- 1Institute of Forensic Medicine and Laboratory Medicine, Jining Medical University, Jining, China
- 2College of Medical Engineering, Jining Medical University, Jining, China
The defense mechanisms of the vertebrate brain against infections are at the forefront of immunological studies. Unlike other body parts, the brain not only fends off pathogenic infections but also minimizes the risk of self-damage from immune cell induced inflammation. Some neuropeptides produced by either nerve or immune cells share remarkable similarities with antimicrobial peptides (AMPs) in terms of size, structure, amino acid composition, amphiphilicity, and net cationic charge. These similarities extend to a wide range of antibacterial activities demonstrated in vitro, effectively protecting nerve tissue from microbial threats. This review systematically examines 12 neuropeptides, pituitary adenylate cyclase-activating peptide (PACAP), vasoactive intestinal peptide (VIP), α-melanocyte stimulating hormone (α-MSH), orexin-B (ORXB), ghrelin, substance P (SP), adrenomedullin (AM), calcitonin-gene related peptide (CGRP), urocortin-II (UCN II), neuropeptide Y (NPY), NDA-1, and catestatin (CST), identified for their antimicrobial properties, summarizing their structural features, antimicrobial effectiveness, and action mechanisms. Importantly, the majority of these antimicrobial neuropeptides (9 out of 12) also possess significant anti-inflammatory properties, potentially playing a key role in preserving immune tolerance in various disorders. However, the connection between this anti-inflammatory property and the brain’s infection defense strategy has rarely been explored. Our review suggests that the combined antimicrobial and anti-inflammatory actions of neuropeptides could be integral to the brain’s defense strategy against pathogens, marking an exciting direction for future research.
1 Introduction
Currently, the immune response mechanism of the vertebrate brain remains poorly understood (1, 2). Bacterial, fungal or viral infections in brain tissue are extremely rare due to the presence of the blood−brain barrier (BBB), which offers strong defense against blood-borne pathogens (2, 3). The hypothalamus and pituitary stalk are exceptions to the BBB. Infections in this region, however, are highly uncommon. It is possible that the brain possesses an undiscovered layer of immune defense (2).
Recent studies have indicated the potential role of neuropeptides in regulating the immune response and neuroinflammation (4, 5). They have direct anti-infective properties, protecting nerve tissue from microbial invasion (6). AMPs or host defense peptides, are short proteins found in various living organisms (7). AMPs serve as the host’s primary defense against pathogens and possess the ability to stimulate the innate immune response (8). Many neuropeptides share similarities with AMPs in terms of size, structure, amino acid composition, amphiphilicity, and net cationic charge. In vitro studies have shown that certain neuropeptides possess antimicrobial activity (9). The presence of this antimicrobial activity suggests its potential involvement in the innate immune response.
Furthermore, specific neuropeptides synthesized by nerve cells (10) (such as astrocytes and sertoli cells) or immune cells (including lymphocytes, neutrophils, and mast cells) exhibit potent anti-inflammatory effects and actively contribute to the regulation of immune tolerance in various immune disorders (11). It has been reported that immune cells possess receptors for neuropeptides, which confirms the involvement of neuropeptides in immune regulation (12). In response to various invasive and inflammatory stimuli, neuropeptides can inhibit the expression of proinflammatory cytokines (11). Furthermore, they can exert immunomodulatory effects by modulating the balance between effector T cells and regulatory T cells, suppressing inflammation, and maintaining immune tolerance (13). These findings highlight neuropeptides as promising therapeutic candidates for treating autoimmune diseases and inflammatory disorders (11).
Recent studies have revealed the potential role of neuropeptides with antimicrobial (14) and anti-inflammatory (13) properties in the brain’s defense against pathogens. In this review, we comprehensively explore the structural properties and antimicrobial activities of neuropeptides, providing a thorough summary of their antimicrobial effects against various microorganisms, including gram-positive bacteria, gram-negative bacteria, fungi, parasites, and viruses. Additionally, we comprehensively evaluated the immunomodulatory activity of these neuropeptides and their therapeutic potential. The selective utilization of the antimicrobial and immunomodulatory properties of these neuropeptides holds promise for developing a potential therapeutic approach, offering a novel and effective treatment strategy for CNS infectious disease (11).
2 Antimicrobial mechanism of AMPs
Antimicrobial neuropeptides represent a unique class of AMPs that possess both neural and antimicrobial properties (1). In this section, we mainly introduce the structural characteristics and antimicrobial mechanism of AMPs to enhance our understanding of antimicrobial neuropeptides.
2.1 Structural characteristics of AMPs
The structural diversity of AMPs allows them to adopt different secondary structures, enabling them to employ unique mechanisms for targeting pathogens (15). AMPs exhibit structural variability that is mainly determined by the cell of the peptide source (16). Understanding the structural characteristics of AMPs is essential for further investigation into their antimicrobial mechanisms. Typically, AMPs consist of 10-50 amino acids and have a molecular weight less than 10 kDa (17). The antimicrobial activity of AMPs is influenced by various physicochemical properties, including amino acid composition, peptide length, presence of positively charged residues, lipid composition, hydrophobic characteristics, net molecular charge, and helicity of spatial structure (18).
According to the structural model of nearly 900 AMPs, natural AMPs can be classified into four major families: α, β, αβ, and non-αβ (Figure 1) (19). Among these families, α-helix and β-sheet structures are the most commonly observed in AMPs found in nature.
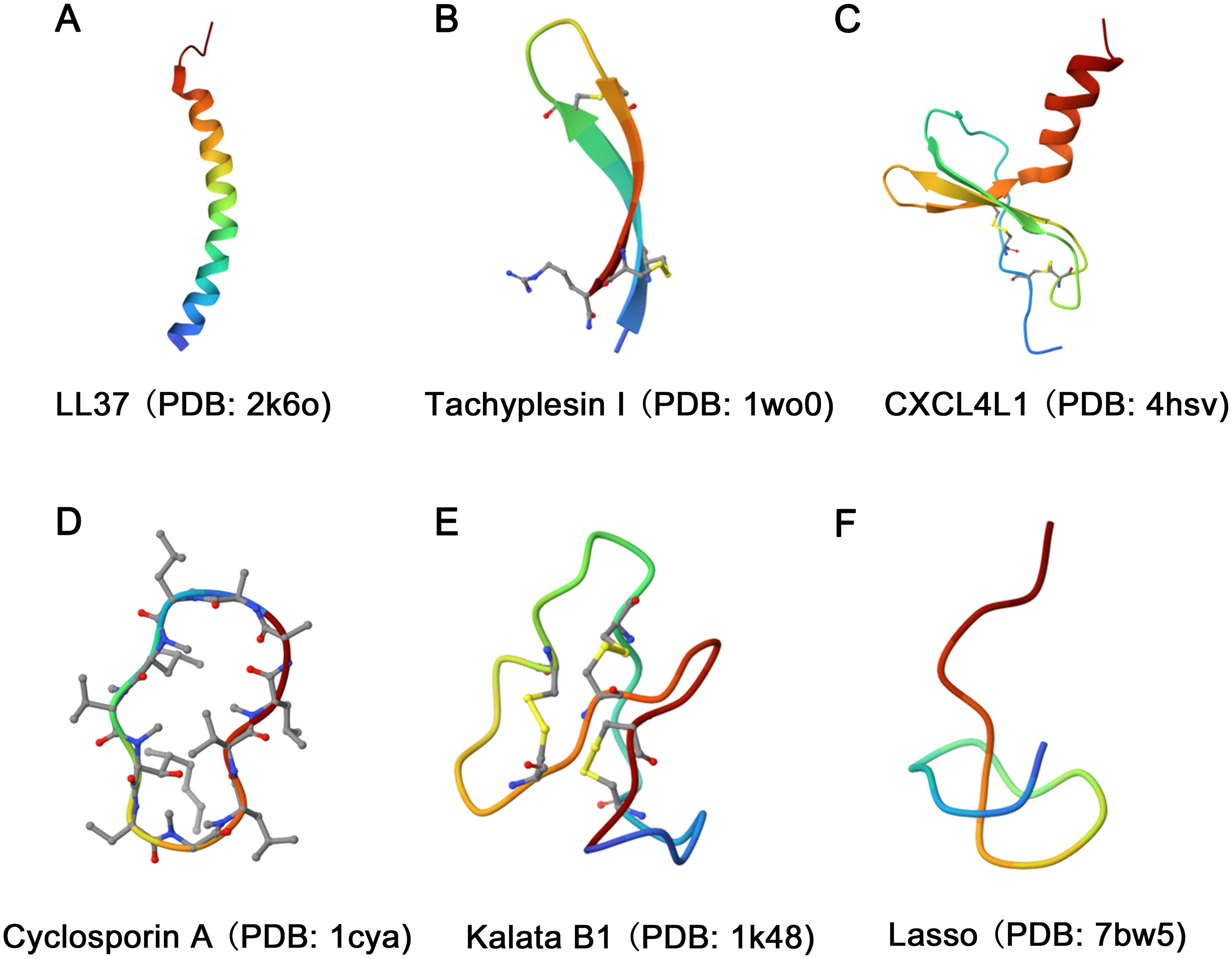
Figure 1. Diversity in the structural characteristics of AMPs. (A) The structure of LL37 (PDB ID: 2k6o) is characterized by an α-helix conformation. (B) Tachyplesin I (PDB ID: 1wo0) has a typical β-sheet conformation. (C) The structure of CXCL4L1 (PDB ID: 4hsv) is classified as an αβ family. (D, E) The structures of Cyclosporin A (PDB ID: 1cya) and Kalata B1 (PDB ID: 1k48) were characterized as loop. (F) The Lasso (PDB ID: 7bw5) structure exhibits random coiling.
The α family of AMPs primarily adopts a linear α-helix conformation as their dominant secondary structure (Figure 1A). However, it is important to note that both His-rich and Trp-rich peptides have the potential to form α-helix structures, causing some overlap between classes. Examples of α family AMPs include lactoferricin B, human antimicrobial peptide LL-37, and pituitary adenylate cyclase-activating peptide (PACAP), among others. The β family is characterized by the presence of at least two β chains arranged in a specific structural pattern (20), with cysteine stability and β-folding (Figure 1B). Human α-defensins and tachyplesin I are examples of AMPs that adopt this structure. The αβ family of AMPs includes both α-helical and β-sheet conformations (Figure 1C). Relevant examples include β-defensins, β-Amyloid (Aβ), CXCL4L1, antimicrobial chemokines, and RNases. Finally, the non-αβ family of AMPs lacks both α-helix and β-sheet structures. However, this family exhibits an extensive secondary structure, including loop peptides (Figures 1D, E) and random coils (Figure 1F) (21).
2.2 Antimicrobial mechanism of AMPs
AMPs are vital components of the innate immune system and possess strong antibacterial, antifungal, antiparasitic, and antiviral activities (22). Moreover, AMPs play a crucial role in various intracellular processes, such as angiogenesis, arteriogenesis, inflammatory response modulation, cell signal transduction, and the wound healing cascade (23). Numerous mechanisms of action for AMPs have been proposed, but the primary mode of action for most AMPs is the destruction of pathogenic microorganisms by damaging their cell membranes, also known as membrane damage mechanism (24). Antimicrobial neuropeptides also firstly employ this mechanism, similar to that of conventional cationic AMPs, to combat microorganisms. The process can be summarized as follows: (1) Positively charged neuropeptides bind to the negatively charged surfaces of microbes through electrostatic interactions; (2) This binding destabilizes the negatively charged phospholipid bilayer, leading to membrane damage; (3) Membrane permeability is altered; and (4) Microorganisms die due to hypotonicit (25). The membrane damage mechanism of AMPs primarily includes four types: Barrel-stave mode, Toroidal-pore mode, Carpet mode and Aggregate mode. The Barrel-stave model (Figure 2A) (26) begins with the incorporation of AMPs into the phospholipid bilayer in three possible orientations: parallel, vertical, or inclined. When the peptide/lipid ratio reaches a certain threshold, resulting in energetical and physical changes in the membrane structure, including the helical hydrophobic regions of the peptides are close to the hydrophobic regions of the membrane phospholipid, while the hydrophilic regions of the peptide are inwards (27, 28). This alignment of helical molecules generated a central lumen, establishing the Barrel-stave model. The Toroidal-pore model (Figure 2B) is similar to a transmembrane ion channel and induces bending of the phospholipid bilayer when AMPs accumulate to a certain level. The peptides spiral into the membrane, bind to the lipids, and form a porous ring complex, eliminating the need to span the complete phospholipid bilayer (28). The Carpet model, similar to that of detergent (Figure 2C), involves the continuous accumulation of AMPs. When their concentration reaches a threshold, clusters of AMPs cover the phospholipid bilayer, resulting in membrane disruption akin to detergent action without channel formation (18). The Aggregate model (Figure 2D) facilitates the formation of peptide-lipid complexes, ultimately leading to ion leakage channels and cellular death (18). The permeability of peptides across membranes is directly influenced by their topological amphiphilic structure rather than by their linear structure, as demonstrated by numerous studies. As a result, the charge is systematically distributed in regular clusters across the polypeptide surface, which forms the basis of its antimicrobial efficacy (29).
