- 1Chinese Academy of Medical Sciences Oxford Institute, University of Oxford, Oxford, United Kingdom
- 2Department of Dermatology, Oxford University Hospitals NHS Foundation Trust, Oxford, United Kingdom
- 3MRC Translational Immune Discovery Unit , University of Oxford, Oxford, United Kingdom
- 4Oxford Kidney and Transplant Unit, Oxford University Hospitals NHS Foundation Trust, Oxford, United Kingdom
Chronic kidney disease affects 1 in 10 people globally, with a prevalence twenty times that of cancer. A subset of individuals will progress to end-stage renal disease (ESRD) where renal replacement therapy is required to maintain health. Cutaneous disease, including xerosis and pruritus, are endemic amongst patients with ESRD. In the uraemia-associated immune deficiency of ESRD, impaired circulating immune responses contribute to increased infection risk and poorer vaccination response. Clinical manifestations of dysregulated adaptive immunity within the skin have been well-described and have been posited to play a role in cutaneous features of ESRD. However, our understanding of the mechanisms by which adaptive immunity within the skin is affected by uraemia is relatively limited. We provide an overview of how the cutaneous adaptive immune system is impacted both directly and indirectly by uraemia, highlighting that much work has been extrapolated from the circulating immune system and often has not been directly evaluated in the skin compartment. We identify knowledge gaps which may be addressed by future research. Ultimately, greater understanding of these pathways may facilitate novel therapeutic approaches to ameliorate widespread cutaneous symptomatology in ESRD.
Introduction
Chronic kidney disease (CKD) is a major cause of non-communicable morbidity and mortality, with approximately 850 million cases worldwide (10% of the global population) (1). This represents a twenty-fold greater prevalence compared to cancer (2). By 2040, CKD will be the fifth leading cause of years of life lost (3). A subset of individuals progress to end-stage renal disease (ESRD), requiring renal replacement therapy or kidney transplantation.
Advanced CKD and its associated physiological milieu (uraemia) has been long linked with systemic immune dysfunction. Reduced delayed-type hypersensitivity (DTH) responses and dampened responses to vaccination indicates this extends to peripheral sites such as skin (4, 5). Uraemic immune dysfunction culminates in increased infection risk and poorer outcomes (6, 7).
Skin issues are widespread in patients with advanced CKD (8). Shared pathogenic mechanisms may cause both renal disease and induce dermatopathology [for example, systemic lupus erythematosus or Henoch-Schönlein Purpura (IgA vasculitis)]; exploration of these is beyond the scope of this review. Independent of CKD aetiology, many cutaneous symptoms are overrepresented in uraemic patients. For example, pruritus affects at least half of patients, with increasing prevalence as disease progresses (9–12). Other uraemic skin manifestations include xerosis, acquired ichthyosis, altered pigmentation, purpura, and nail/mucosal changes (8). Cutaneous manifestations in CKD have been recently reviewed (13). Immunopathology has been postulated to contribute to many of these conditions.
As a barrier organ, almost every cell type in the skin is capable of initiating and maintaining an immune response. Previous experimental methods for studying the contribution of each to cutaneous immunity were limited in that they could analyze only a small number of markers simultaneously, necessitating pre-determined hypotheses, and/or lacked the ability to perform dynamic, functional assessments of behaviour.
New approaches, such as single-cell and spatial transcriptomic profiling, offer the ability to examine cell behaviour and signalling pathways across thousands of genes, often with the benefit of single-cell resolution or preservation of spatial context (14). These have revealed novel perspectives on cellular interactions in both skin homeostasis and pathology (15), uncovering previously unappreciated heterogeneity within cutaneous populations and permitting new insights into immune-stroma-epithelial crosstalk (16), permitting deployment of targeted therapies such as biologics targeting signalling pathways into clinical practice (17). Cutaneous immune profiling has revealed spatially and transcriptomically distinct leucocyte subpopulations, allowing distinction of specialised, skin-resident subsets, those transiently circulating through tissue, and those recruited in inflammation (18). Taken together, a picture is emerging of an incredibly complex immune network within the skin that acts in concert to protect against pathogens.
Development of adaptive immune responses within the skin require successful coordination of a series of communication networks, often commencing with Langerhans cell (LC) or dermal dendritic cell antigen acquisition and migration to draining lymph nodes (enhanced by inflammatory signalling from keratinocytes, fibroblasts and melanocytes) and culminating in recruitment of circulating lymphocytes through chemokine and cytokine signalling and upregulation of adhesion molecules by endothelial cells (19–23). Resolution of inflammation is accompanied by local retention of a small number of tissue-resident lymphocytes which subsequently act as sentinels and first responders, promoting rapid cutaneous memory responses following antigen re-encounter (24, 25). Cellular immune responses within skin are supported and complemented by humoral responses, with constitutive local production of antibody which is upregulated in the setting of inflammation (26–28). These processes are modulated by regulatory cutaneous leucocyte populations, such as regulatory T cells, which dampen inflammation (29). Dysregulation in any one of these steps may lead to skin pathology.
Much remains unclear about the interaction between uraemia and immune dysfunction, and how this extends to the skin. This review therefore presents an overview of the field, identifying knowledge gaps where new techniques may identify novel pathways and therapeutic approaches to ameliorate disease.
Changes in circulating adaptive immunity with uraemia
Kidney failure correlates with accumulation of circulating pro-inflammatory markers, an exhausted T cell phenotype, and skewing of T follicular helper subtypes, typically becoming more marked as kidney impairment progresses to ESRD (30, 31). Increased prevalence of virus-associated cancers, tuberculosis, and impaired vaccination response to T cell-dependent antigens in ESRD patients indicate a demonstrable impact of T cell impairment (32).
Circulating lymphopenia is common, becoming most profound in ESRD (33–35). T-cell lymphopenia may also be driven by increased T cell susceptibility to apoptosis (36). The mechanisms responsible for the impaired responses of CD4+ T lymphocytes in dialysis patients remains unclear. However, disruptions in T lymphocyte activation may be caused by the presence of uremic toxins and proinflammatory cytokines or may be associated with early proliferative senescence (37).
Advanced CKD may lead to imbalanced T cell responses, with a shift towards Th2 polarisation (38, 39). Dialysis may impair T cells’ ability to produce Th1 cytokines upon ex vivo stimulation, potentially indicating ‘exhaustion’ (40). Accordingly, ESRD is associated with accumulation of circulating T cells with an exhausted phenotype (41). Exhaustion, arising as a result of chronic antigenic stimulation and particularly described in the setting of cancer and chronic viral infection, leads to dampened effector function and the upregulation of inhibitory receptors (42, 43). Exhausted T cells may revert to fully functional T cells under certain conditions, but ultimately leads to cell death and impaired immune response if conditions persist (42–44). Other polarisation fates also may be affected, with a shift in the Th17: Treg axis towards Th17 responses described in CKD (45–47).
Despite a shift towards Th2 polarisation, usually associated with antibody-mediated responses, the production of antigen-specific effector memory CD4+ T cells after vaccination is severely impaired in patients with ESRD (32). This is crucial for achieving an adequate humoral response. As CKD progresses in adults, there is a reduction in circulating B cell number, particularly driven by loss of immature B cells and accumulation of double-negative B cells, which may play an inhibitory role in humoral responses (48, 49). Elevated plasma levels of IL-7 are found in uraemia, which promote the transformation of pre-B cells into B cells (50). B cell lymphopenia appears to be relevant prognostically in ESRD, with lower circulating B cell count associated with elevated all-cause mortality risk (51).
Children with CKD exhibit notably reduced numbers of memory type B cells, despite normal transitional B cell counts (52). This may be due to increased B cell susceptibility to apoptosis in uraemia, linked to reduced Bcl-2 expression (53). This suggests that B cell deficiency may be multifactorial with enhanced susceptibility of uraemic B cells to apoptosis and impaired maturation in the periphery through resistance to differentiation and survival signals.
Alterations in cutaneous immunity in uraemia
Uraemic immune dysfunction has been particularly studied in circulating populations, whereas understanding the effect upon peripheral immunity, particularly the skin, is limited. However, reduced delayed type hypersensitivity response and increased skin allograft survival in patients with advanced CKD (54), both of which require T cell mediated immunity, support the concept that immune dysfunction extends to the skin in uraemia. Most research in this area has described morphological rather than functional shifts in cutaneous immunity, based on histological analysis rather than dynamic evaluation.
Priming of cutaneous adaptive immune responses are likely to be altered in uraemia. Patients with advanced CKD demonstrate reduced LC density within the epidermis (55, 56). Whether this represents enhanced turnover, loss of self-renewal or altered migration remains unclear. LCs are equipped with Toll-like receptors (TLR) that allow them to directly detect pathogen-associated molecular patterns (PAMPs) from viruses and bacteria (57). This capability facilitates their phenotypic maturation and differential cytokine production, highlighting the crucial role of LCs in regulating skin immune responses. Intrarenal TLR2 and TLR4-mediated signalling play a role in initiating and aggravating CKD in various settings (58); extrarenal signalling similarly mediated through uraemic toxins may also be enhanced (59). Identifying the key endogenous ligands for TLRs involved in renal disease will be crucial for developing TLR blockade as a potential therapeutic approach for these conditions.
Keratinocytes also express pattern recognition receptors, including TLR that recognise highly conserved structures including microbial-derived lipopolysaccharides, flagellins and DNA sequences (60–63). Activation via TLR leads to secretion of proinflammatory cytokines and chemokines, driving recruitment and activation of circulating leucocytes, and upregulation of antigen presenting capability (64–67). Uraemic toxins stimulate TLR directly in vitro (68), and therefore may act in vivo to drive inflammation and trigger chronic LC migration out of the skin.
Fibroblasts, present in the dermal layer, are increasingly being recognised as active players in cutaneous immunity. Heterogeneity in fibroblast behaviour is increasingly apparent leading to delineation of subsets which modulate cutaneous immunity in health and disease (69–72). Indirect evidence, such as impaired response to growth hormone signalling (73), and impaired wound healing (74), suggests uraemia impacts cutaneous fibroblast behaviour, however the effect upon modulation of immune response remains unexplored. Shifts in both the type and distribution of T cell populations have been described in skin from non-diabetic patients with advanced CKD, with increased epidermal CD8+/NK and reduced dermal CD4+T cell density compared to non-uraemic controls (56). Changes in T cell localisation and density support the clinical observation that priming, and recruitment of the cutaneous adaptive immune response is altered in CKD. Though uraemia clearly impacts circulating B cells, it is not known whether this reflects changes in the cutaneous compartment. Alterations in circulatory and cutaneous immunity previously described in the setting of advanced CKD are summarized in Figure 1.
