- 1Amity Institute of Biotechnology, Amity University Rajasthan, Jaipur, India
- 2Research and Scientific Studies Unit, College of Nursing and Allied Health Sciences, Jazan University, Jazan, Saudi Arabia
- 3Department of Obstetrics and Gynecology, Kyung Hee University Medical Center, Seoul, Republic of Korea
- 4Plasma Bioscience Research Center, Applied Plasma Medicine Center, Department of Electrical & Biological Physics, Kwangwoon University, Seoul, Republic of Korea
- 5Department of Biologics, College of Pharmacy, Hambakmoeiro 191, Yeonsu-gu, Incheon, Republic of Korea
Triple-negative breast cancer (TNBC) stands as the most complex and daunting subtype of breast cancer affecting women globally. Regrettably, treatment options for TNBC remain limited due to its clinical complexity. However, immunotherapy has emerged as a promising avenue, showing success in developing effective therapies for advanced cases and improving patient outcomes. Improving TNBC treatments involves reducing side effects, minimizing systemic toxicity, and enhancing efficacy. Unlike traditional cancer immunotherapy, engineered nonmaterial’s can precisely target TNBC, facilitating immune cell access, improving antigen presentation, and triggering lasting immune responses. Nanocarriers with enhanced sensitivity and specificity, specific cellular absorption, and low toxicity are gaining attention. Nanotechnology-driven immunoengineering strategies focus on targeted delivery systems using multifunctional molecules for precise tracking, diagnosis, and therapy in TNBC. This study delves into TNBC’s tumour microenvironment (TME) remodeling, therapeutic resistance, and immunoengineering strategies using nanotechnology.
Introduction
Breast cancer is developing in the breast tissue. It is one of the most common forms of cancer in women but can also be found in men, albeit less frequently (1). Breast cancer can originate in various parts of the breast, including the milk ducts, lobules, or connective tissue (2). Triple-negative breast cancer (TNBC) is a highly aggressive subtype with significant intra-tumoral heterogeneity that often develops resistance to treatments (2). TNBC are classified into six subgroups: immunomodulatory, mesenchymal, luminal androgen receptor, basal-like 1, basal-like 2, and mesenchymal stem cells, based on the results of the transcriptome analysis (Figure 1). Tumour heterogeneity and a lack of biomarkers offer significant hurdles in overcoming treatment resistance and relapse (3).
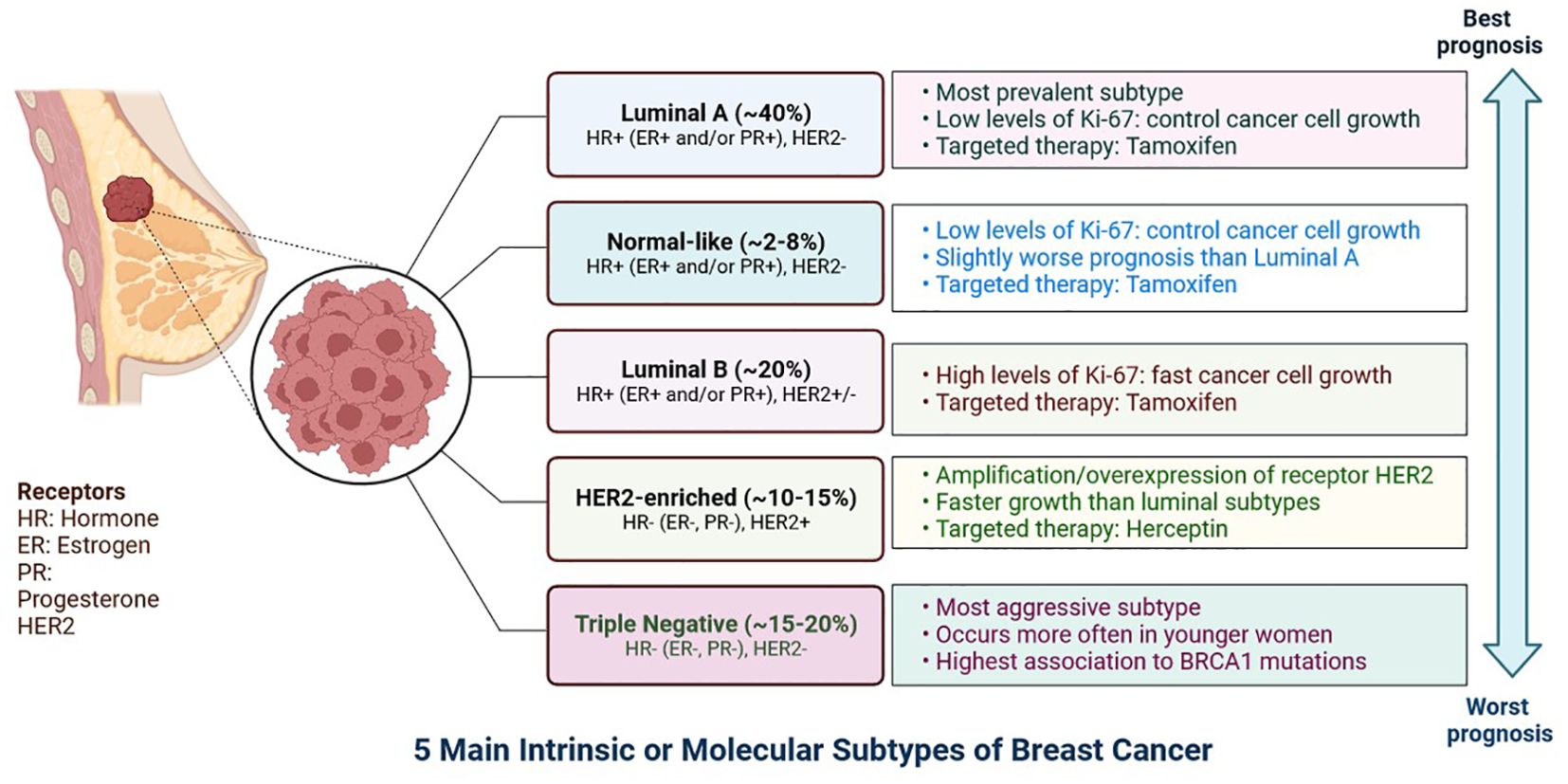
Figure 1. The expression of hormone receptors (progesterone receptor (PR) and oestrogen receptor (ER), the proliferation marker Ki-67, and the receptor tyrosine kinase HER2 can be used to classify breast cancer subtypes. Certain breast cancer subtypes can be treated with targeted medications like Tamoxifen, which targets the ER, and Herceptin, which targets the HER2 protein. The prognosis differs according on the kind of breast cancer.
The tumour immune microenvironment (TIME) is made up of a wide range of immune cells, as well as stromal cells, which contribute to its heterogeneity (3). The TIME composition changes throughout tumour growth and progression, as well as anti-tumor treatment (2, 3). In addition to the extracellular matrix (ECM), immune cells that are present in the tumour microenvironment (TME) and play a part in the development and spread of tumour necrosis include fibroblasts, antigen-presenting cells, and tumour-infiltrating lymphocytes (TILs) (4, 5).
Reprogramming the cellular physiology and avoiding immune damage are two recently identified signs of potential universality that have arisen in the last ten years (5). The body’s immune responses are enhanced and encouraged by tumour immunotherapy, which has the impact of eliminating tumours. It has emerged as an important anti-tumour therapy approach with significant clinical effectiveness and advantages over radiation, chemotherapy, and selective therapy. Current therapies used in clinical practice for the treatment of TNBC are shown in Table 1 (6).
Although a variety of tumour immunotherapeutic medicines have been developed, their broad use has been constrained by difficulties in their administration, including poor tumour permeability and low tumour cell absorption rates (7). Due to their targeting abilities, biocompatibility, and functions, nonmaterials have recently become a viable option for treating a variety of disorders (8). Additionally, nanoparticles have a number of qualities that make up for the shortcomings of conventional tumour immunotherapy, such as high drug loading capacity, effective tumour targeting, and ease of modification, which has led to the widespread use of nanomaterials in tumour immunotherapy (8). With the development of novel and potent therapies like checkpoint blockade therapy and CAR T-cell therapy that have significantly improved patient outcomes, immunotherapy has achieved clinical success in the past ten years. However, these treatments can be made more effective overall, reduce systemic toxicities, and reduce off-target effects (9). Different types of immune cells, such as dendritic cells for immunisation or T cells for enhancing adaptive immunity, can be targeted and modified by adjusting the nanomaterial’s features, such as size, shape, charge, and surface chemistry (10).
Currently, immunotherapy is a novel option for many solid tumours that have failed conventional therapies. There are various immunotherapeutic options available, including immunocheckpoint inhibitors (ICIs), inhibitors of cytotoxic T-lymphocyte-associated antigen 4 (CTLA-4) and programmed death receptor-1 (PD-1), as well as inhibitors of programmed death receptor-ligand 1 (PD-L1) (11). Due to its low tumour mutation burden and restricted T-cell infiltration, breast cancer has traditionally been regarded as a “cold” tumour. However, TNBC shows a larger amount of infiltrating lymphocytes, creating an advantageous immunological milieu for the possible application of ICIs (12).
Remodeling of tumour microenvironment in TNBC
Multiple immunological and non-immune cell types contribute to long-lasting inflammation and localised immune suppression in this immune-modified milieu, allowing malignant cells to divide and mutate without being recognised and stopped by the host’s defense system (13). The TME is essential for the growth, spread, and therapeutic response of tumours (14). Creating cancer medicines that work requires an understanding of the TME (15). There is substantial variability in TME among the various subtypes of TNBC (16). These immune cells have the ability to aid immune evasion and tumour progression (17). Through the release of angiogenic substances, including vascular endothelial growth factor (VEGF), the TME can encourage angiogenesis (Figure 2) (18). Increased angiogenesis can feed the tumor with nutrients and oxygen, promoting growth and metastasis. There are various mechanisms by which tumours avoid immune recognition (19). TME is made up of different cell types, including fibroblasts, blood vessels, extracellular matrix elements (ECM), cancer cells, immune cells, and signalling molecules. Cancer therapy can be enhanced by modulating tumour blood vessels (Figures 2, 3) (20). The ECM, an intricate web of proteins and glycoproteins, gives tissues structural support (21). The ECM’s composition and stiffness may change in TNBC, which may encourage tumour invasion and metastasis (Figure 3) (21). Fibroblast recruitment and activation contribute to the formation of a supportive stroma (22). Epithelial-to-mesenchymal transition (EMT) enables cancer cell invasion and migration (23). Angiogenesis ensures vascular endothelial growth factor (VEGF), nutrients, and oxygen supply to the growing tumour (24). There are various mechanisms by which tumours avoid immune recognition. In the absence of co-stimulation, tumour antigens are taken up and presented by APCs to tolerize T cells (25). Antibody against tumour cell-surface antigens induces endocytosis, degradation of the antigen, and immune selection of antigen-loss variants (25). Factors (e.g., TGFβ, IL-10, and IDO) secreted by tumour cells directly inhibit T cells, induce T regulatory cells, and generate the physical barrier to the immune system (Figure 4) (26).
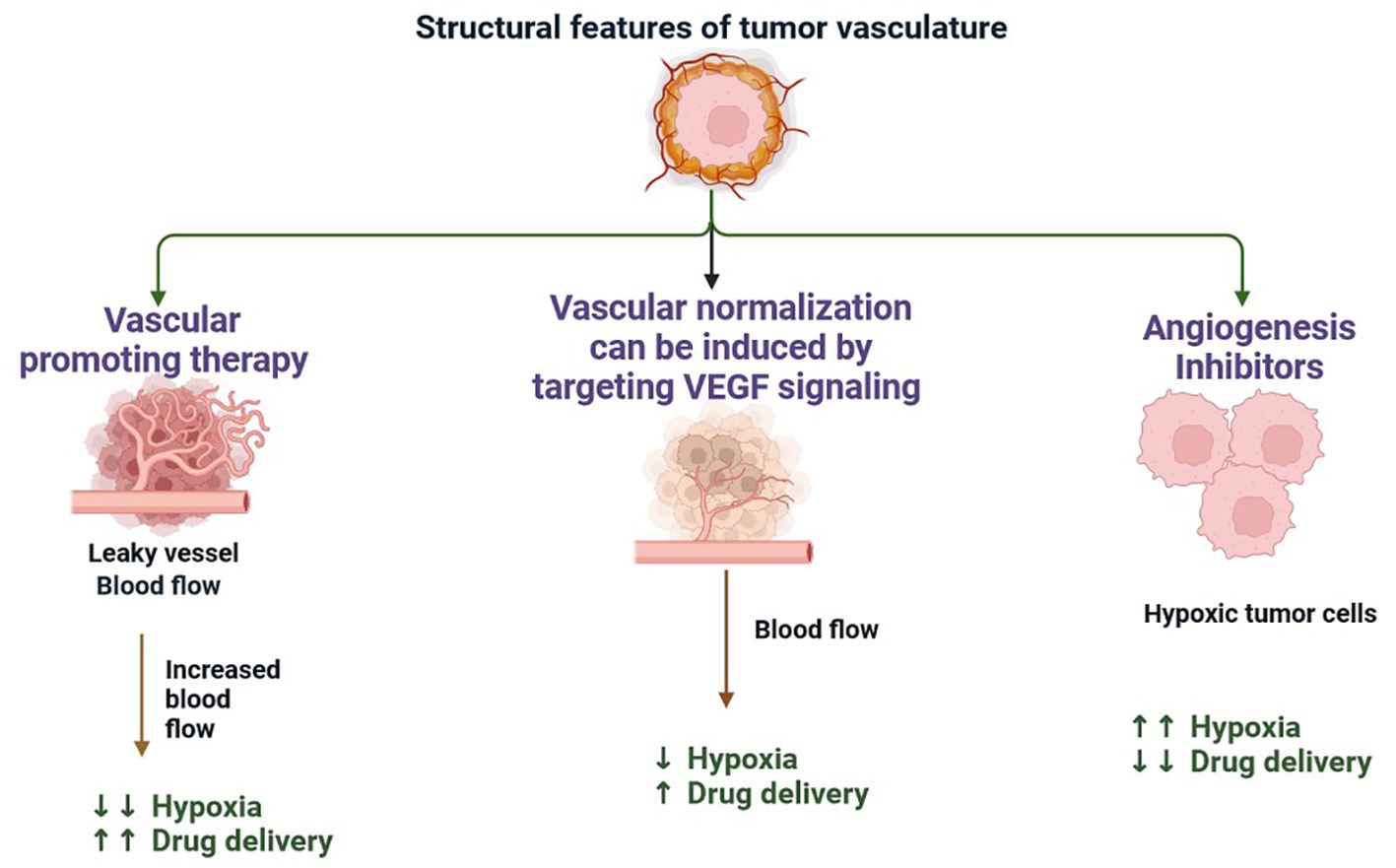
Figure 2. The formation of new blood vessels from the pre-existing vasculature is the main method of neovascularisation in tumours with their hypoxic and necrotic regions acting as inducers of angiogenesis. When a capillary receives an angiogenic stimulus, endothelial cell basal membrane and extracellular matrix are degraded, releasing endothelial cells from their basement membrane anchors (including integrins). This process is mediated by metalloproteases, leading to disruption of tight junctions, vasodilation, and pericyte detachment. Existing soluble growth factors coupled with the synthesis of a new matrix by stromal cells enable the migration and proliferation of endothelial cells.
