). Studies have shown that in bladder cancer, the EMT process could be reversed upon inhibition of GD2 synthesis (51). Additionally, GD2 might have indirect impacts on the development of tumors. It has been noted to suppress human dendritic cells (64) and T-cell immune responses (24, 65), most likely via myeloid-derived suppressor cells (66) and regulatory T cells (67). Moreover, GD2-positive melanoma cells produce small extracellular vesicles (sEVs), which enable nearby GD2-negative melanoma cells to take on more malignant characteristics, such as cell proliferation, invasion and adhesion (68).
GD2 has been used as a biomarker of retinoblastoma (23, 48, 58, 69), aggressive high-grade bladder cancer (51), and a CSC-specific cell surface marker in breast cancer (38). Additionally, correlation has been verified between GD2 expression and lung cancer malignant phenotypes (70). Moreover, GD2 synthase serves as a marker for minimal disseminated disease in retinoblastoma patients (69, 71–73).
The blood-brain barrier (BBB) prevents intravenous anti-GD2 mAbs from entering the central nervous system (CNS), making GD2 an ideal target for CNS extranodal neuroectodermal tumors (74). Three mechanisms of action have been identified for anti-GD2 monoclonal antibody (mAb) against tumor cells expressing GD2 (26):
Firstly, anti-GD2 mAbs initiate phagocytosis of tumor cells by macrophages, destruction of tumor cells by natural killer (NK) cells, and granulocyte-mediated cytotoxicity (Figure 2A) (75, 76). Siglec (sialic acid-binding immunoglobulin-like lectin)-7 is an inhibitory receptor expressed on immune cells, inhibits immune cell activity through the cytoplasmic immunoreceptor tyrosine-based inhibitory motif (ITIM) domain (77). Siglec-7 is a ligand for GD2 and binds to GD2 to inhibit immune cell activity via ITIM. Anti-GD2 antibody blocks GD2 from binding to Siglec-7 so that immune cells are not suppressed.
Figure 2. Immunotherapeutic mechanisms on GD2. (A) Binding of the Fc segment of the antibody to the FcR of the effector cell (e.g. granulocyte) initiates the killing of the cell. (B) Antibodies mediate tumour cell lysis via complement-dependent cytotoxicity. (C) Antibodies directly induce cell death. (D) CAR-T cells recognize GD2 and promote cell lysis. (E) Nanoparticles recognize GD2 by antibodies and deliver cytotoxic drugs and RNA to tumour cells.
Furthermore, anti-GD2 mAbs mediate the lysis of tumor cells through complement-dependent cytotoxicity (Figure 2B) (76, 78). Besides, it was shown that cell death directly induced by anti-GD2 mAb was associated with a mitochondria-dependent pathway. Anti-GD2 mAb interacted with GD2 to cause rapid hyperpolarization of the membrane potential of mitochondria, followed by apoptotic volume decrease (AVD) and alteration of cell membrane permeability, suggesting that anti-GD2 mAb-induced cell death is characterized by apoptosis and necrosis (Figure 2C) (79). We summarize some of the studies on anti-GD2 monoclonal antibodies (Table 1).
Table 1. lists studies on the anti-GD2 monoclonal antibody collected from https://clinicaltrials.gov/.
Dinutuximab (Ch14.18/SP2/0): Dinutuximab binds specifically to GD2 and is the first GD2 monoclonal antibody licensed for the treatment of high-risk neuroblastoma (HRNB) (80). The Children’s Oncology Group (COG) conducted a large randomized trial in 2010, which revealed that immunotherapy with the addition of ch14.18, alternating with granulocyte–macrophage colony-stimulating factor(GM-CSF) and interleukin-2, significantly improved outcome as compared with standard therapy in patients with HRNB (81) with similar long-term follow-up results (82).
Dinutuximab beta: Dinutuximab beta is an analog of Dinutuximab, which has been approved for use as frontline post-consolidation therapy (81) and as a second-line drug to improve survival chances in patients with HRNB (83, 84).
Naxitamab (humanized anti-GD2 mAb, Hu3F8): Naxitamab is an FDA-approved anti-GD2 monoclonal antibody that is being used in combination with GM-CSF in children and adults with HRNB (85), and is associated with modest toxic effects, low immunogenicity, and substantial anti-neuroblastoma activity (86).
Dinutuximab binding to CD16-expressing NK-92MI cells incites strong antitumor effects on RB cell. Mechanism analysis shows antibody-dependent cell-mediated cytotoxicity (ADCC) is increased, meanwhile, the perforin-granzyme cytolytic pathway is activated by NK cells (7).
A combination of Dinutuximab and anti-CD47 antibodies results in the recruitment of tumor-associated macrophages (TAMs) to mediate robust and durable anti-tumor responses, which might expand the clinical use of anti-GD2 mAbs to other GD2+ diseases beyond NB (77). Potent synergy for the combination of Dinutuximab and anti-CD47 has been established in a xenograft mouse model of NB, where the combination eradicates tumors (77). Additionally, a similar effect was seen in small cell lung cancer, where the combination significantly reduced tumor burden and extended survival (77).
Recently, a novel IgA anti-GD2 immunotherapy (IgA3.0 ch14.18) was developed, which could replace Dinutuximab to treat HRNB since it provides similar efficiency but does not induce neuropathic pain (87). Moreover, it offers an extended half-life, and enhanced stability, and may reduce the risk of developing potential side effects, such as Berger’s disease (87).
Compared to Dinutuximab, a humanized anti-GD2 monoclonal antibody with the K322A mutation (hu14.18K322A), is effective in treating refractory or recurrent neuroblastoma, potentially reducing pain and other side effects (88). Currently, hu14.18K322A has entered phase II clinical trials and has been shown to significantly improve early response and outcomes in children with newly diagnosed HRNB (89).
To enhance anti-tumor efficacy, an immunocytokine (IC) was developed by linking interleukin (IL)-2 to the COOH terminus of a humanized anti-GD2 mAb (hu14.18-IL2) (90, 91). Recently, Nguyen, R. et al. developed two new ICs, hu14.18-IL15 and -IL21 and demonstrated their superior antibody-dependent cell-mediated cytotoxicity compared to hu14.18-IL2 in fully immunocompetent syngeneic mouse models with orthotopic NB. This ultimately led to complete tumor regression and long-term survival beyond 100 days (92). IL15 and IL21 act on both CD8+ T cells and NK cells (93). Moreover, IL15 also acts on the myeloid compartment by decreasing the recruitment of polymorphonuclear/granulocytic myeloid-derived suppressor cells to the tumor microenvironment and increasing pro-inflammatory M1-like TAMs (92).
Radiopharmaceutical therapy (RPT) is a type of radionuclide labeling associated with monoclonal antibodies. It has been shown that actinium-225 radiolabels humanized anti-GD2 mAb (hu3F8), which is specifically targeted to the tumor site by an antibody, causes an ionization event that damages the double-stranded DNA of the tumor cells (94). Hu3F8-based alpha-particle therapy has been proven to be a safe and effective approach for OS. Liatsou, I et al. demonstrated the feasibility of such therapy by treating both an orthotopic canine OS xenograft mouse model and two dogs with spontaneously occurring OS, using an alpha-particle emitter labeled Hu3F8 (94). Moreover, hu3F8 labeled with Indium-111 enables good imaging of human and canine OS patients (95).
Treatment with anti-GD2 antibodies is associated with a temporary painful neuropathy syndrome in patients (81), which could be attributed to the antibodies’ ability to bind to the GD2+ myelin sheath of nerve fibers (96, 97), complement fixation (97) and direct electrophysiologic effects on nerve fibers (98).
At least 40% of NB patients relapse despite having received anti-GD2 mAbs during upfront therapy (81, 99). Killer immunoglobulin-like receptors (KIR) could affect NK cell activity which in turn affects patients’ response to immunotherapy. Recurrent HRNB Patients with a KIR2DL2+/ligand+ genotype or a KIR3DL1+/ligand+ genotype had better survival outcome in the setting of GD2-directed immunotherapy (100), shedding light on therapy selection in the setting of relapsed and refractory disease.