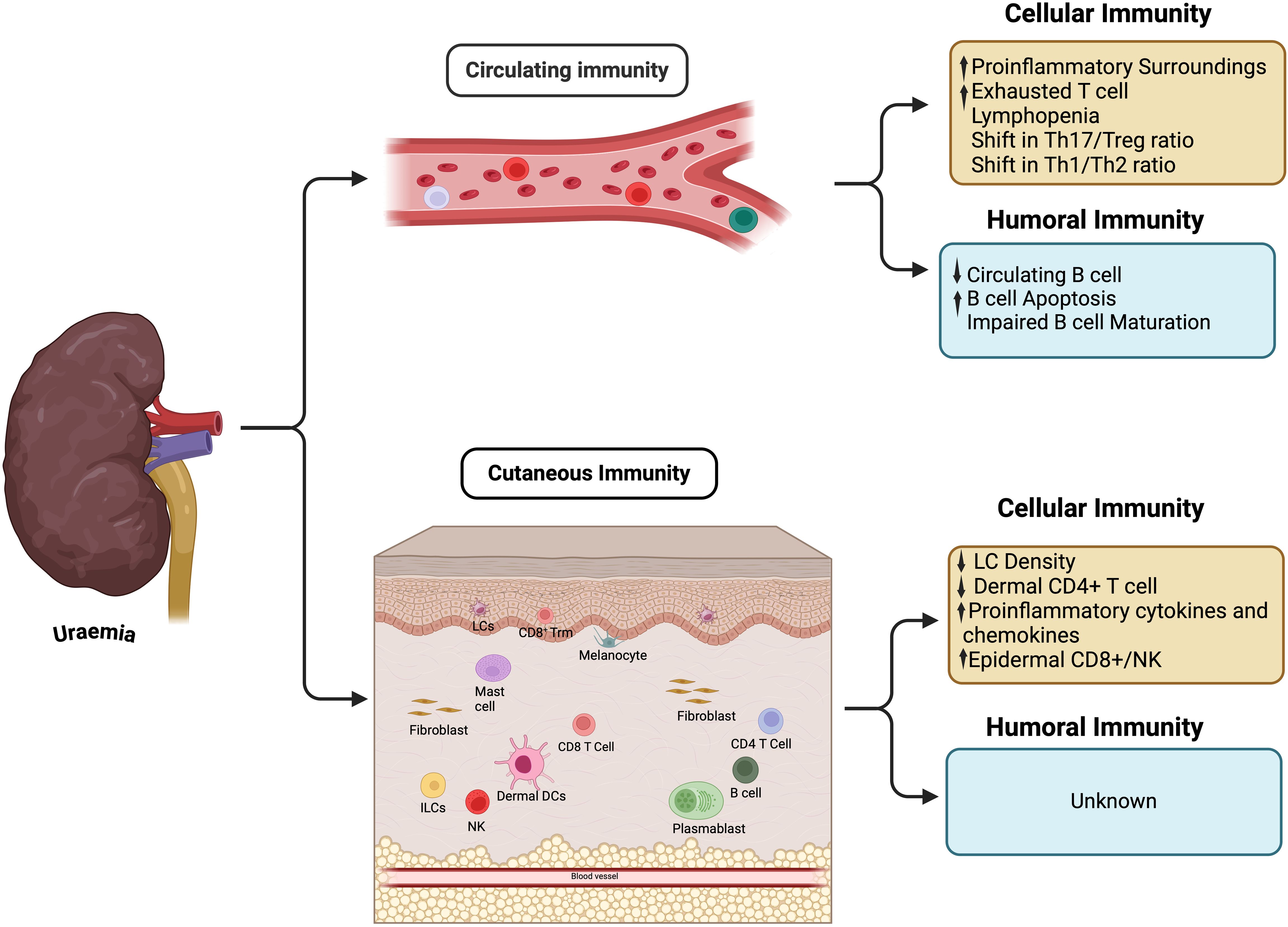
Figure 1. Immunity changes in circulation and cutaneous tissue. ILCs, Innate lymphoid cells; LCs, Langerhans cells; NK, Natural killer; Trm, Tissue resident memory.
Drivers of uraemic immune dysregulation
Changes in the microenvironment may drive uraemia-associated immune dysfunction both directly and indirectly
Direct effect: microinflammation and uraemia-associated toxicity
Uraemia is associated with chronic, low-level systemic inflammation termed ‘microinflammation’ (75), This is driven by oxidative stress associated with accumulation of proinflammatory cytokines, advanced glycation end-products (AGE), metalloproteases and overproduction of reactive oxygen species (76–79). Renal replacement therapy (i.e. dialysis) artificially removes many of these toxins from the body albeit in a relatively inefficient manner (80); this means that many of these products continue to accumulate even with treatment. Dialysis, particularly haemodialysis, may contribute to immune dysfunction and inflammation through dialysis membrane-driven activation of complement cascades and stimulation of circulating granulocytes, monocytes and T cells, and has been recently reviewed (81).
Microinflammation may directly influence cutaneous immunity and indeed uraemic pruritus may represent a clinical manifestation of this altered neuro-immunological interaction, though mechanistic understanding is limited (82, 83). Up to 40% of patients report this symptom, with pruritic individuals exhibiting increased serum C-reactive protein, as well as dysregulated circulating Th1/Th2 lymphocyte balance, compared to non-pruritic controls. Serum C-reactive protein and IL-6 levels are increased in pruritic uraemic individuals, with circulating T cells demonstrating Th1 skewing, compared to non-pruritic controls (84). There is an increase in the cytokines produced by Th1 lymphocytes including IFN-γ, IL-6, and TNF-α (76). In contrast, levels of IL-31, a Th2 lymphocyte pruritogenic cytokine, are increased in uraemic individuals (85). Microinflammation may impact upon cutaneous immunity through other mechanisms. For example, acute inflammatory signalling leads to upregulation of inflammatory cytokines by local fibroblasts followed by resolution of inflammation. However, in the setting of chronic inflammation fibroblast behaviour changes in a heterogenous manner - some differentiate into myofibroblasts leading to extracellular matrix deposition, which stiffens tissue and compromises leucocyte infiltration (86). Others differentiate into inflammatory fibroblasts that promote tissue retention of leucocytes. Which pathway is dominant in uraemic skin inflammation is unknown, but can be surmised to the former given the reduction in cutaneous leucocyte number in CKD.
Microinflammation may underpin the loss of LC seen in uraemic skin, though promotion of LC migration to draining lymph nodes (19). In the setting of chronic microinflammation unchecked LC migration may deplete the normally self-renewing cutaneous LC pool, with altered transendothelial trafficking preventing replenishment from the circulation (87).
Over 100 uraemia-associated toxic metabolites have been described, many of which have not been evaluated for their immunological effect (88). Indoxyl sulfate (IS) and p-cresyl sulfate (PCS) are two well-studied solutes, which exemplify how uraemic toxins may directly influence skin immunity and drive inflammation (89).
In vitro and animal models indicate IS and PCS may induce keratinocyte and fibroblast inflammation and drive histamine-independent itch (90–93). IS may also act directly upon skin-resident leucocytes, leading to differentiation and activation of proinflammatory macrophages (94–97). Ligation of the aryl-hydrocarbon receptor (AhR) on monocytes and macrophages by IS was shown to induce secretion of inflammatory mediators, driving chemotaxis and recruitment of effector T cells that induce endothelial apoptosis and promote further inflammation (98, 99). PCS induces circulating monocyte activation but impairs antigen processing (100). A recent study demonstrated PCS and IS synergise in contributing to the increasing proportion of pro-inflammatory, intermediate monocytes in CKD patients (101), suggesting that the cocktail of uraemic solutes present in vivo may have greater effects upon immunity than the study of individual molecules in vitro.
Uraemia-associated toxic metabolites may modulate T and B cell behaviour directly. IS inhibits Th2 cell differentiation, leading to loss of IL-4 producing CD4+ T cells, in an AhR-dependent manner in a murine model of asthma (102). This could drive Th1 skewing, inflammation and skin pathology in uraemic individuals. Blood PCS concentration correlates with levels of terminally differentiated CD8+ T cells in ESRD patients, potentially suggesting a mechanistic link between uraemic toxins and premature immune aging in patients with ESRD (33). B cells may be similarly activated by uraemic toxins through AhR. PCS directly leads to B cell lymphopenia in animal models (103), whilst AhR co-stimulatory signalling plays a critical role in driving B cell proliferation, but also regulates class switching and plasma cell differentiation, promoting development of a regulatory phenotype (104, 105).
Uraemia-associated microinflammation and toxin accumulation result in vascular inflammation, leading to endothelial dysfunction, prothrombotic changes, increased vascular permeability and accelerated vascular ageing (106, 107). In vitro studies have shown that the rupture of cell-cell junctions and reduced transendothelial electrical resistance indicated by uraemic toxins lead to structural damage of the endothelial monolayer, which could be associated with vascular injury and the development of chronic vascular diseases (108–110). This may enhance uraemic toxin and circulating inflammatory cytokine diffusion into peripheral tissues, including the skin (111). It’s unclear how this alters adhesion and tissue migration dynamics of circulating leucocytes, but conceivably could lead to impaired tissue infiltration if endothelial behaviour and signalling is dysregulated.
Ageing is associated with antigen-independent inflammation, leading to cutaneous recruitment of monocytes. Active vitamin D3 has been demonstrated to reduce this inflammation (112). 25-Vitamin D3 undergoes 1α hydroxylation in the kidney in order to acquire biological activity - consequently many patients with advanced CKD demonstrate functional vitamin D3 deficiency, requiring use of active vitamin D3 to prevent mineral bone disease. 25-Vitamin D3 reverses uraemia-associated inflammation in monocytes in vitro (113), but whether uraemia-associated skin inflammation may respond to this therapy in a clinical setting remains to be seen.
Electrolyte disturbance, associated with impaired clearance by the kidneys, may directly contribute to immune dysfunction. Total body sodium is frequently increased in uraemia. Skin is a major storage organ for sodium, where it exerts pleiotropic effects (114). Sodium may inhibit M2 macrophage differentiation and regulatory T cell function (115, 116), whilst simultaneously activating T cells (117). Similarly, hyperkalaemia is a common finding in patients with advanced CKD, with elevated potassium concentration having been shown to inhibit CD8+ T cell effector function (118). To what extent either of these mechanisms are active within uraemic skin are unclear but may play a contributory role to cutaneous immune dysfunction.
AGE are covalent compounds created by spontaneous (enzyme-independent) reaction of long-lived proteins, lipids and other macromolecules with monosaccharides and amino acids (119). Over time, this leads to pathophysiological cross-linked structures which alter tissue dynamics, including in skin (79). AGE accumulation is accelerated in the presence of oxidative stress, such as that found in CKD. Their accumulation in uraemia can be clinically quantified by fluorescence assessment (120) and may associate with poorer clinical outcomes (121, 122). AGE may act as a trigger for innate immune activation through TLR and a receptor for AGE (RAGE) expressed on cutaneous myofibrovascular populations, dendritic cells and monocytes (123–125), whilst potentiating oxidative stress and microinflammation in a positive feedback loop (126). Conversely, enhanced local fibrosis and tissue rigidity resulting from AGE accumulation has been suggested to prevent chemotaxis, particularly in neutrophils (127).