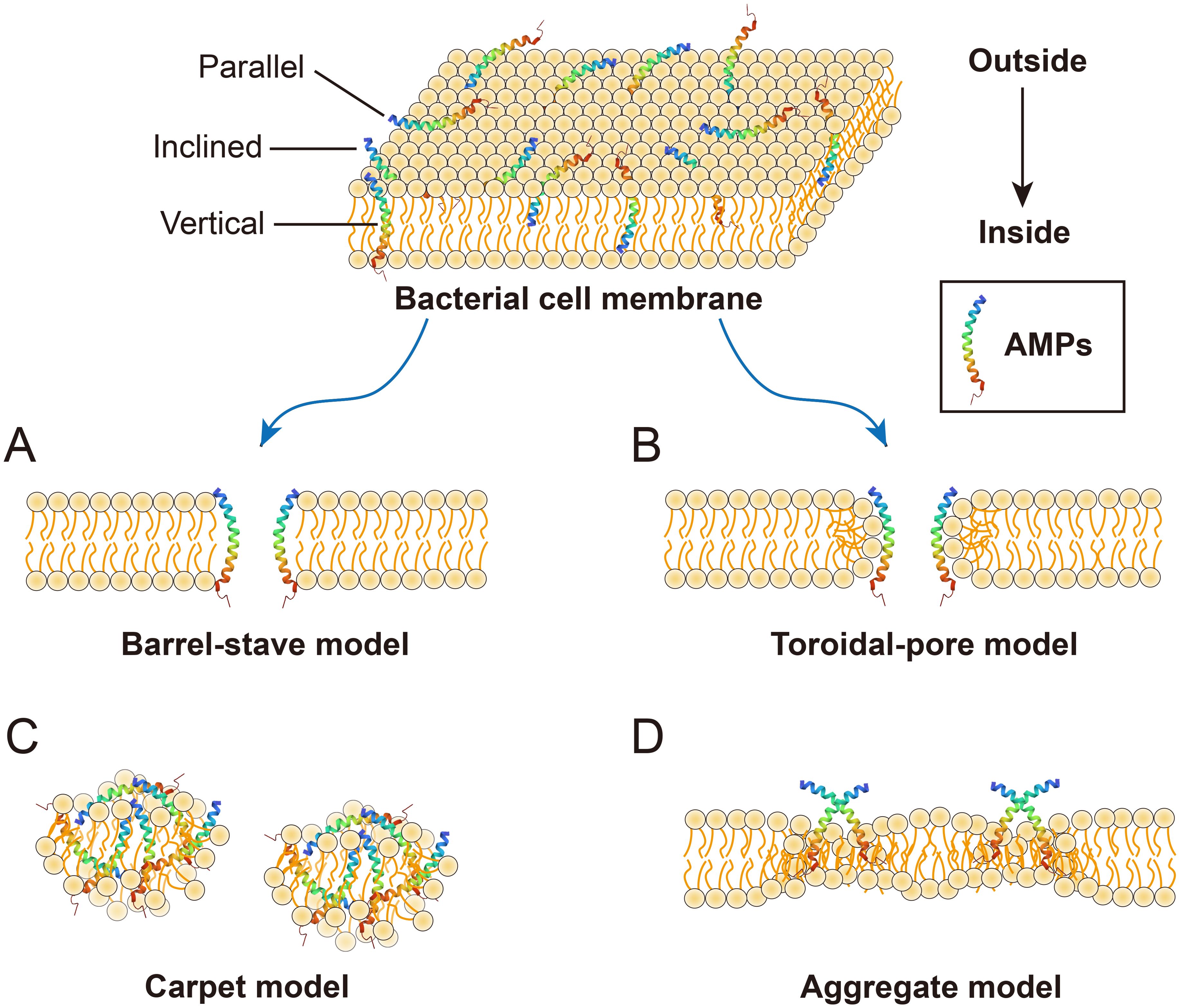
Figure 2. Membrane damage models of AMPs. (A) Barrel-septal mode: multiple helical molecules are arranged in parallel to form the central lumen; (B) Toroldal-pore mode: it is similar to the Barrel-septal model except that toroidal pore complexes do not need to span the full double layer; (C) The carpet model peptide: AMPs cover the membrane in clusters and cause membrane rupture in a surfactant-like manner; (D) Aggregate mode: this pattern facilitates the formation of channels, enabling the leakage of ions and intracellular contents, thereby inducing cell death.
Currently, there is a growing emphasis on investigating the impact of AMPs in inhibiting or eradicating biofilms (30). Unlike phospholipid bilayer membranes, biofilms exist in a rootless form in nature (31). In 1999, Costerton et al. (32) introduced the concept of biofilms, which are structural communities of bacteria, fungi, and viruses adhered to any biotic surface enveloped by a self-produced polymer matrix consisting of proteins, exopolysaccharides, DNA, lipids, and other fragments. In general, a biofilm is an assemblage of organisms formed by the aggregation of microbial cells and matrix (33). The typical biofilm formation process primarily includes four sequential stages: adhesion, proliferation, maturation, and dispersal (34, 35) (Figure 3A). Initially, bacteria adhere to the surface, and as their population proliferates, they secrete extracellular polysaccharides, thereby establishing a robust biofilm matrix. Subsequently, the cells continue to grow and divide, facilitating the subsequent detachment and dissemination of the bacteria (36). The effects of AMPs on biofilms primarily include inhibiting the formation and adhesion of biofilms, eradicating preformed biofilms, and impeding biofilm propagation and detachment. However, the mechanism of action of AMPs on biofilms varies across different periods and can be categorized into the following five hypothetical mechanisms (Figure 3B): (1) The rapid destruction of biofilm-embedded cells indicates that AMPs act by membrane damage of the bacteria (34). (2) Disruption of quorum sensing signaling: AMPs increase the twisting movement of bacteria on the surface of the biofilm by stimulating type IV Pili-mediated pulling motion, down-regulating the transcription of Las and Rhl in the induction system (37), and down-regulating the genes that migrate and transport binding proteins from extrachromosomal elements to inhibit transporter expression (38), thereby repressing the formation of communal biofilms (34). (3) Repression of the alarm system to mitigate biofilm resistance against AMPs, thereby preventing strict bacterial response (39). (4) Destruction of biofilm potential: after the release of bacteriocin, which disrupts the biofilm matrix, ATP is released, thereby enhancing the permeability of the biofilm and eradicating preexisting biofilms, ultimately leading to bacterial cell death (40). (5) Degradation of the polysaccharide and biofilm matrix. Certain enzymatically active AMPs, such as piscidin-3 exhibit nucleosidase activity capable of impairing extracellular DNA (eDNA) of Pseudomonas aeruginosa (39). Peptide PI can degrade the extracellular polymeric substances (EPS) produced by Streptococcus mutans, leading to reduced biofilm formation (34). Furthermore, certain non-enzymatically active AMPs, such as hepcidin 20, exhibit the ability to modulate the extracellular matrix integrity by specifically targeting polysaccharide intercellular adhesin (PIA) and inducing structural alterations within the biofilm (34).
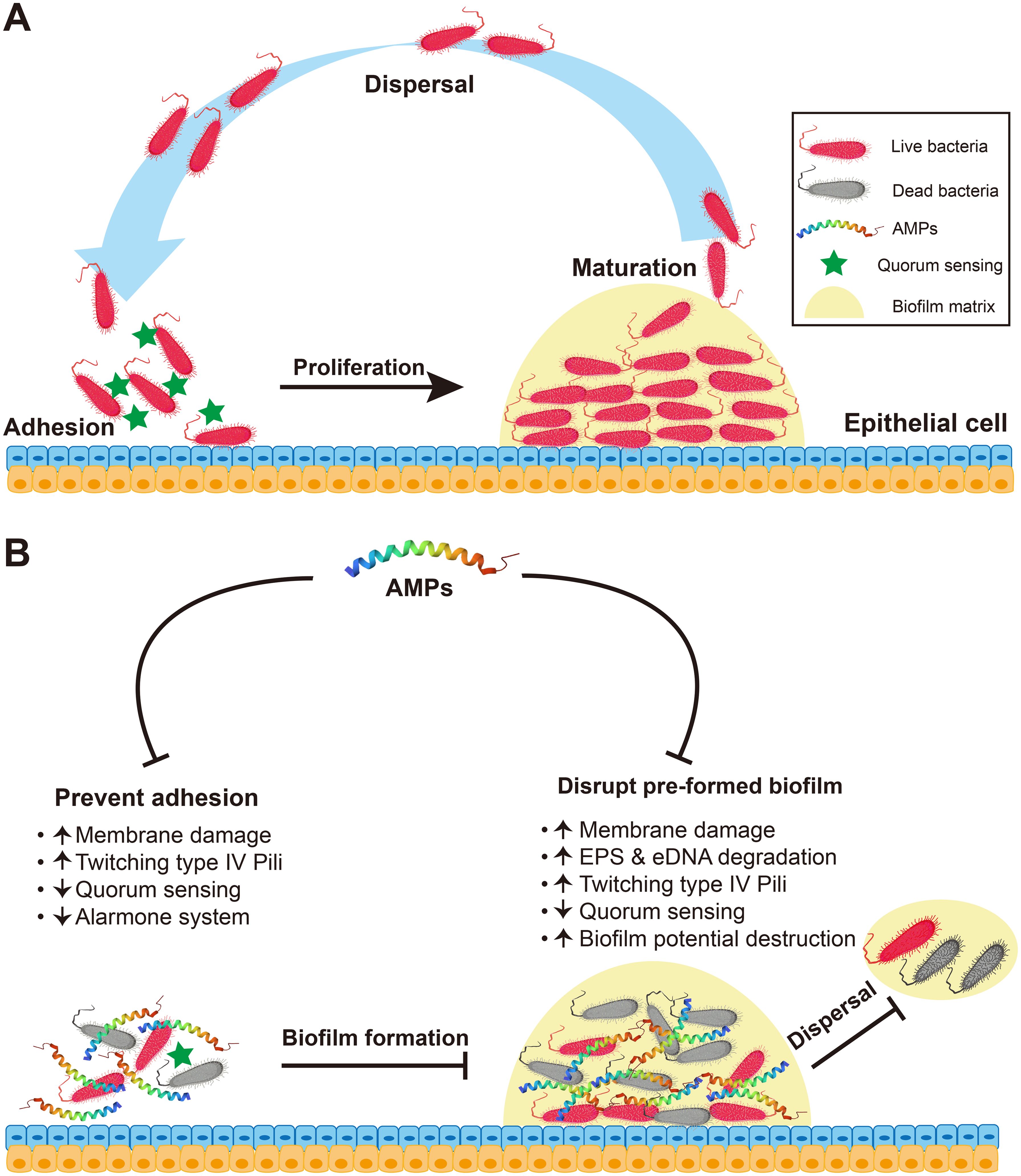
Figure 3. Antibiofilm mechanism of AMPs. (A) The process of biofilm formation involves the stages of adhesion, proliferation, maturation and dispersal. (B) Antibiofilm mechanism of AMPs. On the one hand, in the inhibition of bacterial adhesion and biofilm formation, AMPs can directly kill preattached pathogenic microorganisms via membrane damage; enhance type IV pili-mediated pulling movement, thereby accelerating bacterial twisting on surfaces and effectively inhibiting bacterial adhesion and biofilm formation; down-regulating the transcription of genes associated with quorum sensing (e.g., Las, Rhl); and suppress the alarm system to avoid biofilm resistance to AMPs. On the other hand, AMPs can kill preformed biofilms. In addition to causing membrane damage, enhancing type IV pili-mediated pulling movement and down-regulating the quorum sensing, AMPs can enhance the degradation of the synthetic components of biofilm EPS and eDNA and lead to the destruction or degradation of the membrane potential enclosed by a biofilm.
In addition to membrane damage and antibiofilm mechanisms, AMPs can also exert their antimicrobial effects through various pathways. First, AMPs can regulate the expression of genes involved in cell wall synthesis, thereby inhibiting this process and exhibiting antibacterial activity (41). Furthermore, AMPs can target peptidoglycan, which is the primary constituent of the bacterial cell wall (42). After the inwards growth of the cell wall and the formation of a transverse cross wall (septum), the newly synthesized peptidoglycan undergoes hydrolysis during cell division under the action of AMP, ultimately resulting in bacterial death (42). Additionally, AMPs possess endotoxin-neutralizing properties that enhance innate immunity and effectively exert antimicrobial effects (43). Finally, AMPs exert their antimicrobial effect by impeding or terminating the translation process, thereby inhibiting intracellular nucleic acid and protein synthesis through complex mechanisms (44–46).
Compared to conventional antibiotics that only target a single site, AMPs possess multiple targets, enabling them to eliminate pathogens from various directions, thereby significantly reducing the emergence of antibiotic-resistant bacteria (47). Resistance to AMPs is more difficult than resistance to antibiotics, and the therapeutic mechanisms employed against drug-resistant bacterial infections can be categorized into the following five different approaches: biofilm penetration, re-sensitization, intracellular bacteriostatic function, immune activity regulation, and biofilm inhibition (47).