More than 40% of NB patients fail to respond or develop resistance to anti-GD2 mAbs (101). Recently, two mechanisms for the development of anti-GD2 mAbs resistance have been identified. Firstly, GD2 expression is reduced when NB patients relapse (102). Adeiye A et al. found that NB patients with high expression of YAP (yes-associated protein) had high resistance to GD2 immunotherapy (103). Mechanistically, YAP transcriptionally suppresses ST8SIA1 that encodes GD3 synthase, the rate-limiting enzyme for GD2 synthesis, suggesting that YAP could serve as both a mediator and potential biomarker of anti-GD2 mAb resistance. Additionally, NB-derived small extracellular vesicles (sEVs) induce resistance to Dinutuximab by promoting an immunosuppressive tumor microenviroment characterized by a decrease in tumor-infiltrating NK cells and an increase in TAMs (104). Meanwhile, Tipifarnib, a farnesyltransferase inhibitor, enhances the efficacy of Dinutuximab by inhibiting sEV secretion (104). Given that Tipifarnib has entered phase II clinical trials in pediatric patients with advanced solid tumors, the combination of Dinutuximab and Tipifairnib could be rapidly translated to the clinic for HRNB patients.
Chimeric antigen receptors(CAR)-T therapy has shown great promise in relapsed or refractory B-cell cancers (105, 106). However, in solid tumors, only a minority of patients have a good prognosis (107–109). This is mainly due to the limited persistence of CAR-T cells in vivo and impaired T-cell function when treating solid tumors (110). In four trials, a cumulative total of 42 patients with active NB were treated with GD2-CAR T-cell-based therapy, of which only three achieved a sustained objective response (111–114).
Similarly, NK cells have been applied in various types of CARs immunotherapy against both hematological malignancies (115) and solid tumors (116–121). Preclinical and clinical trials using CAR NK cells have demonstrated that CAR NK cells have several advantages over CAR T cells, such as increased general availability of modified immune cells from healthy donors, potent anti-tumor effects, and therapeutic safety (122–126).
GD2 has been suggested as a possible target for RB-specific CAR-T cell treatment by Andersch, L et al. (Figure 2D) (17). RB cells are effectively targeted and killed in vitro by GD2-CAR T cells (17, 44) in a manner dependent on antigen density and cytokine release (17). The increased expression of inhibitory molecules programmed death protein 1(PD-1) in CAR T cells and programmed death ligand 1 (PD-L1) in RB cells, suggests the presence of immune escape in tumor cells, and repeated exposure to antigens also inhibits the function of CAR-T cells (44). This suggests that combination therapy involving immune checkpoint inhibitors and GD2-CAR T cells may be beneficial for patients with RB.
Furthermore, Wang, K. et al. developed a local immunotherapy strategy that effectively eradicated RB cells in a mouse model without impairing vision, and even enhanced the structural and functional recovery of the retina. This was achieved by employing a hydrogel-encapsulated GD2-CAR-T releasing IL-15, which enhances the antitumor effects of GD2-CAR-Ts by sustaining their local survival (45).
Wagner, J. et al. have identified four major challenges to CAR-T therapy for solid tumors (108): Firstly, there is a limited number of targetable antigens and heterogeneous antigen expression. Heterogeneity of the structures in carbohydrate chains is a characteristic hallmark of gangliosides (127). GD2 is heterogeneously expressed in RB (7), DMG (128), pediatric high-grade gliomas (128) and NSCLC (129) tumor tissue. Secondly, there is restricted T cell viability and longevity prior to reaching tumor areas. Thirdly, T cells remain incapable of effectively navigating to tumor sites and overcoming physical barriers. Lastly, a tumor microenvironment suppresses immunity.
Among them, the lack of tumor-specific targets is a key factor hindering progress in CAR development for solid tumors. To date, clinical studies of adoptive cell therapy for NB have focused on GD2-CAR T cells. However, potential other targets or more refined CAR engineering will need to be sought out to improve the efficacy of CAR T cells for NB. Recently, Sun, M. et al. developed a CAR T therapy called CT3.28H.BBζ for children with NB, which involves a new immunotherapy target called glypican-2(GPC2) which outperformed GD-2 CAR, K666.28H.BBζ, both in vivo and in vitro trials (130). In addition, Bergaggio, E. et al. developed CAR constructs targeting the anaplastic lymphoma kinase (ALK) receptor (131), which is selectively expressed by neuroblastoma cells, and showed that ALK.CAR-Ts was as effective as GD2. CAR-Ts in a metastatic NB model. ALK.CAR-Ts were less active than GD2.CAR-Ts in cell lines with low ALK expression but high GD2 expression. Besides, ALK.CAR-Ts were more active than GD2.CAR-Ts in cell lines with low GD2 expression. Given the complementary nature of ALK.CAR-Ts and GD2.CAR-Ts, dual CAR constructs that co-target ALK and GD2 could combine the broad activity of GD2.CAR-Ts with the specificity of ALK.CAR-Ts.
The tonic signaling of CAR is thought to be a vital factor in controlling CAR-T efficacy. Strong CAR signaling can lead to rapid T-cell exhaustion, which can impair anti-tumor function (132). Chen, J. el al. reveal that positively charged plaques (PCPs) on the surface of CAR antigen-binding domains mediate CAR clustering and generate CAR tonic signals (133). For GD2-CAR with high tonic signals, reducing PCPs or increasing ionic strength in the culture medium during ex vivo CAR-T cell expansion minimizes spontaneous CAR activation and alleviates CAR-T cell exhaustion. This suggests that rational adjustment of PCPs to optimize tonic signaling and in vivo fitness of CAR-T cells is a promising design strategy for the next-generation CAR-T cells. Furthermore, they developed a bioinformatics tool (PCP score) to quantify positively charged plaques on the surface of the CAR as a predictor of CAR tonic signals. They proposed that a PCP score around 46-56 can produce optimal CAR signals. Targeting CARs at the T-cell receptor alpha constant (TRAC) gene to modify tonic signaling and phenotype could present a viable strategy to slow T-cell differentiation in the treatment of GD2+ solid tumors. Mueller, K.P. et al. developed viral-free TRAC-targeted CAR T cells for GD2+ solid tumors using CRISPR technology and found evidence of viral-free CRISPR CAR T cells induced solid tumor regression in vivo by reducing differentiation, tonic signaling, and exhaustion (134).
All currently commercialized CAR-T cells use viral vector-based transgene delivery (135). These therapies fail to meet clinical needs, in part due to high costs and supply chain constraints associated with manufacturing and qualifying GMP-grade vectors. Balke-Want, H. et al. demonstrated for the first time that plasmid DNA-mediated homology-independent targeted insertion (HITI) could be used for targeted insertion of a transgene into primary human T cells and demonstrated that the combination of HITI with CRISPR EnrichMENT (CEMENT) resulted in clinically relevant CAR-T cell yields, thus providing a highly efficient, genotoxic-free, clinical-scale production process (136). To transfer the “all-in-one” vector concept from T cells to NK cells for the treatment of solid malignancies, Rudek, L.S. et al. designed alpha retroviral “all-in-one” vector that combine constitutive GD2-CAR expression and inducible cytokine expression under the control of an inducible NFkB promoter element (137). This demonstrated a tightly linked induction of antigen-specific transgenes, with enhanced cytolytic potential, as evidenced by increased NK cell activation and cytokine release in NK cell subsets.
The duration of passive immune effects triggered by anti-GD2 monoclonal antibodies is generally short-lived (138), whereas vaccination against GD2 as a form of active immunization may have a prolonged effect on tumors (139). Vaccination may specifically target tumor-associated antigens (TAAs), whereas non-specific active immunization may influence normal tissues of the body, which is an important distinction between the two (140). Since many TAAs are autoantigens, the body may develop immune tolerance, which can be effectively addressed by anti-Id Abs as an antigen-based vaccine (141). The mechanism of anti-Id antibody production is that when the antigen enters the body, it immunizes against the Ag and produces Ab1 against that Ag, which in turn can produce Ab2 against Ab1, i.e., anti-Id antibody. Additionally, portion of Ab2 can mimic the starting antigen and produce a specific immune response similar to that of the starting antigen, which is referred to as Ab2β (142). A major advantage of anti-Id vaccines is that they can be administered against antigens of non-protein origin. In a phase I clinical trial against an anti-unique antibody (TriGem), 40 out of 47 patients had hyperimmune sera that had Ab3 capable of binding specifically to GD2, and this anti-unique antibody vaccine showed a good IgG immune response as well as minimal toxicity (143). Another vaccine combines gangliosides with an immune carrier protein (KLH) in combination with an immune adjuvant thereby exerting an immune response. In a phase I trial, GD2 and GD3, i.e., GD2L and GD3L, formed from lactone, were combined with the immune carrier protein keyhole limpet hemocyanin (KLH) to form GD2L-KLH, GD3L-KLH, and finally with the immune adjuvant, OPT-821. The treatment also included oral immunostimulant, β-glucan. 12 out of 15 patients developed an antibody response to GD2 and/or GD3, and 6 of 10 evaluable patients showed disappearance of minimal residual disease (MRD) (144). In a subsequent phase II trial, the use of GD2/GD3 vaccine and oral β-glucan produced a strong antibody response in HR-NB patients with previous disease progression, and the results showed that high titers of anti-GD2-IgG1 were associated with improved survival (145).