Taken together, uraemic patients accumulate electrolytes and AGEs due to reduced renal clearance and oxidative stress. Electrolyte imbalances may cause immune dysfunction by directly inhibiting the adaptive immune system, whereas AGEs may act more via initiation of innate immunity, exacerbating microinflammation. The latter synergises with deficiency in functional vitamin D3, leading to antigen-independent inflammation.
Indirect effect: uraemia-driven immunosenescence
A bidirectional link between chronic kidney disease and ageing is increasingly apparent. Whilst chronological age is a major risk factor for chronic kidney disease (128), progression of biological ageing may itself be accelerated in the uraemic milieu and is not reversed following kidney transplantation (129).
The immune system undergoes a progressive change in composition and functional capacity during biological ageing, termed immunosenescence. This manifests as increased susceptibility to infectious diseases, reduced response to vaccination, and elevated mortality risk in the general population (130–132). An underlying feature is ‘inflamm-ageing’, a chronic low-level non-specific inflammatory state with increased serum levels of C-reactive protein, IL-6 and IL-1β (133).
Although immunosenescence affects the entire immune system, most research has focused on the adaptive immune response, particularly in circulating T cells. Hallmarks of immunosenescence include thymic involution, a reduced naïve/memory ratio in circulating T and B cells and progressive loss in the functional and replicative capacity of lymphocytes (134–136).
T cell immunosenescence is typified by the accumulation of terminally differentiated, effector cells, characterized by loss of costimulatory molecules such as CD27 and CD28 and expression of killer cell lectin-like receptor subfamily G (KLRG-1) and CD57 (137, 138). Functionally, these cells demonstrate decreased replicative ability, increased production of proinflammatory cytokines and impaired TCR signal transduction with increased reliance upon killer receptor signalling (139–141).
The circulating immune landscape in uraemia shares many similarities with immunosenescence (142, 143). An average difference of 20 years between the immunological and chronological age in uraemic patients has been observed, indicating a prematurely aged T cell compartment (144). Microinflammation and oxidative stress drives accelerated thymic involution and premature loss of naïve T cell output (145). Accelerated peripheral T cell turnover may further contribute, with uraemic T cells demonstrating premature alterations in age-associated changes in TCR signalling cascades (146), and expression of early apoptotic markers (147, 148), suggesting increased susceptibility to activation-induced apoptosis (34). Many uraemic/ageing-related changes seen in circulating blood are also present in lymph nodes, albeit without accumulation of terminally differentiated T cells (149).
Cytomegalovirus (CMV) plays a major role in driving circulating T cell immunosenescence (150, 151). Uraemic patients demonstrate more frequent CMV reactivation, potentially creating a cycle of progressive immune impairment and increased CMV turnover (152, 153). The recent discovery of CMV-driven immune responses against senescent fibroblasts in skin by CD4+ T cells raise the question of whether a parallel CMV infection-immune circuit dominates cutaneous aging/uraemia (154).
Optimal responses against antigens by T cells are achieved through a broad TCR repertoire. However, reduced/skewed circulating TCR repertoire diversity is a hallmark of both immunosenescence and uraemia, and may therefore contribute to impaired responses (155, 156). Skewing occurs as accumulated terminally differentiated T cells are oligoclonal, often targeted against CMV (157, 158).
Other features of premature ageing, such as decreased CD4/CD8 ratio, are also seen and may be particularly marked in paediatric populations with renal impairment (35, 159, 160). Similarly, circulating B cells, NK cells, and dendritic cells demonstrate changes in ESRD comparable to aged individuals (50, 56, 161). Direct and in-direct effect of uraemia upon cutaneous immunity summarised in Figure 2.
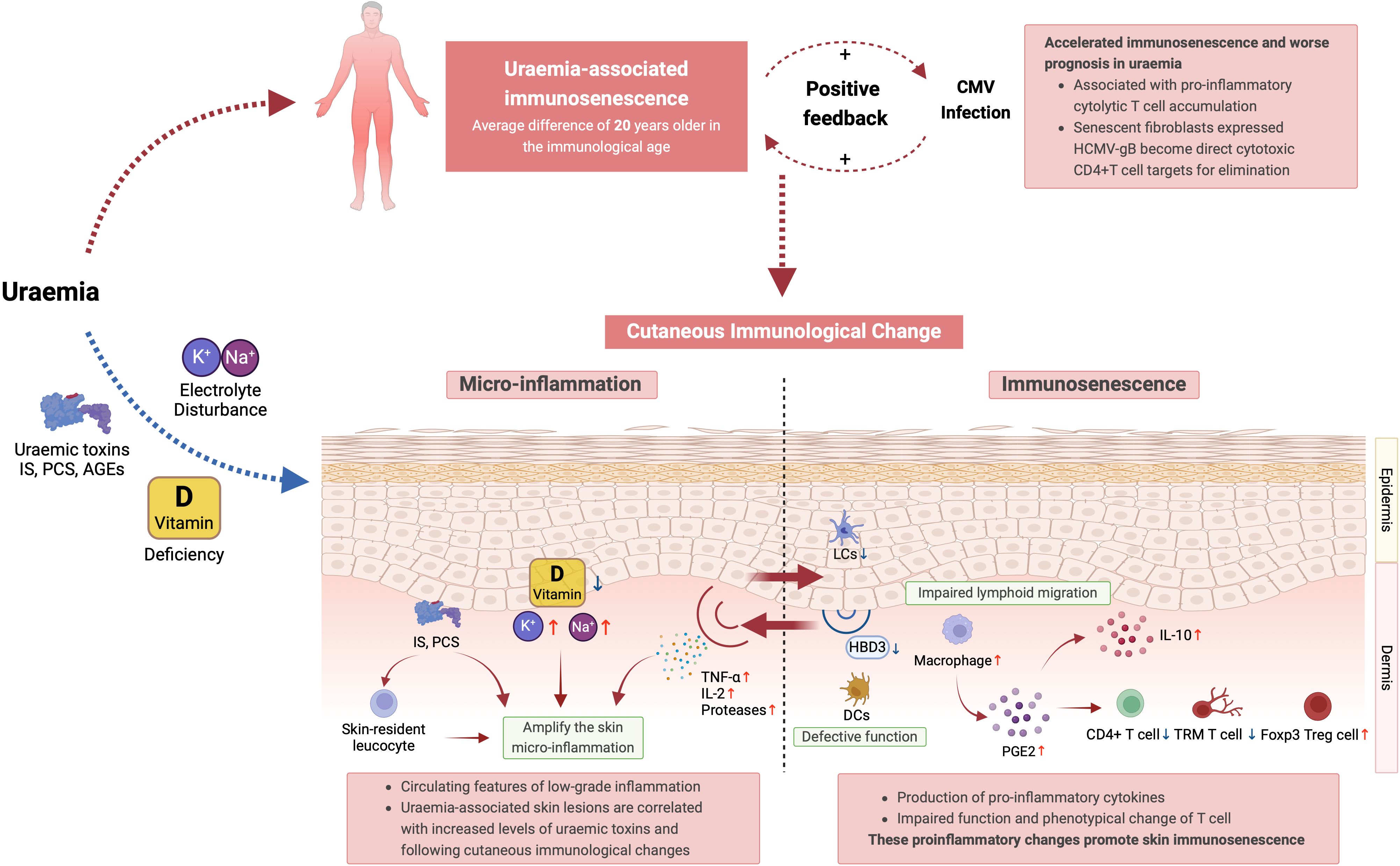
Figure 2. Summary of the direct and in-direct effects of uraemia upon cutaneous immunity. IS, indoxyl sulfate; PCS, p-cresyl sulfate; TCR, T cell receptor; NK Cell, natural killer cell; DCs, dendritic cells; HCMV-gB, human cytomegalovirus glycoprotein B; LCs, langerhans cells; HBD3, human beta-defensin 3; PGE2, prostaglandin E2; TRM T cells, tissue-resident memory T cells; Foxp3 Treg cells, forkhead box P3 regulatory T cells.
Cutaneous immunity in immunosenescence
Given the functional and phenotypical overlap between uraemia-associated immune deficiency and immunosenescence in blood, one may hypothesise this extends to the periphery also, although this has not been previously explored. Studies of ageing in skin may therefore be informative by extrapolation to uraemia and cutaneous immunity.
Cutaneous ageing may be associated with impaired adaptive immune priming and homing. A loss of LC and impaired lymphoid migration is seen in older people, akin to the loss of LC described in uraemia (162–164). Alongside impaired lymphocyte priming, this may also reduce the epidermal pool of defensins (165). Dermal dendritic cells also demonstrate defective trafficking, signalling and T cell stimulation from aged skin (166).
Macrophage-derived signalling is a major source of chronic, antigen-independent, low-level inflammation within aged skin (167). Aged skin demonstrates an altered response to damage, leading to enhanced recruitment of prostaglandin E2 (PGE2)-secreting inflammatory monocytes (168). These monocytes inhibit effector T cell activation and proliferation and induce expansion of FOXP3+Treg, which may explain their accumulation in aged skin (169–173). Their behaviour in uraemia is unknown.
In elderly individuals, cutaneous T cells maintain their density, diversity, and cytokine production, representing a long-lived, highly stable reservoir of immunity against infection and malignancy (174, 175). Decreased responses to Varicella Zoster virus (VZV) and tumour protection in older adults have not been directly attributed to an intrinsic defect within ageing CD4 TRMs (176). Instead, enhanced inhibitory signalling upon T cells from other leucocytes, particularly myeloid populations, may create an immunoregulatory microenvironment (177). Counterintuitively, excessive non-specific production of pro-inflammatory cytokines may cause inhibition of antigen-specific T-cell function (178).
Fibroblast senescence plays a major role in contributing to age-related chronic skin inflammation. Senescent fibroblasts secrete inflammatory mediators (termed the senescence associated secretory phenotype or SASP). This drives local inflammation, both in an antigen-independent manner but also through direct targeting of senescent cutaneous fibroblasts by effector T cell populations (154). It is feasible that uraemia amplifies production or downstream effects of SASP-related inflammation and skin ageing.
Studies on blood suggest significant overlap in ageing- and uraemia- associated immune dysfunction. Limited available data do provide tentative indications that this overlap may extend to skin, though further studies are needed to evaluate the function of cutaneous T cells in uraemia and how this mirrors biological ageing.
Outstanding questions and future research directions
The existing literature on the effect of uraemia upon immunity highlights a number of areas of limited understanding. Studies to date have typically focused on single populations, in vitro models or low plex, targeted gene expression or histological analyses. This has created inconsistences and disconnects.
One such example is how uraemic micro-inflammation seems to drive a Th1 shift in T cells, demonstrated by accumulation of cytolytic, senescent CD4+ and CD8+ T cells. However, the literature also suggests a shift towards a Th2 phenotype with reduced Th1 cytokine production amongst T cells. How do we reconcile this? The classical Th1/Th2 paradigm may be insufficiently complex to explain T cell behaviour in the setting of uraemia, leading to dysregulated T cell differentiation - as exemplified by impaired vaccine responses despite a Th2-skewed state. Alternatively, findings from studies in blood may not be reflective of the peripheral tissue microenvironment.