3 Neuropeptides with antimicrobial activity
Numerous studies have consistently demonstrated the direct antimicrobial effects of neuropeptides in vitro, substantiating their established role as antimicrobial agents. These neuropeptides include PACAP, vasoactive intestinal peptide (VIP), α-melanocyte stimulating hormone (α-MSH), orexin-B (ORXB), ghrelin, substance P (SP), adrenomedullin (AM), calcitonin-gene related peptide (CGRP), urocortin-II (UCN II), neuropeptide Y (NPY), NDA-1, and catestatin (CST) (Table 1). In addition, most of them can be secreted by immune cells and play an immunomodulatory role (Figure 4).
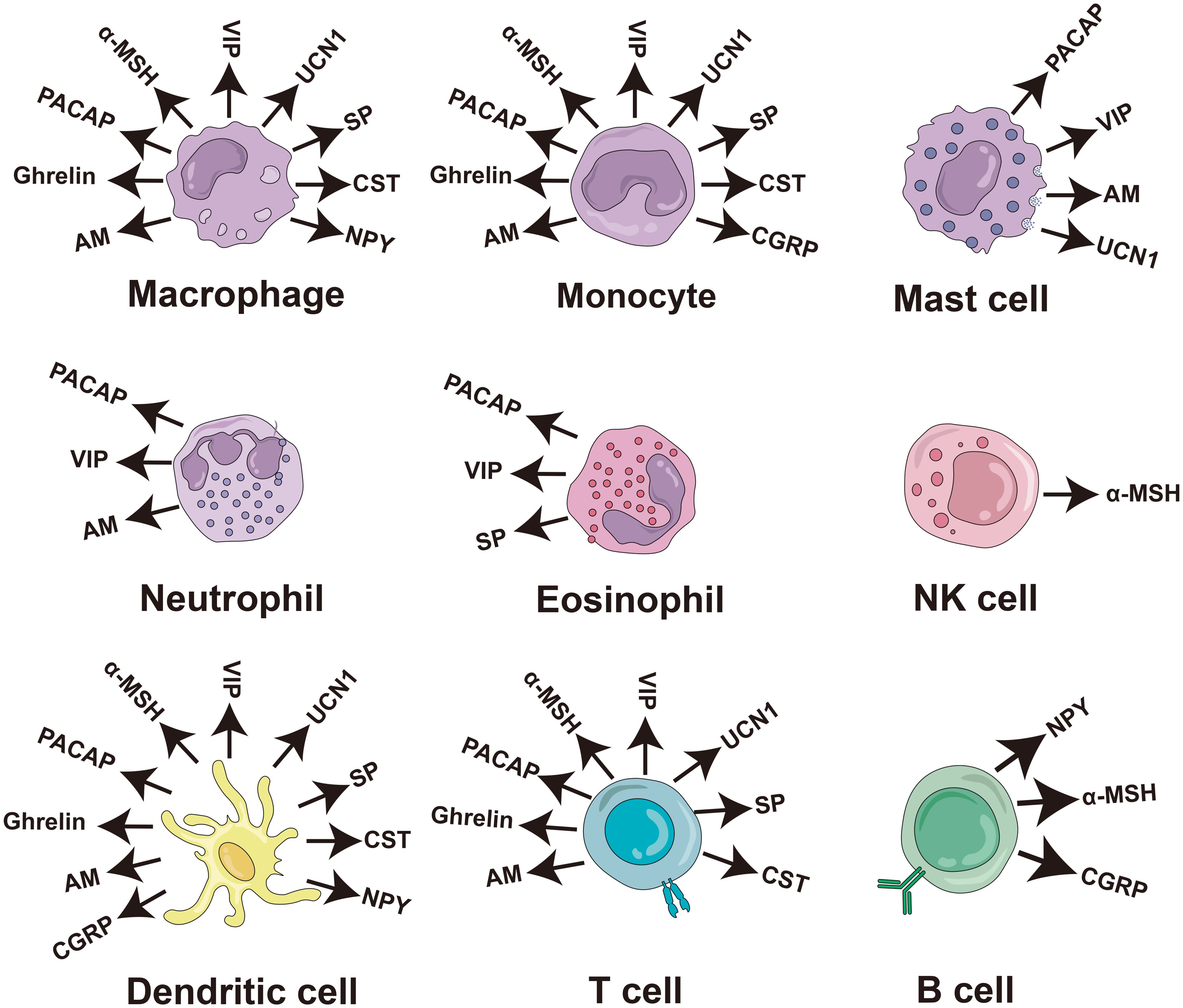
Figure 4. Production of antimicrobial neuropeptides by cells of the human immune system. The diagram of the immune cells was refined based on Krause’s work (48). PACAP, pituitary adenylate cyclase-activating peptide; VIP, vasoactive intestinal peptide; α-MSH, α-melanocyte stimulating hormone; SP, substance P; AM, adrenomedullin; CGRP, calciton-in-gene related peptide; UCN II, urocortin-II; NPY, neuropeptide Y; CST, catestatin; NK cell, natural killer cell.
3.1 Pituitary adenylate cyclase activating peptide (PACAP)
In 1989, Miyata et al. (100) successfully isolated PACAP from sheep hypothalamic tissue and searched for peptides that can stimulate the secretion of pituitary hormones. PACAP, an α-helical peptide, belongs to the VIP/secretin/glucagon superfamily and exhibits 68% sequence similarity with VIP (51). PACAP exists in two amidated forms, PACAP27 and PACAP38, with PACAP38 being the predominant form (101). It is mainly found in thymocytes, lymphocytes, and plasma cells of the spleen and lymph nodes in the immune system (102), and it has potent anti-inflammatory effects (49).
In 2021, Lee et al. (1) conducted a comparative study of the human neuropeptide and AMP databases using bioinformatics methods. They discovered that PACAP38 showed potential as an AMP, sharing similarities with the known AMP LL37 in terms of secondary structure, amino acid composition, and average hydrophobicity. Subsequently, Lee et al. (1) employed X-ray scattering techniques to demonstrate that PACAP38 can penetrate the bacterial plasma membrane, causing membrane damage. However, it had no effect on mammalian cell membranes. Notably, PACAP38 exhibited significant antimicrobial activity against the gram-positive bacterium Bacillus subtilis (minimum inhibitory concentration, MIC: 5 µM), the fungus Candida albicans (MIC: 75 µM), and the cancer cell line H460 (half maximal inhibitory concentration, IC50: 14.97 ± 1.16 µM) (52). Furthermore, PACAP38 exhibited antiviral effects by stimulating the synthesis and release of the β-chemokines CCL3 and CCL5, thereby inhibiting HIV-1 replication (50).
3.2 Vasoactive intestinal peptide (VIP)
VIP was initially isolated from the pig duodenum by Said et al. (103). It is a 3.3 kDa polypeptide composed of 28 amino acids (104). The structure of VIPs can change in response to variations in the surrounding environment (105). According to previous studies, approximately 27% of VIPs adopt an α-helix conformation, whereas 27.2% adopt a β-sheet structure, and the remaining 45.8% is characterized as randomly coiled, but it appears to be an α-helix when bound to a lipid or anion (56). VIP is found in two sources within the immune system: terminals present in central and peripheral lymphoid organs and immune cells, mostly lymphocytes. VIP plays an anti-inflammatory role in immune regulation (53).
VIP exerts its antibacterial function through the membrane damage mechanism, although its efficacy against bacteria is more pronounced at a lower NaCl concentration compared to the physiological level of 150 mM (106). Moreover, the diminished antimicrobial activities of VIP in 150 mM NaCl can be restored by the addition of LL-37, suggesting that VIP may exhibit bactericidal effects in conjunction with LL-37 within the physiological milieu of mucosal tissue (107). Notable activity was observed against the gram-negative bacteria E. coli (MIC: 1.7 μM) and P. aeruginosa (MIC: 1.4 μM), as well as the fungus C. albicans (MIC: 15.6 µM) (55). Furthermore, VIP plays a significant role in combating parasites (54). Unlike the membrane damage mechanism commonly induced by AMPs, VIP is initially endocytosed by the parasite, leading to disruption of intracellular lysosome integrity and cytoplasmic glycolytic enzyme function, ultimately resulting in parasite death (54).
3.3 α-Melanocyte stimulating hormone (α-MSH)
α-MSH, which was originally discovered in the bovine pituitary gland, is a member of the melanocortin family (61, 108). It is a 13-amino acid polypeptide with a C-terminal amide derived from the enzymatic cleavage of a 36 kD precursor protein known as Proopiomelanocortin (POMC) (109). However, the secondary structure of α-MSH remains unknown. α-MSH is primarily produced by nerve terminals, blood, and various immune cells, such as TH2 cells and macrophages, and exhibits potent anti-inflammatory activity (11, 58).
α-MSH is an antimicrobial neuropeptide that exerts its antibacterial effect by inhibiting bacterial adhesion and molecular penetration during the early stages of infection (59), similar to the mechanism of antibiofilm action. It down-regulates the levels of β-1 integrin and HSP70, key molecules involved in Staphylococcus aureus invasion of keratinocytes, thereby exhibiting antibacterial activity (59). Moreover, α-MSH effectively permeabilizes the cell membrane of C. albicans, resulting in the release of intracellular contents and playing an antifungal role (110). Fjell et al. (60) demonstrated a potent anti-candidiasis effect of α-MSH, leading to its application in the treatment of vulvovaginal candidiasis and chronic respiratory infections, with promising results observed during phase II clinical trials. In terms of antiviral activity, α-MSH suppresses activation of the transcription factor NF-κB, which is known to enhance HIV expression (57).
3.4 Orexin-B (ORXB)
The neuropeptide ORXB, consisting of 28 amino acids (64), was initially discovered in a small group of neurons in the hypothalamus and is derived from the hydrolysis of a single precursor protein known as prepro-orexin (111). Orexin-A (ORXA) and ORXB are two isopeptides belonging to the orexin family (112). ORXB is the ancestral form and exhibits significant similarity to VIP in terms of its amphiphilic nature, isoelectric point, and net charge (106). Specifically, ORXB adopts a disordered conformation in aqueous solution and an α-helix structure in a simulated membrane environment (113). It is mainly expressed in neurons located in the lateral and dorsomedial hypothalamus (114) and can regulate the phagocytic function of macrophages in terms of immune regulation (62).
The antimicrobial efficacy of ORXB, similar to that of VIP, was significantly enhanced when it was coexposed to LL-37 at a physiological NaCl concentration of 150 mM (115). This enhancement can be attributed to the interaction between cationic LL-37 and amphiphilic ORXB, which facilitates the binding of ORXB to bacterial membranes and subsequently induces membrane damage (116). ORXB exhibits a broad range of antibacterial activities against both gram-negative bacteria (E. coli, Salmonella typhimurium, Klebsiella pneumoniae) and gram-positive bacteria (S. aureus) and demonstrates a strong antibacterial effect at concentrations greater than 25 μg/ml (66). In addition, ORXB has been found to have antiviral activity in inhibiting the infectivity of herpes simplex virus 1 (HSV-1), exceeding the IC50 value of 100 μg/mL (66).
3.5 Ghrelin
In 1999, Kojima et al. (117) first discovered that Ghrelin is a growth hormone-releasing acylated peptide composed of 28 amino acids secreted by the stomach, and its secondary structure is a putative α-helix (118, 119). Two forms of ghrelin have been identified, namely, acylated ghrelin (AG) and deacylated ghrelin (DAG) (70), with DAG found at higher circulating levels than the AG form (120). Ghrelin is primarily expressed in human peripheral T lymphocytes, B lymphocytes, and neutrophils (121) and exhibits significant anti-inflammatory effects (67).
Ghrelin also has significant antimicrobial effects (70) and exerts its antimicrobial effect primarily through a membrane damage mechanism (122). Moreover, ghrelin concentrations equal to or exceeding 12.5 μg/ml exhibit a significant bactericidal effect against gram-negative bacteria (E. coli, P. aeruginosa), whereas the bactericidal effects on gram-positive S. aureus and Enterococcus faecalis are minimal or absent (70). Additionally, ghrelin shows potent antiparasitic activity, such as lysis of parasites by pore formation and plasma membrane disruption, particularly in African trypanosomes (68).
3.6 Substance P (SP)
In 1931, SP was first identified in the brain and gut of horses by Euler et al. (123), followed by its isolation and subsequent determination of its amino acid sequence in the hypothalamus of cattle (124, 125). SP consists of 11 amino acid residues, and its secondary structure is primarily an α-helix structure (126) It belongs to the tachykinin family. SP is mainly secreted by neurons and can also be produced by inflammatory cells, such as macrophages and dendritic cells, where it promotes the inflammatory response and immune regulation (73).
Moreover, SP has been reported to possess significant antimicrobial activity. Two mechanisms have been identified: one is the acceleration of pathogen virulence factor production when exposed to SP (10 µM), which leads to cytotoxic effects and ultimately pathogen death (127, 128). The other mechanism is the induction of shedding of the S-layer (a barrier against AMPs) of Bacillus cereus (129), resulting in damage to cell membrane integrity and exerting its antimicrobial activity (128). SP has demonstrated good antimicrobial activity against the gram-negative bacterium E. coli (MIC: 5.7 µg/ml), gram-positive bacterium Acidophilus (MIC: 74.1 µg/ml), and fungus C. albicans (MIC: 8.1 µg/ml) (55, 115). In addition, SP can competitively bind to the measles virus (MV) receptor and neurokinin-1 receptor, thereby preventing infection of CD46+ neurons (130). In the fight against parasites, SP (10−8 M) reduces the adherence of Leishmania brasiliensis to macrophages, resulting in a repellent chemotactic effect (74).