Nanoparticles can deliver cytotoxic drugs or RNA targets to tumor cells for therapeutic effects (Figure 2E). Nanoparticles for drug delivery can be made from lipids, polymers (e.g., dendrimers), organometallic compounds, and viruses (146). Most nanoparticles targeting tumors will usually consist of a polyethylene glycol (PEG) coating and a targeting ligand. Initially, the reticulo-endothelial system (RES) destroys the nanoparticles, which can be protected by the use of PEGs (147, 148). With this protection, the nanoparticles can safely reach the lesion and release the drug, increasing the accumulation in the bloodstream and at the site of the tumor. Secondly, the targeting ligands may be structures such as monoclonal antibodies (149), antibody fragments (150), aptamers (151), peptide-based targeting molecules (152) and small molecules (153), which can deliver the nanoparticles to the tumor lesion. When MicroRNA-34a is overexpressed in tumor cells, it can activate the cysteine asparaginase-mediated apoptotic pathway (149). Tivnan et al. found that anti-GD2 nanoparticle-mediated targeted delivery of miR-34a inhibited the growth of tumor cells by coupling MoAb ch14.18 to silica nanoparticles piggybacked with MicroRNA-34a (149). Adrian et al. found that neuroblastoma can down-regulate vascular endothelial growth factor A (VEGF-A) through GD2-mediated endocytosis by uptake of anti-GD2 liposome piggybacked siRNA (154). Etoposide was encapsulated into liposomes and coupled with anti-GD2mAb to form immunoliposomes, which not only targeted GD2-positive tumor cells and inhibited tumor cells proliferation, but also reduced systemic side effects (155).
This review briefly describes the expression of ganglioside disialoglycate (GD2) on the surface of retinoblastoma and its biological functions. We review several immunotherapeutic approaches against GD2, an anti-GD2 monoclonal antibody that has been applied in the clinical treatment of neuroblastoma and achieved good therapeutic effect, and recent research progress. Cellular immunotherapy attacks tumor cells via T cells or NK cells, which has shown promising therapeutic effects in the treatment of B-cell malignancies. However, the current research on GD2 is not yet available good therapeutic effect, while the treatment for solid tumors is currently in the stage of preclinical research and clinical trials. The GD2 vaccine, an active immunotherapy, has demonstrated favorable antibody responses in clinical trials. Targeted nanoparticles can deliver cytotoxic drugs or RNA to tumor cells via antibodies and other substances.
XZ: Writing – original draft, Writing – review & editing. WY: Writing – original draft, Writing – review & editing. YW: Writing – review & editing. RD: Writing – review & editing. CW: Writing – review & editing. JL: Writing – original draft, Writing – review & editing. YH: Writing – original draft, Writing – review & editing.
The author(s) declare financial support was received for the research, authorship, and/or publication of this article. This work is supported by Science and technology project jointly established by the Science and Technology Department of the State Administration of Traditional Chinese Medicine (GZY-KJS-SD-2023-079), Shandong Provincial Health Commission(M-2022053), the National Natural Science Foundation of China (Grant No. 82104289), Yuandu Scholar Grant of Weifang City to JL, Science and Technology Innovation Plan from Weifang Medical University (041004), Weifang Science and Technology Bureau Plan Project (2021YX081), Shandong Provincial Medical Association Young Talent Promotion Project (2023_GJ_0039), Weifang Key Laboratory of Biomimetic Diagnostics, Qilu Biancang Traditional Chinese Medicine Talent Cultivation Project. WY is also supported by the Top Talent Support Program for young and middle-aged people of Wuxi Health Committee (BJ2023070).
The authors declare that the research was conducted in the absence of any commercial or financial relationships that could be construed as a potential conflict of interest.
All claims expressed in this article are solely those of the authors and do not necessarily represent those of their affiliated organizations, or those of the publisher, the editors and the reviewers. Any product that may be evaluated in this article, or claim that may be made by its manufacturer, is not guaranteed or endorsed by the publisher.
1. Yun J, Li Y, Xu CT, Pan BR. Epidemiology and Rb1 gene of retinoblastoma. Int J Ophthalmol. (2011) 4:103–9. doi: 10.3980/j.issn.2222-3959.2011.01.24
2. Global Retinoblastoma Study G. The Global Retinoblastoma Outcome Study: a prospective, cluster-based analysis of 4064 patients from 149 countries. Lancet Glob Health. (2022) 10:e1128–e40. doi: 10.1016/S2214-109X(22)00250-9
3. Global Retinoblastoma Study G, Fabian ID, Abdallah E, Abdullahi SU, Abdulqader RA, Adamou Boubacar S, et al. Global retinoblastoma presentation and analysis by national income level. JAMA Oncol. (2020) 6:685–95. doi: 10.1001/jamaoncol.2019.6716
4. Wang L, Li S, Mei J, Ye L. Immunotherapies of retinoblastoma: Effective methods for preserving vision in the future. Front Oncol. (2022) 12:949193. doi: 10.3389/fonc.2022.949193
5. Chen M, Luo C, Zhao J, Devarajan G, Xu H. Immune regulation in the aging retina. Prog Retin Eye Res. (2019) 69:159–72. doi: 10.1016/j.preteyeres.2018.10.003
6. Park DY, Lee J, Kim J, Kim K, Hong S, Han S, et al. Plastic roles of pericytes in the blood-retinal barrier. Nat Commun. (2017) 8:15296. doi: 10.1038/ncomms15296
7. Wang H, Yang J, Pan H, Tai MC, Maher MH, Jia R, et al. Dinutuximab synergistically enhances the cytotoxicity of natural killer cells to retinoblastoma through the perforin-granzyme B pathway. Onco Targets Ther. (2020) 13:3903–20. doi: 10.2147/OTT.S228532
8. Ancona-Lezama D, Dalvin LA, Shields CL. Modern treatment of retinoblastoma: A 2020 review. Indian J Ophthalmol. (2020) 68:2356–65. doi: 10.4103/ijo.IJO_721_20
9. Martinez-Sanchez M, Hernandez-Monge J, Rangel M, Olivares-Illana V. Retinoblastoma: from discovery to clinical management. FEBS J. (2022) 289:4371–82. doi: 10.1111/febs.v289.15
10. Kaliki S, Shields CL, Shah SU, Eagle RC Jr., Shields JA, Leahey A. Postenucleation adjuvant chemotherapy with vincristine, etoposide, and carboplatin for the treatment of high-risk retinoblastoma. Arch Ophthalmol. (2011) 129:1422–7. doi: 10.1001/archophthalmol.2011.289
11. Qaddoumi I, Bass JK, Wu J, Billups CA, Wozniak AW, Merchant TE, et al. Carboplatin-associated ototoxicity in children with retinoblastoma. J Clin Oncol. (2012) 30:1034–41. doi: 10.1200/JCO.2011.36.9744
12. Abramson DH, Dunkel IJ, Brodie SE, Marr B, Gobin YP. Superselective ophthalmic artery chemotherapy as primary treatment for retinoblastoma (chemosurgery). Ophthalmology. (2010) 117:1623–9. doi: 10.1016/j.ophtha.2009.12.030
13. Kaliki S, Shields CL. Retinoblastoma: achieving new standards with methods of chemotherapy. Indian J Ophthalmol. (2015) 63:103–9. doi: 10.4103/0301-4738.154369
14. Kim JY, Park Y. Treatment of retinoblastoma: the role of external beam radiotherapy. Yonsei Med J. (2015) 56:1478–91. doi: 10.3349/ymj.2015.56.6.1478
15. Leclerc R, Olin J. An overview of retinoblastoma and enucleation in pediatric patients. AORN J. (2020) 111:69–79. doi: 10.1002/aorn.v111.1