Deeper evaluation of cell behaviour in the setting of uraemia is needed to understand this more clearly. Novel profiling approaches, such as single-cell transcriptomic profiling and spatial profiling, offer the opportunity to re-examine cutaneous cell behaviour in an unbiased way, evaluating the whole transcriptome simultaneously across multiple cell populations. In addition to highlighting pathways driving intrinsic cell dysfunction, ‘stepping back’ to evaluate the relationship between spatially-approximate cells within tissue may reveal novel ligand-receptor interactions and signalling networks suitable for therapeutic manipulation. Spatial transcriptomic profiling offers the opportunity to do this using small quantities of archived skin samples without need for specific tissue processing prior to embedding (14).
Finally, it is unclear whether we can reverse this immune dysfunction. The parallels between ageing and uraemia raises the question of whether therapeutic approaches to slow or reverse tissue senescence and improve immune function may also be effective in CKD. ‘Senolytic’ agents, such as smal molecules like rapamycin (179), chimeric antigen receptor-T-cell (CAR-T) and TCR therapy (180) and immune checkpoint inhibitors (181) have shown encouraging potential in combating cellular senescence. Limiting CMV turnover could slow immune ageing - a small phase I study found improved vaccination response and reduced number of senescent CD4+ T cells in patients with vasculitis when treated with the antiviral valacyclovir (182). Interventional studies in patients with CKD are needed to evaluate whether these are effective approaches to improve uraemia-associated immune dysfunction.
Conclusion
Uraemia is commonly associated with skin disease, with relatively few specific therapies available for uraemic dermatopathology. In the setting of advanced kidney disease, altered immunity may play a critical contributory role in driving cutaneous symptomatology and predisposing to poorer antigen-specific responses.
Our understanding of these processes, outlined in this review, indicates notable alterations in the immunity of uraemic patients, yet the precise underlying causes of many of these changes remain largely elusive and have focused on circulating rather than tissue-resident leucocyte populations. While numerous studies have described the peripheral immune response in uraemic patients, there has been limited investigation into cutaneous immunity.
Our review highlights knowledge gaps in this field and emphasises the need for mechanistic investigations to identify novel therapeutic avenues to ameliorate symptoms and improve the substantial skin-related morbidity and mortality experienced by this cohort.
Author contributions
NZ: Investigation, Visualization, Writing – original draft, Writing – review & editing. ZL: Investigation, Visualization, Writing – original draft. GO: Supervision, Writing – review & editing. MB: Supervision, Writing – review & editing.
Funding
The author(s) declare financial support was received for the research, authorship, and/or publication of this article. MB, ZL and NZ are supported by grants from the British Skin Foundation, Oxford Hospital Charities, Oxford Transplant Foundation and Chinese Academy of Medical Sciences (CAMS) Innovation Fund for Medical Science (CIFMS), China (grant number: 2018-I2M-2-002). GO is supported by the Medical Research Council.
Acknowledgments
Figures in this manuscript were created using Biorender.com.
Conflict of interest
The authors declare that the research was conducted in the absence of any commercial or financial relationships that could be construed as a potential conflict of interest.
Publisher’s note
All claims expressed in this article are solely those of the authors and do not necessarily represent those of their affiliated organizations, or those of the publisher, the editors and the reviewers. Any product that may be evaluated in this article, or claim that may be made by its manufacturer, is not guaranteed or endorsed by the publisher.
Abbreviations
AhR, Aryl-hydrocarbon receptor; CKD, Chronic kidney disease; CMV, Cytomegalovirus; DTH, Delayed-type hypersensitivity; ESRD, End-stage renal failure; IS, Indoxyl sulfate; KLRG1, Killer Cell Lectin-like Receptor Superfamily G; LC, Langerhans cell; PCS, P-cresyl sulfate; PGE2, Prostaglandin E2; SASP, Senescence-associated secretory phenotype; TCR, T cell receptor; TLR, Toll-like receptor; VZV, Varicella zoster virus.
References
1. GBD Chronic Kidney Disease Collaboration. Global, regional, and national burden of chronic kidney disease, 1990-2017: a systematic analysis for the Global Burden of Disease Study 2017. Lancet. (2020) 395:709–33. doi: 10.1016/S0140-6736(20)30045-3
2. Jager KJ, Kovesdy C, Langham R, Rosenberg M, Jha V, Zoccali C. A single number for advocacy and communication-worldwide more than 850 million individuals have kidney diseases. Kidney Int. (2019) 96:1048–50. doi: 10.1016/j.kint.2019.07.012
3. Foreman KJ, Marquez N, Dolgert A, Fukutaki K, Fullman N, McGaughey M, et al. Forecasting life expectancy, years of life lost, and all-cause and cause-specific mortality for 250 causes of death: reference and alternative scenarios for 2016-40 for 195 countries and territories. Lancet. (2018) 392:2052–90. doi: 10.1016/S0140-6736(18)31694-5
4. Betjes MG. Immune cell dysfunction and inflammation in end-stage renal disease. Nat Rev Nephrol. (2013) 9:255–65. doi: 10.1038/nrneph.2013.44
5. Butani L, Calogiuri G. Hypersensitivity reactions in patients receiving hemodialysis. Ann Allergy Asthma Immunol. (2017) 118:680–4. doi: 10.1016/j.anai.2017.04.006
6. Cheikh Hassan HI, Tang M, Djurdjev O, Langsford D, Sood MM, Levin A. Infection in advanced chronic kidney disease leads to increased risk of cardiovascular events, end-stage kidney disease and mortality. Kidney Int. (2016) 90:897–904. doi: 10.1016/j.kint.2016.07.013
7. James MT, Laupland KB, Tonelli M, Manns BJ, Culleton BF, Hemmelgarn BR. Risk of bloodstream infection in patients with chronic kidney disease not treated with dialysis. Arch Intern Med. (2008) 168:2333–9. doi: 10.1001/archinte.168.21.2333
8. Goel V, Sil A, Das A. Cutaneous manifestations of chronic kidney disease, dialysis and post-renal transplant: A review. Indian J Dermatol. (2021) 66:3–11. doi: 10.4103/ijd.IJD_502_20
9. Abdallah AM, Elsheikh SM, ElBarbary RA. Prevalence and determinants of severity of uremic pruritus in hemodialysis patients: a multicentric study. J Investig Med. (2023) 71:42–6. doi: 10.1136/jim-2022-002360
10. Dyachenko P, Shustak A, Rozenman D. Hemodialysis-related pruritus and associated cutaneous manifestations. Int J Dermatol. (2006) 45:664–7. doi: 10.1111/j.1365-4632.2005.02592.x
11. Hu X, Sang Y, Yang M, Chen X, Tang W. Prevalence of chronic kidney disease-associated pruritus among adult dialysis patients: A meta-analysis of cross-sectional studies. Med (Baltimore). (2018) 97:e10633. doi: 10.1097/MD.0000000000010633
12. Zhao JH, Zhu QS, Li YW, Wang LL. Determinants of the intensity of uremic pruritus in patients receiving maintenance hemodialysis: A cross-sectional study. PloS One. (2021) 16:e0245370. doi: 10.1371/journal.pone.0245370
13. Arriaga Escamilla D, Lakhani A, Antony S, Salazar Villegas KN, Gupta M, Ramnath P, et al. Dermatological manifestations in patients with chronic kidney disease: A review. Cureus. (2024) 16:e52253. doi: 10.7759/cureus.52253
14. Kumaran G, Carroll L, Muirhead N, Bottomley MJ. How can spatial transcriptomic profiling advance our understanding of skin diseases? J Invest Dermatol. (2024). doi: 10.1016/j.jid.2024.07.006
15. Jovic D, Liang X, Zeng H, Lin L, Xu F, Luo Y. Single-cell RNA sequencing technologies and applications: A brief overview. Clin Transl Med. (2022) 12:e694. doi: 10.1002/ctm2.v12.3
16. Sol S, Boncimino F, Todorova K, Mandinova A. Unraveling the functional heterogeneity of human skin at single-cell resolution. Hematol Oncol Clin North Am. (2024) 38:921–38. doi: 10.1016/j.hoc.2024.05.001
17. Wride AM, Chen GF, Spaulding SL, Tkachenko E, Cohen JM. Biologics for psoriasis. Dermatol Clin. (2024) 42:339–55. doi: 10.1016/j.det.2024.02.001
18. Thai AA, Young RJ, Bressel M, Angel C, McDowell L, Tiong A, et al. Comprehensive profiling identifies tumour and immune microenvironmental differences in clinical subsets of cutaneous squamous cell carcinoma. Br J Dermatol. (2023) 189:588–602. doi: 10.1093/bjd/ljad250
19. Kashem SW, Haniffa M, Kaplan DH. Antigen-presenting cells in the skin. Annu Rev Immunol. (2017) 35:469–99. doi: 10.1146/annurev-immunol-051116-052215
20. Kortekaas Krohn I, Aerts JL, Breckpot K, Goyvaerts C, Knol E, Van Wijk F, et al. T-cell subsets in the skin and their role in inflammatory skin disorders. Allergy. (2022) 77:827–42. doi: 10.1111/all.15104