3.7 Adrenomedullin (AM)
The 52-amino acid polypeptide AM belongs to the CGRP family (131, 132). It was initially extracted from adrenal medullary pheochromocytoma by Kitamura et al. (133) in 1993. The secondary structure of AM shows a conserved α-helical region from residues 21 to 33 (optimum antimicrobial activity), whereas the remaining residues do not consistently show ordered regions (42). AM can be synthesized by several immune cells, including macrophages, monocytes and T cells, as well as by lymphoid organs and the gastrointestinal tract (11). Its expression increases under inflammatory conditions, and exerts potent anti-inflammatory effects (11).
AM is also an antimicrobial neuropeptide (78) that functions mainly through a membrane damage mechanism (42). Moreover, AM induces bacterial death by disrupting the peptidoglycan in the cell wall (134). Notably, between 2003 and 2006, Allaker’s research team (42, 78, 135) observed that AM demonstrated equal sensitivity to the gram-negative bacteria E. coli (MIC: 0.06 µM), Haemophilus influenza (MIC: 2 µM), and the gram-positive bacteria S. aureus (MIC: 2 µM) and S. mutans (MIC: 2 µM) (78).
3.8 Calcitonin gene-related peptide (CGRP)
CGRP, a 37-amino acid neuropeptide, was initially discovered in human medullary carcinoma (81, 136). It belongs to the calcitonin superfamily and adopts an α-helix structure (83). There are two primary forms of CGRP: α-CGRP and β-CGRP. Compared with β-CGRP, α-CGRP is prevalent in both central and peripheral neurons and elicits a stronger immunogenic response (83). CGRP is primarily released from trigeminal ganglia cells and is widely expressed in immune cells, including dendritic cells, T cells, and macrophages (137). It exerts anti-inflammatory effects by modulating innate immune responses (82).
CGRP also has significant antimicrobial effects on various microorganisms found in the skin, respiratory tract, and other anatomical regions (55, 138). CGRP exerts its antimicrobial effect through membrane damage and antibiofilm mechanisms (139, 140), It exhibits considerable efficacy against gram-negative E. coli (MIC: 2.1 µg/ml), P. aeruginosa (MIC: 5.9 µg/ml), and the fungus C. albicans (MIC: 63.1 µg/ml), whereas its bactericidal effects on gram-positive bacteria are minimal (55, 115).
3.9 Urocortin-II (UCN II)
Urocortins (UCNs), including UCN I, UCN II, UCN III, Urotensin 1 (found only in fishes), and Sauvagine (found only in amphibians) (141), were initially discovered by Reyes et al. (142) in 2001. UCN II, composed of 38 amino acid residues, has an α-helix structure and possesses amphiphilic properties (89). It belongs to the corticotropin-releasing factor (CRF) family (143). The UCN II can be detected in various types of immune cells, including macrophages/monocytes, T cells, and mast cells, and plays a remarkable anti-inflammatory role in immune responses (144).
UCN II exhibits a broad spectrum of antimicrobial properties (145). Its bactericidal activity is primarily exerted through membrane damage (88, 145). It has good bactericidal effects against gram-negative E. coli (median effective concentration, EC50: 2.81 µM) and gram-positive bacteria such as S. mutans (EC50 > 20 µM) and Micrococcus luteus (EC50: 4.92 µM) (145). In parasites, UCN II destroys promastigotes by forming pores in their membranes, similar to a membrane damage mechanism. The application of UCN II in L. major-infected BALB/c mice significantly controls infection (145).
3.10 Neuropeptide Y (NPY)
In 1982, NPY was initially isolated from pig brain tissue by Tatemoto et al. (146). It is one of the most abundant neuropeptides in the brain, even more so than VIP (147). NPY belongs to the NPY family and consists of 36 amino acids (148, 149). Its secondary structure is the PP-fold, a generalized α-helix, which comprises a long N-terminal polyproline helix, a type II β-helix, and a long amphiphilic α-helix (150). NPY is ubiquitously present in both the central and peripheral nervous systems, as well as in immune cells such as macrophages, lymphocytes, and neutrophils (151). It acts as an important immunomodulator and plays a significant anti-inflammatory role (92).
NPY also exhibits broad-spectrum antimicrobial effects (152). Its antimicrobial mechanism involves disintegrating the bacterial membrane, leading to lysis and death of pathogenic microorganisms (151, 153). Notably, NPY shows particularly strong antibacterial activity against gram-negative E. coli (MIC: 4.2-11 µM), Aeromonas caviae (MIC: 14 µM), gram-positive Nocarida brasiliensis (MIC: 7 µM), and the fungus C. albicans (MIC: 1-2 µM) (115, 152). Furthermore, NPY at a concentration of 10-9 M has been shown to exhibit a significant chemoavoidance effect on L. brasiliensis parasites (74). Although the protective effects of NPY during retroviral pathogenesis in the central nervous system (CNS), such as in HIV and Ebola, are evident, the complete underlying mechanisms remain unclear (91, 115).
3.11 Hydra NDA-1
NDA-1, a neuropeptide specific to Hydra, was discovered by Augustin et al. (95) in 2017. It consists of 38 amino acids and has a β-sheet secondary structure. NDA-1 is secreted in sensory and ganglion neurons in the ectodermal epithelium, with high expression in the head (hypostome) and foot of Hydra (95).
NDA-1 exhibits a broad spectrum of antibacterial activity in vitro and can influence the Hydra microbiome, resulting in a lower abundance of Curvibacter sp. microbiota in the body column and foot tissue than in the tentacles. The antimicrobial mechanism of NDA-1 may involve interactions between its hydrophobic bag and the bacterial membrane, similar to the membrane damage mechanism (95). It demonstrates high toxicity against the gram-negative bacteria Curvibacter sp. (MIC: 0.4 µM), Acinetobacter sp. (MIC: 7 µM), and E. coli (MIC > 14 µM), as well as the gram-positive bacteria Bacillus megaterium (MIC: 0.4 µM), Trichococcus pasteurii (MIC: 0.9 µM), and Trichococcus collinsii (MIC: 0.4 µM) (95).
3.12 Catestatin (CST)
In 1997, Mahata et al. (96) first discovered and identified CST as a catecholamine release inhibitory peptide consisting of 21 amino acids. CST has a highly alkaline nature with an amphiphilic conformation (97) and adopts a β-sheet secondary structure (154). Furthermore, CST is primarily expressed in peripheral mononuclear cells, mast cells, and macrophages and exerts its anti-inflammatory effect on immune regulation (98, 155).
The antimicrobial activity of CST was initially demonstrated by Briolat et al. (156) in 2005. The antimicrobial mechanism of CST is similar to that of typical AMPs, as CST has been shown to cause membrane damage (99). Notably, CST exhibits significant antimicrobial efficacy against various pathogens, including the gram-negative bacteria E. coli (MIC: 15 µM) and P. aeruginosa (MIC: 50 µM), the gram-positive bacterium M. luteus (MIC: 5 µM) and Group A Streptococcus (MIC: 75 µM), and the filamentous fungus Aspergillus fumigatus (MIC: 80 µM) (156, 157).
4 Therapeutic potential in brain infectious disease
4.1 Macrophages act as bacterial carriers to break through the BBB
The BBB serves as a highly regulated interface between the bloodstream and the brain, playing a crucial role in the CNS by facilitating infection signaling to the brain (158). During infections and autoimmune diseases, macrophages can infiltrate the brain to eliminate pathogens, such as Group B Streptococcus (GBS) infection of the CNS, which triggers the recruitment of macrophages through immune deficiency (Imd) (159). In recent years, it has been discovered that macrophages serve as replicative niches for various bacteria, such as P. aeruginosa, E. coli, Yersinia pestis, Group A Streptococcus, and GBS (160). These macrophages can act as splenic reservoirs of sepsis and facilitate the survival and replication of Streptococcus pneumoniae within the intracellular environment. In other words, macrophages function as bacterial carriers, akin to “Trojan horses,” enabling successful traversal of the BBB and subsequent brain infection (160).
Bacteria must overcome diverse antimicrobial stimuli to survive within macrophages (160). Metal toxicity represents a prominent mechanism employed by macrophages for bacterial eradication (161). Korir et al. (160) demonstrated that GBS strains possess virulence mechanisms that enable prolonged survival within macrophages. GBS cells express cadD, which encodes a crucial metal efflux transporter that helps remove excess metal ions from the cell, thereby conferring resistance to metal toxicity (160). Apart from the cadD locus, sczA and czcD have also been identified as being involved in metal efflux (162). Moreover, cadD orthologues have been detected in other pathogens, such as S. aureus (160). In general, when pathogens enter macrophages, they evade intracellular metal toxicity through metal efflux, thereby enabling prolonged survival (160). Additionally, studies have also revealed that alterations in macrophage polarization are partially attributed to variations in macrophage stimulation during different S. aureus infection scenarios (163). M1 (pro-inflammatory) or M2 (anti-inflammatory) polarization leads to different responses of macrophages to S. aureus. M1 polarization resulted in bacterial death through reactive oxygen species (ROS), acidic pH, enzyme nutrient restriction and AMPs (164, 165). However, under certain circumstances, S. aureus can manipulate macrophage autophagy to induce M2 polarized, and effectively evade and manipulate macrophages, ultimately hindering macrophage recruitment, phagocytosis and degrative abilities (163).
4.2 Antimicrobial neuropeptides are upregulated during pathogen infection
Numerous neuropeptides share similarities with AMPs in terms of their size, hydrophobicity, charge, and amino acid composition (55). These neuropeptides, which are involved in neurological or neuroendocrine signaling processes, have shown a wide range of antimicrobial effects against various microorganisms (55). Generally, the upregulation of neuropeptide expression is often observed in response to bacterial-induced inflammatory conditions, such as pulpitis, periodontal disease, and in vivo bacteremia (55, 166). Lundy’s research team (166–168) discovered that in the odontoblastic and subodontoplastic layers of the dental pulp close to caries lesions, the sprouting of peptidergic nerves leads to increased levels of neuropeptides (such as VIP, NPY, and SP) at the site of local inflammation, thereby facilitating their direct antimicrobial actions. Furthermore, Lee et al. (1) reported a significant upregulation of PACAP expression—up to a 50-fold increase—in response to infection with S. aureus or C. albicans, suggesting that PACAP is involved in the antimicrobial defense of the CNS by preventing the infiltration of inflammatory cells.
4.3 Anti-inflammatory activity of AMPs
For a substantial period, the neuroendocrine and immune systems are considered two separate networks that regulate the balance between the host and its surroundings (169). The neuroendocrine system responds when stimulated by external environmental factors, whereas the immune system assumes its role in fighting invading bacteria, viruses, and other pathogens (169). However, in the past three decades, significant progress in research on the immune system and neuroendocrine system has established a complex and profound interplay between these two systems (170). On the one hand, the neuroendocrine system modulates immune responses through the release of hypothalamic and pituitary hormones, as well as the activation of the autonomic nervous system (171). On the other hand, the immune system detects stimuli that go unnoticed by the neuroendocrine system, such as bacteria, viruses, and tumors, and converts them into signals that prompt a response from the neuroendocrine system, helping to regulate fever and sleep problems (169). This bidirectional communication mechanism between these two entities plays a critical role in perceiving external stimuli, maintaining homeostasis, orchestrating immune responses, and governing growth and development (172). The underlying mechanism can be attributed to a shared biochemical language among them, including common neurotransmitters (such as neuropeptides and hormones), immune cytokines, and other ligands, along with their respective receptors (173). The complex interplay between the neuroendocrine system and the immune system plays a pivotal role in eradicating pathogens and restoring immune homeostasis (13). However, when this delicate equilibrium is disrupted, it can trigger a cascade of detrimental effects on infectious and autoimmune diseases, exerting a profound impact on pathological processes (174).
The induction of immune tolerance is crucial for maintaining immune homeostasis, regulating autologous reactive T cells, preventing the development of autoimmune diseases, and achieving transplantation tolerance (175). Inflammation is an essential process for pathogen eradication; however, uncontrolled inflammation, especially in the brain, can lead to severe adverse effects on the host. Therefore, the investigation of endogenous factors that regulate immune tolerance and inflammation represents a crucial research topic within the field of immunology.
Between 2000 and 2008, Delgado’s team made the groundbreaking discovery that neuropeptides secreted by immune cells exert inhibitory effects on inflammation while maintaining immune homeostasis. These neuropeptides mainly include VIP, α-MSH, UCN I, AM, and cortistatin (11, 13, 169). Among the antibacterial neuropeptides mentioned in this paper, the main ones with anti-inflammatory activity include PACAP (176), VIP (11), α-MSH (11), Ghrelin (177), AM (11), NPY (178), UCN II (179), CGRP (180), and CST (98). The mechanism of action can be summarized as follows: antimicrobial neuropeptides exert their effects on macrophages, monocytes, and microglia through regulatory T cells, leading to the inhibition of the production and release of inflammatory factors (TNF-α, IL-6, and IL-1β), chemokines (CCL5, IL-8, and IP-10), and NO. Additionally, they promote the production of anti-inflammatory cytokines such as TGFβ, which exerts their anti-inflammatory effects (181). Moreover, antibacterial neuropeptides play a pivotal role in maintaining the equilibrium between TH2 and regulatory T cells as well as between TH1 cells within the body, thereby ensuring a state of homeostasis between anti-inflammatory and proinflammatory factors to prevent the onset of autoimmune diseases (11).