16. Kaewkhaw R, Rojanaporn D. Retinoblastoma: etiology, modeling, and treatment. Cancers (Basel). (2020) 12:2304. doi: 10.3390/cancers12082304
17. Andersch L, Radke J, Klaus A, Schwiebert S, Winkler A, Schumann E, et al. CD171- and GD2-specific CAR-T cells potently target retinoblastoma cells in preclinical in vitro testing. BMC Cancer. (2019) 19:895. doi: 10.1186/s12885-019-6131-1
18. Singh L, Singh MK, Rizvi MA, Bakhshi S, Meel R, Lomi N, et al. Clinical relevance of the comparative expression of immune checkpoint markers with the clinicopathological findings in patients with primary and chemoreduced retinoblastoma. Cancer Immunol Immunother. (2020) 69:1087–99. doi: 10.1007/s00262-020-02529-4
19. Ganesan B, Parameswaran S, Sharma A, Krishnakumar S. Clinical relevance of B7H3 expression in retinoblastoma. Sci Rep. (2020) 10:10185. doi: 10.1038/s41598-020-67101-7
20. Krishnakumar S, Mohan A, Mallikarjuna K, Venkatesan N, Biswas J, Shanmugam MP, et al. EpCAM expression in retinoblastoma: a novel molecular target for therapy. Invest Ophthalmol Vis Sci. (2004) 45:4247–50. doi: 10.1167/iovs.04-0591
21. Chen X, Kunda PE, Lin J, Zhou M, Huang J, Zhang H, et al. SYK-targeted dendritic cell-mediated cytotoxic T lymphocytes enhance the effect of immunotherapy on retinoblastoma. J Cancer Res Clin Oncol. (2018) 144:675–84. doi: 10.1007/s00432-018-2584-x
22. Cheever MA, Allison JP, Ferris AS, Finn OJ, Hastings BM, Hecht TT, et al. The prioritization of cancer antigens: a national cancer institute pilot project for the acceleration of translational research. Clin Cancer Res. (2009) 15:5323–37. doi: 10.1158/1078-0432.CCR-09-0737
23. Portoukalian J, David MJ, Gain P, Richard M. Shedding of GD2 ganglioside in patients with retinoblastoma. Int J Cancer. (1993) 53:948–51. doi: 10.1002/ijc.2910530614
24. Machy P, Mortier E, Birkle S. Biology of GD2 ganglioside: implications for cancer immunotherapy. Front Pharmacol. (2023) 14:1249929. doi: 10.3389/fphar.2023.1249929
25. Horwacik I, Golik P, Grudnik P, Kolinski M, Zdzalik M, Rokita H, et al. Structural basis of GD2 ganglioside and mimetic peptide recognition by 14G2a antibody. Mol Cell Proteomics. (2015) 14:2577–90. doi: 10.1074/mcp.M115.052720
26. Nazha B, Inal C, Owonikoko TK. Disialoganglioside GD2 expression in solid tumors and role as a target for cancer therapy. Front Oncol. (2020) 10:1000. doi: 10.3389/fonc.2020.01000
27. Mandon EC, Ehses I, Rother J, van Echten G, Sandhoff K. Subcellular localization and membrane topology of serine palmitoyltransferase, 3-dehydrosphinganine reductase, and sphinganine N-acyltransferase in mouse liver. J Biol Chem. (1992) 267:11144–8. doi: 10.1016/S0021-9258(19)49887-6
28. Hanada K, Kumagai K, Tomishige N, Yamaji T. CERT-mediated trafficking of ceramide. Biochim Biophys Acta. (2009) 1791:684–91. doi: 10.1016/j.bbalip.2009.01.006
29. Sandhoff R, Schulze H, Sandhoff K. Ganglioside metabolism in health and disease. Prog Mol Biol Transl Sci. (2018) 156:1–62. doi: 10.1016/bs.pmbts.2018.01.002
30. Iber H, Zacharias C, Sandhoff K. The c-series gangliosides GT3, GT2 and GP1c are formed in rat liver Golgi by the same set of glycosyltransferases that catalyse the biosynthesis of asialo-, a- and b-series gangliosides. Glycobiology. (1992) 2:137–42. doi: 10.1093/glycob/2.2.137
31. Crespo PM, Iglesias-Bartolome R, Daniotti JL. Ganglioside GD3 traffics from the trans-Golgi network to plasma membrane by a Rab11-independent and brefeldin A-insensitive exocytic pathway. J Biol Chem. (2004) 279:47610–8. doi: 10.1074/jbc.M407181200
32. Julien S, Bobowski M, Steenackers A, Le Bourhis X, Delannoy P. How do gangliosides regulate RTKs signaling? Cells. (2013) 2:751–67. doi: 10.3390/cells2040751
33. Cheresh DA, Reisfeld RA, Varki AP. O-acetylation of disialoganglioside GD3 by human melanoma cells creates a unique antigenic determinant. Science. (1984) 225:844–6. doi: 10.1126/science.6206564
34. Fleurence J, Fougeray S, Bahri M, Cochonneau D, Clemenceau B, Paris F, et al. Targeting O-acetyl-GD2 ganglioside for cancer immunotherapy. J Immunol Res. (2017) 2017:5604891. doi: 10.1155/2017/5604891
35. Ye JN, Cheung NK. A novel O-acetylated ganglioside detected by anti-GD2 monoclonal antibodies. Int J Cancer. (1992) 50:197–201. doi: 10.1002/ijc.2910500207
36. Fleurence J, Cochonneau D, Fougeray S, Oliver L, Geraldo F, Terme M, et al. Targeting and killing glioblastoma with monoclonal antibody to O-acetyl GD2 ganglioside. Oncotarget. (2016) 7:41172–85. doi: 10.18632/oncotarget.9226
37. Huwiler A, Kolter T, Pfeilschifter J, Sandhoff K. Physiology and pathophysiology of sphingolipid metabolism and signaling. Biochim Biophys Acta. (2000) 1485:63–99. doi: 10.1016/S1388-1981(00)00042-1
38. Battula VL, Shi Y, Evans KW, Wang RY, Spaeth EL, Jacamo RO, et al. Ganglioside GD2 identifies breast cancer stem cells and promotes tumorigenesis. J Clin Invest. (2012) 122:2066–78. doi: 10.1172/JCI59735
39. Liang YJ, Ding Y, Levery SB, Lobaton M, Handa K, Hakomori SI. Differential expression profiles of glycosphingolipids in human breast cancer stem cells vs. cancer non-stem cells. Proc Natl Acad Sci U.S.A. (2013) 110:4968–73. doi: 10.1073/pnas.1302825110
40. Yanagisawa M, Yoshimura S, Yu RK. Expression of GD2 and GD3 gangliosides in human embryonic neural stem cells. ASN Neuro. (2011) 3:e00054. doi: 10.1042/AN20110006
41. Lammie G, Cheung N, Gerald W, Rosenblum M, Cordoncardo C. Ganglioside gd(2) expression in the human nervous-system and in neuroblastomas - an immunohistochemical study. Int J Oncol. (1993) 3:909–15. doi: 10.3892/ijo.3.5.909
42. Jin HJ, Nam HY, Bae YK, Kim SY, Im IR, Oh W, et al. GD2 expression is closely associated with neuronal differentiation of human umbilical cord blood-derived mesenchymal stem cells. Cell Mol Life Sci. (2010) 67:1845–58. doi: 10.1007/s00018-010-0292-z
43. Martinez C, Hofmann TJ, Marino R, Dominici M, Horwitz EM. Human bone marrow mesenchymal stromal cells express the neural ganglioside GD2: a novel surface marker for the identification of MSCs. Blood. (2007) 109:4245–8. doi: 10.1182/blood-2006-08-039347
44. Sujjitjoon J, Sayour E, Tsao ST, Uiprasertkul M, Sanpakit K, Buaboonnam J, et al. GD2-specific chimeric antigen receptor-modified T cells targeting retinoblastoma - assessing tumor and T cell interaction. Transl Oncol. (2021) 14:100971. doi: 10.1016/j.tranon.2020.100971
45. Wang K, Chen Y, Ahn S, Zheng M, Landoni E, Dotti G, et al. GD2-specific CAR T cells encapsulated in an injectable hydrogel control retinoblastoma and preserve vision. Nat Cancer. (2020) 1:990–7. doi: 10.1038/s43018-020-00119-y
46. Wang BY, Huang JY, Chen HC, Lin CH, Lin SH, Hung WH, et al. The comparison between adenocarcinoma and squamous cell carcinoma in lung cancer patients. J Cancer Res Clin Oncol. (2020) 146:43–52. doi: 10.1007/s00432-019-03079-8
47. Suzuki M, Cheung NK. Disialoganglioside GD2 as a therapeutic target for human diseases. Expert Opin Ther Targets. (2015) 19:349–62. doi: 10.1517/14728222.2014.986459
48. Chantada GL, Rossi J, Casco F, Fandino A, Scopinaro M, de Davila MT, et al. An aggressive bone marrow evaluation including immunocytology with GD2 for advanced retinoblastoma. J Pediatr Hematol Oncol. (2006) 28:369–73. doi: 10.1097/00043426-200606000-00009
49. Wu ZL, Schwartz E, Seeger R, Ladisch S. Expression of GD2 ganglioside by untreated primary human neuroblastomas. Cancer Res. (1986) 46:440–3.