21. Kubo A, Nagao K, Yokouchi M, Sasaki H, Amagai M. External antigen uptake by Langerhans cells with reorganization of epidermal tight junction barriers. J Exp Med. (2009) 206:2937–46. doi: 10.1084/jem.20091527
22. Meyer M, Hensbergen PJ, van der Raaij-Helmer EM, Brandacher G, Margreiter R, Heufler C, et al. Cross reactivity of three T cell attracting murine chemokines stimulating the CXC chemokine receptor CXCR3 and their induction in cultured cells and during allograft rejection. Eur J Immunol. (2001) 31:2521–7. doi: 10.1002/1521-4141(200108)31:8<2521::AID-IMMU2521>3.0.CO;2-Q
23. Van Raemdonck K, Van den Steen PE, Liekens S, Van Damme J, Struyf S. CXCR3 ligands in disease and therapy. Cytokine Growth Factor Rev. (2015) 26:311–27. doi: 10.1016/j.cytogfr.2014.11.009
24. Mackay LK, Braun A, Macleod BL, Collins N, Tebartz C, Bedoui S, et al. Cutting edge: CD69 interference with sphingosine-1-phosphate receptor function regulates peripheral T cell retention. J Immunol. (2015) 194:2059–63. doi: 10.4049/jimmunol.1402256
25. Mackay LK, Stock AT, Ma JZ, Jones CM, Kent SJ, Mueller SN, et al. Long-lived epithelial immunity by tissue-resident memory T (TRM) cells in the absence of persisting local antigen presentation. Proc Natl Acad Sci U S A. (2012) 109:7037–42. doi: 10.1073/pnas.1202288109
26. Geherin SA, Gómez D, Glabman RA, Ruthel G, Hamann A, Debes GF. IL-10+ Innate-like B cells are part of the skin immune system and require α4β1 integrin to migrate between the peritoneum and inflamed skin. J Immunol. (2016) 196:2514–25. doi: 10.4049/jimmunol.1403246
27. Saul L, Ilieva KM, Bax HJ, Karagiannis P, Correa I, Rodriguez-Hernandez I, et al. IgG subclass switching and clonal expansion in cutaneous melanoma and normal skin. Sci Rep. (2016) 6:29736. doi: 10.1038/srep29736
28. van Laar JM. B-cell depletion with rituximab: a promising treatment for diffuse cutaneous systemic sclerosis. Arthritis Res Ther. (2010) 12:112. doi: 10.1186/ar2977
29. Ali N, Rosenblum MD. Regulatory T cells in skin. Immunology. (2017) 152:372–81. doi: 10.1111/imm.2017.152.issue-3
30. Girndt M, Sester U, Sester M, Kaul H, Köhler H. Impaired cellular immune function in patients with end-stage renal failure. Nephrol Dial Transplant. (1999) 14:2807–10. doi: 10.1093/ndt/14.12.2807
31. Hartzell S, Bin S, Cantarelli C, Haverly M, Manrique J, Angeletti A, et al. Kidney failure associates with T cell exhaustion and imbalanced follicular helper T cells. Front Immunol. (2020) 11:583702. doi: 10.3389/fimmu.2020.583702
32. Litjens NH, Huisman M, van den Dorpel M, Betjes MG. Impaired immune responses and antigen-specific memory CD4+ T cells in hemodialysis patients. J Am Soc Nephrol. (2008) 19:1483–90. doi: 10.1681/ASN.2007090971
33. Chiu YL, Shu KH, Yang FJ, Chou TY, Chen PM, Lay FY, et al. A comprehensive characterization of aggravated aging-related changes in T lymphocytes and monocytes in end-stage renal disease: the iESRD study. Immun Ageing. (2018) 15:27. doi: 10.1186/s12979-018-0131-x
34. Litjens NH, van Druningen CJ, Betjes MG. Progressive loss of renal function is associated with activation and depletion of naive T lymphocytes. Clin Immunol. (2006) 118:83–91. doi: 10.1016/j.clim.2005.09.007
35. Yoon JW, Gollapudi S, Pahl MV, Vaziri ND. Naïve and central memory T-cell lymphopenia in end-stage renal disease. Kidney Int. (2006) 70:371–6. doi: 10.1038/sj.ki.5001550
36. Borges A, Borges M, Fernandes J, Nascimento H, Sameiro-Faria M, Miranda V, et al. Apoptosis of peripheral CD4(+) T-lymphocytes in end-stage renal disease patients under hemodialysis and rhEPO therapies. Ren Fail. (2011) 33:138–43. doi: 10.3109/0886022X.2011.553300
37. Lisowska KA, Dębska-Ślizień A, Jasiulewicz A, Heleniak Z, Bryl E, Witkowski JM. Hemodialysis affects phenotype and proliferation of CD4-positive T lymphocytes. J Clin Immunol. (2012) 32:189–200. doi: 10.1007/s10875-011-9603-x
38. Alvarez-Lara MA, Carracedo J, Ramírez R, Martín-Malo A, Rodríguez M, Madueño JA, et al. The imbalance in the ratio of Th1 and Th2 helper lymphocytes in uraemia is mediated by an increased apoptosis of Th1 subset. Nephrol Dial Transplant. (2004) 19:3084–90. doi: 10.1093/ndt/gfh382
39. Libetta C, Rampino T, Dal Canton A. Polarization of T-helper lymphocytes toward the Th2 phenotype in uremic patients. Am J Kidney Dis. (2001) 38:286–95. doi: 10.1053/ajkd.2001.26092
40. Zamauskaite A, Perez-Cruz I, Yaqoob MM, Madrigal JA, Cohen SB. Effect of renal dialysis therapy modality on T cell cytokine production. Nephrol Dial Transplant. (1999) 14:49–55. doi: 10.1093/ndt/14.1.49
41. Ducloux D, Legendre M, Bamoulid J, Rebibou JM, Saas P, Courivaud C, et al. ESRD-associated immune phenotype depends on dialysis modality and iron status: clinical implications. Immun Ageing. (2018) 15:16. doi: 10.1186/s12979-018-0121-z
42. Wherry EJ, Kurachi M. Molecular and cellular insights into T cell exhaustion. Nat Rev Immunol. (2015) 15:486–99. doi: 10.1038/nri3862
43. Yi JS, Cox MA, Zajac AJ. T-cell exhaustion: characteristics, causes and conversion. Immunology. (2010) 129:474–81. doi: 10.1111/j.1365-2567.2010.03255.x
44. Blank CU, Haining WN, Held W, Hogan PG, Kallies A, Lugli E, et al. Defining ‘T cell exhaustion’. Nat Rev Immunol. (2019) 19:665–74. doi: 10.1038/s41577-019-0221-9
45. Hendrikx TK, van Gurp EA, Mol WM, Schoordijk W, Sewgobind VD, Ijzermans JN, et al. End-stage renal failure and regulatory activities of CD4+CD25bright+FoxP3+ T-cells. Nephrol Dial Transplant. (2009) 24:1969–78. doi: 10.1093/ndt/gfp005
46. Zhang J, Hua G, Zhang X, Tong R, Du X, Li Z. Regulatory T cells/T-helper cell 17 functional imbalance in uraemic patients on maintenance haemodialysis: a pivotal link between microinflammation and adverse cardiovascular events. Nephrol (Carlton). (2010) 15:33–41. doi: 10.1111/j.1440-1797.2009.01172.x
47. Zhu X, Li S, Zhang Q, Zhu D, Xu Y, Zhang P, et al. Correlation of increased Th17/Treg cell ratio with endoplasmic reticulum stress in chronic kidney disease. Med (Baltimore). (2018) 97:e10748. doi: 10.1097/MD.0000000000010748
48. Chen X, Guo H, Jin D, Lu Y, Zhang L. Distribution characteristics of circulating B cell subpopulations in patients with chronic kidney disease. Sci Rep. (2023) 13:20797. doi: 10.1038/s41598-023-47742-0
49. Sachinidis A, Garyfallos A. Double Negative (DN) B cells: A connecting bridge between rheumatic diseases and COVID-19? Mediterr J Rheumatol. (2021) 32:192–9. doi: 10.31138/mjr.32.3.192
50. Pahl MV, Gollapudi S, Sepassi L, Gollapudi P, Elahimehr R, Vaziri ND. Effect of end-stage renal disease on B-lymphocyte subpopulations, IL-7, BAFF and BAFF receptor expression. Nephrol Dial Transplant. (2010) 25:205–12. doi: 10.1093/ndt/gfp397
51. Molina M, Allende LM, Ramos LE, Gutiérrez E, Pleguezuelo DE, Hernández ER, et al. CD19(+) B-cells, a new biomarker of mortality in hemodialysis patients. Front Immunol. (2018) 9:1221. doi: 10.3389/fimmu.2018.01221
52. Bouts AH, Davin JC, Krediet RT, Monnens LA, Nauta J, Schröder CH, et al. Children with chronic renal failure have reduced numbers of memory B cells. Clin Exp Immunol. (2004) 137:589–94. doi: 10.1111/j.1365-2249.2004.02571.x
53. Fernández-Fresnedo G, Ramos MA, González-Pardo MC, de Francisco AL, López-Hoyos M, Arias M. B lymphopenia in uremia is related to an accelerated in vitro apoptosis and dysregulation of Bcl-2. Nephrol Dial Transplant. (2000) 15:502–10. doi: 10.1093/ndt/15.4.502
54. Dammin GJ, Couch NP, Murray JE. Prolonged survival of skin homografts in uremic patients. Ann N Y Acad Sci. (1957) 64:967–76. doi: 10.1111/j.1749-6632.1957.tb52488.x
55. McKerrow KJ, Hawthorn RJ, Thompson W. An investigation of circulating and in situ lymphocyte subsets and Langerhans cells in the skin and cervix of patients with chronic renal failure. Br J Dermatol. (1989) 120:745–55. doi: 10.1111/j.1365-2133.1989.tb01372.x
56. Mettang T, Fritz P, Weber J, Machleidt C, Hübel E, Kiefer T, et al. Epidermal Langerhans cells in uremic patients on hemodialysis or continuous ambulatory peritoneal dialysis. Nephron. (1993) 65:278–83. doi: 10.1159/000187488
57. Flacher V, Bouschbacher M, Verronèse E, Massacrier C, Sisirak V, Berthier-Vergnes O, et al. Human Langerhans cells express a specific TLR profile and differentially respond to viruses and Gram-positive bacteria. J Immunol. (2006) 177:7959–67. doi: 10.4049/jimmunol.177.11.7959
58. Fahmy HW, Moneim MS. The effect of prolonged blood stasis on a microarterial repair. J Reconstr Microsurg. (1988) 4:139–43. doi: 10.1055/s-2007-1006912
59. Esposito P, Verzola D, Saio M, Picciotto D, Frascio M, Laudon A, et al. The contribution of muscle innate immunity to uremic cachexia. Nutrients. (2023) 15:2832. doi: 10.3390/nu15132832
60. Elbe A, Stingl G. Dendritic cells as stimulator cells of MHC class I-restricted immune responses. Adv Exp Med Biol. (1995) 378:341–5. doi: 10.1007/978-1-4615-1971-3_76
61. Gieryńska M, Schollenberger A, Cespedes IS, Pawlak E. Dendritic epidermal T cells: sentinels of the skin. Postepy Hig Med Dosw (Online). (2009) 63:114–22.