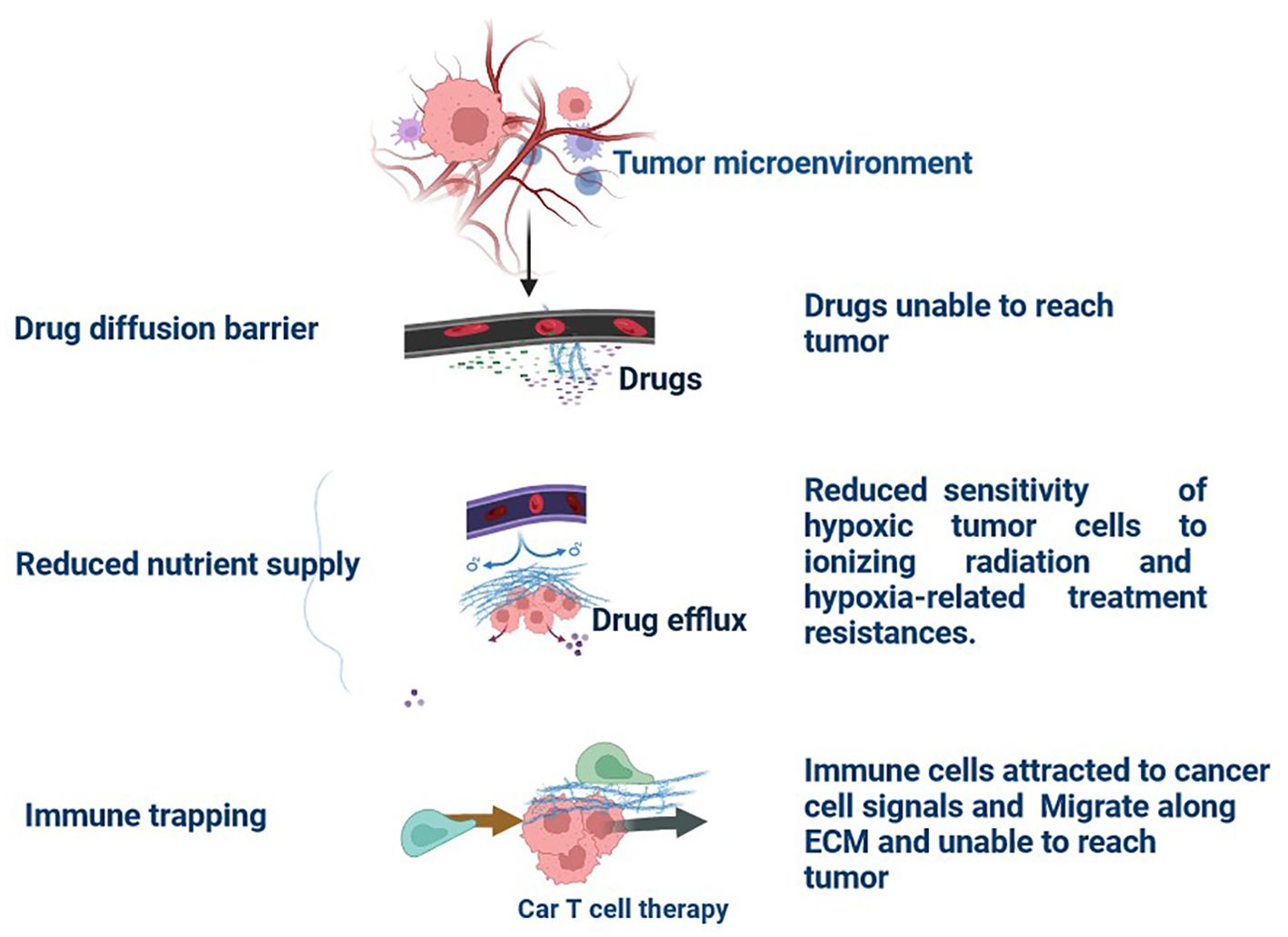
Figure 3. Tumour Extracellular Matrix Reduces Therapeutic Efficiency in TNBC: The tumour microenvironment (TME) comprises all components of a umor. Of these components, the extracellular matrix (ECM) is the least well studied. Solid tumours induce high expression of ECM molecules (collagens, proteoglycans, hyaluronic acid, and laminins), which become complex and disordered, resulting in altered haracteristics. Here, the ECM acts as a physical barrier, reducing the delivery of therapeutics, nutrients, and immune cells to solid tumours and leading to a poorer prognosis.
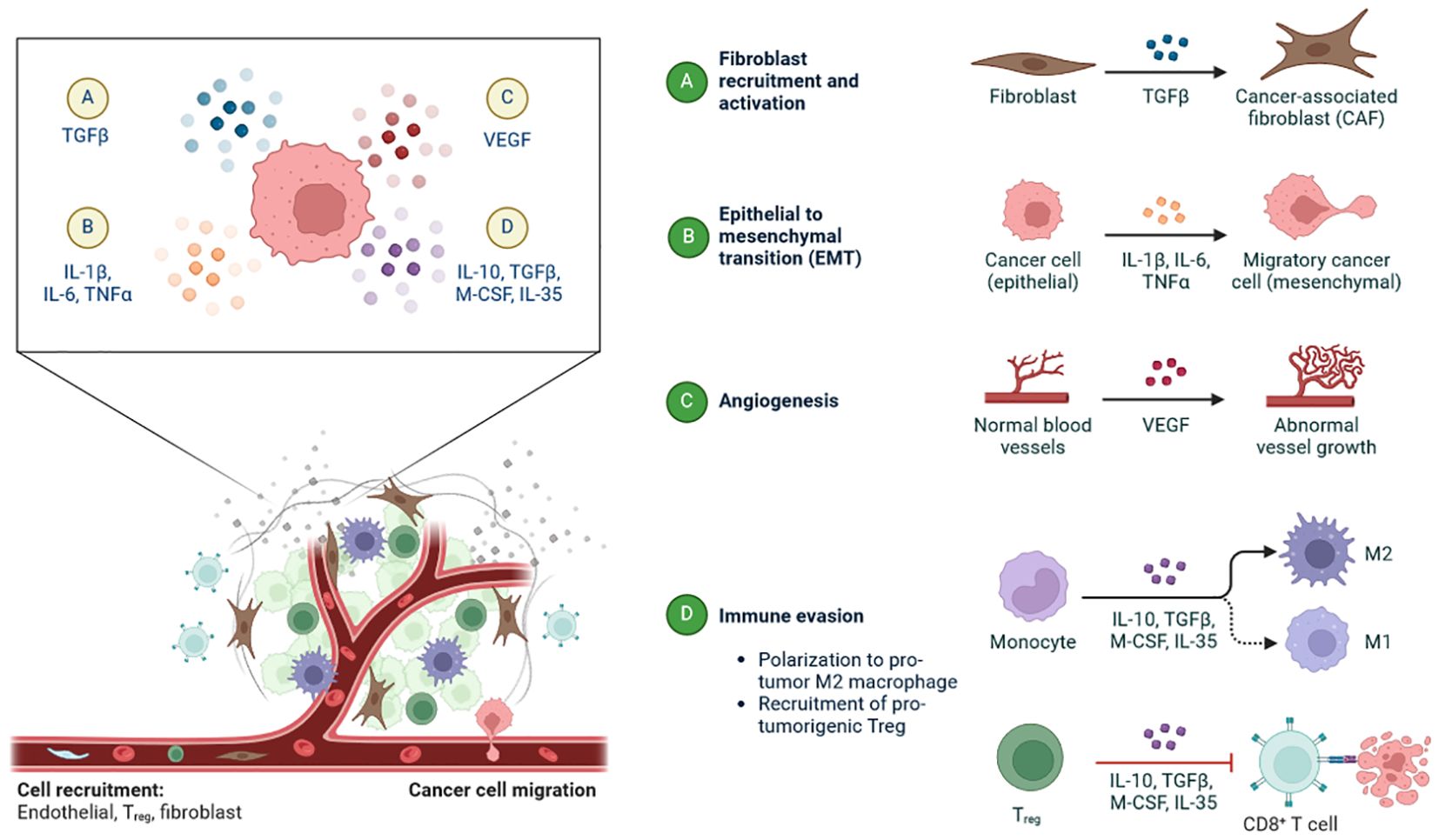
Figure 4. The tumour microenvironment plays a pivotal role in TNBC-associated changes: (A) Recruitment of fibroblast and activation by and regulates the TGFβ-TNBC-associated fibroblast (TAF). (B) Epithelial-to-mesenchymal transition (EMT) occurs in TNBC (epithelial) by IL-1β, IL-6, and TNFα-to Migratory cancer cells (mesenchymal). (C) Conversion of normal blood vessels into abnormal vessel growth by VEGF in angiogenesis. (D) Activation of M1, M2, and CD8+ T cells in immune invasion mechanism by IL-10, TGFβ, M-CSF, IL-35-M1 and M2 macrophases.
TAFs in remodeling of tumour microenvironment
TAFs (Tumour-Associated Fibroblasts) can activate in TNBC and release cytokines and growth factors that encourage tumour cell growth, invasion, and resistance to treatment (27). There are frequently areas of low oxygen (hypoxia) within the TME due to the TNBC tumours’ fast development (28). Angiogenesis, tumour aggressiveness, and therapy resistance can all be increased by hypoxia (29). There are six key approaches outlined, all with the aim of boosting the effector function of NK cells and helping to target cancer cells (30). Some, such as cytokines and immune checkpoint inhibitors, are clinically approved, while others are still in pre-clinical stages (31). Further work is needed to boost the efficacy of these treatments in solid tumours (Figure 4). In addition to affecting TAMs (Tumour-Associated Macrophages) and MDSCs (Myeloid-Derived Suppressor Cells). activating Natural killer (NK) cells is another approach being investigated for decreasing the immunosuppressive microenvironment (Figure 4) (7, 32, 33). The immunological activity of NK cells, a particular type of specialised innate lymphoid cells, is independent of MHC-mediated antigen presentation, Acts naturally as cytotoxic agents that inhibit the growth, migration, and colonization of metastatic cells, fighting both primary cancer cells and metastasis (34). Tumor Necrosis Factor Alpha (TNF-α), interferon-γ (IFNγ), and different interleukins are only a few of the cytokines that NK cells can release to control the immune response. They can also take part in other downstream immunological pathways (35). TAMs and MDSCs both contribute to the creation of an immunosuppressive microenvironment. T cells and NK cells can no longer act as immune cells when these cells are present (36). The nanoregulator can activate MDSCs to hasten the polarisation of TAMs towards the M1 phenotype and reactivate cytotoxic T cells to stop tumour cell proliferation. This further delays recurrence and reduces the chance that CTCs will establish themselves in the liver and lung thanks to the increased production of memory T cells that suppress cancer (37).
Role of tumour microenvironment in Immune checkpoint inhibitors
The use of immune checkpoint inhibitors as a therapeutic approach to combat this immunosuppressive feature of the TME has been studied. High amounts of TILs are present in some TNBCs, which may indicate a better prognosis (38). Immunotherapies that stimulate TILs have demonstrated promise for treating TNBC. Targeted treatments and immunotherapies have been developed as a result of our growing understanding of TME remodeling in TNBC (39, 40). Chemotherapy and immunotherapy are ineffective primarily because of abnormalities in the TME that act as barriers to drug transport. TNBC tissue has significant ECM deposition and severe fibrosis, which causes tumour vascular compression and lowers perfusion, which impairs drug delivery (41). Cancer-associated fibroblasts (CAFs) release cytokines that influence the ECM, epigenetic alterations, immunosuppression, and proliferation of tumour cells (41).
TME remodelling (attracting CD4+T cells, CD8+T cells, and NK cells) enhances the effectiveness of immunotherapy, an essential approach used to treat breast cancer, as TILs in the TME have a direct link to the prognosis of TNBC (42). The targets for redesigning the TME have significance for the course of therapy and prognosis of TNBC because they affect TAMs and dendritic cells (DCs), as well as tumour hypoxia, tumour blood vessel modulation, CAF and ECM regulation, and CAF and ECM regulation (Figure) (43, 44). Through the release of cytokines, chemokines, and ECM remodelling factors, CAFs play a crucial role in promoting cancer progression and treatment resistance (27, 44–48). In order to kill cancer cells and sabotage CD47-SIRPa (CD47-signal regulatory protein alpha) connections, the hybrid cell membrane nanovesicles, or hNVs, can interface with circulating tumour cells (CTCs) in vascular lumens and accumulate at the site of resection (48). It has been found that gene enrichment of IFN-γ receptor, JAK2, and interferon regulatory factor 1 occurs in individuals who do not react to anti-CTLA-4 therapy.
The blockade of the PD-1 pathway brings quiescent antitumor T cells to active life (Figure 5). The pro-tumour activity of neutrophils is blocked by using antibodies to target CD33 in immunosuppressive neutrophils (49–51). CXCR2 (CXC chemokine receptor 2) is inhibited by blocking the recruitment of pro-tumour neutrophils to the TME, which blocks activation signals such as G-CSF or TNFα and ROS production by inhibiting contact with T cells (49, 52). We can enhance the anti-tumour capacities of neutrophils by interfering with innate immune inhibitory checkpoints to restore antibody-mediated anti-tumour activities, by targeting proteins downstream of the inhibitory receptor, by using IgA-based therapeutic mAbs that bind to the activating Fc receptors, and by modifying the Fc region of IgG therapeutic antibodies that increases affinity to Fc receptors, as shown in Figure 6 (53).
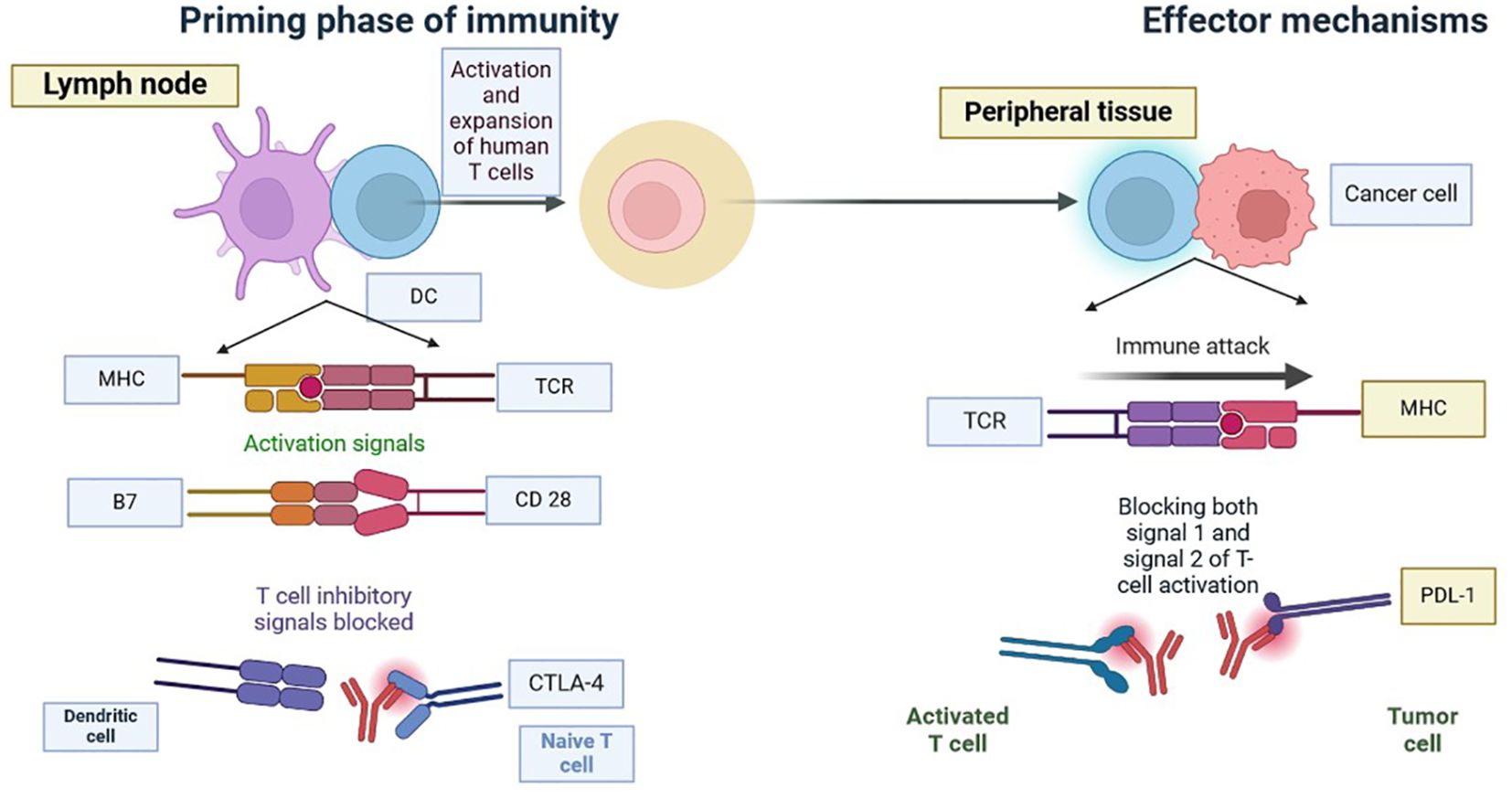
Figure 5. Blockade of CTLA-4 or PD-1 Signalling in Tumour Immunotherapy: Tumour immunotherapy has received a lot of attention since it has the potential to improve the immune system’s ability to recognise and fight cancer cells. Researchers hope to overcome tumours’ immune evasion strategies by suppressing CTLA-4 or PD-1 signalling pathways, thereby enhancing patient outcomes and extending therapy options. Tumour immunotherapy has received a lot of attention since it has the potential to improve the immune system’s ability to recognise and fight TNBC cells. To overcome tumours’ immune evasion strategies by suppressing CTLA-4 or PD-1 signalling pathways, thereby enhancing patient outcomes and extending therapy options.