4.4 Is the antimicrobial and anti-inflammatory activity of antimicrobial neuropeptides a defense mechanism of the brain?
The mechanism by which the vertebrate brain defends against pathogen infection is currently a key research area. Inflammatory attacks, facilitated by immune cells, can cause damage, and whereas the brain has limited ability to repair itself, so pathogenic microorganisms must be eliminated with minimal collateral damage to the organ itself (1, 2). Thus, it is likely that the brain possesses an immune defense mechanism that has yet to be fully understood. Through an extensive review of the literature, it has been observed that certain neuropeptides synthesized by nerve cells or immune cells share significant similarities with AMPs in terms of their physicochemical properties. These neuropeptides have demonstrated a wide range of antimicrobial activity in vitro, effectively guarding nerve tissue against microbial invasion (1). Furthermore, these antimicrobial neuropeptides have also been demonstrated potent anti-inflammatory properties and can contribute to the regulation of immune tolerance in various immune disorders (11). Notably, the connection between this anti-inflammatory activity and the brain’s defense against pathogen infection remains poorly explored. Therefore, we proposed a hypothesis that the antimicrobial activity of neuropeptides can efficiently eliminate pathogenic microorganisms in the brain, whereas their anti-inflammatory activity can suppress the occurrence of off-target inflammation. Further investigations of this topic will be a primary focus of future research. Additionally, neuropeptides possessing both antimicrobial and anti-inflammatory properties may hold great potential as a novel class of antimicrobial drugs. Extensive pharmaceutical research and clinical testing may prove valuable in the treatment of bacterial meningoencephalitis. If successfully developed into a pharmaceutical agent, this approach could offer a significant breakthrough in the treatment of brain infectious diseases, particularly in light of concerns regarding the misuse of antibiotics.
5 Conclusion
Antimicrobial neuropeptides protecting vertebrate brains against infection is a newly discovered brain defense mechanism in 2021 (1). However, given the limited regenerative capacity of the brain in response to immune cell-mediated inflammatory attacks, the presence of an unexplored layer of immune defense mechanisms has become increasingly significant.
In this review, we have provided a concise summary of the physicochemical characteristics and potential antimicrobial mechanisms of AMPs. Several neuropeptides, which are produced by nerve cells or immune cells, are remarkably similar to AMPs and exhibit a broad spectrum of antimicrobial activities. Subsequently, we have undertaken an extensive review of 12 previously documented neuropeptides that possess antimicrobial properties. Our comprehensive analysis included an exploration of their origin, structural attributes, possible antimicrobial mechanisms, and observed efficacy against microbial agents. Furthermore, it has been noted that a majority of these antimicrobial neuropeptides (9 out of 12) also exhibit potent anti-inflammatory activity, indicating their potential involvement in regulating immune disorders. Consequently, the combined antimicrobial and anti-inflammatory activities of neuropeptides could play a pivotal role in fortifying the defense mechanisms of the brain against pathogenic invaders. Moving forward, we anticipate the validation of this hypothesis in future research endeavors.
Author contributions
XL: Writing – original draft. KC: Writing – original draft. RL: Writing – original draft. ZZ: Funding acquisition, Writing – review & editing. XH: Conceptualization, Funding acquisition, Investigation, Supervision, Writing – original draft, Writing – review & editing.
Funding
The author(s) declare that financial support was received for the research, authorship, and/or publication of this article. This research was funded by National Natural Science Foundation of China (grant no. 32303065); Shandong Provincial Natural Science Foundation (grant no. ZR2022QC194); Medical and Health Science and Technology Development Project of Shandong Province (grant no. 202202050630); Research Fund for Lin He’s Academician Workstation of New Medicine and Clinical Translation in Jining Medical University (grant no. XJ2022006401).
Acknowledgments
We would like to thank the reviewers for their kind and helpful comments on the original manuscript.
Conflict of interest
The authors declare that the research was conducted in the absence of any commercial or financial relationships that could be construed as a potential conflict of interest.
Publisher’s note
All claims expressed in this article are solely those of the authors and do not necessarily represent those of their affiliated organizations, or those of the publisher, the editors and the reviewers. Any product that may be evaluated in this article, or claim that may be made by its manufacturer, is not guaranteed or endorsed by the publisher.
Abbreviations
α-MSH, α-Melanocyte stimulating hormone; Aβ, β-Amyloid; AG, Acylated ghrelin; AM, Adrenomedullin; AMP, Antimicrobial peptide; BBB, Blood-brain barrier; CGRP, Calcitonin gene-related peptide; CNS, Central nervous system; CRF, Corticotropin-releasing factor; CST, Catestatin; DAG, Deacylated ghrelin; EC50, Median effective concentration; EPS, Extracellular polymeric substances; eDNA, Extracellular DNA; GBS, Group B Streptococcus; HSV-1, Herpes simplex virus 1; IC50, Half maximal inhibitory concentration; MIC, Minimum inhibitory concentration; MV, Measles virus; NPY, Neuropeptide Y; ORXA, Orexin-A; ORXB, Orexin-B; ROS, Reactive oxygen species; PACAP, Pituitary adenylate cyclase-activating peptide; PIA, Polysaccharide intercellular adhesin; POMC, Proopiomelanocortin; SP, Substance P; UCN II, Urocortin-II; VIP, Vasoactive intestinal peptide.
References
1. Lee EY, Chan LC, Wang H, Lieng J, Hung M, Srinivasan Y, et al. PACAP is a pathogen-inducible resident antimicrobial neuropeptide affording rapid and contextual molecular host defense of the brain. P Natl Acad Sci USA. (2021) 118:e1917623117. doi: 10.1073/pnas.1917623117
2. Zasloff M. An ancient neuropeptide defends the brain against infection. P Natl Acad Sci USA. (2021) 118:e2023990118. doi: 10.1073/pnas.2023990118
3. Govic YL, Demey B, Cassereau J, Bahn YS, Papon N. Pathogens infecting the central nervous system. PloS Pathog. (2022) 18:e1010234. doi: 10.1371/journal.ppat.1010234
4. Klose CS, Mahlakõiv T, Moeller JB, Rankin LC, Flamar AL, Kabata H, et al. The neuropeptide neuromedin U stimulates innate lymphoid cells and type 2 inflammation. Nature. (2017) 549:282–6. doi: 10.1038/nature23676
5. Wallrapp A, Riesenfeld SJ, Burkett PR, Abdulnour REE, Nyman J, Dionne D, et al. The neuropeptide NMU amplifies ILC2-driven allergic lung inflammation. Nature. (2017) 549:351–6. doi: 10.1038/nature24029
6. Augustyniak D, Kramarska E, Mackiewicz P, Orczyk-Pawiłowicz M, Lundy FT. Mammalian Neuropeptides as Modulators of Microbial Infections: Their dual role in defense versus virulence and pathogenesis. Int J Mol Sci. (2021) 22:3658. doi: 10.3390/ijms22073658
7. Mazurkiewicz-Pisarek A, Baran J, Ciach T. Antimicrobial peptides: challenging journey to the pharmaceutical, biomedical, and cosmeceutical use. Int J Mol Sci. (2023) 24:9031. doi: 10.3390/ijms24109031
8. Seyfi R, Kahaki FA, Ebrahimi T, Montazersaheb S, Eyvazi S, Babaeipour V, et al. Antimicrobial peptides (AMPs): roles, functions and mechanism of action. Int J Pept Res Ther. (2020) 26:1451–63. doi: 10.1007/s10989-019-09946-9
9. Zaccone G, Capillo G, Fernandes JMO, Kiron V, Lauriano ER, Alesci A, et al. Expression of the antimicrobial peptide piscidin 1 and neuropeptides in fish gill and skin: A potential participation in neuro-immune interaction. Mar Drugs. (2022) 20:145. doi: 10.3390/md20020145
10. Lauritano D, Mastrangelo F, D’Ovidio C, Ronconi G, Caraffa A, Gallenga CE, et al. Activation of mast cells by neuropeptides: the role of pro-inflammatory and anti-inflammatory cytokines. Int J Mol Sci. (2023) 24:4811. doi: 10.3390/ijms24054811
11. Gonzalez-Rey E, Chorny A, Delgado M. Regulation of immune tolerance by anti-inflammatory neuropeptides. Nat Rev Immunol. (2007) 7:52–63. doi: 10.1038/nri1984
12. Puri S, Kenyon BM, Hamrah P. Immunomodulatory role of neuropeptides in the cornea. Biomedicines. (2022) 10:1985. doi: 10.3390/biomedicines10081985
13. Delgado M, Ganea D. Anti-inflammatory neuropeptides: A new class of endogenous immunoregulatory agents. Brain Behav Immun. (2008) 22:1146–51. doi: 10.1016/j.bbi.2008.06.001
14. Weaver DF. Endogenous antimicrobial-immunomodulatory molecules: networking biomolecules of innate immunity. Chembiochem. (2024) 25:e202400089. doi: 10.1002/cbic.202400089
15. Browne K, Chakraborty S, Chen R, Willcox MD, Black DS, Walsh WR, et al. A new era of antibiotics: the clinical potential of antimicrobial peptides. Int J Mol Sci. (2020) 21:7047. doi: 10.3390/ijms21197047
16. Gottler LM, Ramamoorthy A. Structure, membrane orientation, mechanism, and function of pexiganan—a highly potent antimicrobial peptide designed from magainin. BBA-Biomembranes. (2009) 1788:1680–6. doi: 10.1016/j.bbamem.2008.10.009
17. Chen N, Jiang C. Antimicrobial peptides: Structure, mechanism, and modification. Eur J Med Chem. (2023) 255:115377. doi: 10.1016/j.ejmech.2023.115377
18. Min KH, Kim KH, Ki MR, Pack SP. Antimicrobial peptides and their biomedical applications: A review. Antibiotics. (2024) 13:794. doi: 10.3390/antibiotics13090794
19. Chettri D, Rani A, Sharma B, Selvaraj M, Assiri MA, Verma AK. Antimicrobial Peptides: Source, application and recent developments. Process Biochem. (2024) 145:288–301. doi: 10.1016/j.procbio.2024.07.002
20. Bin Hafeez A, Jiang X, Bergen PJ, Zhu Y. Antimicrobial peptides: an update on classifications and databases. Int J Mol Sci. (2021) 22:11691. doi: 10.3390/ijms222111691
21. Wang G. Unifying the classification of antimicrobial peptides in the antimicrobial peptide database. Methods Enzymol. Acad Press. (2022) 663:1–18. doi: 10.1016/bs.mie.2021.09.006
22. Kordi M, Borzouyi Z, Chitsaz S, hadi Asmaei M, Salami R, Tabarzad M. Antimicrobial peptides with anticancer activity: Today status, trends and their computational design. Arch Biochem Biophys. (2023) 733:109484. doi: 10.1016/j.abb.2022.109484
23. Ghanbarzadeh Z, Hemmati S, Mohagheghzadeh A. Humanizing plant-derived snakins and their encrypted antimicrobial peptides. Biochimie. (2022) 199:92–111. doi: 10.1016/j.biochi.2022.04.011
24. Zhang M, Li S, Zhao J, Shuang Q, Xia Y, Zhang F. A novel endogenous antimicrobial peptide MP-4 derived from koumiss of Inner Mongolia by peptidomics, and effects on Staphylococcus aureus. LWT. (2024) 191:115595. doi: 10.1016/j.lwt.2023.115595
25. Bhattacharjya S, Zhang Z, Ramamoorthy A. LL-37: structures, antimicrobial activity, and influence on amyloid-related diseases. Biomolecules. (2024) 14:320. doi: 10.3390/biom14030320
26. Montis C, Marelli E, Valle F, Bombelli FB, Pigliacelli C. Engineering the interaction of short antimicrobial peptides with bacterial barriers. Mol Syst Des Eng. (2024) 9:541–60. doi: 10.1039/D4ME00021H
27. Bertrand B, Garduño-Juárez R, Munoz-Garay C. Estimation of pore dimensions in lipid membranes induced by peptides and other biomolecules: A review. BBA-Biomembranes. (2021) 1863:183551. doi: 10.1016/j.bbamem.2021.183551
28. Zhang QY, Yan ZB, Meng YM, Hong XY, Shao G, Ma JJ, et al. Antimicrobial peptides: mechanism of action, activity and clinical potential. Military Med Res. (2021) 8:1–25. doi: 10.1186/s40779-021-00343-2
29. Yeung AT, Gellatly SL, Hancock RE. Multifunctional cationic host defence peptides and their clinical applications. Cell Mol Life Sci. (2011) 68:2161–76. doi: 10.1007/s00018-011-0710-x
30. Taheri-Araghi S. Synergistic action of antimicrobial peptides and antibiotics: current understanding and future directions. Front Microbiol. (2024) 15:1390765. doi: 10.3389/fmicb.2024.1390765
31. Nguyen LT, Haney EF, Vogel HJ. The expanding scope of antimicrobial peptide structures and their modes of action. Trends Biotechnol. (2011) 29:464–72. doi: 10.1016/j.tibtech.2011.05.001