50. Mansoori M, Mirzaei A, Abdi Rad I, Mahmodlou R, Mansouri F, Saeednejad Zanjani L, et al. Upregulation of ganglioside GD2 synthase (GD2S), as a new putative cancer stem cell marker in breast carcinomas. Med J Islam Repub Iran. (2021) 35:148. doi: 10.47176/mjiri.35.148
51. Vantaku V, Donepudi SR, Ambati CR, Jin F, Putluri V, Nguyen K, et al. Expression of ganglioside GD2, reprogram the lipid metabolism and EMT phenotype in bladder cancer. Oncotarget. (2017) 8:95620–31. doi: 10.18632/oncotarget.21038
52. Cheresh DA, Rosenberg J, Mujoo K, Hirschowitz L, Reisfeld RA. Biosynthesis and expression of the disialoganglioside GD2, a relevant target antigen on small cell lung carcinoma for monoclonal antibody-mediated cytolysis. Cancer Res. (1986) 46:5112–8.
53. Yoshida S, Kawaguchi H, Sato S, Ueda R, Furukawa K. An anti-GD2 monoclonal antibody enhances apoptotic effects of anti-cancer drugs against small cell lung cancer cells via JNK (c-Jun terminal kinase) activation. Jpn J Cancer Res. (2002) 93:816–24. doi: 10.1111/j.1349-7006.2002.tb01324.x
54. Shibuya H, Hamamura K, Hotta H, Matsumoto Y, Nishida Y, Hattori H, et al. Enhancement of Malignant properties of human osteosarcoma cells with disialyl gangliosides GD2/GD3. Cancer Sci. (2012) 103:1656–64. doi: 10.1111/j.1349-7006.2012.02344.x
55. Kailayangiri S, Altvater B, Meltzer J, Pscherer S, Luecke A, Dierkes C, et al. The ganglioside antigen G(D2) is surface-expressed in Ewing sarcoma and allows for MHC-independent immune targeting. Br J Cancer. (2012) 106:1123–33. doi: 10.1038/bjc.2012.57
56. Bailey K, Cost C, Davis I, Glade-Bender J, Grohar P, Houghton P, et al. Emerging novel agents for patients with advanced Ewing sarcoma: a report from the Children’s Oncology Group (COG) New Agents for Ewing Sarcoma Task Force. F1000Res. (2019) 8:F1000 Faculty Rev-493. doi: 10.12688/f1000research
57. Mount CW, Majzner RG, Sundaresh S, Arnold EP, Kadapakkam M, Haile S, et al. Potent antitumor efficacy of anti-GD2 CAR T cells in H3-K27M(+) diffuse midline gliomas. Nat Med. (2018) 24:572–9. doi: 10.1038/s41591-018-0006-x
58. Shen H, Tang Y, Xu X, Tang H. Detection of the GD2+/CD56+/CD45- immunophenotype by flow cytometry in cerebrospinal fluids from a patient with retinoblastoma. Pediatr Hematol Oncol. (2013) 30:30–2. doi: 10.3109/08880018.2012.737094
59. Groux-Degroote S, Rodriguez-Walker M, Dewald JH, Daniotti JL, Delannoy P. Gangliosides in cancer cell signaling. Prog Mol Biol Transl Sci. (2018) 156:197–227. doi: 10.1016/bs.pmbts.2017.10.003
60. Cazet A, Bobowski M, Rombouts Y, Lefebvre J, Steenackers A, Popa I, et al. The ganglioside G(D2) induces the constitutive activation of c-Met in MDA-MB-231 breast cancer cells expressing the G(D3) synthase. Glycobiology. (2012) 22:806–16. doi: 10.1093/glycob/cws049
61. Liang YJ, Wang CY, Wang IA, Chen YW, Li LT, Lin CY, et al. Interaction of glycosphingolipids GD3 and GD2 with growth factor receptors maintains breast cancer stem cell phenotype. Oncotarget. (2017) 8:47454–73. doi: 10.18632/oncotarget.17665
62. Cavdarli S, Groux-Degroote S, Delannoy P. Gangliosides: the double-edge sword of neuro-ectodermal derived tumors. Biomolecules. (2019) 9:311. doi: 10.3390/biom9080311
63. Aixinjueluo W, Furukawa K, Zhang Q, Hamamura K, Tokuda N, Yoshida S, et al. Mechanisms for the apoptosis of small cell lung cancer cells induced by anti-GD2 monoclonal antibodies: roles of anoikis. J Biol Chem. (2005) 280:29828–36. doi: 10.1074/jbc.M414041200
64. Shurin GV, Shurin MR, Bykovskaia S, Shogan J, Lotze MT, Barksdale EM Jr. Neuroblastoma-derived gangliosides inhibit dendritic cell generation and function. Cancer Res. (2001) 61:363–9.
65. Lee HC, Wondimu A, Liu Y, Ma JS, Radoja S, Ladisch S. Ganglioside inhibition of CD8+ T cell cytotoxicity: interference with lytic granule trafficking and exocytosis. J Immunol. (2012) 189:3521–7. doi: 10.4049/jimmunol.1201256
66. Wondimu A, Liu Y, Su Y, Bobb D, Ma JS, Chakrabarti L, et al. Gangliosides drive the tumor infiltration and function of myeloid-derived suppressor cells. Cancer Res. (2014) 74:5449–57. doi: 10.1158/0008-5472.CAN-14-0927
67. Jales A, Falahati R, Mari E, Stemmy EJ, Shen W, Southammakosane C, et al. Ganglioside-exposed dendritic cells inhibit T-cell effector function by promoting regulatory cell activity. Immunology. (2011) 132:134–43. doi: 10.1111/j.1365-2567.2010.03348.x
68. Yesmin F, Furukawa K, Kambe M, Ohmi Y, Bhuiyan RH, Hasnat MA, et al. Extracellular vesicles released from ganglioside GD2-expressing melanoma cells enhance the Malignant properties of GD2-negative melanomas. Sci Rep. (2023) 13:4987. doi: 10.1038/s41598-023-31216-4
69. Laurent VE, Otero LL, Vazquez V, Camarero S, Gabri MR, Labraga M, et al. Optimization of molecular detection of GD2 synthase mRNA in retinoblastoma. Mol Med Rep. (2010) 3:253–9. doi: 10.3892/mmr_00000248
70. Esaki N, Ohkawa Y, Hashimoto N, Tsuda Y, Ohmi Y, Bhuiyan RH, et al. ASC amino acid transporter 2, defined by enzyme-mediated activation of radical sources, enhances Malignancy of GD2-positive small-cell lung cancer. Cancer Sci. (2018) 109:141–53. doi: 10.1111/cas.2018.109.issue-1
71. Laurent VE, Sampor C, Solernou V, Rossi J, Gabri M, Eandi-Eberle S, et al. Detection of minimally disseminated disease in the cerebrospinal fluid of children with high-risk retinoblastoma by reverse transcriptase-polymerase chain reaction for GD2 synthase mRNA. Eur J Cancer. (2013) 49:2892–9. doi: 10.1016/j.ejca.2013.04.021
72. Aschero R, Torbidoni A, Sampor C, Laurent V, Zugbi S, Winter U, et al. Minimally disseminated disease and outcome in overt orbital retinoblastoma. Pediatr Blood Cancer. (2019) 66:e27662. doi: 10.1002/pbc.27662
73. Pascual-Pasto G, Olaciregui NG, Vila-Ubach M, Paco S, Monterrubio C, Rodriguez E, et al. Preclinical platform of retinoblastoma xenografts recapitulating human disease and molecular markers of dissemination. Cancer Lett. (2016) 380:10–9. doi: 10.1016/j.canlet.2016.06.012
74. Ahmed M, Goldgur Y, Hu J, Guo HF, Cheung NK. In silico driven redesign of a clinically relevant antibody for the treatment of GD2 positive tumors. PloS One. (2013) 8:e63359. doi: 10.1371/journal.pone.0063359
75. Perez Horta Z, Goldberg JL, Sondel PM. Anti-GD2 mAbs and next-generation mAb-based agents for cancer therapy. Immunotherapy. (2016) 8:1097–117. doi: 10.2217/imt-2016-0021
76. Eichholz T, Heubach F, Arendt AM, Seitz C, Brecht IB, Ebinger M, et al. Targeted therapies in retinoblastoma: GD2-directed immunotherapy following autologous stem cell transplantation and evaluation of alternative target B7-H3. Cancer Immunol Immunother. (2024) 73:19. doi: 10.1007/s00262-023-03587-0
77. Theruvath J, Menard M, Smith BAH, Linde MH, Coles GL, Dalton GN, et al. Anti-GD2 synergizes with CD47 blockade to mediate tumor eradication. Nat Med. (2022) 28:333–44. doi: 10.1038/s41591-021-01625-x
78. Taylor RP, Lindorfer MA. The role of complement in mAb-based therapies of cancer. Methods. (2014) 65:18–27. doi: 10.1016/j.ymeth.2013.07.027