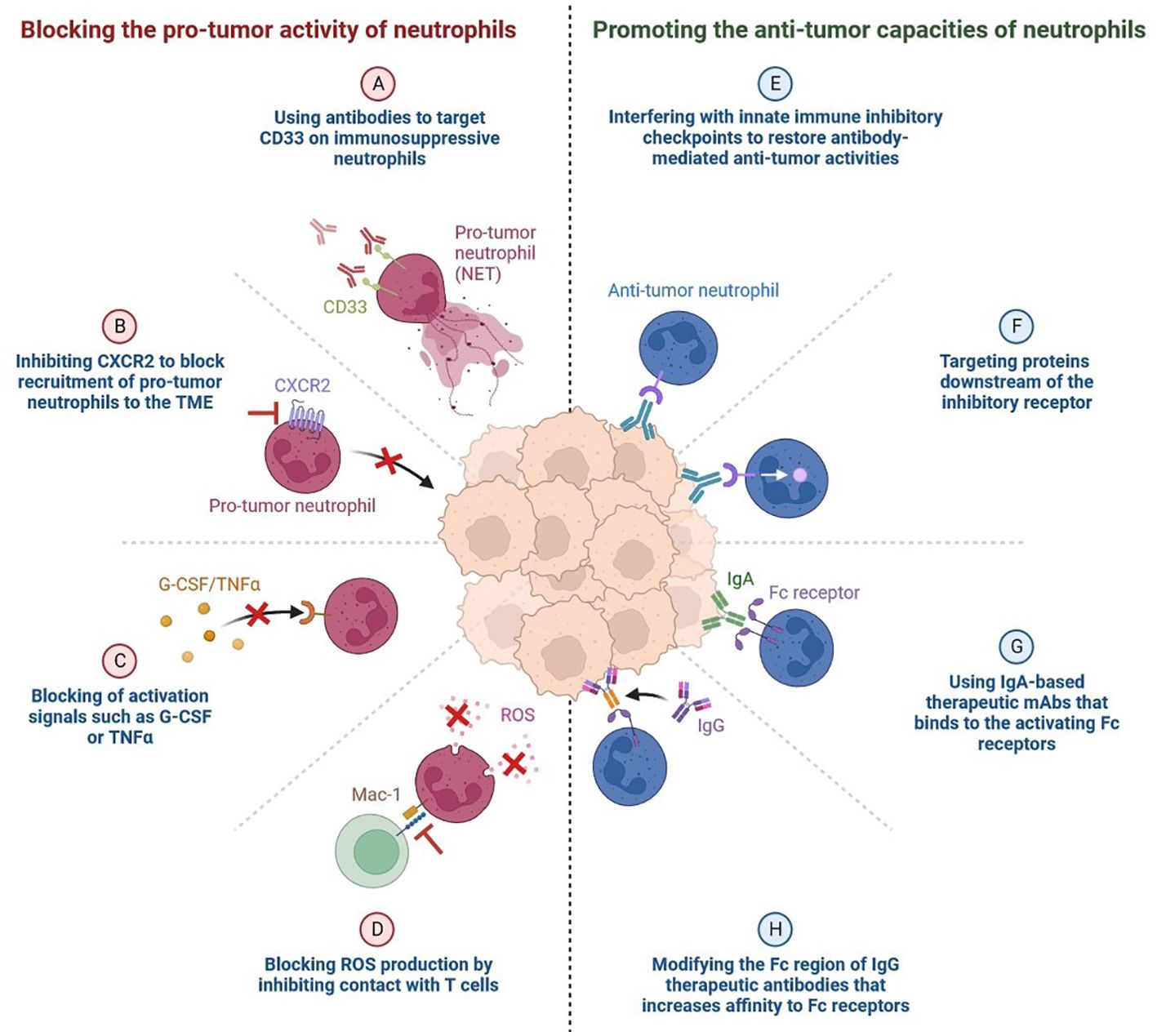
Figure 6. Targeting Neutrophils: The Pro- and Anti-tumor Activities of Neutrophils Blocking the pro-tumor activity of neutrophils. (A) Using antibodies to target CD33 on immunosuppressive neutrophils. (B) Inhibiting CXCR2 to block recruitment of pro-tumor neutrophils to the TME. (C) Blocking of activation signals such as G-CSF or TNFα. (D) Blocking ROS (Reactive oxygen species) production by inhibiting contact with T cells. (E) Promoting the anti-tumor capacities of neutrophils. (F) Interfering with innate immune inhibitory checkpoints to restore antibody-mediated anti-tumor activities.. (G) Targeting proteins downstream of the inhibitory receptor. (H) Using IgA-based therapeutic mAbs (Monoclonal antibodies) that binds to the activating Fc receptors.
Adenosine and tumour microenvironment
Adenosine, released by various cells within the tumour microenvironment, modulates local immune responses (Figure 7). This molecule impacts B lymphocytes, T lymphocytes, natural killer cells, macrophages, dendritic cells, and myeloid-derived suppressor cells, leading to immunosuppression that favours tumour growth (54). Understanding adenosine’s role in the tumour microenvironment provides a basis for identifying potential therapeutic targets for cancer immunotherapy (Figure 7) (53, 54).
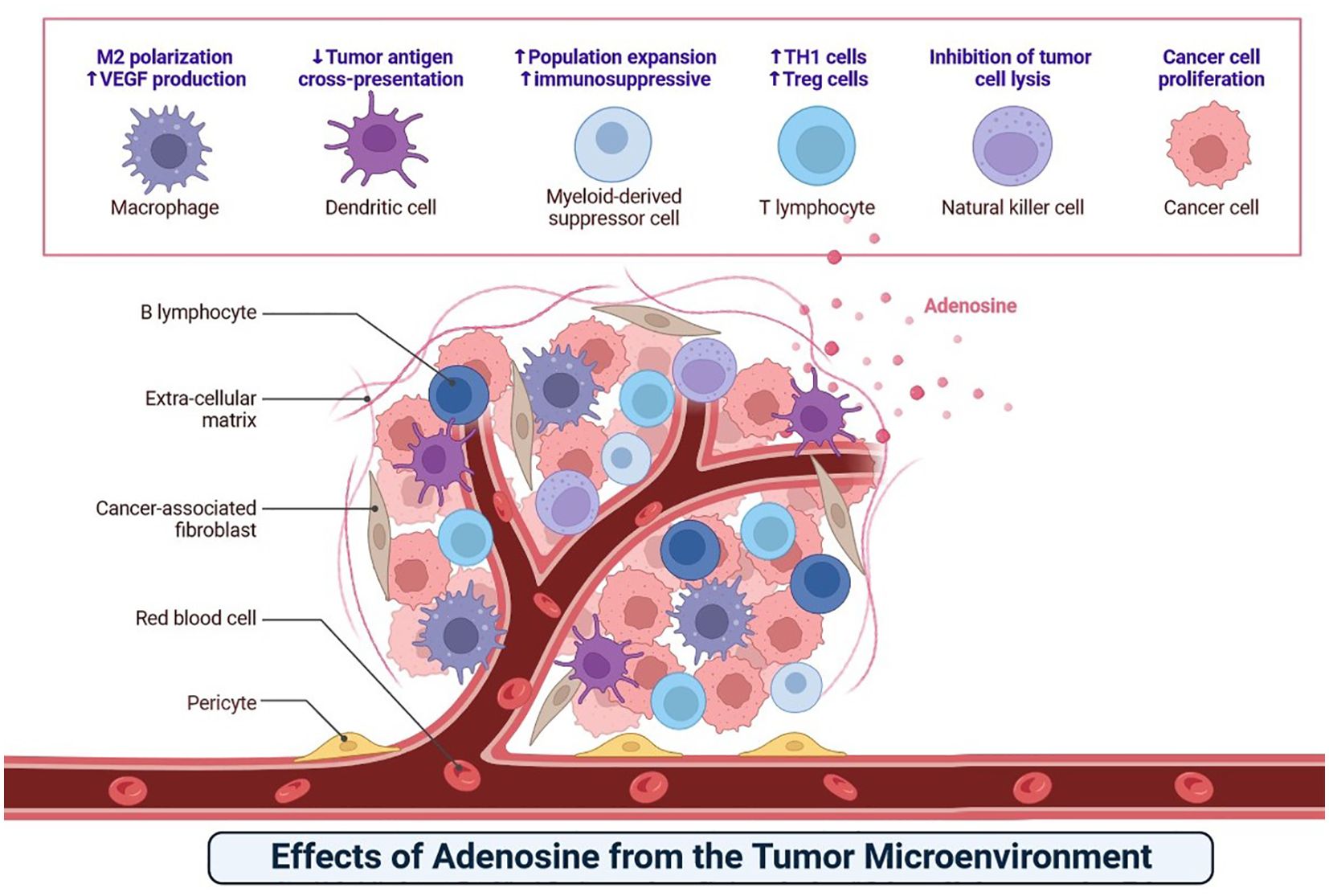
Figure 7. The adenosine causes immunosuppression that promotes cancer growth by interfering with B lymphocytes, T lymphocytes, natural killer cells, macrophages, dendritic cells, and myeloid-derived suppressor cells.
KRAS (Kirsten rat sarcoma virus) Mutations
KRAS (Kirsten rat sarcoma virus) is an oncogene that is mutated in about 25% of all cancers, but it only recently became a promising therapeutic target for anti-cancer therapy because of significant scientific breakthroughs (Figure 8) (1, 53, 55). KRAS encodes a protein called K-Ras, which, when mutated, contributes to the development of cancer through pathways that promote growth, proliferation, and differentiation. KRAS-mutant cancers have been difficult to treat via drugs due to the small size of K-Ras and its lack of binding sites (55). However, recent developments have allowed scientists to target this protein, showing how cancer treatment is evolving to be more personalised and specific. The K-Ras protein has a molecular ‘on/off’ switch that turns K-Ras off when bound to GDP and on when bound to GTP (55). Mutations in the KRAS gene lock K-Ras into the ‘on’ state, resulting in uncontrolled cell growth that leads to cancer. It is difficult for any drug to inhibit K-Ras by outcompeting GTP/GDP for the binding site (56). However, when K-Ras is bound to GDP, there is a groove next to the GTP/GDP binding site that a drug can bind to, locking K-Ras into its ‘off’ state (Figure 9) (57).
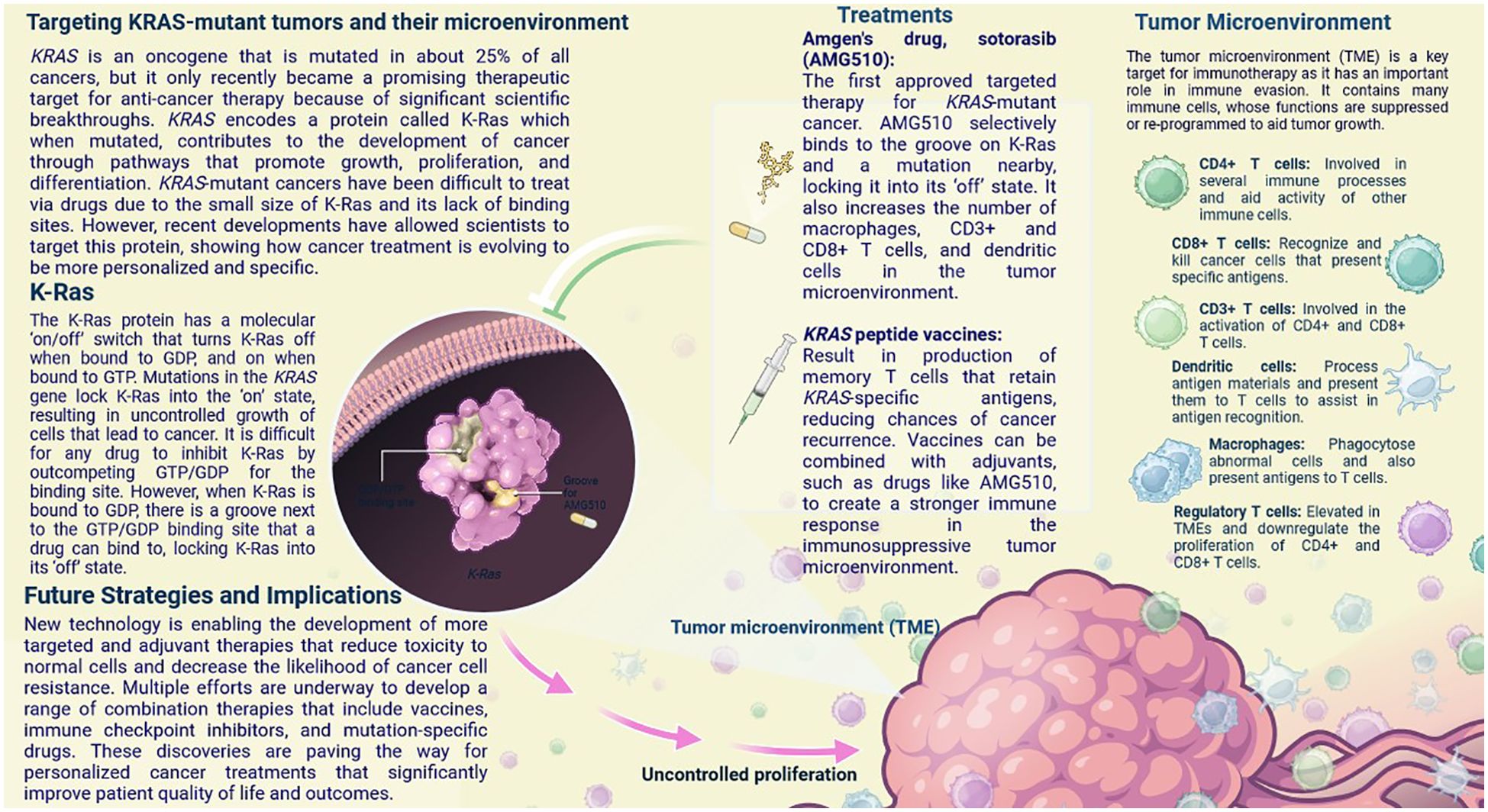
Figure 9. KRAS is an oncogene that is mutated in about 25% of all cancers, but it only recently became a promising therapeutic target for anti-cancer therapy. In this infographic, learn how recent breakthroughs have allowed scientists to develop treatments that specifically target mutant K-Ras, the protein product of KRAS, and alter the immunosuppressive tumor microenvironment to increase treatment efficacy.
New technology is enabling the development of more targeted and adjuvant therapies that reduce toxicity to normal cells and decrease the likelihood of cancer cell resistance. Multiple efforts are underway to develop a range of combination therapies that include vaccines, immune checkpoint inhibitors, and mutation-specific drugs (57). These discoveries are paving the way for personalized cancer treatments that significantly improve patient quality of life and outcomes. The first approved targeted therapy for KRAS-mutant cancer (57). AMG510 selectively binds to the groove on K-Ras and a mutation nearby, locking it into its ‘off’ state. It also increases the number of macrophages, CD3+ and CD8+ T cells, and dendritic cells in the tumour microenvironment (58). Result in the production of memory T cells that retain KRAS-specific antigens, reducing the chances of cancer recurrence.
Vaccines can be combined with adjuvants, such as drugs like AMG510 (Sotorasib), to create a stronger immune response in the immunosuppressive tumour microenvironment (59). These exciting new developments show how cancer treatments are becoming more specific and personalised compared to classic cancer therapies like chemotherapy (57). The remodelling of TME can be achieved by either decreasing the matrix barrier or enhancing the immunosuppressive microenvironment, concentrating on the gut microbiota and metabolites, and modifying immune cells and cytokines (58, 59). These methods contribute to the improvement of TNBC anti-tumour therapy as an entire concept by using appropriate model (60).
Preclinical models in mimic of TNBC
Preclinical models that accurately mimic TNBC aetiology are vital for assessing new therapy options and identifying long-term benefits for patients (60). To maximise model predictive accuracy while conserving time and resources, novel therapeutic strategies are planned, taking into account preclinical studies and clinical trial expenditures. Individualised therapies for TNBC patients are in high demand to improve their future healthcare (60, 61). There is a unique opportunity to use preliminary models and techniques in laboratory studies. To study TNBC disease at lab work to identify key genetic, transcriptomic, and proteomic players in tumour initiation and progression and identify potential anticancer agents for precision medicine. An ideal preclinical TNBC model should have close histological similarities to the tumour, maintain druggable genetic changes for targeted treatments, and be easy to handle and grow well in vitro and in vivo (Figure 10) (60, 61).