62. Lai Y, Gallo RL. Toll-like receptors in skin infections and inflammatory diseases. Infect Disord Drug Targets. (2008) 8:144–55. doi: 10.2174/1871526510808030144
63. Newton K, Dixit VM. Signaling in innate immunity and inflammation. Cold Spring Harb Perspect Biol. (2012) 4:a006049. doi: 10.1101/cshperspect.a006049
64. Burgeson RE, Christiano AM. The dermal-epidermal junction. Curr Opin Cell Biol. (1997) 9:651–8. doi: 10.1016/S0955-0674(97)80118-4
65. Nestle FO, Di Meglio P, Qin JZ, Nickoloff BJ. Skin immune sentinels in health and disease. Nat Rev Immunol. (2009) 9:679–91. doi: 10.1038/nri2622
66. Sun L, Liu W, Zhang LJ. The role of toll-like receptors in skin host defense, psoriasis, and atopic dermatitis. J Immunol Res. (2019) 2019:1824624. doi: 10.1155/2019/1824624
67. Tay SS, Roediger B, Tong PL, Tikoo S, Weninger W. The skin-resident immune network. Curr Dermatol Rep. (2014) 3:13–22. doi: 10.1007/s13671-013-0063-9
68. Andrade LS, Dalboni MA, Carvalho JTG, Grabulosa CC, Pereira NBF, Aoike DT, et al. In vitro effect of uremic serum on barrier function and inflammation in human colonocytes. J Bras Nefrol. (2018) 40:217–24. doi: 10.1590/2175-8239-jbn-3949
69. Ascensión AM, Fuertes-Álvarez S, Ibañez-Solé O, Izeta A, Araúzo-Bravo MJ. Human dermal fibroblast subpopulations are conserved across single-cell RNA sequencing studies. J Invest Dermatol. (2021) 141:1735–44.e35. doi: 10.1016/j.jid.2020.11.028
70. He H, Suryawanshi H, Morozov P, Gay-Mimbrera J, Del Duca E, Kim HJ, et al. Single-cell transcriptome analysis of human skin identifies novel fibroblast subpopulation and enrichment of immune subsets in atopic dermatitis. J Allergy Clin Immunol. (2020) 145:1615–28. doi: 10.1016/j.jaci.2020.01.042
71. Solé-Boldo L, Raddatz G, Schütz S, Mallm JP, Rippe K, Lonsdorf AS, et al. Single-cell transcriptomes of the human skin reveal age-related loss of fibroblast priming. Commun Biol. (2020) 3:188. doi: 10.1038/s42003-020-0922-4
72. Vorstandlechner V, Laggner M, Kalinina P, Haslik W, Radtke C, Shaw L, et al. Deciphering the functional heterogeneity of skin fibroblasts using single-cell RNA sequencing. FASEB J. (2020) 34:3677–92. doi: 10.1096/fj.201902001RR
73. Ugarte F, Irarrazabal C, Oh J, Dettmar A, Ceballos ML, Rojo A, et al. Impaired phosphorylation of JAK2-STAT5b signaling in fibroblasts from uremic children. Pediatr Nephrol. (2016) 31:965–74. doi: 10.1007/s00467-015-3289-x
74. Maroz N, Simman R. Wound healing in patients with impaired kidney function. J Am Coll Clin Wound Spec. (2013) 5:2–7. doi: 10.1016/j.jccw.2014.05.002
75. Franceschi C, Garagnani P, Parini P, Giuliani C, Santoro A. Inflammaging: a new immune-metabolic viewpoint for age-related diseases. Nat Rev Endocrinol. (2018) 14:576–90. doi: 10.1038/s41574-018-0059-4
76. Pająk J, Nowicka D, Szepietowski JC. Inflammaging and immunosenescence as part of skin aging-A narrative review. Int J Mol Sci. (2013) 3:13–22. doi: 10.3390/ijms24097784
77. Pilkington SM, Bulfone-Paus S, Griffiths CEM, Watson REB. Inflammaging and the skin. J Invest Dermatol. (2021) 141:1087–95. doi: 10.1016/j.jid.2020.11.006
78. Sun J, Liu X, Shen C, Zhang W, Niu Y. Adiponectin receptor agonist AdipoRon blocks skin inflamm-ageing by regulating mitochondrial dynamics. Cell Prolif. (2021) 54:e13155. doi: 10.1111/cpr.v54.12
79. Wang L, Jiang Y, Zhao C. The effects of advanced glycation end-products on skin and potential anti-glycation strategies. Exp Dermatol. (2024) 33:e15065. doi: 10.1111/exd.15065
80. Tattersall J. Residual renal function in incremental dialysis. Clin Kidney J. (2018) 11:853–6. doi: 10.1093/ckj/sfy082
81. Angeletti A, Zappulo F, Donadei C, Cappuccilli M, Di Certo G, Conte D, et al. Immunological effects of a single hemodialysis treatment. Med (Kaunas). (2020) 56:71. doi: 10.3390/medicina56020071
82. Duque MI, Thevarajah S, Chan YH, Tuttle AB, Freedman BI, Yosipovitch G. Uremic pruritus is associated with higher kt/V and serum calcium concentration. Clin Nephrol. (2006) 66:184–91. doi: 10.5414/CNP66184
83. Manenti L, Tansinda P, Vaglio A. Uraemic pruritus: clinical characteristics, pathophysiology and treatment. Drugs. (2009) 69:251–63. doi: 10.2165/00003495-200969030-00002
84. Kimmel M, Alscher DM, Dunst R, Braun N, Machleidt C, Kiefer T, et al. The role of micro-inflammation in the pathogenesis of uraemic pruritus in haemodialysis patients. Nephrol Dial Transplant. (2006) 21:749–55. doi: 10.1093/ndt/gfi204
85. Ko MJ, Peng YS, Chen HY, Hsu SP, Pai MF, Yang JY, et al. Interleukin-31 is associated with uremic pruritus in patients receiving hemodialysis. J Am Acad Dermatol. (2014) 71:1151–9.e1. doi: 10.1016/j.jaad.2014.08.004
86. Buckley CD, Pilling D, Lord JM, Akbar AN, Scheel-Toellner D, Salmon M. Fibroblasts regulate the switch from acute resolving to chronic persistent inflammation. Trends Immunol. (2001) 22:199–204. doi: 10.1016/S1471-4906(01)01863-4
87. Otsuka M, Egawa G, Kabashima K. Uncovering the mysteries of langerhans cells, inflammatory dendritic epidermal cells, and monocyte-derived langerhans cell-like cells in the epidermis. Front Immunol. (2018) 9:1768. doi: 10.3389/fimmu.2018.01768
88. Duranton F, Cohen G, De Smet R, Rodriguez M, Jankowski J, Vanholder R, et al. Normal and pathologic concentrations of uremic toxins. J Am Soc Nephrol. (2012) 23:1258–70. doi: 10.1681/ASN.2011121175
89. Wang CP, Lu YC, Tsai IT, Tang WH, Hsu CC, Hung WC, et al. Increased levels of total p-cresylsulfate are associated with pruritus in patients with chronic kidney disease. Dermatology. (2016) 232:363–70. doi: 10.1159/000445429
90. Kim SJ, Zhang X, Cho SB, Kim CH, Park HC, Moon SJ. Uremic solutes of indoxyl sulfate and p-cresol enhance protease-activated receptor-2 expression in vitro and in vivo in keratinocytes. Hum Exp Toxicol. (2021) 40:113–23. doi: 10.1177/0960327120945758
91. Steinhoff M, Neisius U, Ikoma A, Fartasch M, Heyer G, Skov PS, et al. Proteinase-activated receptor-2 mediates itch: a novel pathway for pruritus in human skin. J Neurosci. (2003) 23:6176–80. doi: 10.1523/JNEUROSCI.23-15-06176.2003
92. Yosipovitch G. Dry skin and impairment of barrier function associated with itch - new insights. Int J Cosmet Sci. (2004) 26:1–7. doi: 10.1111/j.0142-5463.2004.00199.x
93. Lekawanvijit S, Adrahtas A, Kelly DJ, Kompa AR, Wang BH, Krum H. Does indoxyl sulfate, a uraemic toxin, have direct effects on cardiac fibroblasts and myocytes? Eur Heart J. (2010) 31:1771–9. doi: 10.1093/eurheartj/ehp574
94. Glorieux G, Gryp T, Perna A. Gut-derived metabolites and their role in immune dysfunction in chronic kidney disease. Toxins (Basel). (2020) 12:245. doi: 10.3390/toxins12040245
95. Ito S, Higuchi Y, Yagi Y, Nishijima F, Yamato H, Ishii H, et al. Reduction of indoxyl sulfate by AST-120 attenuates monocyte inflammation related to chronic kidney disease. J Leukoc Biol. (2013) 93:837–45. doi: 10.1189/jlb.0112023
96. Nakano T, Katsuki S, Chen M, Decano JL, Halu A, Lee LH, et al. Uremic toxin indoxyl sulfate promotes proinflammatory macrophage activation via the interplay of OATP2B1 and dll4-notch signaling. Circulation. (2019) 139:78–96. doi: 10.1161/CIRCULATIONAHA.118.034588
97. Stockler-Pinto MB, Soulage CO, Borges NA, Cardozo L, Dolenga CJ, Nakao LS, et al. From bench to the hemodialysis clinic: protein-bound uremic toxins modulate NF-κB/Nrf2 expression. Int Urol Nephrol. (2018) 50:347–54. doi: 10.1007/s11255-017-1748-y
98. Kim HY, Yoo TH, Cho JY, Kim HC, Lee WW. Indoxyl sulfate-induced TNF-α is regulated by crosstalk between the aryl hydrocarbon receptor, NF-κB, and SOCS2 in human macrophages. FASEB J. (2019) 33:10844–58. doi: 10.1096/fj.201900730R
99. Kim HY, Yoo TH, Hwang Y, Lee GH, Kim B, Jang J, et al. Indoxyl sulfate (IS)-mediated immune dysfunction provokes endothelial damage in patients with end-stage renal disease (ESRD). Sci Rep. (2017) 7:3057. doi: 10.1038/s41598-017-03130-z
100. Azevedo ML, Bonan NB, Dias G, Brehm F, Steiner TM, Souza WM, et al. p-Cresyl sulfate affects the oxidative burst, phagocytosis process, and antigen presentation of monocyte-derived macrophages. Toxicol Lett. (2016) 263:1–5. doi: 10.1016/j.toxlet.2016.10.006
101. Borges Bonan N, Schepers E, Pecoits-Filho R, Dhondt A, Pletinck A, De Somer F, et al. Contribution of the uremic milieu to an increased pro-inflammatory monocytic phenotype in chronic kidney disease. Sci Rep. (2019) 9:10236. doi: 10.1038/s41598-019-46724-5
102. Hwang YJ, Yun MO, Jeong KT, Park JH. Uremic toxin indoxyl 3-sulfate regulates the differentiation of Th2 but not of Th1 cells to lessen allergic asthma. Toxicol Lett. (2014) 225:130–8. doi: 10.1016/j.toxlet.2013.11.027
103. Espi M, Koppe L, Fouque D, Thaunat O. Chronic kidney disease-associated immune dysfunctions: impact of protein-bound uremic retention solutes on immune cells. Toxins (Basel). (2020) 12:300. doi: 10.3390/toxins12050300
104. Vaidyanathan B, Chaudhry A, Yewdell WT, Angeletti D, Yen WF, Wheatley AK, et al. The aryl hydrocarbon receptor controls cell-fate decisions in B cells. J Exp Med. (2017) 214:197–208. doi: 10.1084/jem.20160789