79. Doronin II, Vishnyakova PA, Kholodenko IV, Ponomarev ED, Ryazantsev DY, Molotkovskaya IM, et al. Ganglioside GD2 in reception and transduction of cell death signal in tumor cells. BMC Cancer. (2014) 14:295. doi: 10.1186/1471-2407-14-295
80. Dhillon S. Dinutuximab: first global approval. Drugs. (2015) 75:923–7. doi: 10.1007/s40265-015-0399-5
81. Yu AL, Gilman AL, Ozkaynak MF, London WB, Kreissman SG, Chen HX, et al. Anti-GD2 antibody with GM-CSF, interleukin-2, and isotretinoin for neuroblastoma. N Engl J Med. (2010) 363:1324–34. doi: 10.1056/NEJMoa0911123
82. Yu AL, Gilman AL, Ozkaynak MF, Naranjo A, Diccianni MB, Gan J, et al. Long-term follow-up of a phase III study of ch14.18 (Dinutuximab) + Cytokine immunotherapy in children with high-risk neuroblastoma: COG study ANBL0032. Clin Cancer Res. (2021) 27:2179–89. doi: 10.1158/1078-0432.CCR-20-3909
83. Ladenstein R, Pötschger U, Valteau-Couanet D, Luksch R, Castel V, Ash S, et al. Investigation of the role of dinutuximab beta-based immunotherapy in the SIOPEN high-risk neuroblastoma 1 trial (HR-NBL1). Cancers (Basel). (2020) 12:309. doi: 10.3390/cancers12020309
84. Ladenstein R, Potschger U, Valteau-Couanet D, Luksch R, Castel V, Yaniv I, et al. Interleukin 2 with anti-GD2 antibody ch14.18/CHO (dinutuximab beta) in patients with high-risk neuroblastoma (HR-NBL1/SIOPEN): a multicentre, randomised, phase 3 trial. Lancet Oncol. (2018) 19:1617–29. doi: 10.1016/S1470-2045(18)30578-3
86. Kushner BH, Cheung IY, Modak S, Basu EM, Roberts SS, Cheung NK. Humanized 3F8 anti-GD2 monoclonal antibody dosing with granulocyte-macrophage colony-stimulating factor in patients with resistant neuroblastoma: A phase 1 clinical trial. JAMA Oncol. (2018) 4:1729–35. doi: 10.1001/jamaoncol.2018.4005
87. Stip MC, Evers M, Nederend M, Chan C, Reiding KR, Damen MJ, et al. IgA antibody immunotherapy targeting GD2 is effective in preclinical neuroblastoma models. J Immunother Cancer. (2023) 11:e006948. doi: 10.1136/jitc-2023-006948
88. Navid F, Sondel PM, Barfield R, Shulkin BL, Kaufman RA, Allay JA, et al. Phase I trial of a novel anti-GD2 monoclonal antibody, Hu14.18K322A, designed to decrease toxicity in children with refractory or recurrent neuroblastoma. J Clin Oncol. (2014) 32:1445–52. doi: 10.1200/JCO.2013.50.4423
89. Furman WL, McCarville B, Shulkin BL, Davidoff A, Krasin M, Hsu CW, et al. Improved outcome in children with newly diagnosed high-risk neuroblastoma treated with chemoimmunotherapy: updated results of a phase II study using hu14.18K322A. J Clin Oncol. (2022) 40:335–44. doi: 10.1200/JCO.21.01375
90. Neal ZC, Yang JC, Rakhmilevich AL, Buhtoiarov IN, Lum HE, Imboden M, et al. Enhanced activity of hu14.18-IL2 immunocytokine against murine NXS2 neuroblastoma when combined with interleukin 2 therapy. Clin Cancer Res. (2004) 10:4839–47. doi: 10.1158/1078-0432.CCR-03-0799
91. Yang RK, Kalogriopoulos NA, Rakhmilevich AL, Ranheim EA, Seo S, Kim K, et al. Intratumoral treatment of smaller mouse neuroblastoma tumors with a recombinant protein consisting of IL-2 linked to the hu14.18 antibody increases intratumoral CD8+ T and NK cells and improves survival. Cancer Immunol Immunother. (2013) 62:1303–13. doi: 10.1007/s00262-013-1430-x
92. Nguyen R, Zhang X, Sun M, Abbas S, Seibert C, Kelly MC, et al. Anti-GD2 antibodies conjugated to IL15 and IL21 mediate potent antitumor cytotoxicity against neuroblastoma. Clin Cancer Res. (2022) 28:3785–96. doi: 10.1158/1078-0432.CCR-22-0717
93. Leonard WJ, Lin JX, O’Shea JJ. The γ(c) family of cytokines: basic biology to therapeutic ramifications. Immunity. (2019) 50:832–50. doi: 10.1016/j.immuni.2019.03.028
94. Liatsou I, Fu Y, Li Z, Hasan M, Guo X, Yu J, et al. Therapeutic efficacy of an alpha-particle emitter labeled anti-GD2 humanized antibody against osteosarcoma-a proof of concept study. Eur J Nucl Med Mol Imaging. (2024) 51:1409–20. doi: 10.1007/s00259-023-06528-2
95. Fu Y, Yu J, Liatsou I, Du Y, Josefsson A, Nedrow JR, et al. Anti-GD2 antibody for radiopharmaceutical imaging of osteosarcoma. Eur J Nucl Med Mol Imaging. (2022) 49:4382–93. doi: 10.1007/s00259-022-05888-5
96. Xiao WH, Yu AL, Sorkin LS. Electrophysiological characteristics of primary afferent fibers after systemic administration of anti-GD2 ganglioside antibody. Pain. (1997) 69:145–51. doi: 10.1016/S0304-3959(96)03280-0
97. Sorkin LS, Otto M, Baldwin WM 3rd, Vail E, Gillies SD, Handgretinger R, et al. Anti-GD(2) with an FC point mutation reduces complement fixation and decreases antibody-induced allodynia. Pain. (2010) 149:135–42. doi: 10.1016/j.pain.2010.01.024
98. Hamers FP, Lankhorst AJ, van Laar TJ, Veldhuis WB, Gispen WH. Automated quantitative gait analysis during overground locomotion in the rat: its application to spinal cord contusion and transection injuries. J Neurotrauma. (2001) 18:187–201. doi: 10.1089/08977150150502613
99. Cheung NK, Cheung IY, Kushner BH, Ostrovnaya I, Chamberlain E, Kramer K, et al. Murine anti-GD2 monoclonal antibody 3F8 combined with granulocyte-macrophage colony-stimulating factor and 13-cis-retinoic acid in high-risk patients with stage 4 neuroblastoma in first remission. J Clin Oncol. (2012) 30:3264–70. doi: 10.1200/JCO.2011.41.3807
100. Erbe AK, Diccianni MB, Mody R, Naranjo A, Zhang FF, Birstler J, et al. KIR/KIR-ligand genotypes and clinical outcomes following chemoimmunotherapy in patients with relapsed or refractory neuroblastoma: a report from the Children’s Oncology Group. J Immunother Cancer. (2023) 11:e006530. doi: 10.1136/jitc-2022-006530
101. Keyel ME, Reynolds CP. Spotlight on dinutuximab in the treatment of high-risk neuroblastoma: development and place in therapy. Biologics. (2019) 13:1–12. doi: 10.2147/BTT.S114530
102. Schumacher-Kuckelkorn R, Volland R, Gradehandt A, Hero B, Simon T, Berthold F. Lack of immunocytological GD2 expression on neuroblastoma cells in bone marrow at diagnosis, during treatment, and at recurrence. Pediatr Blood Cancer. (2017) 64:46–56. doi: 10.1002/pbc.26184
103. Pilgrim AA, Jonus HC, Ho A, Cole AC, Shim J, Goldsmith KC. The yes-associated protein (YAP) is associated with resistance to anti-GD2 immunotherapy in neuroblastoma through downregulation of ST8SIA1. Oncoimmunology. (2023) 12:2240678. doi: 10.1080/2162402X.2023.2240678
104. Liu X, Wills CA, Chen L, Zhang J, Zhao Y, Zhou M, et al. Small extracellular vesicles induce resistance to anti-GD2 immunotherapy unveiling tipifarnib as an adjunct to neuroblastoma immunotherapy. J Immunother Cancer. (2022) 10:e004399. doi: 10.1136/jitc-2021-004399
105. June CH, Sadelain M. Chimeric antigen receptor therapy. N Engl J Med. (2018) 379:64–73. doi: 10.1056/NEJMra1706169
106. Maude SL, Laetsch TW, Buechner J, Rives S, Boyer M, Bittencourt H, et al. Tisagenlecleucel in children and young adults with B-cell lymphoblastic leukemia. N Engl J Med. (2018) 378:439–48. doi: 10.1056/NEJMoa1709866
107. Rafiq S, Hackett CS, Brentjens RJ. Engineering strategies to overcome the current roadblocks in CAR T cell therapy. Nat Rev Clin Oncol. (2020) 17:147–67. doi: 10.1038/s41571-019-0297-y