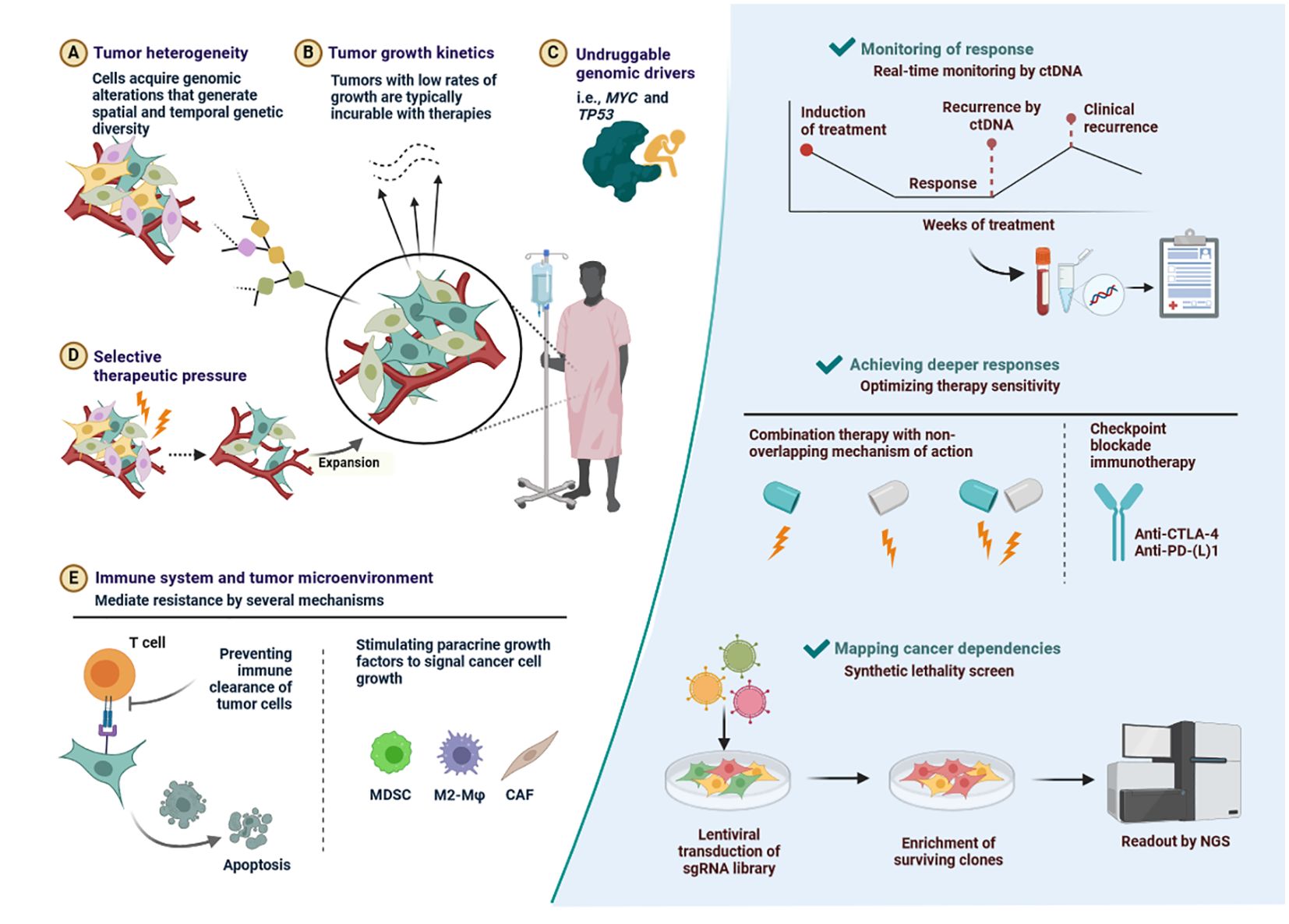
Figure 10. Key determinants of drug resistance as well as some potential, general solutions. (A) Tumor heterogeneity-Cells acquire genomic alterations that generate spatial and temporal genetic diversity. (B) Tumor growth kinetics-Tumors with low rates of growth are typically incurable with therapies. (C) Undruggable genomic drivers-i.e., MYC and TP53. (D) Selective therapeutic pressure-Expansion. (E) Immune system and tumor microenvironment-Stimulating paracrine growth factors to signal cancer cell growth.
Novel breast cancer drugs in use or in clinical trials for the treatment of TNBC
Numerous possible agents are undergoing various phases of investigation and advancement. These possible agents/inhibitors carry out their anti-tumor activity (62). An overview of the various inhibitor classes, including poly (ADP-ribose) polymerase (PARP), tyrosine kinase (TK), EGFR, PI3K, heat shock protein (Hsp90), histone deacetylase (HDAC), angiogenesis, insulin-like growth factor (IGF), and mammalian target of rapamycin (mTOR), as well as the mechanism of action depicted. In summary, ssDNA break repair is the aim of poly (ADP-ribose) polymerase inhibitors (PARPI). An 88% overall response rate was observed with the combination of paclitaxel and olaparib (40, 63, 64).
Receptor tyrosine kinase (RTK) inhibitors have been investigated for treatment options in TNBC. Unexpectedly, the bulk of EGFR-TKI studies against TNBC are not encouraging, despite the fact that EGFR is expressed in 89% of TNBC and looks to be a viable therapeutic target (64). Since only around 10% of TNBC has FGFR (Fibroblast Growth Factor Receptors) identified as a therapeutic target, pan-FGFR inhibitors such alofanib and PD173074 prevent SUM52PE from proliferating and cause apoptosis by blocking the MAPK and PI3K signalling pathways (65). Although bevacizumab and apatinib, which target VEGF2 (Vascular endothelial growth factor), have not shown encouraging effects in clinical studies, VEGF expression is linked to a poor prognosis in TNBC. On the other hand, sunitinib, an anti-VEGFR (Vascular endothelial growth factor) tyrosine kinase inhibitor, is starting to show promise as a treatment option in breast cancer studies (66).
The TNBC cell surface RTK (Receptor tyrosine kinases) MET (Mesenchymal–epithelial transition) triggers the activation of many downstream effectors, including as Src, AKT, ERK, and RAS. The tivntinib (MET inhibitor) phase II study is disappointing; however MET+EGFR inhibition synergistically decreased cell viability, demonstrating the combination’s higher effectiveness (67). Patients with TNBC have participated in clinical studies to assess a variety of PARPIs, including olaparib, veliparib, and talazoparib. The phase III trial of olaparib for BRCA-mTNBC (OLYMPIAD; NCT02032823) will The cytoplasmic mic kinases known as non-receptor tyrosine kinases (NRTKs) include MEK (Mitogen-activated extracellular signal-regulated kinase), Src, and the PI3-AKT-mTOR signalling cascade (68, 69). When combined with everolimus, dual mTORC1/2 inhibitors effectively limit the growth of many TNBC cell lines. Ongoing clinical studies are being conducted on TNBC using dual mTOR/P13K inhibitors and mTOR+PARP inhibitors (69). Early in the clinical development process, the PI3-AKT-mTOR pathway is one of the growing multi-targets of pharmaceuticals. MEK is a part of the MAPK signalling cascade, and MEK inhibitors (U0126) have been shown to dramatically diminish the invasiveness of MDA-MB-2311 in vitro, while selumetinib has been shown to suppress lung metastasis in xenograft models (70, 71). The addition of Src inhibitors, such as dasatinib to cetuximab + cis platin, improved the prevention of cell growth and invasion in TNBC (71). Src is a cytoplasmin oncoprotein. HDACs (Histone deacetylases) and Hsp 90 are two examples of epigenetic targets that are being researched for the therapy of TNBC (72). Tumour suppressor and DNA repair gene expression are known to be inhibited by HDACs. HDACi’s (Histone deacetylase inhibitors) in combination with cisplatin and DNA methyltransferase inhibitors are being studied in two clinical studies (72).
It is possible for Hsp90 to block many growths, signalling pathways, and survival cascades. Although olaparib and paclitaxel are still being tested in phase 1 clinical trials, a Hsp90 inhibitor called genetecibo (Ganetespib) has been shown to decrease tumour volume in xenografts produced from MDA-MB 231 (73). The anti-androgens bicalutamide and enzalutamide reduce proliferation, invasion, and migration of cancer cells by targeting the androgen receptor (AR) in different TNBC cell lines (73). This suggests that they may serve as a surrogate biomarker for response to other treatments. The VGS subtype Nav1. 5 neonatal splice variant is known as voltage-gated sodium channel (VGSC) (74). The foundation of clinical care of TNBC is VGSC-inhibiting medications, which include phenytoin, ranolazine, and riluzole. These medications all reduce metastatic cell behaviours in vitro and/or in vivo (75).
NP-based formulations called Liposomes-Doxorubicin nanodrug and Myocet, which were licenced for the treatment of breast cancer in 1998 (Taiwan) and 2000 (EMA), respectively, are now being used in clinical settings to treat metastatic breast cancer (76). A multitude of nanoparticles, including liposomal and polymeric nanoparticle platforms, are being developed for the treatment of cancer. The mitoxantrone-containing liposomal nanoparticles known as plm60-s (Mitoxantrone HCl liposome injection) are undergoing a phase II clinical study for the treatment of breast cancer. In phase II of a clinical study, the LiPlaCisa liposome nanoparticle containing cisplatin is showing promising outcomes for metastatic breast cancer (76).
Numerous immunotherapies, including as immune checkpoint inhibitors, activation of cytotoxic T lymphocytes (CTLs), adaptive cell transfer-based treatment (ACT), and modification of the tumour microenvironment (TME), have been tried. These cutting-edge immune-modulatory techniques can treat TNBC and have become individualized immunotherapy as shown in Table 2 (77).
TME and therapeutic resistance in TNBC
Various immune-promoting and immune-resistant components interact to form a complex network which regulates the TME (78). CTL interacts with APC and NK via cytokines and is essential to immunotherapy (51). Tumor-associated macrophages (TAM), myeloid-derived suppressor cells (MDSC), and regulatory T cells (Treg) are examples of immune-regulating cells (79). These cells impede the growth of T cells by directly expressing immunosuppressive cytokines and immunological checkpoint molecules. An immunosuppressive TME can also be produced by these cells’ malfunctions through indirect pathways including PD-1 pathway hijacking and interactions with Treg (80).
T cell infiltration is impeded by aberrant tumour neovascularization and cancer-associated fibroblasts (CAF). The TME becomes more acidic because of hypoxia-induced metabolic abnormalities, which creates a barrier to antitumor immune activity (81). Tumorigenesis generates aberrant antigens on the tumour cell surface that can activate APCs, mostly DCs. However, tumours frequently change the structure or downregulate MHC-I, which hinders antigen presentation and encourages immune escape (81).
TNBC patients that are resistant to ICB treatment frequently have downregulated levels of HLA-I, the human version of this molecule. This may be caused by mutations in the invariant β2-microglobulin (β2m) gene and other HLA-I encoding genes (51). The control of HLA-I expression is also dependent on transcription factors including NF-κB and NLRC5, as well as epigenetic processes, the disruption of which would have a major impact on antigen presentation (82). A prime example is interferon (IFN), which may upregulate HLA class I heavy chains (β2m, TAP1, TAP2, or Tapasin) and cause HLA-I to be downregulated when it is impaired (83). Furthermore, peptide antigen processing and presentation will be impacted by genetic flaws in any of the proteins that make up the MHC-I processing apparatus, such as downregulation of the TAP transporters. Important phases in the immune activation cascade involve APC activation and recruitment. Immune escape is supported by the reduction of chemokines which recruit APCs (84). In order to prevent APC phagocytosis, tumours can also suppress “eat me” signals like CRT and HMGB1, and upregulate “don’t eat me” signals like CD47, CD24, etc. When TNBC is present, the glycosylation of B7-H4 stabilises and stops this protein from degrading, which suppresses eIF2α phosphorylation (85). This results in a decrease in CRT surface expression and lets the tumour avoid immune destruction (85). It has been demonstrated that dendritic cells downregulate CD80 expression, preventing T cell activation via CD28, and exhibit high amounts of PD-L1. This is believed to be one of the mechanisms for the failure of ICB therapies.
In addition to gene mutations, epigenetic modifications such as DNA methylation, RNA interference, histone modification, etc., can serve as immune escape mechanisms (86). By inhibiting the production of immune genes, epigenetic changes may have a negative impact on tumour immunity. For example, loss of methylation may be the mechanism via which highly altered malignancies avoid immune responses and account for the seemingly contradictory observation that tumours with large chromosomal copy number changes have low antitumor immune activity. DNA methylation can also mute genes related to immunity (87).
Abnormal MAPK pathway activation is inextricably connected to tumour formation and progression as well as treatment resistance mechanisms in different types of cancer. Low TIL in the basal-like breast cancer subtype is associated with activating alterations in the Ras/MAPK pathway, such as shortening of NF1, amplifications of KRAS, BRAF, and RAF1, according to data from the TCGA database (88). In addition, MEK expression has some predictive value for TNBC patients’ overall survival (OS) and recurrence-free survival (RFS) following neoadjuvant treatment (89). The antigen-presenting molecules MHC-I and -II expression seem to be inversely correlated with MEK activity. The prevailing consensus explains this by stating that the Ras/MAPK pathway might impede the IFNγ-mediated inflammatory response, which in turn impacts the IFNγ-mediated production of MHC-I, MHC-II, and PD-L1, suppressing antigen presentation (90). The WNT pathway controls DC-mediated innate immunity. ATF3, a transcriptional repressor that stops CCL4 from being transcribed, can be activated by β-catenin. Lack of CCL4 reduces the effectiveness of ICB treatments by impeding both the infiltration and activation of CD8+ T cells as well as the stimulation of CD103+ DCs (91). Thus, cancer cells have the ability to immunoedit by down regulating or changing key molecules in the IFN-γ signalling cascade, which ultimately leads to immune evasion. Cancer cells have the ability to up regulate PD-L1 transcription and expression as a negative feedback mechanism (92). There is some overlap between IFN-γ and type I IFN’s roles in antitumor immunity. More research is necessary since it is unclear how they work, what kind of reaction is elicited, and which cell population is reacting (93).
PTEN deficiency can impact T cell recruitment and function, which can lead to ICB therapy resistance. The loss of PTEN causes immunosuppressive factors like VEGF and CCL2 to express more. It has been demonstrated that anti-VEGF antibodies increase T cell infiltration and activation within the tumour (94). Therefore, PTEN deletion probably reduces T cell invasion through unregulated VEGF expression. Drug resistance in cancer therapy is major cause of mortality. Figure 10 has described outlined key determinants of drug resistance as well as some potential, general solutions.
Exogenous nanomaterials can boost host immune escape and enhance the efficacy of immunotherapy. Nano-immunomodulators have been developed to specifically target the immunosuppressive microenvironment in order to loosen this rigid state (95). These agents can re-open the immune system in situ and slow the growth of tumours. The major nano-immunomodulators are predicted to target the microenvironment and inhibit metastasis based on the ability of reactivated immune cells to migrate (95). The biomimetic technique greatly reduces the toxicity of nano-assisted immunotherapy when compared to conventional nonmaterials, increasing its safety. Overall, by reducing the immunosuppressive effects on tumor-associated immune cells, remodelling the immunological state of the tumour microenvironment offers a workable strategy for immunotherapy (96).
Nanotechnology based Immunoengineering approaches
In the realm of nanoscience, a variety of physiochemical, biological, and functional characteristics are required to generate promising nanoparticles for use in biomedicine. The most crucial factor is size: conformational structure, targeted size (1 and 200 nm), high drug loading efficiency, a long half-life in circulation with minimal systemic toxicity, selective localization, and high adhesion in the tumour environment (97). There are various types of polymeric, inorganic, and lipid-based nanoparticles that, to their advantage, have been used against cancer cells (Figure 11) (98).
Gold nanoparticles (GNPs) have been shown to enhance radiation cytotoxicity in human cancer cells. Increased absorption of GNPs by cells may lead to greater radiation effects. We studied the radiosensitization effect of glucose-capped GNPs (16 nm and 49 nm) on MDA-MB-231 cells under megavoltage X-rays. Electron microscopy revealed that Glu-GNPs are primarily found in cells’ cytoplasm, including endosomes and lysosomes. ICP-AES shows that MDA-MB-231 cells absorb more 49-nm Glu-GNPs than 16-nm Glu-GNPs (P<0.05) (98). A lipid-based nanoparticle was chemically coupled to an inhibitor of the ECM-related enzyme, lysyl oxidase 1 (LOX), which inhibits the crosslinking of elastin and collagen fibres, resulting in a novel nanotherapeutic targeting the ECM. Conjugated vesicles loaded with chemotherapy-therapeutic epirubicin showed improved inhibition of TNBC cell proliferation in vitro and in vivo. Compared to free epirubicin and epirubicin-loaded nanoparticles, the in vivo results showed increased survival, reduced cytotoxicity, and improved biocompatibility (99). Using layer-by-layer films to adapt a liposomal doxorubicin delivery design with a synergistic siRNA can significantly reduce tumours in TNBC (100). Nevertheless, the use of nanomedicine in TNBC is restricted by the lack of ligands and a known highly expressed tumour target. Tumour microenvironment-based nanotherapy is described in Table 2, and strategies for therapeutic drugs via nanocarriers are shown in Table 3 (100).
Versatile liposomal nanocarrier
Liposomes are 400 nm spherical vesicles, lipid bilayer, versatile nanocarriers, offering the effective drug distribution since they may encapsulate medications in either a lipid membrane or an aqueous core (111). Three different processes are commonly used to manufacture liposomal nanoparticles: reverse phase evaporation, solvent injection (which precipitates lipid from a dissolved lipid in solution), and extrusion (which produces nanoparticles with a predetermined cross-sectional area) (112). The dual drug targeted method has shown better outcomes in TNBC xenograft mice, liposomes containing sorafenib and doxorubicin have been shown to have increased antitumor efficaciousness. Clinical trial has been completed on a novel micelle-encapsulated doxorubicin formulation (NK911) with high efficacy and minimum toxicity (112). Antagomir-10b, an anti-metastasis agent, and PTX (Paclitaxel), an anti-cancer agent, were co-delivered via a liposomal drug delivery method to reduce lung metastases of breast cancer and limit the growth of 4T1 tumours (113). The lipid-conjugated estrogenic (bioactive; 47.03%) NPs were seen to display a substantially greater, or 87%, reduction of breast cancer development in engrafted mice (MDA-MB-231 cells) (113). Thus far, EndoTAG-1 and MM-398, liposomes laden with paclitaxel and irinotecan, have progressed to clinical trials in patients with TNBC (112).