105. Villa M, Gialitakis M, Tolaini M, Ahlfors H, Henderson CJ, Wolf CR, et al. Aryl hydrocarbon receptor is required for optimal B-cell proliferation. EMBO J. (2017) 36:116–28. doi: 10.15252/embj.201695027
106. Cunha RSD, Santos AF, Barreto FC, Stinghen AEM. How do uremic toxins affect the endothelium? Toxins (Basel). (2020) 12:412. doi: 10.3390/toxins12060412
107. Druml W. Systemic consequences of acute kidney injury. Curr Opin Crit Care. (2014) 20:613–9. doi: 10.1097/MCC.0000000000000150
108. Chen SC, Huang SY, Wu CC, Hsu CF. P-cresylsulfate, the protein-bound uremic toxin, increased endothelial permeability partly mediated by src-induced phosphorylation of VE-cadherin. Toxins (Basel). (2020) 12:62. doi: 10.3390/toxins12020062
109. Maciel RAP, Cunha RS, Busato V, Franco CRC, Gregório PC, Dolenga CJR, et al. Uremia impacts VE-cadherin and ZO-1 expression in human endothelial cell-to-cell junctions. Toxins (Basel). (2018) 10:404. doi: 10.3390/toxins10100404
110. Tang WH, Wang CP, Yu TH, Tai PY, Liang SS, Hung WC, et al. Protein-bounded uremic toxin p-cresylsulfate induces vascular permeability alternations. Histochem Cell Biol. (2018) 149:607–17. doi: 10.1007/s00418-018-1662-0
111. Harper SJ, Tomson CR, Bates DO. Human uremic plasma increases microvascular permeability to water and proteins in vivo. Kidney Int. (2002) 61:1416–22. doi: 10.1046/j.1523-1755.2002.00252.x
112. Chambers ES, Vukmanovic-Stejic M, Turner CT, Shih BB, Trahair H, Pollara G, et al. Vitamin D(3) replacement enhances antigen-specific immunity in older adults. Immunother Adv. (2021) 1:ltaa008. doi: 10.1093/immadv/ltaa008
113. Brito RBO, Rebello JF, Grabulosa CC, Pinto W, Morales A Jr., Elias RM, et al. 25-vitamin D reduces inflammation in uremic environment. Sci Rep. (2020) 10:128. doi: 10.1038/s41598-019-56874-1
114. Chattopadhyay A, Tully J, Shan J, Sheikh S, Ohliger M, Gordon JW, et al. Sodium in the skin: a summary of the physiology and a scoping review of disease associations. Clin Exp Dermatol. (2023) 48:733–43. doi: 10.1093/ced/llad080
115. Binger KJ, Gebhardt M, Heinig M, Rintisch C, Schroeder A, Neuhofer W, et al. High salt reduces the activation of IL-4- and IL-13-stimulated macrophages. J Clin Invest. (2015) 125:4223–38. doi: 10.1172/JCI80919
116. Hernandez AL, Kitz A, Wu C, Lowther DE, Rodriguez DM, Vudattu N, et al. Sodium chloride inhibits the suppressive function of FOXP3+ regulatory T cells. J Clin Invest. (2015) 125:4212–22. doi: 10.1172/JCI81151
117. Matthias J, Maul J, Noster R, Meinl H, Chao YY, Gerstenberg H, et al. Sodium chloride is an ionic checkpoint for human T(H)2 cells and shapes the atopic skin microenvironment. Sci Transl Med. (2019) 11:eaau0683. doi: 10.1126/scitranslmed.aau0683
118. Eil R, Vodnala SK, Clever D, Klebanoff CA, Sukumar M, Pan JH, et al. Ionic immune suppression within the tumour microenvironment limits T cell effector function. Nature. (2016) 537:539–43. doi: 10.1038/nature19364
119. Gill V, Kumar V, Singh K, Kumar A, Kim JJ. Advanced glycation end products (AGEs) may be a striking link between modern diet and health. Biomolecules. (2019) 9:888. doi: 10.3390/biom9120888
120. Viramontes Hörner D, Taal MW. Skin autofluorescence: an emerging biomarker in persons with kidney disease. Curr Opin Nephrol Hypertens. (2019) 28:507–12. doi: 10.1097/MNH.0000000000000549
121. Viramontes Hörner D, Selby NM, Taal MW. Skin autofluorescence and malnutrition as predictors of mortality in persons receiving dialysis: a prospective cohort study. J Hum Nutr Diet. (2020) 33:852–61. doi: 10.1111/jhn.12764
122. Yabuuchi J, Ueda S, Yamagishi SI, Nohara N, Nagasawa H, Wakabayashi K, et al. Association of advanced glycation end products with sarcopenia and frailty in chronic kidney disease. Sci Rep. (2020) 10:17647. doi: 10.1038/s41598-020-74673-x
123. Brett J, Schmidt AM, Yan SD, Zou YS, Weidman E, Pinsky D, et al. Survey of the distribution of a newly characterized receptor for advanced glycation end products in tissues. Am J Pathol. (1993) 143:1699–712.
124. Lohwasser C, Neureiter D, Weigle B, Kirchner T, Schuppan D. The receptor for advanced glycation end products is highly expressed in the skin and upregulated by advanced glycation end products and tumor necrosis factor-alpha. J Invest Dermatol. (2006) 126:291–9. doi: 10.1038/sj.jid.5700070
125. Yue Q, Song Y, Liu Z, Zhang L, Yang L, Li J. Receptor for advanced glycation end products (RAGE): A pivotal hub in immune diseases. Molecules. (2022) 27:4922. doi: 10.3390/molecules27154922
126. Sultana R, Parveen A, Kang MC, Hong SM, Kim SY. Glyoxal-derived advanced glycation end products (GO-AGEs) with UVB critically induce skin inflammaging: in vitro and in silico approaches. Sci Rep. (2024) 14:1843. doi: 10.1038/s41598-024-52037-z
127. Hu H, Jiang H, Ren H, Hu X, Wang X, Han C. AGEs and chronic subclinical inflammation in diabetes: disorders of immune system. Diabetes Metab Res Rev. (2015) 31:127–37. doi: 10.1002/dmrr.v31.2
128. Mallappallil M, Friedman EA, Delano BG, McFarlane SI, Salifu MO. Chronic kidney disease in the elderly: evaluation and management. Clin Pract (Lond). (2014) 11:525–35. doi: 10.2217/cpr.14.46
129. Meijers RW, Litjens NH, de Wit EA, Langerak AW, Baan CC, Betjes MG. Uremia-associated immunological aging is stably imprinted in the T-cell system and not reversed by kidney transplantation. Transpl Int. (2014) 27:1272–84. doi: 10.1111/tri.12416
130. Goronzy JJ, Weyand CM. Understanding immunosenescence to improve responses to vaccines. Nat Immunol. (2013) 14:428–36. doi: 10.1038/ni.2588
131. Liu Z, Liang Q, Ren Y, Guo C, Ge X, Wang L, et al. Immunosenescence: molecular mechanisms and diseases. Signal Transduct Target Ther. (2023) 8:200. doi: 10.1038/s41392-023-01451-2
132. Walford RL. The immunologic theory of aging. Gerontologist. (1964) 4:195–7. doi: 10.1093/geront/4.4.195
133. Michaud M, Balardy L, Moulis G, Gaudin C, Peyrot C, Vellas B, et al. Proinflammatory cytokines, aging, and age-related diseases. J Am Med Dir Assoc. (2013) 14:877–82. doi: 10.1016/j.jamda.2013.05.009
134. Akbar AN, Henson SM, Lanna A. Senescence of T lymphocytes: implications for enhancing human immunity. Trends Immunol. (2016) 37:866–76. doi: 10.1016/j.it.2016.09.002
135. Frasca D, Diaz A, Romero M, Garcia D, Blomberg BB. B cell immunosenescence. Annu Rev Cell Dev Biol. (2020) 36:551–74. doi: 10.1146/annurev-cellbio-011620-034148
136. Goronzy JJ, Weyand CM. Successful and maladaptive T cell aging. Immunity. (2017) 46:364–78. doi: 10.1016/j.immuni.2017.03.010
137. Fagnoni FF, Vescovini R, Mazzola M, Bologna G, Nigro E, Lavagetto G, et al. Expansion of cytotoxic CD8+ CD28- T cells in healthy ageing people, including centenarians. Immunology. (1996) 88:501–7. doi: 10.1046/j.1365-2567.1996.d01-689.x
138. Lian J, Yue Y, Yu W, Zhang Y. Immunosenescence: a key player in cancer development. J Hematol Oncol. (2020) 13:151. doi: 10.1186/s13045-020-00986-z
139. Fantecelle CH, Covre LP, Garcia de Moura R, Guedes HLM, Amorim CF, Scott P, et al. Transcriptomic landscape of skin lesions in cutaneous leishmaniasis reveals a strong CD8(+) T cell immunosenescence signature linked to immunopathology. Immunology. (2021) 164:754–65. doi: 10.1111/imm.v164.4
140. Jallah BP, Kuypers DRJ. Impact of immunosenescence in older kidney transplant recipients: associated clinical outcomes and possible risk stratification for immunosuppression reduction. Drugs Aging. (2024) 41:219–38. doi: 10.1007/s40266-024-01100-5
141. Pereira BI, Akbar AN. Convergence of innate and adaptive immunity during human aging. Front Immunol. (2016) 7:445. doi: 10.3389/fimmu.2016.00445
142. Sampani E, Stangou M, Daikidou DV, Nikolaidou V, Asouchidou D, Dimitriadis C, et al. Influence of end stage renal disease on CD28 expression and T-cell immunity. Nephrol (Carlton). (2021) 26:185–96. doi: 10.1111/nep.13784
143. Van Laecke S, Van Damme K, Dendooven A. Immunosenescence: an unexplored role in glomerulonephritis. Clin Transl Immunol. (2022) 11:e1427. doi: 10.1002/cti2.1427
144. Betjes MG, Langerak AW, van der Spek A, de Wit EA, Litjens NH. Premature aging of circulating T cells in patients with end-stage renal disease. Kidney Int. (2011) 80:208–17. doi: 10.1038/ki.2011.110
145. Betjes MG. Uremia-associated ageing of the thymus and adaptive immune responses. Toxins (Basel). (2020) 12:224. doi: 10.3390/toxins12040224
146. Huang L, Litjens NHR, Kannegieter NM, Klepper M, Baan CC, Betjes MGH. pERK-dependent defective TCR-mediated activation of CD4(+) T cells in end-stage renal disease patients. Immun Ageing. (2017) 14:14. doi: 10.1186/s12979-017-0096-1
147. Meier P, Dayer E, Blanc E, Wauters JP. Early T cell activation correlates with expression of apoptosis markers in patients with end-stage renal disease. J Am Soc Nephrol. (2002) 13:204–12. doi: 10.1681/ASN.V131204
148. Moser B, Roth G, Brunner M, Lilaj T, Deicher R, Wolner E, et al. Aberrant T cell activation and heightened apoptotic turnover in end-stage renal failure patients: a comparative evaluation between non-dialysis, haemodialysis, and peritoneal dialysis. Biochem Biophys Res Commun. (2003) 308:581–5. doi: 10.1016/S0006-291X(03)01389-5