108. Wagner J, Wickman E, DeRenzo C, Gottschalk S. CAR T cell therapy for solid tumors: bright future or dark reality? Mol Ther. (2020) 28:2320–39. doi: 10.1016/j.ymthe.2020.09.015
109. Sun M, Cao Y, Okada R, Reyes-Gonzalez JM, Stack HG, Qin H, et al. Preclinical optimization of a GPC2-targeting CAR T-cell therapy for neuroblastoma. J Immunother Cancer. (2023) 11:e005881. doi: 10.1136/jitc-2022-005881
110. Majzner RG, Mackall CL. Clinical lessons learned from the first leg of the CAR T cell journey. Nat Med. (2019) 25:1341–55. doi: 10.1038/s41591-019-0564-6
111. Pule MA, Savoldo B, Myers GD, Rossig C, Russell HV, Dotti G, et al. Virus-specific T cells engineered to coexpress tumor-specific receptors: persistence and antitumor activity in individuals with neuroblastoma. Nat Med. (2008) 14:1264–70. doi: 10.1038/nm.1882
112. Louis CU, Savoldo B, Dotti G, Pule M, Yvon E, Myers GD, et al. Antitumor activity and long-term fate of chimeric antigen receptor-positive T cells in patients with neuroblastoma. Blood. (2011) 118:6050–6. doi: 10.1182/blood-2011-05-354449
113. Heczey A, Louis CU, Savoldo B, Dakhova O, Durett A, Grilley B, et al. CAR T cells administered in combination with lymphodepletion and PD-1 inhibition to patients with neuroblastoma. Mol Ther. (2017) 25:2214–24. doi: 10.1016/j.ymthe.2017.05.012
114. Straathof K, Flutter B, Wallace R, Jain N, Loka T, Depani S, et al. Antitumor activity without on-target off-tumor toxicity of GD2-chimeric antigen receptor T cells in patients with neuroblastoma. Sci Transl Med. (2020) 12:eabd6169. doi: 10.1126/scitranslmed.abd6169
115. Jamali A, Hadjati J, Madjd Z, Mirzaei HR, Thalheimer FB, Agarwal S, et al. Highly efficient generation of transgenically augmented CAR NK cells overexpressing CXCR4. Front Immunol. (2020) 11:2028. doi: 10.3389/fimmu.2020.02028
116. Seidel D, Shibina A, Siebert N, Wels WS, Reynolds CP, Huebener N, et al. Disialoganglioside-specific human natural killer cells are effective against drug-resistant neuroblastoma. Cancer Immunol Immunother. (2015) 64:621–34. doi: 10.1007/s00262-015-1669-5
117. Kailayangiri S, Altvater B, Spurny C, Jamitzky S, Schelhaas S, Jacobs AH, et al. Targeting Ewing sarcoma with activated and GD2-specific chimeric antigen receptor-engineered human NK cells induces upregulation of immune-inhibitory HLA-G. Oncoimmunology. (2017) 6:e1250050. doi: 10.1080/2162402X.2016.1250050
118. Klapdor R, Wang S, Morgan M, Dork T, Hacker U, Hillemanns P, et al. Characterization of a novel third-generation anti-CD24-CAR against ovarian cancer. Int J Mol Sci. (2019) 20:660. doi: 10.3390/ijms20030660
119. Lee YE, Ju A, Choi HW, Kim JC, Kim EE, Kim TS, et al. Rationally designed redirection of natural killer cells anchoring a cytotoxic ligand for pancreatic cancer treatment. J Control Release. (2020) 326:310–23. doi: 10.1016/j.jconrel.2020.07.016
120. Tseng HC, Xiong W, Badeti S, Yang Y, Ma M, Liu T, et al. Efficacy of anti-CD147 chimeric antigen receptors targeting hepatocellular carcinoma. Nat Commun. (2020) 11:4810. doi: 10.1038/s41467-020-18444-2
121. Lu Z, Peng Z, Liu C, Wang Z, Wang Y, Jiao X, et al. Current status and future perspective of immunotherapy in gastrointestinal cancers. Innovation (Camb). (2020) 1:100041. doi: 10.1016/j.xinn.2020.100041
122. Lin C, Zhang J. Reformation in chimeric antigen receptor based cancer immunotherapy: Redirecting natural killer cell. Biochim Biophys Acta Rev Cancer. (2018) 1869:200–15. doi: 10.1016/j.bbcan.2018.01.005
123. Xie G, Dong H, Liang Y, Ham JD, Rizwan R, Chen J. CAR-NK cells: A promising cellular immunotherapy for cancer. EBioMedicine. (2020) 59:102975. doi: 10.1016/j.ebiom.2020.102975
124. Wrona E, Borowiec M, Potemski P. CAR-NK cells in the treatment of solid tumors. Int J Mol Sci. (2021) 22:5899. doi: 10.3390/ijms22115899
125. Balatsoukas A, Rossignoli F, Shah K. NK cells in the brain: implications for brain tumor development and therapy. Trends Mol Med. (2022) 28:194–209. doi: 10.1016/j.molmed.2021.12.008
126. Lupo KB, Matosevic S. Natural killer cells as allogeneic effectors in adoptive cancer immunotherapy. Cancers (Basel). (2019) 11:769. doi: 10.3390/cancers11060769
127. Yu RK, Tsai YT, Ariga T, Yanagisawa M. Structures, biosynthesis, and functions of gangliosides–an overview. J Oleo Sci. (2011) 60:537–44. doi: 10.5650/jos.60.537
128. de Billy E, Pellegrino M, Orlando D, Pericoli G, Ferretti R, Businaro P, et al. Dual IGF1R/IR inhibitors in combination with GD2-CAR T-cells display a potent anti-tumor activity in diffuse midline glioma H3K27M-mutant. Neuro Oncol. (2022) 24:1150–63. doi: 10.1093/neuonc/noab300
129. Wroblewski JM, Bixby DL, Borowski C, Yannelli JR. Characterization of human non-small cell lung cancer (NSCLC) cell lines for expression of MHC, co-stimulatory molecules and tumor-associated antigens. Lung Cancer. (2001) 33:181–94. doi: 10.1016/S0169-5002(01)00210-0
130. Cao X, Li W, Yu Y, Liu T, Zhou Y. China enters CAR-T cell therapy era. Innovation (Camb). (2022) 3:100197. doi: 10.1016/j.xinn.2021.100197
131. Bergaggio E, Tai WT, Aroldi A, Mecca C, Landoni E, Nuesch M, et al. ALK inhibitors increase ALK expression and sensitize neuroblastoma cells to ALK.CAR-T cells. Cancer Cell. (2023) 41:2100–16.e10. doi: 10.1016/j.ccell.2023.11.004
132. Lynn RC, Weber EW, Sotillo E, Gennert D, Xu P, Good Z, et al. c-Jun overexpression in CAR T cells induces exhaustion resistance. Nature. (2019) 576:293–300. doi: 10.1038/s41586-019-1805-z
133. Chen J, Qiu S, Li W, Wang K, Zhang Y, Yang H, et al. Tuning charge density of chimeric antigen receptor optimizes tonic signaling and CAR-T cell fitness. Cell Res. (2023) 33:341–54. doi: 10.1038/s41422-023-00789-0
134. Mueller KP, Piscopo NJ, Forsberg MH, Saraspe LA, Das A, Russell B, et al. Production and characterization of virus-free, CRISPR-CAR T cells capable of inducing solid tumor regression. J Immunother Cancer. (2022) 10:e004446. doi: 10.1136/jitc-2021-004446
135. Lyu L, Feng Y, Chen X, Hu Y. The global chimeric antigen receptor T (CAR-T) cell therapy patent landscape. Nat Biotechnol. (2020) 38:1387–94. doi: 10.1038/s41587-020-00749-8
136. Balke-Want H, Keerthi V, Gkitsas N, Mancini AG, Kurgan GL, Fowler C, et al. Homology-independent targeted insertion (HITI) enables guided CAR knock-in and efficient clinical scale CAR-T cell manufacturing. Mol Cancer. (2023) 22:100. doi: 10.1186/s12943-023-01799-7
137. Rudek LS, Zimmermann K, Galla M, Meyer J, Kuehle J, Stamopoulou A, et al. Generation of an NFkappaB-driven alpharetroviral “All-in-one” Vector construct as a potent tool for CAR NK cell therapy. Front Immunol. (2021) 12:751138. doi: 10.3389/fimmu.2021.751138
138. Sait S, Modak S. Anti-GD2 immunotherapy for neuroblastoma. Expert Rev Anticancer Ther. (2017) 17:889–904. doi: 10.1080/14737140.2017.1364995
139. Cheung NK, Guo HF, Heller G, Cheung IY. Induction of Ab3 and Ab3’ antibody was associated with long-term survival after anti-G(D2) antibody therapy of stage 4 neuroblastoma. Clin Cancer Res. (2000) 6:2653–60.