Micelles
Micelles are hydrophobic particles with a hydrophobic core formed by Vander Waals bonding, and they range in size from 5 to 100 nm. They are stabilized by a hydrophilic shell (114). Micelles have the ability to transport hydrophobic and water soluble drugs for the treatment of cancer due to their amphiphilic nature. Using styrene-co-malefic acid (SMA) (115). Researcher has synthesized an amicellar system to deliver RL71, a hydrophobic derivative of curcumin, for TNBC treatment. Due to a prolonged release profile and higher cellular absorption mediated by endocytosis, the system exhibited greater toxicity to cancer cells (115). Another synthesized cetuximab-conjugated vitamin E D-alphatocopheryl polyethylene glycol succinate to deliver docetaxel medication selectively (115).
Dendrimers
Dendrimer was tested as a targeted diagnostic module in a TNBC cancer mouse model.A novel dendrimer, G4PAMAM, was generated and subcutaneously injected into mice as a dual model for imaging and drug delivery (66). It was combined with DL680 (NIR dye) and GdDOTA (MRI contrast). A near-infrared (NIR) fluorescence imaging scan and an MRI scan demonstrated the targeted diagnostic usage of this small sized (GdDOTA) 42-G4PAMAM-DL680 (GdDOTA) may be employed for certain diagnostic applications (116).
Polymeric nanoparticles
Misnomer nanoparticles are polymeric nanoparticles (50 nm and 10 μm in size), These nanoparticles have the added benefit of encapsulating medications and proteins without requiring any structural modification. They can be made from natural or artificial polymers (117).
The combination of succinobucol and P188 (poloxamer) is showing promise as the best oral therapy for breast cancer. Succinobucol NPs have a better bioavailability (13 fold), which amplifies their capacity to prevent tumour cell migration and vascular cell adhesion molecule-1 (VCAM-1) invasion (118). Moreover, miRNA and siRNA have been shown to be delivered by polymeric NPs in conjunction with therapeutic drugs to reduce the tumour (119). Antisense-miR-21 and antisense-miR-10b were co-delivered by PLGA-b PEG polymer NPs at a concentration of 0.15 mg/kg, whereas co-loaded DOX and siRNA (multidrug resistance protein) induced an 8-fold decrease in tumour volume and growth overall, respectively (119). In TNBC tumour models, a potential ligand called Arg-Gly-Asp (RGD) either helps with targeted drug delivery or suppresses malignant invasion in a different way. For example, alphavbeta 3 (αvβ-3) integrin receptor adhesions and invasion have been demonstrated to be inhibited by cyclic RGD-functionalized solid lipid NP (RGD-SLN), which is overexpressed in invasive TNBC tumours (120). RGD-DMPLN’s targeted treatment efficacy was evaluated in a metastatic TNBC mouse model that was optimized with the MDA-MB-231-lucD3H2LN cell line. The total effectiveness of treating cancer is increased by this kind of targeted distribution of synergistic drugs; further research is needed to enable broader applicability in the case of breast cancer (120).
DNA nanostructures in TNBC therapy
DNA nanostructures are designed with required forms, sizes, and configurations, such as tetrahedral, bipyramids, cages, and cubes, by taking use of the most fundamental property of DNA, which is Watson-Crick complementary nucleic acid base pairing (121). These DNA nanostructures may include tiny functional molecules and/or ligands for bioimaging or site-specific attachment (121). It was tagged at the base with red-emissive glutathione protected gold nanoclusters (GSH-Au NCs) and included actinomycin (AMD) in the minor groove of the DNA (122). Cetuximab’s has found effective in EGFR over expressing cancer cells, which is conjugated TH (THC3) with intercalated doxorubicin (DOX) medication, or THDC3, demonstrated preferential death of MDA-MB-468 cancer cells (122). The low IC50 value of 0.91 μM for THDC3 relative to 3.06 μM for free DOX indicates the strong and specific killing effectiveness of THDC3. Due to improved absorption of Cy3 THC3 into MDA-MB-68 cells, a different modified formulation comprising one Cy3 probe and three cetuximab, or Cy3-THC3, exhibits high signalling intensity (122). These two (THDC3 and Cy3-THC3) modest TH alterations demonstrate improved cancer cell targeting and killing, making them a great option for cancer nanomedicine, particularly for TNBC (122).
Multifunctional metal nanoparticles
Metallic nanoparticles (NPs) like titanium dioxide (TiO2), gold (Au), silver (Ag), platinum (Pt), zinc (ZnO) are employed in cancer treatment. These nanoparticles’ magnetic, optical, thermal, and electrical characteristics may present several opportunities in therapeutic and diagnostic assays (123). The usefulness for intended therapeutic results is increased when metal nanoparticles’ surfaces are modified by conjugating distinct groups. NPs from the transition class of metals heat up the cells by converting electromagnetic energy to heat, causing hyperthermia (a non-invasive technique) that kills the tumour cells. Some metal nanoparticles possess distinct physiochemical characteristics that confer intrinsic strong anti-cancer action (124).
The most promising metal nanoparticle (NP) now available for delivering the well-known anti-cancer medication paclitaxel is gold nanoparticles (AuNPs) (125). Serum-coated AuNR has the innate capacity to suppress the expression of genes linked to energy production. Both in vitro and in vivo cancer cell migration and invasion are hindered as a result of decreased energy (125).
The cisplatin-coated AuNR in combination with an NIR laser has shown to inhibit/suppress the TNBC tumour and its metastasis. The antiproliferative, proapoptotic, and anti-angiogenic properties of silver nanoparticles (Ag NPs) on cancer cells are well-known (125). As a radiosensitive drug, AuNPs interacts with the acidic environment of cancer cells to enhance oxidative stress through the generation of reactive oxygen species (ROS), which ultimately cause damage and death (124, 125). The researchers have revealed encouraging outcomes from treating gliomas with AgNPs and then radiation. Additionally, it was shown that these NPs prevented cancer cells from expressing endothelial growth factor (VEGF), which reduced metastasis (126).
Zinc oxide nanoparticles (ZnO NPs) are used in cancer therapy and have even been shown to decrease toxicity and boost effectiveness in breast cancer cells when used in conjunction with the medications paclitaxel and cisplatin (127). In addition to copper (CuO NP), iron oxide (Fe2O3), silica, cerium oxide, and titanium oxide, other metal nanoparticles (NPs) are also being investigated and employed in the detection and treatment of breast cancer (127).
Carbon nanotubesin TNBC therapy
Carbon nanotubes (CNTs) are folded into single-walled or multi-walled cylindrical structures. A slight modification in chemical change may perform a variety of tasks and has great potential for cancer treatment (128). Single-walled nanotubes (with a diameter of 1-2 nm) that may enter cells have both localised and sustained effects. By decreasing the number of macrophages and blood vessels within the tumour, oxidised multi-walled carbon nanotubes (o-MWNTs) offer a unique strategy for cancer treatment (129).
Aptamers nucleicacid based ligands
Short oligonucleotide segments of single-stranded DNA or RNA are known as aptamers. Because of the aptamer’s distinct three-dimensional confirmation, it binds the target molecule with great affinity and strength (130). The degradation by nucleases is the sole restriction, yet its great stability attracted interest for the creation of molecular probes. The cell-SELEX approach has been used to precisely target a surface membrane protein on TNBC tumours, utilising the recently discovered LXL-1 aptamer (130). Using PDGF-aptamer coated with gold nanoparticles, the differential overexpression of the platelet-derived growth factor (PDGF) receptor in the TNBC cell line has been found. Mammaglobin A2 and B1 are overexpressed in breast cancer cells MCF7 and MDA-MB-415, according to observations (131). Terahertz (THz) chemical microscopy (TCM), which employs THz radiation, was used to find metastatic breast cancer using MAMA2 and MAMB1 aptamers. The nucleo-lin receptor in TNBC theranostic in certain breast cancer cells is exclusively bound by a unique 26-mer G-rich DNA aptamer; nevertheless, further research and combination with medication administration are needed for such an aptamer-based precision targeted diagnostic (132).
Antibodies conjugated fluorescent nanoparticles
Antibodies are Y-shaped proteins with two epitopes that bind to their receptors with affinity and specificity (133). These are considered to be the best class of ligands for targeting.After conceptualising the differentially up-regulated expression of urokinase plasminogen activator receptor (uPAR) and tissue factor (TF) receptor in TNBC, researchers have proposed and confirmed the use of an anti-TF antibody labelled with copper-64 (anti-TF-antibody-64Cu) in an in vitro model of TNBC through PET imaging (134). Fluorescence microscopy and ultrasonography are also used to identify anti-EGFR and anti-VEGFR antibodies conjugated with fluorescent NP and ultrasonic contrast agents. Preclinical investigation on TNBC xenograft mice has demonstrated a satisfactory response (treatment) to Iodine-124 (124I)-tagged B-B4 antibody (targeting syndecan-1; CD138 antigen) and a good visualisation of the TNBC tumour (134–155).
Peptides
Peptides are ligands with a low molecular weight that have a high degree of selectivity for intracellular activities. Chlorotoxin, RGD, P-selection, and tumour metastasis targeting (TMT) are a few peptides that are effective in targeting metastatic breast cancer (135, 156–173). By using NIR fluorescence imaging in breast cancer models of TNBC mice. CK3 peptide (Cys-Leu-Lys-Ala-asp-Lys-Ala Lys-Cys) binding to neuropilin 1 (NRP-1 transmembrane protein) (136, 174–201). Activated cell-penetrating peptides (ACPPs), which target the matrix metalloproteinase (MMP)-2 enzymes, increase tumour uptake and contrast imaging in in vivo tumour necrosis models when covalently attached to cyclic-RGD peptide (137). Better and more efficient targeting of αvβ3 integrin receptors was achieved by connecting modified Fe2O3 NPs to cyclic RGD peptides (138). PGD-peptide and P-selectin, when combined with liposomal NP, have the ability to bind to different tumour areas by overexpressing their respective receptors on breast cancer cells (139).
Other small molecules
The possible targeted agent for cancer imaging has potential for direct imaging agents such as 18F-FDG, which is used as a glucose analogue. Studies have revealed that the folate molecule drives the super-paramagnetic iron oxide contrast agent (P1133) to folate receptors and internalises it in actively developing TNBC (140, 202–230). Even folic acid-conjugated AuNR demonstrated increased absorption in 4T1 metastatic breast cancer cells and targeted the folate receptor (141). Both quantum dots (QDs) and carbon dots (CDots) have shown potential in imaging and early detection of TNBC (141). TNBC development and metastasis are mediated by the cellular target chemokine receptor type 4 (CXCR4) (141). By increasing the cellular absorption into MDA-MB-231 cells, plerixafor or AMD3100 (CXCR4 ligand) conjugated poly(lactide-co-glycolide) NPs improved siRNA-mediated gene silencing. Similarly, in a lung metastatic model of breast cancer, AMD3100-loaded human serum albumin-encapsulated NPs target CXCR4 (141, 231–242).
Due to the strong affinity of hyaluronic acid (HA) for the CD44 receptor, MDA-MB-231Br (a kind of metastatic breast cancer cell) may take up an ultra-small (~5 kDa) HA-PTX nanoconjugate through CD44 receptor-mediated endocytosis (142). Tumour inhibition was much stronger when the urokinase plasminogen activator receptor (uPAR) targeting peptide was attached to poly (lactic-co-glycolic acid)-b-PEG polymers containing two antisense siRNAs. Novel contrast agents in MRI have been employed, using functionalized fullerenes (143, 243–250). In biomedicine, other small carbon molecules with unique physical and chemical characteristics, such as nanocarbons and nanodiamonds, are also developing and require more investigation.
Virus-like particles: innovative nano-vehicles for theranostics in the future
Virus-like particles (VLPs) are multimeric nanostructures that self-assemble and range in size from 0.1 to 100 nm. They are produced when viral structural genes are expressed in heterologous systems (144). The VLPs are allowed to travel through the bloodstream, and their functional viral proteins are on the cell surface, which promote cell entrance and penetration (144). By targeting and infiltrating certain tumour cells through receptor-mediated endocytosis, VLP’s can encapsulate tiny molecules or drugs that may be used to treat cancer. Eventually, the encapsulated medicine will be released inside the cancer cell. The most amazing capacity is to avoid lysosomal degradation by escaping endosomes; this promotes medication availability and protects drugs in blood plasma (144).
The major drawback to using VLP as a drug delivery strategy is that, because of its viral proteinaceous particle and easy uptake by dendritic cells, it triggers an innate immune response (145). Nevertheless, after traditional chemotherapy failed, it provided a glimmer of hope for the treatment of TNBC (145). Moreover, improvements in medication absorption and biocompatibility may offset the aforementioned drawbacks. Human papillomavirus (HPV), bacteriophage, polyomavirus, Ebola, influenza, hepatitis E virus (HEV), and tobacco mosaic virus (TMV) are the sources of several VLPs (146). The bulk of VLPs exhibit natural tropism to sialic acids or heparin sulphates, which restricts their application as targeted nanocarriers. However, certain VLPs exhibit natural tropism to specific organs or tissues, such as HEV VLPs for the liver and hepatocytes (146).
The self-assembled Bacteriphage MS2 VLP, which is modified with SP94 peptide and encapsulated with doxorubicin, cisplatin, and 5-fluoro-uracil to selectively transport and kill human hepatocellular carcinoma (HCC) in the Hep3B cell line, is a classic example of VLPs as targeted therapeutic carriers (147). Dodecahedron, chemically coupled with the anticancer antibiotic Bleomycin (BLM) and produced from adenovirus (Ad3), or Db-BLM, causes ds-DNA breaks at lower concentrations, which kills transformed cells. Thus, the adaptability, cell-specific targeting, effective cell entry, absence of endosomal sequestering, multivalency, biocompatibility, massive encapsulation, and secure delivery mechanism of vector lambda proteins are responsible for their widespread use. Even with all of their benefits, VLPs as a medication delivery mechanism are still in their infancy and require validation using animal models (148).
Conclusion and future prospect
Addressing the advantages of nanotechnology in TNBC treatment, researchers highlight the potential for targeted drug delivery systems that can enhance the efficacy of therapies while minimizing side effects. Adjuvant treatment, which includes hormone therapy (letrozole, tamoxifen) and chemotherapy (paclitaxel, eribulin), has a number of long-term adverse effects that lower the patient’s quality of life. Chemotherapy, which includes neoadjunant chemotherapy, anthracyclin, and taxane-based chemotherapy, is still the only choice for treating TNBC. A sophisticated, innovative, and successful therapy is required due to the 50% recurrence rate and 37% death rate, despite thorough and vigorous management.
The conjugation of multifunctional smart nanoparticles with therapeutic fluorophore allows them to penetrate various biological barriers, target, and penetrate cancer cells through a passive mechanism called the enhanced permeability and retention (EPR) effect. Ultimately, the controlled release of medication within the cancer cells is achieved. However, challenges such as the need for extensive safety evaluations and the complexity of manufacturing nanomaterials remain significant hurdles to overcome. There are a lot of limitations associated with conventional chemotherapeutic drugs, including non-specific targets, poor clinical results, chronic toxicity, poor solubility, low absorption, and potential drug resistance. Immunotherapy has produced new and useful treatment alternatives; however, using these immunostimulatory medications unmodified has led to drawbacks such as off-target effects and quick elimination. Immunotherapy based on nanoparticles has a lot of potential to overcome these challenges in order to increase the therapeutic effect and improve patient outcomes for TNBC patients.
Author contributions
DDS: Conceptualization, Data curation, Formal analysis, Writing – review & editing, Investigation, Methodology, Project administration, Resources, Software, Supervision, Validation, Visualization, Writing – original draft. SH: Data curation, Formal analysis, Writing – review & editing. YK: Data curation, Formal analysis, Writing – review & editing, Funding acquisition. IH: Data curation, Formal analysis, Writing – review & editing, Funding acquisition. DKY: Data curation, Formal analysis, Funding acquisition, Writing – review & editing, Conceptualization, Investigation, Methodology, Project administration, Resources, Software, Supervision, Validation, Visualization, Writing – original draft.
Funding
The author(s) declare financial support was received for the research, authorship, and/or publication of this article. The authors are thankful to the Ministry of Education 2022R1F1A1074105 and Kyung Hee University in 2022(KHU-20220787), Republic of Korea.
Acknowledgments
The authors extend their appreciation to the Deputy ship for Research & Innovation, Ministry of Education in Saudi Arabia for funding this research work through the project number ISP23-101. DDS is thankful to DST-PURSE and DST-FIST–AIMT at Amity University Rajasthan, Jaipur, India. The authors are thankful to Biorender.com for their graphical support.
Conflict of interest
The authors declare that the research was conducted in the absence of any commercial or financial relationships that could be construed as a potential conflict of interest.