32. Costerton JW, Stewart PS, Greenberg EP. Bacterial biofilms: a common cause of persistent infections. Science. (1999) 284:1318–22. doi: 10.1126/science.284.5418.1318
33. Yang L, Harroun TA, Weiss TM, Ding L, Huang HW. Barrel-stave model or toroidal model? A case study on melittin pores. Biophys J. (2001) 81:1475–85. doi: 10.1016/S0006-3495(01)75802-X
34. Yasir M, Willcox MDP, Dutta D. Action of antimicrobial peptides against bacterial biofilms. Materials. (2018) 11:2468. doi: 10.3390/ma11122468
35. Di Somma A, Moretta A, Canè C, Cirillo A, Duilio A. Antimicrobial and antibiofilm peptides. Biomolecules. (2020) 10:652. doi: 10.3390/biom10040652
36. Donlan RM. Biofilm formation: a clinically relevant microbiological process. Clin Infect Dis. (2001) 33:1387–92. doi: 10.1086/322972
37. Jorge P, Lourenco A, Pereira MO. New trends in peptide-based anti-biofilm strategies: a review of recent achievements and bioinformatic approaches. Biofouling. (2012) 28:1033–61. doi: 10.1080/08927014.2012.728210
38. Wang H, Lin L, Tan L, Yu HY, Cheng JW, Pan Y. Molecular pathways underlying inhibitory effect of antimicrobial peptide Nal-P-113 on bacteria biofilms formation of Porphyromonas gingivalis W83 by DNA microarray. BMC Microbiol. (2017) 17:1–7. doi: 10.1186/s12866-017-0948-z
39. Pontes JTC, Toledo Borges AB, Roque-Borda CA, Pavan FR. Antimicrobial peptides as an alternative for the eradication of bacterial biofilms of multi-drug resistant bacteria. Pharmaceutics. (2022) 14:642. doi: 10.3390/pharmaceutics14030642
40. Nagant C, Pitts B, Stewart PS, Feng Y, Savage PB, Dehaye JP. Study of the effect of antimicrobial peptide mimic, CSA-13, on an established biofilm formed by Pseudomonas aeruginosa. Microbiologyopen. (2013) 2:318–25. doi: 10.1002/mbo3.77
41. Ma H, Zhao X, Yang L, Su P, Fu P, Peng J, et al. Antimicrobial peptide AMP-17 affects Candida albicans by disrupting its cell wall and cell membrane integrity. Infect Drug Resist. (2020) 13:2509–20. doi: 10.2147/IDR.S250278
42. Allaker RP, Grosvenor PW, McAnerney DC, Sheehan BE, Srikanta BH, Pell K, et al. Mechanisms of adrenomedullin antimicrobial action. Peptides. (2006) 27:661–6. doi: 10.1016/j.peptides.2005.09.003
43. Bommineni YR, Achanta M, Alexander J, Sunkara LT, Ritchey JW, Zhang G. A fowlicidin-1 analog protects mice from lethal infections induced by methicillin-resistant Staphylococcus aureus. Peptides. (2010) 31:1225–30. doi: 10.1016/j.peptides.2010.03.037
44. Florin T, Maracci C, Graf M, Karki P, Klepacki D, Berninghausen O, et al. An antimicrobial peptide that inhibits translation by trapping release factors on the ribosome. Nat Struct Mol Biol. (2017) 24:752–7. doi: 10.1038/nsmb.3439
45. Boparai JK, Sharma PK. Mini review on antimicrobial peptides, sources, mechanism and recent applications. Protein Pept Lett. (2020) 27:4–16. doi: 10.2174/0929866526666190822165812
46. Sneideris T, Erkamp NA, Ausserwöger H, Saar KL, Welsh TJ, Qian D, et al. Targeting nucleic acid phase transitions as a mechanism of action for antimicrobial peptides. Nat Commun. (2023) 14:7170. doi: 10.1038/s41467-023-42374-4
47. Xuan J, Feng W, Wang J, Wang R, Zhang B, Bo L, et al. Antimicrobial peptides for combating drug-resistant bacterial infections. Drug Resist Update. (2023) 68:100954. doi: 10.1016/j.drup.2023.100954
48. Niccolai LM, Ruddle NH, Krause PJ. Immunoepidemiology. Cham: Springer International Publishing, Springer Nature (2019). doi: 10.1007/978-3-030-25553-4
49. Delgado M, Reduta A, Sharma V, Ganea D. VIP/PACAP oppositely affects immature and mature dendritic cell expression of CD80/CD86 and the stimulatory activity for CD4+ T cells. J Leukoc Biol. (2004) 75:1122–30. doi: 10.1189/jlb.1203626
50. Souza TML, Temerozo JR, Giestal-de-Araujo E, Bou-Habib DC. The effects of neurotrophins and the neuropeptides VIP and PACAP on HIV-1 infection: histories with opposite ends. Neuroimmunomodulat. (2014) 21:268–82. doi: 10.1159/000357434
51. Hirabayashi T, Nakamachi T, Shioda S. Discovery of PACAP and its receptors in the brain. J Headache Pain. (2018) 19:1–8. doi: 10.1186/s10194-018-0855-1
52. Lugo JM, Tafalla C, Oliva A, Pons T, Oliva B, Aquilino C, et al. Evidence for antimicrobial and anticancer activity of pituitary adenylate cyclase-activating polypeptide (PACAP) from North African catfish (Clarias gariepinus): Its potential use as novel therapeutic agent in fish and humans. Fish Shellfish Immun. (2019) 86:559–70. doi: 10.1016/j.fsi.2018.11.056
53. Martinez C, Delgado M, Abad C, Gomariz RP, Ganea D, Leceta J. Regulation of VIP production and secretion by murine lymphocytes. J Neuroimmunol. (1999) 93:126–38. doi: 10.1016/s0165-5728(98)00216-1
54. GONZALEZ-REY E, Chorny A, Delgado M. VIP: an agent with license to kill infective parasites. Ann NY Acad Sci. (2006) 1070:303–8. doi: 10.1196/annals.1317.032
55. Karim I, Linden GJ, Orr DF, Lundy FT. Antimicrobial activity of neuropeptides against a range of micro-organisms from skin, oral, respiratory and gastrointestinal tract sites. J Neuroimmunol. (2008) 200:11–6. doi: 10.1016/j.jneuroim.2008.05.014
56. Zeng L, Zhang X, Xia M, Ye H, Li H, Gao Z. Heme and Cu2+-induced vasoactive intestinal peptide (VIP) tyrosine nitration: A possible molecular mechanism for the attenuated anti-inflammatory effect of VIP in inflammatory diseases. Biochimie. (2023) 214:176–87. doi: 10.1016/j.biochi.2023.07.011
57. Catania A, Cutuli M, Garofalo L, Carlin A, Airaghi L, Barcellini W, et al. The neuropeptide α-MSH in host defense. Ann NY Acad Sci. (2000) 917:227–31. doi: 10.1111/j.1749-6632.2000
58. Luger T, Brzoska T, Scholzen T, Kalden DH, Sunderkötter C, Armstrong C, et al. The role of α-MSH as a modulator of cutaneous inflammation. Ann NY Acad Sci. (2000) 917:232–8. doi: 10.1111/j.1749-6632.2000.tb05388.x
59. Donnarumma G, Paoletti I, Buommino E, Antonietta, Tufano M, Baroni A. [amp]]alpha;-MSH reduces the internalization of Staphylococcus aureus and down-regulates HSP 70, integrins and cytokine expression in human keratinocyte cell lines. Exp Dermatol. (2004) 13:748–54. doi: 10.1111/j.0906-6705.2004.00218.x
60. Fjell CD, Hiss JA, Hancock RE, Schneider G. Designing antimicrobial peptides: form follows function. Nat Rev Drug Discovery. (2012) 11:37–51. doi: 10.1038/nrd3591
61. Herraiz C, Martínez-Vicente I, Maresca V. The α-melanocyte-stimulating hormone/melanocortin-1 receptor interaction: A driver of pleiotropic effects beyond pigmentation. Pigm Cell Melanoma R. (2021) 34:748–61. doi: 10.1111/pcmr.12980
62. Ichinose M, Asai M, Sawada M, Sasaki K, Oomura Y. Induction of outward current by orexin-B in mouse peritoneal macrophages. FEBS Lett. (1998) 440:51–4. doi: 10.1016/s0014-5793(98)01432-x
63. Sakurai T, Amemiya A, Ishii M, Matsuzaki I, Chemelli RM, Tanaka H, et al. Orexins and orexin receptors: a family of hypothalamic neuropeptides and G protein-coupled receptors that regulate feeding behavior. Cell. (1998) 92:573–85. doi: 10.1016/s0092-8674(00)80949-6
64. Sakurai T. Orexins and orexin receptors: implication in feeding behavior. Regul Peptides. (1999) 85:25–30. doi: 10.1016/s0167-0115(99)00076-2
65. Takai T, Takaya T, Nakano M, Akutsu H, Nakagawa A, Aimoto S, et al. Orexin-A is composed of a highly conserved C-terminal and a specific, hydrophilic N-terminal region, revealing the structural basis of specific recognition by the orexin-1 receptor. J Pept Sci. (2006) 12:443–54. doi: 10.1002/psc.747
66. Zannella C, Stelitano D, Folliero V, Palomba L, Bovier TF, Astorri R, et al. Antibacterial and antiviral potential of neuropeptides. Trans Med Rep. (2019) 3:8142. doi: 10.4081/tmr.8142
67. Waseem T, Duxbury M, Ito H, Ashley SW, Robinson MK. Exogenous ghrelin modulates release of pro-inflammatory and anti-inflammatory cytokines in LPS-stimulated macrophages through distinct signaling pathways. Surgery. (2008) 143:334–42. doi: 10.1016/j.surg.2007.09.039
68. Delgado M, Anderson P, Garcia-Salcedo J, Caro M, Gonzalez-Rey E. Neuropeptides kill African trypanosomes by targeting intracellular compartments and inducing autophagic-like cell death. Cell Death Differ. (2009) 16:406–16. doi: 10.1038/cdd.2008.161
69. Staes E, Absil P-A, Lins L, Brasseur R, Deleu M, Lecouturier N, et al. Acylated and unacylated ghrelin binding to membranes and to ghrelin receptor: towards a better understanding of the underlying mechanisms. BBA-Biomembranes. (2010) 1798:2102–13. doi: 10.1016/j.bbamem.2010.07.002
70. Min C, Ohta K, Kajiya M, Zhu T, Sharma K, Shin J, et al. The antimicrobial activity of the appetite peptide hormone ghrelin. Peptides. (2012) 36:151–6. doi: 10.1016/j.peptides.2012.05.006
72. Datar P, Srivastava S, Coutinho E, Govil G. Substance P: structure, function, and therapeutics. Curr Top Med Chem. (2004) 4:75–103. doi: 10.2174/1568026043451636
73. O'Connor TM, O'Connell J, O'Brien DI, Goode T, Bredin CP, Shanahan F. The role of substance P in inflammatory disease. J Cell Physiol. (2004) 201:167–80. doi: 10.1002/jcp.20061
74. Giammarressi M, Vanegas O, Febres A, Silva-López A, López ED, Ponte-Sucre A. Chemotactic activities of vasoactive intestinal peptide, neuropeptide Y and substance P in Leishmania Braziliensis. Exp Parasitol. (2020) 219:108009. doi: 10.1016/j.exppara.2020.108009
75. Zhu Z, Yao Y, Huang S, Ma L, Song J, Zhang W. Antimicrobial and anti-inflammatory activities of the neuropeptide antagonist SPA. J Pept Sci. (2022) 28:e3402. doi: 10.1002/psc.3402
76. Kitamura K, Kangawa K, Kawamoto M, Ichiki Y, Nakamura S, Matsuo H, et al. A novel hypotensive peptide isolated from human pheochromocytoma. Biochem Bioph Res Co. (1993) 192:553. doi: 10.1006/bbrc.1993.1451
77. Hinson JP, Kapas S, Smith DM. Adrenomedullin, a multifunctional regulatory peptide. Endocr Rev. (2000) 21:138–67. doi: 10.1210/edrv.21.2.0396
78. Allaker RP, Kapas S. Adrenomedullin and mucosal defence: interaction between host and microorganism. Regul Peptides. (2003) 112:147–52. doi: 10.1210/edrv.21.2.0396
79. Rullé S, Kioon MDA, Asensio C, Mussard J, Ea HK, Boissier MC, et al. Adrenomedullin, a neuropeptide with immunoregulatory properties induces semi-mature tolerogenic dendritic cells. Immunology. (2012) 136:252–64. doi: 10.1111/j.1365-2567.2012.03577.x
80. Goodman E, Iversen L. Calcitonin gene-related peptide: novel neuropeptide. Life Sci. (1986) 38:2169–78. doi: 10.1016/0024-3205(86)90568-0
81. O'Halloran DJ, Bloom S. Calcitonin gene related peptide. BMJ. (1991) 302:739–40. doi: 10.1136/bmj.302.6779.739
82. Holzmann B. Antiinflammatory activities of CGRP modulating innate immune responses in health and disease. Curr Protein Pept Sc. (2013) 14:268–74. doi: 10.2174/13892037113149990046
83. Watkins HA, Rathbone DL, Barwell J, Hay DL, Poyner DR. Structure–activity relationships for α-calcitonin gene-related peptide. Brit J Pharmacol. (2013) 170:1308–22. doi: 10.1111/bph.12072
84. Russell FA, King R, Smillie S-J, Kodji X, Brain S. Calcitonin gene-related peptide: physiology and pathophysiology. Physiol Rev. (2014) 94:1099–142. doi: 10.1152/physrev.00034.2013
85. N’diaye A, Gannesen A, Borrel V, Maillot O, Enault J, Racine PJ, et al. Substance P and calcitonin gene-related peptide: key regulators of cutaneous microbiota homeostasis. Front Endocrinol. (2017) 8:15. doi: 10.3389/fendo.2017.00015
86. Bamberger CM, BAMBERGER AM. The peripheral CRH/urocortin system. Ann NY Acad Sci. (2000) 917:290–6. doi: 10.1111/j.1749-6632.2000.tb05395.x
87. Tillinger A, Nostramo R, Kvetnansky R, Serova L, Sabban EL. Stress-induced changes in gene expression of urocortin 2 and other CRH peptides in rat adrenal medulla: involvement of glucocorticoids. J Neurochem. (2013) 125:185–92. doi: 10.1111/jnc.12152
88. Zahedifard F, Rafati S. Prospects for antimicrobial peptide-based immunotherapy approaches in Leishmania control. Expert Rev Anti-Infe. (2018) 16:461–9. doi: 10.1080/14787210.2018.1483720
89. Monteiro-Pinto C, Adão R, Leite-Moreira AF, Brás-Silva C. Cardiovascular effects of urocortin-2: pathophysiological mechanisms and therapeutic potential. Cardiovasc Drug Ther. (2019) 33:599–613. doi: 10.1007/s10557-019-06895-9
90. Amano M. Urocortins. In: Handbook of Hormones. Cambridge: Academic Press (2021). p. 49–52. doi: 10.1016/B978-0-12-820649-2.00012-7