149. Dedeoglu B, de Weerd AE, Huang L, Langerak AW, Dor FJ, Klepper M, et al. Lymph node and circulating T cell characteristics are strongly correlated in end-stage renal disease patients, but highly differentiated T cells reside within the circulation. Clin Exp Immunol. (2017) 188:299–310. doi: 10.1111/cei.12934
150. Pawelec G. Immunosenenescence: role of cytomegalovirus. Exp Gerontol. (2014) 54:1–5. doi: 10.1016/j.exger.2013.11.010
151. Tu W, Rao S. Mechanisms underlying T cell immunosenescence: aging and cytomegalovirus infection. Front Microbiol. (2016) 7:2111. doi: 10.3389/fmicb.2016.02111
152. Betjes MG, Huisman M, Weimar W, Litjens NH. Expansion of cytolytic CD4+CD28- T cells in end-stage renal disease. Kidney Int. (2008) 74:760–7. doi: 10.1038/ki.2008.301
153. Betjes MG, Litjens NH, Zietse R. Seropositivity for cytomegalovirus in patients with end-stage renal disease is strongly associated with atherosclerotic disease. Nephrol Dial Transplant. (2007) 22:3298–303. doi: 10.1093/ndt/gfm348
154. Hasegawa T, Oka T, Son HG, Oliver-García VS, Azin M, Eisenhaure TM, et al. Cytotoxic CD4(+) T cells eliminate senescent cells by targeting cytomegalovirus antigen. Cell. (2023) 186:1417–31.e20. doi: 10.1016/j.cell.2023.02.033
155. Naylor K, Li G, Vallejo AN, Lee WW, Koetz K, Bryl E, et al. The influence of age on T cell generation and TCR diversity. J Immunol. (2005) 174:7446–52. doi: 10.4049/jimmunol.174.11.7446
156. Britanova OV, Putintseva EV, Shugay M, Merzlyak EM, Turchaninova MA, Staroverov DB, et al. Age-related decrease in TCR repertoire diversity measured with deep and normalized sequence profiling. J Immunol. (2014) 192:2689–98. doi: 10.4049/jimmunol.1302064
157. Huang L, Betjes MGH, Klepper M, Langerak AW, Baan CC, Litjens NHR. End-stage renal disease causes skewing in the TCR Vβ-repertoire primarily within CD8(+) T cell subsets. Front Immunol. (2017) 8:1826. doi: 10.3389/fimmu.2017.01826
158. Huang L, Langerak AW, Wolvers-Tettero IL, Meijers RW, Baan CC, Litjens NH, et al. End stage renal disease patients have a skewed T cell receptor Vβ repertoire. Immun Ageing. (2015) 12:28. doi: 10.1186/s12979-015-0055-7
159. Crépin T, Legendre M, Carron C, Vachey C, Courivaud C, Rebibou JM, et al. Uraemia-induced immune senescence and clinical outcomes in chronic kidney disease patients. Nephrol Dial Transplant. (2020) 35:624–32. doi: 10.1093/ndt/gfy276
160. George RP, Mehta AK, Perez SD, Winterberg P, Cheeseman J, Johnson B, et al. Premature T cell senescence in pediatric CKD. J Am Soc Nephrol. (2017) 28:359–67. doi: 10.1681/ASN.2016010053
161. Vacher-Coponat H, Brunet C, Lyonnet L, Bonnet E, Loundou A, Sampol J, et al. Natural killer cell alterations correlate with loss of renal function and dialysis duration in uraemic patients. Nephrol Dial Transplant. (2008) 23:1406–14. doi: 10.1093/ndt/gfm596
162. Bhushan M, Cumberbatch M, Dearman RJ, Andrew SM, Kimber I, Griffiths CE. Tumour necrosis factor-alpha-induced migration of human Langerhans cells: the influence of ageing. Br J Dermatol. (2002) 146:32–40. doi: 10.1046/j.1365-2133.2002.04549.x
163. Cumberbatch M, Dearman RJ, Kimber I. Influence of ageing on Langerhans cell migration in mice: identification of a putative deficiency of epidermal interleukin-1beta. Immunology. (2002) 105:466–77. doi: 10.1046/j.1365-2567.2002.01381.x
164. Pilkington SM, Ogden S, Eaton LH, Dearman RJ, Kimber I, Griffiths CEM. Lower levels of interleukin-1β gene expression are associated with impaired Langerhans’ cell migration in aged human skin. Immunology. (2018) 153:60–70. doi: 10.1111/imm.2018.153.issue-1
165. Pilkington SM, Dearman RJ, Kimber I, Griffiths CEM. Langerhans cells express human β-defensin 3: relevance for immunity during skin ageing. Br J Dermatol. (2018) 179:1170–1. doi: 10.1111/bjd.16770
166. Grolleau-Julius A, Harning EK, Abernathy LM, Yung RL. Impaired dendritic cell function in aging leads to defective antitumor immunity. Cancer Res. (2008) 68:6341–9. doi: 10.1158/0008-5472.CAN-07-5769
167. Guimarães GR, Almeida PP, de Oliveira Santos L, Rodrigues LP, de Carvalho JL, Boroni M. Hallmarks of aging in macrophages: consequences to skin inflammaging. Cells. (2021) 10:1323. doi: 10.3390/cells10061323
168. Chambers ES, Vukmanovic-Stejic M, Shih BB, Trahair H, Subramanian P, Devine OP, et al. Recruitment of inflammatory monocytes by senescent fibroblasts inhibits antigen-specific tissue immunity during human aging. Nat Aging. (2021) 1:101–13. doi: 10.1038/s43587-020-00010-6
169. Lages CS, Suffia I, Velilla PA, Huang B, Warshaw G, Hildeman DA, et al. Functional regulatory T cells accumulate in aged hosts and promote chronic infectious disease reactivation. J Immunol. (2008) 181:1835–48. doi: 10.4049/jimmunol.181.3.1835
170. MacKenzie KF, Clark K, Naqvi S, McGuire VA, Nöehren G, Kristariyanto Y, et al. PGE(2) induces macrophage IL-10 production and a regulatory-like phenotype via a protein kinase A-SIK-CRTC3 pathway. J Immunol. (2013) 190:565–77. doi: 10.4049/jimmunol.1202462
171. Sharma S, Yang SC, Zhu L, Reckamp K, Gardner B, Baratelli F, et al. Tumor cyclooxygenase-2/prostaglandin E2-dependent promotion of FOXP3 expression and CD4+ CD25+ T regulatory cell activities in lung cancer. Cancer Res. (2005) 65:5211–20. doi: 10.1158/0008-5472.CAN-05-0141
172. Vukmanovic-Stejic M, Agius E, Booth N, Dunne PJ, Lacy KE, Reed JR, et al. The kinetics of CD4+Foxp3+ T cell accumulation during a human cutaneous antigen-specific memory response in vivo. J Clin Invest. (2008) 118:3639–50. doi: 10.1172/JCI35834
173. Vukmanovic-Stejic M, Sandhu D, Sobande TO, Agius E, Lacy KE, Riddell N, et al. Varicella zoster-specific CD4+Foxp3+ T cells accumulate after cutaneous antigen challenge in humans. J Immunol. (2013) 190:977–86. doi: 10.4049/jimmunol.1201331
174. Koguchi-Yoshioka H, Hoffer E, Cheuk S, Matsumura Y, Vo S, Kjellman P, et al. Skin T cells maintain their diversity and functionality in the elderly. Commun Biol. (2021) 4:13. doi: 10.1038/s42003-020-01551-7
175. Park SL, Buzzai A, Rautela J, Hor JL, Hochheiser K, Effern M, et al. Tissue-resident memory CD8(+) T cells promote melanoma-immune equilibrium in skin. Nature. (2019) 565:366–71. doi: 10.1038/s41586-018-0812-9
176. Vukmanovic-Stejic M, Sandhu D, Seidel JA, Patel N, Sobande TO, Agius E, et al. The characterization of varicella zoster virus-specific T cells in skin and blood during aging. J Invest Dermatol. (2015) 135:1752–62. doi: 10.1038/jid.2015.63
177. Vukmanovic-Stejic M, Chambers ES, Suárez-Fariñas M, Sandhu D, Fuentes-Duculan J, Patel N, et al. Enhancement of cutaneous immunity during aging by blocking p38 mitogen-activated protein (MAP) kinase-induced inflammation. J Allergy Clin Immunol. (2018) 142:844–56. doi: 10.1016/j.jaci.2017.10.032
178. Franceschi C, Campisi J. Chronic inflammation (inflammaging) and its potential contribution to age-associated diseases. J Gerontol A Biol Sci Med Sci. (2014) 69 Suppl 1:S4–9. doi: 10.1093/gerona/glu057
179. Mannick JB, Morris M, Hockey HP, Roma G, Beibel M, Kulmatycki K, et al. TORC1 inhibition enhances immune function and reduces infections in the elderly. Sci Transl Med. (2018) 10:eaaq1564. doi: 10.1126/scitranslmed.aaq1564
180. Giallongo C, Parrinello NL, La Cava P, Camiolo G, Romano A, Scalia M, et al. Monocytic myeloid-derived suppressor cells as prognostic factor in chronic myeloid leukaemia patients treated with dasatinib. J Cell Mol Med. (2018) 22:1070–80. doi: 10.1111/jcmm.2018.22.issue-2
181. Viganò S, Perreau M, Pantaleo G, Harari A. Positive and negative regulation of cellular immune responses in physiologic conditions and diseases. Clin Dev Immunol. (2012) 2012:485781. doi: 10.1155/2012/485781
182. Chanouzas D, Sagmeister M, Faustini S, Nightingale P, Richter A, Ferro CJ++++++++++++++++++++++++++++++++hhhh, et al. Subclinical reactivation of cytomegalovirus drives CD4+CD28null T-cell expansion and impaired immune response to pneumococcal vaccination in antineutrophil cytoplasmic antibody-associated vasculitis. J Infect Dis. (2019) 219:234–44. doi: 10.1093/infdis/jiy493
Keywords: adaptive immune, cutaneous changes, immunosenescence, inflammation, uraemia
Citation: Zibandeh N, Li Z, Ogg G and Bottomley MJ (2024) Cutaneous adaptive immunity and uraemia: a narrative review. Front. Immunol. 15:1464338. doi: 10.3389/fimmu.2024.1464338
Received: 13 July 2024; Accepted: 12 September 2024;
Published: 27 September 2024.
Edited by:
Zhichao Fan, UCONN Health, United StatesReviewed by:
Mathieu Garand, Washington University in St. Louis, United StatesCopyright © 2024 Zibandeh, Li, Ogg and Bottomley. This is an open-access article distributed under the terms of the Creative Commons Attribution License (CC BY). The use, distribution or reproduction in other forums is permitted, provided the original author(s) and the copyright owner(s) are credited and that the original publication in this journal is cited, in accordance with accepted academic practice. No use, distribution or reproduction is permitted which does not comply with these terms.
*Correspondence: Matthew J. Bottomley, bWF0dGhldy5ib3R0b21sZXlAbmRtLm94LmFjLnVr
†These authors have contributed equally to this work