Publisher’s note
All claims expressed in this article are solely those of the authors and do not necessarily represent those of their affiliated organizations, or those of the publisher, the editors and the reviewers. Any product that may be evaluated in this article, or claim that may be made by its manufacturer, is not guaranteed or endorsed by the publisher.
References
1. Feng Y, Spezia M, Huang S, Yuan C, Zeng Z, Zhang L, et al. Breast cancer development and progression: Risk factors, cancer stem cells, signaling pathways, genomics, and molecular pathogenesis. Genes Dis. (2018) 5:77–106. doi: 10.1016/j.gendis.2018.05.001
2. Wang D-Y, Jiang Z, Ben-David Y, Woodgett JR, Zacksenhaus E. Molecular stratification within triple-negative breast cancer subtypes. Sci Rep. (2019) 9:19107. doi: 10.1038/s41598-019-55710-w
3. Liu Y, Li C, Lu Y, Liu C, Yang W. Tumor microenvironment-mediated immune tolerance in development and treatment of gastric cancer. Front Immunol. (2022) 13:1016817. doi: 10.3389/fimmu.2022.1016817
4. Zhao H, Wu L, Yan G, Chen Y, Zhou M, Wu Y, et al. Inflammation and tumor progression: signaling pathways and targeted intervention. Sig Transduct Target Ther. (2021) 6:263. doi: 10.1038/s41392-021-00658-5
5. Sordo-Bahamonde C, Lorenzo-Herrero S, Gonzalez-Rodriguez AP, Martínez-Pérez A, Rodrigo JP, García-Pedrero JM, et al. Chemo-immunotherapy: A new trend in cancer treatment. Cancers. (2023) 15:2912. doi: 10.3390/cancers15112912
6. Zimmermannova O, Caiado I, Ferreira AG, Pereira C-F. Cell fate reprogramming in the era of cancer immunotherapy. Front Immunol. (2021) 12:714822. doi: 10.3389/fimmu.2021.714822
7. Kumar S, Chatterjee M, Ghosh P, Ganguly KK, Basu M, Ghosh MK. Targeting PD-1/PD-L1 in cancer immunotherapy: An effective strategy for treatment of triple-negative breast cancer (TNBC) patients. Genes Dis. (2023) 10:1318–50. doi: 10.1016/j.gendis.2022.07.024
8. Gavas S, Quazi S, Karpiński TM. Nanoparticles for cancer therapy: current progress and challenges. Nanoscale Res Lett. (2021) 16:173. doi: 10.1186/s11671-021-03628-6
9. Zhong L, Li Y, Muluh T, Wang Y. Combination of CAR−T cell therapy and radiotherapy: Opportunities and challenges in solid tumors (Review). Oncol Lett. (2023) 26:281. doi: 10.3892/ol.2023.13867
10. Azharuddin M, Zhu GH, Sengupta A, Hinkula J, Slater NKH, Patra HK. Nano toolbox in immune modulation and nanovaccines. Trends Biotechnol. (2022) 40:1195–212. doi: 10.1016/j.tibtech.2022.03.011
11. Shiravand Y, Khodadadi F, Kashani SMA, Hosseini-Fard SR, Hosseini S, Sadeghirad H, et al. Immune checkpoint inhibitors in cancer therapy. Curr Oncol. (2022) 29:3044–60. doi: 10.3390/curroncol29050247
12. Retecki K, Seweryn M, Graczyk-Jarzynka A, Bajor M. The immune landscape of breast cancer: strategies for overcoming immunotherapy resistance. Cancers. (2021) 13:6012. doi: 10.3390/cancers13236012
13. Burgos-Panadero R, Lucantoni F, Gamero-Sandemetrio E, Cruz-Merino LDL, Álvaro T, Noguera R. The tumour microenvironment as an integrated framework to understand cancer biology. Cancer Lett. (2019) 461:112–22. doi: 10.1016/j.canlet.2019.07.010
14. Meng L, Zheng Y, Liu H, Fan D. The tumor microenvironment: a key player in multidrug resistance in cancer. Oncologie. (2024) 26:41–58. doi: 10.1515/oncologie-2023-0459
15. Wang Q, Shao X, Zhang Y, Zhu M, Wang FXC, Mu J, et al. Role of tumor microenvironment in cancer progression and therapeutic strategy. Cancer Med. (2023) 12:11149–65. doi: 10.1002/cam4.5698
16. Baghban R, Roshangar L, Jahanban-Esfahlan R, Seidi K, Ebrahimi-Kalan A, Jaymand M, et al. Tumor microenvironment complexity and therapeutic implications at a glance. Cell Commun Signal. (2020) 18:59. doi: 10.1186/s12964-020-0530-4
17. Cha YJ, Koo JS. Role of tumor-associated myeloid cells in breast cancer. Cells. (2020) 9:1785. doi: 10.3390/cells9081785
18. Jiang X, Wang J, Deng X, Xiong F, Zhang S, Gong Z, et al. The role of microenvironment in tumor angiogenesis. J Exp Clin Cancer Res. (2020) 39:204. doi: 10.1186/s13046-020-01709-5
19. Al-Ostoot FH, Salah S, Khamees HA, Khanum SA. Tumor angiogenesis: Current challenges and therapeutic opportunities. Cancer Treat Res Commun. (2021) 28:100422. doi: 10.1016/j.ctarc.2021.100422
20. Neophytou CM, Panagi M, Stylianopoulos T, Papageorgis P. The role of tumor microenvironment in cancer metastasis: molecular mechanisms and therapeutic opportunities. Cancers. (2021) 13:2053. doi: 10.3390/cancers13092053
21. Dzobo K, Dandara C. The extracellular matrix: its composition, function, remodeling, and role in tumorigenesis. Biomimetics. (2023) 8:146. doi: 10.3390/biomimetics8020146
22. Asif PJ, Longobardi C, Hahne M, Medema JP. The role of cancer-associated fibroblasts in cancer invasion and metastasis. Cancers. (2021) 13:4720. doi: 10.3390/cancers13184720
23. Khalaf K, Hana D, Chou JT-T, Singh C, Mackiewicz A, Kaczmarek M. Aspects of the tumor microenvironment involved in immune resistance and drug resistance. Front Immunol. (2021) 12:656364. doi: 10.3389/fimmu.2021.656364
24. Kim SK, Cho SW. The evasion mechanisms of cancer immunity and drug intervention in the tumor microenvironment. Front Pharmacol. (2022) 13:868695. doi: 10.3389/fphar.2022.868695
25. Lin H-J, Liu Y, Lofland D, Lin J. Breast cancer tumor microenvironment and molecular aberrations hijack tumoricidal immunity. Cancers. (2022) 14:285. doi: 10.3390/cancers14020285
26. Mofed D, Omran JI, Sabet S, Baiomy AA, Emara M, Salem TZ. The regulatory role of long non- coding RNAs as a novel controller of immune response against cancer cells. Mol Biol Rep. (2022). doi: 10.1007/s11033-022-07947-4
27. Zhang W, Wang J, Liu C, Li Y, Sun C, Wu J, et al. Crosstalk and plasticity driving between cancer-associated fibroblasts and tumor microenvironment: significance of breast cancer metastasis. J Transl Med. (2023) 21:827. doi: 10.1186/s12967-023-04714-2
28. Said SS, Ibrahim WN. Breaking barriers: the promise and challenges of immune checkpoint inhibitors in triple-negative breast cancer. Biomedicines. (2024) 12:369. doi: 10.3390/biomedicines12020369
29. Srivastava N, Usmani SS, Subbarayan R, Saini R, Pandey PK. Hypoxia: syndicating triple negative breast cancer against various therapeutic regimens. Front Oncol. (2023) 13:1199105. doi: 10.3389/fonc.2023.1199105
30. Chen X, Feng L, Huang Y, Wu Y, Xie N. Mechanisms and strategies to overcome PD-1/PD-L1 blockade resistance in triple-negative breast cancer. Cancers. (2022) 15:104. doi: 10.3390/cancers15010104
31. Li Z, Qiu Y, Lu W, Jiang Y, Wang J. Immunotherapeutic interventions of triple negative breast cancer. J Transl Med. (2018) 16:147. doi: 10.1186/s12967-018-1514-7
32. Seidel JA, Otsuka A, Kabashima K. Anti-PD-1 and anti-CTLA-4 therapies in cancer: mechanisms of action, efficacy, and limitations. Front Oncol. (2018) 8:86. doi: 10.3389/fonc.2018.00086
33. Zabeti Touchaei A, Vahidi S. MicroRNAs as regulators of immune checkpoints in cancer immunotherapy: targeting PD-1/PD-L1 and CTLA-4 pathways. Cancer Cell Int. (2024) 24:102. doi: 10.1186/s12935-024-03293-6
34. Salemme V, Centonze G, Cavallo F, Defilippi P, Conti L. The crosstalk between tumor cells and the immune microenvironment in breast cancer: implications for immunotherapy. Front Oncol. (2021) 11:610303. doi: 10.3389/fonc.2021.610303
35. Rothlin CV, Ghosh S. Lifting the innate immune barriers to antitumor immunity. J Immunother Cancer. (2020) 8:e000695. doi: 10.1136/jitc-2020-000695
36. Zalfa C, Paust S. Natural killer cell interactions with myeloid derived suppressor cells in the tumor microenvironment and implications for cancer immunotherapy. Front Immunol. (2021) 12:633205. doi: 10.3389/fimmu.2021.633205
37. Zhu Y, Yu X, Thamphiwatana SD, Zheng Y, Pang Z. Nanomedicines modulating tumor immunosuppressive cells to enhance cancer immunotherapy. Acta Pharm Sin B. (2020) 10:2054–74. doi: 10.1016/j.apsb.2020.08.010
38. Qureshi S, Chan N, George M, Ganesan S, Toppmeyer D, Omene C. Immune checkpoint inhibitors in triple negative breast cancer: the search for the optimal biomarker. Biomark�Insights. (2022) 17:117727192210787. doi: 10.1177/11772719221078774
39. Liu Y, Hu Y, Xue J, Li J, Yi J, Bu J, et al. Advances in immunotherapy for triple-negative breast cancer. Mol Cancer. (2023) 22:145. doi: 10.1186/s12943-023-01850-7
40. Obidiro O, Battogtokh G, Akala EO. Triple negative breast cancer treatment options and limitations: future outlook. Pharmaceutics. (2023) 15:1796. doi: 10.3390/pharmaceutics15071796
41. Yin L, Duan J-J, Bian X-W, Yu S. Triple-negative breast cancer molecular subtyping and treatment progress. Breast Cancer Res. (2020) 22:61. doi: 10.1186/s13058-020-01296-5
42. Zhang X, Ge X, Jiang T, Yang R, Li S. Research progress on immunotherapy in triple−negative breast cancer (Review). Int J Oncol. (2022) 61:95. doi: 10.3892/ijo.2022.5385
43. Furukawa N, Stearns V, Santa-Maria CA, Popel AS. The tumor microenvironment and triple-negative breast cancer aggressiveness: shedding light on mechanisms and targeting. Expert Opin Ther Targets. (2022) 26:1041–56. doi: 10.1080/14728222.2022.2170779
44. Yuan Z, Li Y, Zhang S, Wang X, Dou H, Yu X, et al. Extracellular matrix remodeling in tumor progression and immune escape: from mechanisms to treatments. Mol Cancer. (2023) 22:48. doi: 10.1186/s12943-023-01744-8
45. Du T, Shi Y, Xu S, Wan X, Sun H, Liu B. Long non-coding RNAs in drug resistance of breast cancer. OTT. (2020) 13:7075–87. doi: 10.2147/OTT.S255226
46. Chen Y, Deng Y, Zhang B, Gong C-X. Deregulation of brain insulin signaling in Alzheimer’s disease. Neurosci Bull. (2014) 30:282–94. doi: 10.1007/s12264-013-1408-x
47. Chen F-Y, Zhou Z-Y, Zhang K-J, Pang J, Wang S-M. Long non-coding RNA MIR100HG promotes the migration, invasion and proliferation of triple-negative breast cancer cells by targeting the miR-5590-3p/OTX1 axis. Cancer Cell Int. (2020) 20:508. doi: 10.1186/s12935-020-01580-6
48. Mao X, Xu J, Wang W, Liang C, Hua J, Liu J, et al. Crosstalk between cancer-associated fibroblasts and immune cells in the tumor microenvironment: new findings and future perspectives. Mol Cancer. (2021) 20:131. doi: 10.1186/s12943-021-01428-1
49. Zheng Y, Han Y, Sun Q, Li Z. Harnessing anti-tumor and tumor-tropism functions of macrophages via nanotechnology for tumor immunotherapy. Exploration. (2022) 2:20210166. doi: 10.1002/EXP.20210166
50. Xu Y, Wang X, Liu L, Wang J, Wu J, Sun C. Role of macrophages in tumor progression and therapy (Review). Int J Oncol. (2022) 60:57. doi: 10.3892/ijo.2022.5347
51. Zheng Y, Li S, Tang H, Meng X, Zheng Q. Molecular mechanisms of immunotherapy resistance in triple-negative breast cancer. Front Immunol. (2023) 14:1153990. doi: 10.3389/fimmu.2023.1153990
52. Lu X, Wang X, Cheng H, Wang X, Liu C, Tan X. Anti-triple-negative breast cancer metastasis efficacy and molecular mechanism of the STING agonist for innate immune pathway. Ann Med. (2023) 55:2210845. doi: 10.1080/07853890.2023.2210845
53. Kumar M, Jalota A, Sahu SK, Haque S. Therapeutic antibodies for the prevention and treatment of cancer. J BioMed Sci. (2024) 31:6. doi: 10.1186/s12929-024-00996-w
54. Xing J, Zhang J, Wang J. The immune regulatory role of adenosine in the tumor microenvironment. IJMS. (2023) 24:14928. doi: 10.3390/ijms241914928
55. Mustachio LM, Chelariu-Raicu A, Szekvolgyi L, Roszik J. Targeting KRAS in cancer: promising therapeutic strategies. Cancers. (2021) 13:1204. doi: 10.3390/cancers13061204
56. Yin G, Huang J, Petela J, Jiang H, Zhang Y, Gong S, et al. Targeting small GTPases: emerging grasps on previously untamable targets, pioneered by KRAS. Sig Transduct Target Ther. (2023) 8:212. doi: 10.1038/s41392-023-01441-4
57. Zhu C, Guan X, Zhang X, Luan X, Song Z, Cheng X, et al. Targeting KRAS mutant cancers: from druggable therapy to drug resistance. Mol Cancer. (2022) 21:159. doi: 10.1186/s12943-022-01629-2
58. Asimgil H, Ertetik U, Çevik NC, Ekizce M, Doğruöz A, Gökalp M, et al. Targeting the undruggable oncogenic KRAS: the dawn of hope. JCI Insight. (2022) 7:e153688. doi: 10.1172/jci.insight.153688
59. Yang H, Zhou X, Fu D, Le C, Wang J, Zhou Q, et al. Targeting RAS mutants in Malignancies: successes, failures, and reasons for hope. Cancer Commun. (2023) 43:42–74. doi: 10.1002/cac2.12377
60. Durinikova E, Buzo K and Arena S. Preclinical models as patients’ avatars for precision medicine in colorectal cancer: past and future challenges. J Exp Clin Cancer Res. (2021) 40:185. doi: 10.1186/s13046-021-01981-z
61. Miserocchi G, Bocchini M, Cortesi M, Arienti C, De Vita A, Liverani C, et al. Combining preclinical tools and models to unravel tumor complexity: Jump into the next dimension. Front Immunol. (2023) 14:1171141. doi: 10.3389/fimmu.2023.1171141
62. Yang X, Guo Y, Chen C, Shao B, Zhao L, Zhou Q, et al. Interaction between intestinal microbiota and tumour immunity in the tumour microenvironment. Immunology. (2021) 164:476–93. doi: 10.1111/imm.13397
63. Greco S, Fabbri N, Spaggiari R, De Giorgi A, Fabbian F, Giovine A. Update on classic and novel approaches in metastatic triple-negative breast cancer treatment: A comprehensive review. Biomedicines. (2023) 11:1772. doi: 10.3390/biomedicines11061772
64. Magwenyane AM, Ugbaja SC, Amoako DG, Somboro AM, Khan RB, Kumalo HM. Heat shock protein 90 (HSP90) inhibitors as anticancer medicines: A review on the computer-aided drug discovery approaches over the past five years. Comput Math Methods Med. (2022) 2022:1–20. doi: 10.1155/2022/2147763
65. Jaradat SK, Ayoub NM, Al Sharie AH, Aldaod JM. Targeting receptor tyrosine kinases as a novel strategy for the treatment of triple-negative breast cancer. Technol Cancer Res Treat. (2024) 23:15330338241234780. doi: 10.1177/15330338241234780
66. Thakur V, Kutty RV. Recent advances in nanotheranostics for triple negative breast cancer treatment. J Exp Clin Cancer Res. (2019) 38:430. doi: 10.1186/s13046-019-1443-1