91. Du M, Butchi NB, Woods T, Morgan TW, Peterson KE. Neuropeptide Y has a protective role during murine retrovirus-induced neurological disease. J Virol. (2010) 84:11076–88. doi: 10.1128/JVI.01022-10
92. Dimitrijević M, Stanojević S. The intriguing mission of neuropeptide Y in the immune system. Amino Acids. (2013) 45:41–53. doi: 10.1007/s00726-011-1185-7
93. Reichmann F, Holzer P. Neuropeptide Y: A stressful review. Neuropeptides. (2016) 55:99–109. doi: 10.1016/j.npep.2015.09.008
94. Michel MC. Neuropeptide Y. In: Encyclopedia Mol Pharmacol. (2022), Cham: Springer Nature. 1104–7. doi: 10.1007/978-3-030-57401-7_99
95. Augustin R, Schröder K, Murillo Rincón AP, Fraune S, Anton-Erxleben F, Herbst E,M, et al. A secreted antibacterial neuropeptide shapes the microbiome of Hydra. Nat Commun. (2017) 8:698. doi: 10.1038/s41467-017-00625-1
96. Mahata SK, O'Connor DT, Mahata M, Yoo SH, Taupenot L, Wu H, et al. Novel autocrine feedback control of catecholamine release. A discrete chromogranin a fragment is a noncompetitive nicotinic cholinergic antagonist. J Clin Invest. (1997) 100:1623–33. doi: 10.1172/JCI119686
97. Sahu BS, Obbineni JM, Sahu G, Allu PK, Subramanian L, Sonawane PJ, et al. Functional genetic variants of the catecholamine-release-inhibitory peptide catestatin in an Indian population: allele-specific effects on metabolic traits. J Biol Chem. (2012) 287:43840–52. doi: 10.1074/jbc.M112.407916
98. Muntjewerff EM, Dunkel G, Nicolasen MJ, Mahata SK, van den Bogaart G. Catestatin as a target for treatment of inflammatory diseases. Front Immunol. (2018) 9:2199. doi: 10.3389/fimmu.2018.02199
99. Jati S, Mahata S, Das S, Chatterjee S, Mahata SK. Catestatin: antimicrobial functions and potential therapeutics. Pharmaceutics. (2023) 15:1550. doi: 10.3390/pharmaceutics15051550
100. Miyata A, Arimura A, Dahl RR, Minamino N, Uehara A, Jiang L, et al. Isolation of a novel 38 residue-hypothalamic polypeptide which stimulates adenylate cyclase in pituitary cells. Biochem Bioph Res Co. (1989) 164:567–74. doi: 10.1016/0006-291x(89)91757-9
101. Delgado M, Abad C, Martinez C, Juarranz MG, Leceta J, Ganea D, et al. PACAP in immunity and inflammation. Ann NY Acad Sci. (2003) 992:141–57. doi: 10.1111/j.1749-6632.2003.tb03145.x
102. Abad C, Martinez C, Leceta J, Juarranz MG, Delgado M, Gomariz RP. Pituitary adenylate-cyclase-activating polypeptide expression in the immune system. Neuroimmunomodulat. (2002) 10:177–86. doi: 10.1159/000067180
103. Said SI, Mutt V. Polypeptide with broad biological activity: isolation from small intestine. Science. (1970) 169:1217–8. doi: 10.1126/science.169.3951.1217
104. Englander EW, Greeley GH Jr. Postpyloric gastrointestinal peptides. In: Physiology of the gastrointestinal tract. (2006). New York: Academic Press. 121–59. doi: 10.1016/B978-012088394-3/50008-8
105. Iwasaki M, Akiba Y, Kaunitz JD. Recent advances in vasoactive intestinal peptide physiology and pathophysiology: focus on the gastrointestinal system. F1000Res. (2019) 8:1629. doi: 10.12688/f1000research.18039.1
106. Ohta K, Kajiya M, Zhu T, Nishi H, Mawardi H, Shin J, et al. Additive effects of orexin B and vasoactive intestinal polypeptide on LL-37-mediated antimicrobial activities. J Neuroimmunol. (2011) 233:37–45. doi: 10.1016/j.jneuroim.2010.11.009
107. Dürr UH, Sudheendra U, Ramamoorthy A. LL-37, the only human member of the cathelicidin family of antimicrobial peptides. BBA-Biomembranes. (2006) 1758:1408–25. doi: 10.1016/j.bbamem.2006.03.030
108. Lee TH, Lerner AB, Buettner-Janusch V. Adrenocorticotropic Hormone and Melanocyte-Stimulating Hormone from Human Pituitary Glands. Ciba Foundation Symposium-Human Pituitary Hormones (Colloquia on Endocrinology) Vol. 13. . Chichester, UK: John Wiley & Sons, Ltd (1960) p. 251–65. doi: 10.1002/9780470719183
109. Miró L, Amat C, Rosell-Cardona C, Campbell JM, Polo J, Pérez-Bosque A, et al. Dietary supplementation with spray-dried porcine plasma attenuates colon inflammation in a genetic mouse model of inflammatory bowel disease. Int J Mol Sci. (2020) 21:6760. doi: 10.3390/ijms21186760
110. Singh P. Fungicidal activity of alpha-melanocyte stimulating hor-mone in specific solvent augmented at explicit pH against Candida albicans. J Microbiol Antimicrob Agent. (2019) 5:21–9.
111. Price ML, Ley CD, Gorvin CM. The emerging role of heterodimerisation and interacting proteins in ghrelin receptor function. J Endocrinol. (2022) 252:R23–39. doi: 10.1530/JOE-21-0206
112. Soya S, Sakurai T. Evolution of orexin neuropeptide system: structure and function. Front Neurosci-Switz. (2020) 14:691. doi: 10.3389/fnins.2020.00691
113. Rotondo M, Honisch C, Tartaggia S, Ruzza P. Circular dichroism study of orexin B under oxidative stress conditions. Molecules. (2023) 28:484. doi: 10.3390/molecules28020484
114. Azhdari-Zarmehri H, Semnanian S, Fathollahi Y. Orexin-a modulates firing of rat rostral ventromedial medulla neurons: an in vitro study. Cell J. (2015) 17:163. doi: 10.22074/cellj.2015.524
115. Augustyniak D, Nowak J, Lundy FT. Direct and indirect antimicrobial activities of neuropeptides and their therapeutic potential. Curr Protein Pept Sc. (2012) 13:723–38. doi: 10.2174/138920312804871139
116. Eichenbaum H. To sleep, perchance to integrate. P Natl Acad Sci USA. (2007) 104:7317–8. doi: 10.1073/pnas.0702503104
117. Kojima M, Hosoda H, Date Y, Nakazato M, Matsuo H, Kangawa K. Ghrelin is a growth-hormone-releasing acylated peptide from stomach. Nature. (1999) 402:656–60. doi: 10.1038/45230
118. Kukol A. The structure of ghrelin. Vitam Horm. (2007) 77:1–12. doi: 10.1016/S0083-6729(06)77001-7
119. Kahlenberg JM, Kaplan MJ. Little peptide, big effects: the role of LL-37 in inflammation and autoimmune disease. J Immunol. (2013) 191:4895–901. doi: 10.4049/jimmunol.1302005
120. Perpétuo L, Voisin PM, Amado F, Hirtz C, Vitorino R. Ghrelin and adipokines: An overview of their physiological role, antimicrobial activity and impact on cardiovascular conditions. Vitam Horm. (2021) 115:477–509. doi: 10.1016/bs.vh.2020.12.019
121. Hattori N. Expression, regulation and biological actions of growth hormone (GH) and ghrelin in the immune system. Growth Horm IGF Res. (2009) 19:187–97. doi: 10.1016/j.ghir.2008.12.001
122. Chorny A, Anderson P, Gonzalez-Rey E, Delgado M. Ghrelin protects against experimental sepsis by inhibiting high-mobility group box 1 release and by killing bacteria. J Immunol. (2008) 180:8369–77. doi: 10.4049/jimmunol.180.12.8369
123. Euler U, Gaddum J. An unidentified depressor substance in certain tissue extracts. J Physiol. (1931) 72:74–87. doi: 10.1113/jphysiol.1931.sp002763
124. Leeman SE, Mroz EA. Substance p. Life Sci. (1974) 15:2033–44. doi: 10.1016/0024-3205(74)90020-4
125. Pickel VM, Reis DJ, Leeman SE. Ultrastructural localization of substance P in neurons of spinal cord. Brain Res. (1977) 122:534–40. doi: 10.1016/0006-8993(77)90463-2
126. Chassaing G, Convert O, Lavielle S. Preferential conformation of substance P in solution. Eur J Biochem. (1986) 154:77–85. doi: 10.1111/j.1432-1033.1986.tb09361.x
127. Jääskeläinen E, Teplova V, Andersson M, Andersson LC, Tammela P, Andersson M, et al. In vitro assay for human toxicity of cereulide, the emetic mitochondrial toxin produced by food poisoning Bacillus cereus. Toxicol In Vitro. (2003) 17:737–44. doi: 10.1016/s0887-2333(03)00096-1
128. Mijouin L, Hillion M, Ramdani Y, Jaouen T, Duclairoir-Poc C, Follet-Gueye M-L, et al. Effects of a skin neuropeptide (substance p) on cutaneous microflora. PloS One. (2013) 8:e78773. doi: 10.1371/journal.pone.0078773
129. de la Fuente-Núñez C, Mertens J, Smit J, Hancock RE. The bacterial surface layer provides protection against antimicrobial peptides. Appl Environ Microb. (2012) 78:5452–6. doi: 10.1128/AEM.01493-12
130. Makhortova NR, Askovich P, Patterson CE, Gechman LA, Gerard NP, Rall GF. Neurokinin-1 enables measles virus trans-synaptic spread in neurons. Virology. (2007) 362:235–44. doi: 10.1016/j.virol.2007.02.033
131. Fischer JP, Els-Heindl S, Beck-Sickinger AG. Adrenomedullin–current perspective on a peptide hormone with significant therapeutic potential. Peptides. (2020) 131:170347. doi: 10.1016/j.peptides.2020.170347
132. Adrenomedullin Ogoshi M. Handbook of hormones. Cambridge: Academic Press (2021) p. 417–9. doi: 10.1016/B978-0-12-820649-2.00108-X
133. Kitamura K, Kangawa K, Kawamoto M, Ichiki Y, Nakamura S, Matsuo H, et al. Adrenomedullin: a novel hypotensive peptide isolated from human pheochromocytoma. Biochem Bioph Res Co. (1993) 192:553–60. doi: 10.1006/bbrc.1993.1451
134. Giesbrecht P, Kersten T, Maidhof H, Wecke J. Staphylococcal cell wall: morphogenesis and fatal variations in the presence of penicillin. Microbiol Mol Biol R. (1998) 62:1371–414. doi: 10.1128/mmbr.62.4.1371-1414.1998
135. Allaker RP, Kapas S. Adrenomedullin expression by gastric epithelial cells in response to infection. Clin Vaccine Immunol. (2003) 10:546–51. doi: 10.1128/cdli.10.4.546-551.2003
136. Wimalawansa SJ. Calcitonin gene-related peptide and its receptors: molecular genetics, physiology, pathophysiology, and therapeutic potentials. Endocr Rev. (1996) 17:533–85. doi: 10.1210/edrv-17-5-533
137. Mikami N, Matsushita H, Kato T, Kawasaki R, Sawazaki T, Kishimoto T, et al. Calcitonin gene-related peptide is an important regulator of cutaneous immunity: effect on dendritic cell and T cell functions. J Immunol. (2011) 186:6886–93. doi: 10.4049/jimmunol.1100028
138. Benguettat O, Jneid R, Soltys J, Loudhaief R, Brun-Barale A, Osman D, et al. The DH31/CGRP enteroendocrine peptide triggers intestinal contractions favoring the elimination of opportunistic bacteria. PloS Pathog. (2018) 14:e1007279. doi: 10.1371/journal.ppat.1007279
139. Augustyniak D, Jankowski A, Mackiewicz P, Skowyra A, Gutowicz J, Drulis-Kawa Z. Innate immune properties of selected human neuropeptides against Moraxella catarrhalis and nontypeable Haemophilus influenzae. BMC Immunol. (2012) 13:1–17. doi: 10.1186/1471-2172-13-24
140. N’Diaye AR, Leclerc C, Kentache T, Hardouin J, Poc CD, Konto-Ghiorghi Y, et al. Skin-bacteria communication: Involvement of the neurohormone Calcitonin Gene Related Peptide (CGRP) in the regulation of Staphylococcus epidermidis virulence. Sci Rep-UK. (2016) 6:35379. doi: 10.1038/srep35379
141. Hauger RL, Grigoriadis DE, Dallman MF, Plotsky PM, Vale WW, Dautzenberg FM. International Union of Pharmacology. XXXVI. Current status of the nomenclature for receptors for corticotropin-releasing factor and their ligands. Pharmacol Rev. (2003) 55:21–6. doi: 10.1124/pr.55.1.3
142. Reyes T, Lewis K, Perrin M, Kunitake K, Vaughan J, Arias C, et al. Urocortin II: a member of the corticotropin-releasing factor (CRF) neuropeptide family that is selectively bound by type 2 CRF receptors. P Natl Acad Sci USA. (2001) 98:2843–8. doi: 10.1073/pnas.051626398
143. Calderón-de la Sancha FJ, Carrasco-Navarro U, Santander G, Barrios-González J, Mejía A. Novel antimicrobial activity of protein produced by Streptomyces lividans TK24 against the phytopathogen Clavibacter michiganensis. Arch Microbiol. (2022) 204:687. doi: 10.1007/s00203-022-03290-1
144. Gonzalez-Rey E, Chorny A, Varela N, O'Valle F, Delgado M. Therapeutic effect of urocortin on collagen-induced arthritis by down-regulation of inflammatory and Th1 responses and induction of regulatory T cells. Arthritis Rheumatol. (2007) 56:531–43. doi: 10.1002/art.22394
145. Campos-Salinas J, Caro M, Cavazzuti A, Forte-Lago I, Beverley SM, O’Valle F, et al. Protective role of the neuropeptide urocortin II against experimental sepsis and leishmaniasis by direct killing of pathogens. J Immunol. (2013) 191:6040–51. doi: 10.4049/jimmunol.1301921
146. Tatemoto K, Carlquist M, Mutt V. Neuropeptide Y—a novel brain peptide with structural similarities to peptide YY and pancreatic polypeptide. Nature. (1982) 296:659–60. doi: 10.1038/296659a0
147. Allen JM. Neuropeptide Y: its central and peripheral significance to molecular and cellular endocrinology. Princip Med Bio. (1997) 10:467–84. doi: 10.1016/S1569-2582(97)80166-3
148. Holzer P, Reichmann F, Farzi A. Neuropeptide Y, peptide YY and pancreatic polypeptide in the gut–brain axis. Neuropeptides. (2012) 46:261–74. doi: 10.1016/j.npep.2012.08.005