140. Foon KA, Yannelli J, Bhattacharya-Chatterjee M. Colorectal cancer as a model for immunotherapy. Clin Cancer Res. (1999) 5:225–36.
141. Ladjemi MZ, Chardes T, Corgnac S, Garambois V, Morisseau S, Robert B, et al. Vaccination with human anti-trastuzumab anti-idiotype scFv reverses HER2 immunological tolerance and induces tumor immunity in MMTV.f.huHER2(Fo5) mice. Breast Cancer Res. (2011) 13:R17. doi: 10.1186/bcr2826
142. Ladjemi MZ. Anti-idiotypic antibodies as cancer vaccines: achievements and future improvements. Front Oncol. (2012) 2:158. doi: 10.3389/fonc.2012.00158
143. Foon KA, Lutzky J, Baral RN, Yannelli JR, Hutchins L, Teitelbaum A, et al. Clinical and immune responses in advanced melanoma patients immunized with an anti-idiotype antibody mimicking disialoganglioside GD2. J Clin Oncol. (2000) 18:376–84. doi: 10.1200/JCO.2000.18.2.376
144. Kushner BH, Cheung IY, Modak S, Kramer K, Ragupathi G, Cheung NK. Phase I trial of a bivalent gangliosides vaccine in combination with beta-glucan for high-risk neuroblastoma in second or later remission. Clin Cancer Res. (2014) 20:1375–82. doi: 10.1158/1078-0432.CCR-13-1012
145. Cheung IY, Cheung NV, Modak S, Mauguen A, Feng Y, Basu E, et al. Survival impact of anti-GD2 antibody response in a phase II ganglioside vaccine trial among patients with high-risk neuroblastoma with prior disease progression. J Clin Oncol. (2021) 39:215–26. doi: 10.1200/JCO.20.01892
146. Zhang P, Meng J, Li Y, Yang C, Hou Y, Tang W, et al. Nanotechnology-enhanced immunotherapy for metastatic cancer. Innovation (Camb). (2021) 2:100174. doi: 10.1016/j.xinn.2021.100174
147. Gabizon A, Martin F. Polyethylene glycol-coated (pegylated) liposomal doxorubicin. Rationale for use in solid tumours. Drugs. (1997) 54 Suppl 4:15–21. doi: 10.2165/00003495-199700544-00005
148. Papahadjopoulos D, Allen TM, Gabizon A, Mayhew E, Matthay K, Huang SK, et al. Sterically stabilized liposomes: improvements in pharmacokinetics and antitumor therapeutic efficacy. Proc Natl Acad Sci USA. (1991) 88:11460–4. doi: 10.1073/pnas.88.24.11460
149. Tivnan A, Orr WS, Gubala V, Nooney R, Williams DE, McDonagh C, et al. Inhibition of neuroblastoma tumor growth by targeted delivery of microRNA-34a using anti-disialoganglioside GD2 coated nanoparticles. PloS One. (2012) 7:e38129. doi: 10.1371/journal.pone.0038129
150. Cortez-Retamozo V, Backmann N, Senter PD, Wernery U, De Baetselier P, Muyldermans S, et al. Efficient cancer therapy with a nanobody-based conjugate. Cancer Res. (2004) 64:2853–7. doi: 10.1158/0008-5472.CAN-03-3935
151. Ellington AD, Szostak JW. Selection in vitro of single-stranded DNA molecules that fold into specific ligand-binding structures. Nature. (1992) 355:850–2. doi: 10.1038/355850a0
152. Wang M, Lowik DW, Miller AD, Thanou M. Targeting the urokinase plasminogen activator receptor with synthetic self-assembly nanoparticles. Bioconjug Chem. (2009) 20:32–40. doi: 10.1021/bc8001908
153. Goren D, Horowitz AT, Tzemach D, Tarshish M, Zalipsky S, Gabizon A. Nuclear delivery of doxorubicin via folate-targeted liposomes with bypass of multidrug-resistance efflux pump. Clin Cancer Res. (2000) 6:1949–57.
154. Adrian JE, Wolf A, Steinbach A, Rossler J, Suss R. Targeted delivery to neuroblastoma of novel siRNA-anti-GD2-liposomes prepared by dual asymmetric centrifugation and sterol-based post-insertion method. Pharm Res. (2011) 28:2261–72. doi: 10.1007/s11095-011-0457-y
Keywords: retinoblastoma, GD2, anti-GD2 monoclonal antibody, GD2-CAR cell therapy, immunotherapy
Citation: Zhang X, You W, Wang Y, Dejenie R, Wang C, Huang Y and Li J (2024) Prospects of anti-GD2 immunotherapy for retinoblastoma. Front. Immunol. 15:1499700. doi: 10.3389/fimmu.2024.1499700
Received: 21 September 2024; Accepted: 21 October 2024;
Published: 15 November 2024.
Edited by:
Haitao Wang, National Cancer Institute (NIH), United StatesReviewed by:
Longhao Li, Chongqing Medical University, ChinaCopyright © 2024 Zhang, You, Wang, Dejenie, Wang, Huang and Li. This is an open-access article distributed under the terms of the Creative Commons Attribution License (CC BY). The use, distribution or reproduction in other forums is permitted, provided the original author(s) and the copyright owner(s) are credited and that the original publication in this journal is cited, in accordance with accepted academic practice. No use, distribution or reproduction is permitted which does not comply with these terms.
*Correspondence: Jingjing Li, amluZ2ppbmdsaS5tZC5waGRAZ21haWwuY29t; Yan Huang, aHlhbjU2MTlAZ21haWwuY29t
†These authors share first authorship
Disclaimer: All claims expressed in this article are solely those of the authors and do not necessarily represent those of their affiliated organizations, or those of the publisher, the editors and the reviewers. Any product that may be evaluated in this article or claim that may be made by its manufacturer is not guaranteed or endorsed by the publisher.
Research integrity at Frontiers
Learn more about the work of our research integrity team to safeguard the quality of each article we publish.