67. Metibemu DS, Akinloye OA, Akamo AJ, Ojo DA, Okeowo OT, Omotuyi IO. Exploring receptor tyrosine kinases-inhibitors in Cancer treatments. Egypt J Med Hum Genet. (2019) 20:35. doi: 10.1186/s43042-019-0035-0
68. Gonçalves A, Bertucci A, Bertucci F. PARP inhibitors in the treatment of early breast cancer: the step beyond? Cancers. (2020) 12:1378. doi: 10.3390/cancers12061378
69. Singh DD, Parveen A, Yadav DK. Role of PARP in TNBC: mechanism of inhibition, clinical applications, and resistance. Biomedicines. (2021) 9:1512. doi: 10.3390/biomedicines9111512
70. Yang R, Li Y, Wang H, Qin T, Yin X, Ma X. Therapeutic progress and challenges for triple negative breast cancer: targeted therapy and immunotherapy. Mol BioMed. (2022) 3:8. doi: 10.1186/s43556-022-00071-6
71. Li Q, Li Z, Luo T, Shi H. Targeting the PI3K/AKT/mTOR and RAF/MEK/ERK pathways for cancer therapy. Mol BioMed. (2022) 3:47. doi: 10.1186/s43556-022-00110-2
72. Maccallini C, Ammazzalorso A, De Filippis B, Fantacuzzi M, Giampietro L, Amoroso R. HDAC inhibitors for the therapy of triple negative breast cancer. Pharmaceuticals. (2022) 15:667. doi: 10.3390/ph15060667
73. Li Z-N, Luo Y. HSP90 inhibitors and cancer: Prospects for use in targeted therapies (Review). Oncol Rep. (2022) 49:6. doi: 10.3892/or.2022.8443
74. Djamgoz MBA, Fraser SP, Brackenbury WJ. In vivo evidence for voltage-gated sodium channel expression in carcinomas and potentiation of metastasis. Cancers. (2019) 11:1675. doi: 10.3390/cancers11111675
75. Rajaratinam H, Mokhtar NF, Asma-Abdullah N, Fuad WEM. Discovering the triad between nav1.5, breast cancer, and the immune system: A fundamental review and future perspectives. Biomolecules. (2022) 12:310. doi: 10.3390/biom12020310
76. Damaskos C, Garmpis N, Garmpi A, Nikolettos K, Sarantis P, Georgakopoulou VE, et al. Investigational drug treatments for triple-negative breast cancer. JPM. (2021) 11:652. doi: 10.3390/jpm11070652
77. Zhang Y, Li Z, Huang Y, Zou B, Xu Y. Amplifying cancer treatment: advances in tumor immunotherapy and nanoparticle-based hyperthermia. Front Immunol. (2023) 14:1258786. doi: 10.3389/fimmu.2023.1258786
78. Wang L, Cao J, Li C, Wang X, Zhao Y, Li T, et al. Efficacy and safety of mitoxantrone hydrochloride liposome injection in Chinese patients with advanced breast cancer: a randomized, open-label, active-controlled, single-center, phase II clinical trial. Invest New Drugs. (2022) 40:330–9. doi: 10.1007/s10637-021-01182-7
79. Fujimura T, Kambayashi Y, Aiba S. Crosstalk between regulatory T cells (Tregs) and myeloid derived suppressor cells (MDSCs) during melanoma growth. OncoImmunology. (2012) 1:1433–4. doi: 10.4161/onci.21176
80. Carlino F, Diana A, Piccolo A, Ventriglia A, Bruno V, De Santo I, et al. Immune-based therapy in triple-negative breast cancer: from molecular biology to clinical practice. Cancers. (2022) 14:2102. doi: 10.3390/cancers14092102
81. Emami Nejad A, Najafgholian S, Rostami A, Sistani A, Shojaeifar S, Esparvarinha M, et al. The role of hypoxia in the tumor microenvironment and development of cancer stem cell: a novel approach to developing treatment. Cancer Cell Int. (2021) 21:62. doi: 10.1186/s12935-020-01719-5
82. Hazini A, Fisher K, Seymour L. Deregulation of HLA-I in cancer and its central importance for immunotherapy. J Immunother Cancer. (2021) 9:e002899. doi: 10.1136/jitc-2021-002899
83. Aparicio B, Repáraz D, Ruiz M, Llopiz D, Silva L, Vercher E, et al. Identification of HLA class I-restricted immunogenic neoantigens in triple negative breast cancer. Front Immunol. (2022) 13:985886. doi: 10.3389/fimmu.2022.985886
84. Ribeiro R, Carvalho MJ, Goncalves J, Moreira JN. Immunotherapy in triple-negative breast cancer: Insights into tumor immune landscape and therapeutic opportunities. Front Mol Biosci. (2022) 9:903065. doi: 10.3389/fmolb.2022.903065
85. Taylor BC, Balko JM. Mechanisms of MHC-I downregulation and role in immunotherapy response. Front Immunol. (2022) 13:844866. doi: 10.3389/fimmu.2022.844866
86. Scheffges C, Devy J, Giustiniani J, Francois S, Cartier L, Merrouche Y, et al. Identification of CD160-TM as a tumor target on triple negative breast cancers: possible therapeutic applications. Breast Cancer Res. (2024) 26:28. doi: 10.1186/s13058-024-01785-x
87. Xiao Q, Nobre A, Piñeiro P, Berciano-Guerrero M-Á, Alba E, Cobo M, et al. Genetic and epigenetic biomarkers of immune checkpoint blockade response. JCM. (2020) 9:286. doi: 10.3390/jcm9010286
88. Zolota V, Tzelepi V, Piperigkou Z, Kourea H, Papakonstantinou E, Argentou M-I, et al. Epigenetic alterations in triple-negative breast cancer—The critical role of extracellular matrix. Cancers. (2021) 13:713. doi: 10.3390/cancers13040713
89. Jiang W, Wang X, Zhang C, Xue L, Yang L. Expression and clinical significance of MAPK and EGFR in triple−negative breast cancer. Oncol Lett. (2020). doi: 10.3892/ol.2020.11274
90. Omarini C, Guaitoli G, Pipitone S, Moscetti L, Cortesi L, Cascinu S, et al. Neoadjuvant treatments in triple-negative breast cancer patients: where we are now and where we are going. CMAR. (2018) 10:91–103. doi: 10.2147/CMAR.S146658
91. Li X, Xiang Y, Li F, Yin C, Li B, Ke X. WNT/β-catenin signaling pathway regulating T cell-inflammation in the tumor microenvironment. Front Immunol. (2019) 10:2293. doi: 10.3389/fimmu.2019.02293
92. Merikhian P, Eisavand MR, Farahmand L. Triple-negative breast cancer: understanding Wnt signaling in drug resistance. Cancer Cell Int. (2021) 21:419. doi: 10.1186/s12935-021-02107-3
93. Ding H, Wang G, Yu Z, Sun H, Wang L. Role of interferon-gamma (IFN-γ) and IFN-γ receptor 1/2 (IFNγR1/2) in regulation of immunity, infection, and cancer development: IFN-γ-dependent or independent pathway. Biomedicine Pharmacotherapy. (2022) 155:113683. doi: 10.1016/j.biopha.2022.113683
94. Zhou X, Ni Y, Liang X, Lin Y, An B, He X, et al. Mechanisms of tumor resistance to immune checkpoint blockade and combination strategies to overcome resistance. Front Immunol. (2022) 13:915094. doi: 10.3389/fimmu.2022.915094
95. Chehelgerdi M, Chehelgerdi M, Allela OQB, Pecho RDC, Jayasankar N, Rao DP, et al. Progressing nanotechnology to improve targeted cancer treatment: overcoming hurdles in its clinical implementation. Mol Cancer. (2023) 22:169. doi: 10.1186/s12943-023-01865-0
96. Liu S, Li J, Gu L, Wu K, Xing H. Nanoparticles for chemoimmunotherapy against triple-negative breast cancer. IJN. (2022) 17:5209–27. doi: 10.2147/IJN.S388075
97. Hou K, Ning Z, Chen H, Wu Y. Nanomaterial technology and triple negative breast cancer. Front Oncol. (2022) 11:828810. doi: 10.3389/fonc.2021.828810
98. Wang C, Jiang Y, Li X, Hu L. Thioglucose-bound gold nanoparticles increase the radiosensitivity of a triple-negative breast cancer cell line (MDA-MB-231). Breast Cancer. (2015) 22:413–20. doi: 10.1007/s12282-013-0496-9
99. De Vita A, Liverani C, Molinaro R, Martinez JO, Hartman KA, Spadazzi C, et al. Lysyl oxidase engineered lipid nanovesicles for the treatment of triple negative breast cancer. Sci Rep. (2021) 11:5107. doi: 10.1038/s41598-021-84492-3
100. Deng ZJ, Morton SW, Ben-Akiva E, Dreaden EC, Shopsowitz KE, Hammond PT. Layer-by-layer nanoparticles for systemic codelivery of an anticancer drug and siRNA for potential triple-negative breast cancer treatment. ACS Nano. (2013) 7:9571–84. doi: 10.1021/nn4047925
101. Barani M, Hajinezhad MR, Sargazi S, Rahdar A, Shahraki S, Lohrasbi-Nejad A, et al. In vitro and in vivo anticancer effect of pH-responsive paclitaxel-loaded niosomes. J Mater Sci: Mater Med. (2021) 32:147. doi: 10.1007/s10856-021-06623-6
102. Imran H, Tang Y, Wang S, Yan X, Liu C, Guo L, et al. Optimized DOX drug deliveries via chitosan-mediated nanoparticles and stimuli responses in cancer chemotherapy: A review. Molecules. (2023) 29:31. doi: 10.3390/molecules29010031
103. Mikhaylov G, Klimpel D, Schaschke N, Mikac U, Vizovisek M, Fonovic M, et al. Selective targeting of tumor and stromal cells by a nanocarrier system displaying lipidated cathepsin B inhibitor. Angew Chem Int Ed. (2014) 53:10077–81. doi: 10.1002/anie.201402305
104. Tang J, Howard CB, Mahler SM, Thurecht KJ, Huang L, Xu ZP. Enhanced delivery of siRNA to triple negative breast cancer cells in vitro and in vivo through functionalizing lipid-coated calcium phosphate nanoparticles with dual target ligands. Nanoscale. (2018) 10:4258–66. doi: 10.1039/C7NR08644J
105. Da Rocha MCO, Da Silva PB, Radicchi MA, Andrade BYG, De Oliveira JV, Venus T, et al. Docetaxel-loaded solid lipid nanoparticles prevent tumor growth and lung metastasis of 4T1 murine mammary carcinoma cells. J Nanobiotechnol. (2020) 18:43. doi: 10.1186/s12951-020-00604-7
106. Cui G, Wu J, Lin J, Liu W, Chen P, Yu M, et al. Graphene-based nanomaterials for breast cancer treatment: promising therapeutic strategies. J Nanobiotechnol. (2021) 19:211. doi: 10.1186/s12951-021-00902-8
107. Katiyar SS, Muntimadugu E, Rafeeqi TA, Domb AJ, Khan W. Co-delivery of rapamycin- and piperine-loaded polymeric nanoparticles for breast cancer treatment. Drug Delivery. (2016) 23:2608–16. doi: 10.3109/10717544.2015.1039667
108. Zuo S, Wang Z, An X, Wang J, Zheng X, Shao D, et al. Self-assembly engineering nanodrugs composed of paclitaxel and curcumin for the combined treatment of triple negative breast cancer. Front Bioeng Biotechnol. (2021) 9:747637. doi: 10.3389/fbioe.2021.747637
109. Jin X, Wei Y, Liu Y, Lu X, Ding F, Wang J, et al. Resveratrol promotes sensitization to Doxorubicin by inhibiting epithelial-mesenchymal transition and modulating SIRT1/β-catenin signaling pathway in breast cancer. Cancer Med. (2019) 8:1246–57. doi: 10.1002/cam4.1993
110. Zhao Y, Huan M, Liu M, Cheng Y, Sun Y, Cui H, et al. Doxorubicin and resveratrol co-delivery nanoparticle to overcome doxorubicin resistance. Sci Rep. (2016) 6:35267. doi: 10.1038/srep35267
111. Gharoonpour A, Simiyari D, Yousefzadeh A, Badragheh F, Rahmati M. Autophagy modulation in breast cancer utilizing nanomaterials and nanoparticles. Front Oncol. (2023) 13:1150492. doi: 10.3389/fonc.2023.1150492
112. Dasari N, Guntuku GS, Pindiprolu SKSS. Targeting triple negative breast cancer stem cells using nanocarriers. Discover Nano. (2024) 19:41. doi: 10.1186/s11671-024-03985-y
113. Si Y, Zhang Y, Ngo HG, Guan J-S, Chen K, Wang Q, et al. Targeted liposomal chemotherapies to treat triple-negative breast cancer. Cancers. (2021) 13:3749. doi: 10.3390/cancers13153749
114. Wang Q, Atluri K, Tiwari AK, Babu RJ. Exploring the application of micellar drug delivery systems in cancer nanomedicine. Pharmaceuticals. (2023) 16:433. doi: 10.3390/ph16030433
115. Martey O, Nimick M, Taurin S, Sundararajan V, Greish K, Rosengren RJ. Styrene maleic acid-encapsulated RL71 micelles suppress tumor growth in a murine xenograft model of triple negative breast cancer. IJN. (2017) 12:7225–37. doi: 10.2147/IJN.S148908
116. Jiang Q, Liu L, Li Q, Cao Y, Chen D, Du Q, et al. NIR-laser-triggered gadolinium-doped carbon dots for magnetic resonance imaging, drug delivery and combined photothermal chemotherapy for triple negative breast cancer. J Nanobiotechnol. (2021) 19:64. doi: 10.1186/s12951-021-00811-w
117. Zielińska A, Carreiró F, Oliveira AM, Neves A, Pires B, Venkatesh DN, et al. Polymeric nanoparticles: production, characterization, toxicology and ecotoxicology. Molecules. (2020) 25:3731. doi: 10.3390/molecules25163731
118. Chu X, Li W, Hines MG, Lyakhov I, Mellors JW, Dimitrov DS. Human antibody V H domains targeting GPNMB and VCAM-1 as candidate therapeutics for cancers. Mol Pharmaceutics. (2023) 20:2754–60. doi: 10.1021/acs.molpharmaceut.3c00173
119. Bhargava-Shah A, Foygel K, Devulapally R, Paulmurugan R. Orlistat and antisense-miRNA-loaded PLGA-PEG nanoparticles for enhanced triple negative breast cancer therapy. Nanomedicine. (2016) 11:235–47. doi: 10.2217/nnm.15.193
120. Chen R, Ni S, Chen W, Liu M, Feng J, Hu K. Improved anti-triple negative breast cancer effects of docetaxel by RGD-modified lipid-core micelles. IJN. (2021) 16:5265–79. doi: 10.2147/IJN.S313166
121. Zhang Y, Tian X, Wang Z, Wang H, Liu F, Long Q, et al. Advanced applications of DNA nanostructures dominated by DNA origami in antitumor drug delivery. Front Mol Biosci. (2023) 10:1239952. doi: 10.3389/fmolb.2023.1239952
122. Setyawati MI, Kutty RV, Tay CY, Yuan X, Xie J, Leong DT. Novel Theranostic DNA Nanoscaffolds for the Simultaneous Detection and Killing of Escherichia coli and Staphylococcus aureus. ACS Appl Mater Interfaces. (2014) 6:21822–31. doi: 10.1021/am502591c
123. Liu Y, Zhang D, Zhang Z, Liang X, Yang X, Ding N, et al. Multifunctional nanoparticles inhibit tumor and tumor-associated macrophages for triple-negative breast cancer therapy. J Colloid Interface Sci. (2024) 657:598–610. doi: 10.1016/j.jcis.2023.11.156
124. Xu J-J, Zhang W-C, Guo Y-W, Chen X-Y, Zhang Y-N. Metal nanoparticles as a promising technology in targeted cancer treatment. Drug Delivery. (2022) 29:664–78. doi: 10.1080/10717544.2022.2039804
125. Surapaneni SK, Bashir S, Tikoo K. Gold nanoparticles-induced cytotoxicity in triple negative breast cancer involves different epigenetic alterations depending upon the surface charge. Sci Rep. (2018) 8:12295. doi: 10.1038/s41598-018-30541-3
126. Keihan Shokooh M, Emami F, Jeong J-H, Yook S. Bio-inspired and smart nanoparticles for triple negative breast cancer microenvironment. Pharmaceutics. (2021) 13:287. doi: 10.3390/pharmaceutics13020287
127. Hu C, Du W. Zinc oxide nanoparticles (ZnO NPs) combined with cisplatin and gemcitabine inhibits tumor activity of NSCLC cells. Aging. (2020) 12:25767–77. doi: 10.18632/aging.104187
128. Luo X, Wang H, Ji D. Carbon nanotubes (CNT)-loaded ginsenosides Rb3 suppresses the PD-1/PD-L1 pathway in triple-negative breast cancer. Aging. (2021) 13:17177–89. doi: 10.18632/aging.203131