149. Kastin A. Handbook of biologically active peptides. San Diego: Academic press (2013) p. 1143–8.
150. Schwartz TW, Sheikh SP, O'Hare MM. Receptors on phaeochromocytoma cells for two members of the PP-fold family—NPY and PP. FEBS Lett. (1987) 225:209–14. doi: 10.1016/0014-5793(87)81159-6
151. Brogden KA, Guthmiller JM, Salzet M, Zasloff M. The nervous system and innate immunity: the neuropeptide connection. Nat Immunol. (2005) 6:558–64. doi: 10.1038/ni1209
152. Hansen CJ, Burnell KK, Brogden KA. Antimicrobial activity of Substance P and Neuropeptide Y against laboratory strains of bacteria and oral microorganisms. J Neuroimmunol. (2006) 177:215–8. doi: 10.1016/j.jneuroim.2006.05.011
153. Vouldoukis I, Shai Y, Nicolas P, Mor A. Broad spectrum antibiotic activity of skin-PYY. FEBS Lett. (1996) 380:237–40. doi: 10.1016/0014-5793(96)00050-6
154. Tsigelny I, Mahata SK, Taupenot L, Preece NE, Mahata M, Khan I, et al. Mechanism of action of chromogranin A on catecholamine release: molecular modeling of the catestatin region reveals a β-strand/loop/β-strand structure secured by hydrophobic interactions and predictive of activity. Regul Peptides. (1998) 77:43–53. doi: 10.1016/s0167-0115(98)00040-8
155. Aung G, Niyonsaba F, Ushio H, Kajiwara N, Saito H, Ikeda S, et al. Catestatin, a neuroendocrine antimicrobial peptide, induces human mast cell migration, degranulation and production of cytokines and chemokines. Immunology. (2011) 132:527–39. doi: 10.1111/j.1365-2567.2010.03395.x
156. Briolat J, Wu S, Mahata S, Gonthier B, Bagnard D, Chasserot-Golaz S, et al. New antimicrobial activity for the catecholamine release-inhibitory peptide from chromogranin A. Cell Mol Life Sci. (2005) 62:377–85. doi: 10.1007/s00018-004-4461-9
157. Radek KA, Lopez-Garcia B, Hupe M, Niesman IR, Elias PM, Taupenot L, et al. The neuroendocrine peptide catestatin is a cutaneous antimicrobial and induced in the skin after injury. J Invest Dermatol. (2008) 128:1525–34. doi: 10.1038/sj.jid.5701225
158. Galea I. The blood–brain barrier in systemic infection and inflammation. Cell Mol Immunol. (2021) 18:2489–501. doi: 10.1038/s41423-021-00757-x
159. Winkler B, Funke D, Benmimoun B, Spéder P, Rey S, Logan MA, et al. Brain inflammation triggers macrophage invasion across the blood-brain barrier in Drosophila during pupal stages. Sci Adv. (2021) 7:eabh0050. doi: 10.1126/sciadv.abh0050
160. Korir ML, Doster RS, Lu J, Guevara MA, Spicer SK, Moore RE, et al. Streptococcus agalactiae cadD alleviates metal stress and promotes intracellular survival in macrophages and ascending infection during pregnancy. Nat Commun. (2022) 13:5392. doi: 10.1038/s41467-022-32916-7
161. Weiss G, Schaible UE. Macrophage defense mechanisms against intracellular bacteria. Immunol Rev. (2015) 264:182–203. doi: 10.1111/imr.12266
162. Sullivan MJ, Goh KG, Ulett GC. Cellular management of zinc in group B Streptococcus supports bacterial resistance against metal intoxication and promotes disseminated infection. Msphere. (2021) 6:e00105–21. doi: 10.1128/mSphere.00105-21
163. Pidwill GR, Gibson JF, Cole J, Renshaw SA, Foster SJ. The role of macrophages in Staphylococcus aureus infection. Front Immunol. (2021) 11:620339. doi: 10.3389/fimmu.2020.620339
164. Cavinato L, Genise E, Luly FR, Di Domenico E,G, Del Porto P, Ascenzioni F. Escaping the phagocytic oxidative burst: the role of SODB in the survival of Pseudomonas aeruginosa within macrophages. Front Microbiol. (2020) 11:326. doi: 10.3389/fmicb.2020.00326
165. Yang Y, Wang Y, Guo L, Gao W, Tang TL, Yan M. Interaction between macrophages and ferroptosis. Cell Death Dis. (2022) 13:355. doi: 10.1038/s41419-022-04775-z
166. Awawdeh L, Lundy FT, Shaw C, Lamey PJ, Linden GJ, Kennedy JG. Quantitative analysis of substance P, neurokinin A and calcitonin gene-related peptide in pulp tissue from painful and healthy human teeth. Int Endod J. (2002) 35:30–6. doi: 10.1046/j.1365-2591.2002.00451.x
167. El-Karim I, Lundy FT, Linden GJ, Lamey PJ. Extraction and radioimmunoassay quantitation of neuropeptide Y (NPY) and vasoactive intestinal polypeptide (VIP) from human dental pulp tissue. Arch Oral Biol. (2003) 48:249–54. doi: 10.1016/s0003-9969(02)00213-3
168. El Karim IA, Lamey PJ, Ardill J, Linden GJ, Lundy FT. Vasoactive intestinal polypeptide (VIP) and VPAC1 receptor in adult human dental pulp in relation to caries. Arch Oral Biol. (2006) 51:849–55. doi: 10.1016/j.archoralbio.2006.04.009
169. Gonzalez-Rey E, Delgado M. Anti-inflammatory neuropeptide receptors: new therapeutic targets for immune disorders? Trends Pharmacol Sci. (2007) 28:482–91. doi: 10.1016/j.tips.2007.07.001
170. Klein JR. Dynamic interactions between the immune system and the neuroendocrine system in health and disease. Front Endocrinol. (2021) 12:655982. doi: 10.3389/fendo.2021.655982
171. Rivest S. Interactions between the immune and neuroendocrine systems. Prog Brain Res. (2010) 181:43–53. doi: 10.1016/S0079-6123(08)81004-7
172. Weigent DA, Blalock JE. Associations between the neuroendocrine and immune systems. J Leukocyte Biol. (1995) 58:137–50. doi: 10.1002/jlb.58.2.137
173. Blalock JE. The syntax of immune-neuroendocrine communication. Immunol Today. (1994) 15:504–11. doi: 10.1016/0167-5699(94)90205-4
174. ThyagaRajan S, Priyanka HP. Bidirectional communication between the neuroendocrine system and the immune system: relevance to health and diseases. Ann Neurosci. (2012) 19:40. doi: 10.5214/ans.0972.7531.180410
175. Parkin J, Cohen B. An overview of the immune system. Lancet. (2001) 357:1777–89. doi: 10.1016/S0140-6736(00)04904-7
176. Delgado M, Ganea D. Inhibition of endotoxin-induced macrophage chemokine production by vasoactive intestinal peptide and pituitary adenylate cyclase-activating polypeptide in vitro and in vivo. J Immunol. (2001) 167:966–75. doi: 10.4049/jimmunol.167.2.966
177. Taub DD. Novel connections between the neuroendocrine and immune systems: the ghrelin immunoregulatory network. Vitam Horm. (2007) 77:325–46. doi: 10.1016/S0083-6729(06)77014-5
178. Aresti Sanz J, El Aidy S. Microbiota and gut neuropeptides: a dual action of antimicrobial activity and neuroimmune response. Psychopharmacology. (2019) 236:1597–609. doi: 10.1007/s00213-019-05224-0
179. Lui PP, Ainali C, Chu CC, Terranova-Barberio M, Karagiannis P, Tewari A, et al. Human skin CD141+ dendritic cells regulate cutaneous immunity via the neuropeptide urocortin 2. Iscience. (2023) 26:108029. doi: 10.1016/j.isci.2023.108029
180. Rochlitzer S, Veres TZ, Kühne K, Prenzler F, Pilzner C, Knothe S, et al. The neuropeptide calcitonin gene-related peptide affects allergic airway inflammation by modulating dendritic cell function. Clin Exp Allergy. (2011) 41:1609–21. doi: 10.1111/j.1365-2222.2011.03822.x
Keywords: antimicrobial neuropeptides, anti-inflammatory neuropeptides, innate immunity, host defense, neuroimmunology
Citation: Li X, Chen K, Liu R, Zheng Z and Hou X (2024) Antimicrobial neuropeptides and their therapeutic potential in vertebrate brain infectious disease. Front. Immunol. 15:1496147. doi: 10.3389/fimmu.2024.1496147
Received: 13 September 2024; Accepted: 30 October 2024;
Published: 15 November 2024.
Edited by:
Jorge Cervantes, Nova Southeastern University, United StatesReviewed by:
Michael A. Zasloff, Georgetown University Medical Center, United StatesKate Carnevale, Boston University, United States
Copyright © 2024 Li, Chen, Liu, Zheng and Hou. This is an open-access article distributed under the terms of the Creative Commons Attribution License (CC BY). The use, distribution or reproduction in other forums is permitted, provided the original author(s) and the copyright owner(s) are credited and that the original publication in this journal is cited, in accordance with accepted academic practice. No use, distribution or reproduction is permitted which does not comply with these terms.
*Correspondence: Zhaodi Zheng, Wmhhb2RpQG1haWwuam5tYy5lZHUuY24=; Xitan Hou, aG91eGl0YW5AMTI2LmNvbQ==
†These authors have contributed equally to this work