129. Tang L, Xiao Q, Mei Y, He S, Zhang Z, Wang R, et al. Insights on functionalized carbon nanotubes for cancer theranostics. J Nanobiotechnol. (2021) 19:423. doi: 10.1186/s12951-021-01174-y
130. Agnello L, d’Argenio A, Nilo R, Fedele M, Camorani S, Cerchia L. Aptamer-based strategies to boost immunotherapy in TNBC. Cancers. (2023) 15:2010. doi: 10.3390/cancers15072010
131. Jahan S, Karim M, Chowdhury EH. Nanoparticles targeting receptors on breast cancer for efficient delivery of chemotherapeutics. Biomedicines. (2021) 9:114. doi: 10.3390/biomedicines9020114
132. Wang L. Terahertz imaging for breast cancer detection. Sensors. (2021) 21:6465. doi: 10.3390/s21196465
133. Juan A, Cimas FJ, Bravo I, Pandiella A, Ocaña A, Alonso-Moreno C. Antibody conjugation of nanoparticles as therapeutics for breast cancer treatment. IJMS. (2020) 21:6018. doi: 10.3390/ijms21176018
134. Kobayashi K, Sasaki T, Takenaka F, Yakushiji H, Fujii Y, Kishi Y, et al. A novel PET imaging using 64Cu-labeled monoclonal antibody against mesothelin commonly expressed on cancer cells. J Immunol Res. (2015) 2015:268172. doi: 10.1155/2015/268172
135. Naeimi R, Bahmani A, Afshar S. Investigating the role of peptides in effective therapies against cancer. Cancer Cell Int. (2022) 22:139. doi: 10.1186/s12935-022-02553-7
136. Song N, Zhao L, Zhu M, Zhao J. 99m tc-labeled lyP-1 for SPECT imaging of triple negative breast cancer. Contrast Media Mol Imaging. (2019) 2019:1–11. doi: 10.1155/2019/9502712
137. Zhang H, Zhang Y, Zhang C, Yu H, Ma Y, Li Z, et al. Recent advances of cell-penetrating peptides and their application as vectors for delivery of peptide and protein-based cargo molecules. Pharmaceutics. (2023) 15:2093. doi: 10.3390/pharmaceutics15082093
138. Wu P-H, Opadele AE, Onodera Y, Nam J-M. Targeting integrins in cancer nanomedicine: applications in cancer diagnosis and therapy. Cancers. (2019) 11:1783. doi: 10.3390/cancers11111783
139. Nel J, Elkhoury K, Velot É, Bianchi A, Acherar S, Francius G, et al. Functionalized liposomes for targeted breast cancer drug delivery. Bioactive Materials. (2023) 24:401–37. doi: 10.1016/j.bioactmat.2022.12.027
140. Mendes TFS, Kluskens LD, Rodrigues LR. Triple negative breast cancer: nanosolutions for a big challenge. Advanced Sci. (2015) 2:1500053. doi: 10.1002/advs.201500053
141. Nagayama A, Vidula N, Ellisen L, Bardia A. Novel antibody–drug conjugates for triple negative breast cancer. Ther Adv Med Oncol. (2020) 12:175883592091598. doi: 10.1177/1758835920915980
142. Nabil G, Alzhrani R, Alsaab H, Atef M, Sau S, Iyer A, et al. CD44 targeted nanomaterials for treatment of triple-negative breast cancer. Cancers. (2021) 13:898. doi: 10.3390/cancers13040898
143. Zhai B-T, Tian H, Sun J, Zou J-B, Zhang X-F, Cheng J-X, et al. Urokinase-type plasminogen activator receptor (uPAR) as a therapeutic target in cancer. J Transl Med. (2022) 20:135. doi: 10.1186/s12967-022-03329-3
144. Ruzzi F, Semprini MS, Scalambra L, Palladini A, Angelicola S, Cappello C, et al. Virus-like particle (VLP) vaccines for cancer immunotherapy. IJMS. (2023) 24:12963. doi: 10.3390/ijms241612963
145. Nooraei S, Bahrulolum H, Hoseini ZS, Katalani C, Hajizade A, Easton AJ, et al. Virus-like particles: preparation, immunogenicity and their roles as nanovaccines and drug nanocarriers. J Nanobiotechnol. (2021) 19:59. doi: 10.1186/s12951-021-00806-7
146. Zeltins A. Construction and characterization of virus-like particles: A review. Mol Biotechnol. (2013) 53:92–107. doi: 10.1007/s12033-012-9598-4
147. Fu Y, Li J. A novel delivery platform based on Bacteriophage MS2 virus-like particles. Virus Res. (2016) 211:9–16. doi: 10.1016/j.virusres.2015.08.022
148. Murray V, Chen J, Chung L. The interaction of the metallo-glycopeptide anti-tumour drug bleomycin with DNA. IJMS. (2018) 19:1372. doi: 10.3390/ijms19051372
149. Ahn HK, Sim SH, Suh KJ, Kim MH, Jeong JH, Kim JY, et al. Response rate and safety of a neoadjuvant pertuzumab, atezolizumab, docetaxel, and trastuzumab regimen for patients with ERBB2-positive stage II/III breast cancer: the neo-PATH phase 2 nonrandomized clinical trial. JAMA Oncol. (2022) 8:1271–7. doi: 10.1001/jamaoncol.2022.2310
150. Ankireddy SR, Vo VG, An SSA, Kim J. Solvent-free synthesis of fluorescent carbon dots: an ecofriendly approach for the bioimaging and screening of anticancer activity via caspase-induced apoptosis. ACS Appl Bio Mater. (2020) 3:4873–82. doi: 10.1021/acsabm.0c00377
151. Cho SB. Uncovering oncogenic mechanisms of tumor suppressor genes in breast cancer multi-omics data. Int J Mol Sci. (2022) 23. doi: 10.3390/ijms23179624
152. Hwang S, Kim SH, Yoo KH, Chung MH, Lee JW, Son KH. Exogenous 8-hydroxydeoxyguanosine attenuates doxorubicin-induced cardiotoxicity by decreasing pyroptosis in H9c2 cardiomyocytes. BMC Mol Cell Biol. (2022) 23:55. doi: 10.1186/s12860-022-00454-1
153. Jin W. Novel insights into PARK7 (DJ-1), a potential anti-cancer therapeutic target, and implications for cancer progression. J Clin Med. (2020) 9. doi: 10.3390/jcm9051256
154. Jung HJ, Song KS, Son YK, Seong JK, Kim SY, Oh SH. 1,7-Bis(4-hydroxyphenyl)-4-hepten-3-one from Betula platyphylla induces apoptosis by suppressing autophagy flux and activating the p38 pathway in lung cancer cells. Phytother Res. (2020) 34:126–38. doi: 10.1002/ptr.6506
155. Kang MA, Jeon YK, Nam MJ. Auricularia auricula increases an apoptosis in human hepatocellular carcinoma cells via a regulation of the peroxiredoxin1. J Food Biochem. 2020:e13373. doi: 10.1111/jfbc.13373
156. Khaliq SA, Baek MO, Cho HJ, Chon SJ, Yoon MS. C-peptide inhibits decidualization in human endometrial stromal cells via GSK3beta-PP1. Front Cell Dev Biol. (2020) 8:609551. doi: 10.3389/fcell.2020.609551
157. Kim HJ, Kim D, Yoon H, Choi CS, Oh YS, Jun HS. Prevention of oxidative stress-induced pancreatic beta cell damage by broussonetia kazinoki siebold fruit extract via the ERK-nox4 pathway. Antioxidants (Basel). (2020) 9. doi: 10.3390/antiox9050406
158. Kim YJ, Kim KG. Detection and weak segmentation of masses in gray-scale breast mammogram images using deep learning. Yonsei Med J. (2022) 63:S63–73. doi: 10.3349/ymj.2022.63.S63
159. Lee D, Kwak HJ, Kim BH, Kim DW, Kim HY, Kim SH, et al. Brevilin A Isolated from Centipeda minima Induces Apoptosis in Human Gastric Cancer Cells via an Extrinsic Apoptotic Signaling Pathway. Plants (Basel). (2022) 11. doi: 10.3390/plants11131658
160. Lee D, Lee YH, Lee KH, Lee BS, Alishir A, Ko Y-J, et al. Aviculin Isolated from Lespedeza cuneata Induce Apoptosis in Breast Cancer Cells through Mitochondria-Mediated Caspase Activation Pathway. Molecules. (2020) 25. doi: 10.3390/molecules25071708
161. Lee J, Nguyen QN, Park JY, Lee S, Hwang GS, Yamabe N, et al. Protective effect of shikimic acid against cisplatin-induced renal injury: in vitro and in vivo studies. Plants (Basel). (2020) 9. doi: 10.3390/plants9121681
162. Lee J, Oh AR, Lee HY, Moon YA, Lee HJ, Cha JY. Deletion of KLF10 leads to stress-induced liver fibrosis upon high sucrose feeding. Int J Mol Sci. (2020) 22. doi: 10.3390/ijms22010331
163. Lee MS, Lim SH, Yu AR, Hwang CY, Kang I, Yeo EJ. Carfilzomib in combination with bortezomib enhances apoptotic cell death in B16-F1 melanoma cells. Biol (Basel). (2021) 10. doi: 10.3390/biology10020153
164. Lee SY. Ginsenoside rg1 drives stimulations of timosaponin AIII-induced anticancer effects in human osteosarcoma cells. Evid Based Complement Alternat Med. (2020) 2020:8980124. doi: 10.1155/2020/8980124
165. Mishra SK, Bae YS, Lee YM, Kim JS, Oh SH, Kim HM. Sesquiterpene alcohol cedrol chemosensitizes human cancer cells and suppresses cell proliferation by destabilizing plasma membrane lipid rafts. Front Cell Dev Biol. (2020) 8:571676. doi: 10.3389/fcell.2020.571676
166. Nguyen QN, Lee SR, Kim B, Hong J-H, Jang YS, Lee DE, et al. Estrogenic Activity of 4-Hydroxy-Benzoic Acid from Acer tegmentosum via Estrogen Receptor alpha-Dependent Signaling Pathways. Plants (Basel). (2022) 11. doi: 10.3390/plants11233387
167. Nguyen TT, Kim JW, Choi HI, Maeng HJ, Koo TS. Development of an LC-MS/MS method for ARV-110, a PROTAC molecule, and applications to pharmacokinetic studies. Molecules. (2022) 27. doi: 10.3390/molecules27061977
168. Nguyen TTN, Choi H, Jun HS. Preventive effects of dulaglutide on disuse muscle atrophy through inhibition of inflammation and apoptosis by induction of hsp72 expression. Front Pharmacol. (2020) 11:90. doi: 10.3389/fphar.2020.00090
169. Oh HS, Seo HJ. Association between WHO first-step analgesic use and risk of breast cancer in women of working age. Pharm (Basel). (2023) 16. doi: 10.3390/ph16020323
170. Oh S, Son M, Park CH, Jang JT, Son KH, Byun K. The reducing effects of pyrogallol-phloroglucinol-6,6-bieckol on high-fat diet-induced pyroptosis in endothelial and vascular smooth muscle cells of mice aortas. Mar Drugs. (2020) 18. doi: 10.3390/md18120648
171. Park S, Kim J, Choi J, Lee C, Lee W, Park S, et al. Lipid raft-disrupting miltefosine preferentially induces the death of colorectal cancer stem-like cells. Clin Transl Med. (2021) 11:e552. doi: 10.1002/ctm2.552
172. Phung HM, Lee S, Hwang JH, Kang KS. Preventive effect of muscone against cisplatin nephrotoxicity in LLC-PK1 cells. Biomolecules. (2020) 10. doi: 10.3390/biom10101444
173. Yaseen U, Hwang S, Park S, Kim SB, Lee HJ, Cha JY. New insights into the role of KLF10 in tissue fibrosis. Int J Mol Sci. (2024) 25. doi: 10.3390/ijms25021276
174. Yumnam S, Kang MC, Oh SH, Kwon HC, Kim JC, Jung ES, et al. Downregulation of dihydrolipoyl dehydrogenase by UVA suppresses melanoma progression via triggering oxidative stress and altering energy metabolism. Free Radic Biol Med. (2021) 162:77–87. doi: 10.1016/j.freeradbiomed.2020.11.037
175. An SSA, Shim KH, Kang S, Kim YK, Subedi L, Cho H, et al. The potential anti-amyloidogenic candidate, SPA1413, for Alzheimer’s disease. Br J Pharmacol. (2022) 179:1033–48. doi: 10.1111/bph.15691
176. Bayarsaikhan D, Bayarsaikhan G, Lee B. Recent advances in stem cells and gene editing: Drug discovery and therapeutics. Prog Mol Biol Transl Sci. (2021) 181:231–69. doi: 10.1016/bs.pmbts.2021.01.019
177. Bayarsaikhan D, Bayarsaikhan G, Lee J, Lee B. A study on the protective effect of sRAGE-MSCs in a rodent reperfusion model of myocardial infarction. Int J Mol Sci. (2022) 23. doi: 10.3390/ijms232415630
178. Byun K-A, Park HJ, Oh S, Batsukh S, Sun HJ, Kim T, et al. High-intensity focused ultrasound decreases subcutaneous fat tissue thickness by increasing apoptosis and autophagy. Biomolecules. (2023) 13. doi: 10.3390/biom13020392
179. Jung HW, Hwang JH. Anticancer effects of ursi fel extract and its active compound, ursodeoxycholic acid, in FRO anaplastic thyroid cancer cells. Molecules. (2021) 26. doi: 10.3390/molecules26175309
180. Kang MA, Lee J, Lee CM, Park HS, Jang KY, Park SH. IL13Ralpha2 is involved in the progress of renal cell carcinoma through the JAK2/FOXO3 pathway. J Pers Med. (2021) 11. doi: 10.3390/jpm11040284
181. Kim D, Kim HJ, Baek JO, Roh JY, Jun HS. Lysophosphatidic acid mediates imiquimod-induced psoriasis-like symptoms by promoting keratinocyte proliferation through LPAR1/ROCK2/PI3K/AKT signaling pathway. Int J Mol Sci. (2021) 22. doi: 10.3390/ijms221910777
182. Kim HJ, Jung YS, Jung YJ, Kim OH, Oh BC. High-phytate diets increase amyloid beta deposition and apoptotic neuronal cell death in a rat model. Nutrients. (2021) 13. doi: 10.3390/nu13124370
183. Kim HY, Kim YM, Hong S. CK2alpha-mediated phosphorylation of GRP94 facilitates the metastatic cascade in triple-negative breast cancer. Cell Death Discovery. (2024) 10:185. doi: 10.1038/s41420-024-01956-x
184. Kim YM, Hong S. Controversial roles of cold−inducible RNA−binding protein in human cancer (Review). Int J Oncol. (2021) 59. doi: 10.3892/ijo.2021.5271
185. Lee D, Hong JH. Ca(2+) signaling as the untact mode during signaling in metastatic breast cancer. Cancers (Basel). (2021) 13. doi: 10.3390/cancers13061473
186. Lee D, Lee S, Jang YS, Ryoo R, Kim JK, Kang KS, et al. N,N-dimethyl-anthranilic acid from calvatia nipponica mushroom fruiting bodies induces apoptotic effects on MDA-MB-231 human breast cancer cells. Nutrients. (2023) 15. doi: 10.3390/nu15143091
187. Lee D, Shim S, Kang K. 4,6’-anhydrooxysporidinone from fusarium lateritium SSF2 induces autophagic and apoptosis cell death in MCF-7 breast cancer cells. Biomolecules. (2021) 11. doi: 10.3390/biom11060869
188. Lee D, Yu JS, Ha JW, Lee SR, Lee BS, Kim J-C, et al. Antitumor potential of withanolide glycosides from ashwagandha (Withania somnifera) on apoptosis of human hepatocellular carcinoma cells and tube formation in human umbilical vein endothelial cells. Antioxidants (Basel). (2022) 11. doi: 10.3390/antiox11091761
189. Lee D, Yu JS, Ryoo R, Kim J-C, Jang TS, Kang KS, et al. Pulveraven A from the fruiting bodies of Pulveroboletus ravenelii induces apoptosis in breast cancer cell via extrinsic apoptotic signaling pathway. J Antibiot (Tokyo). (2021) 74:752–7. doi: 10.1038/s41429-021-00435-0
190. Lee HK, Cha HS, Nam MJ, Park K, Yang Y-H, Lee J, et al. Broussochalcone A induces apoptosis in human renal cancer cells via ROS level elevation and activation of FOXO3 signaling pathway. Oxid Med Cell Longev. (2021) 2021:2800706. doi: 10.1155/2021/2800706
191. Lee HK, Park SH, Nam MJ. Proteasome inhibitor MG132 induces apoptosis in human osteosarcoma U2OS cells. Hum Exp Toxicol. (2021) 40:1985–97. doi: 10.1177/09603271211017972
192. Lee YJ, Lee SY. Maclurin exerts anti-cancer effects in human osteosarcoma cells via prooxidative activity and modulations of PARP, p38, and ERK signaling. IUBMB Life. (2021) 73:1060–72. doi: 10.1002/iub.2506
193. Lim HM, Lee J, Nam MJ, Park SH. Acetylshikonin induces apoptosis in human colorectal cancer HCT-15 and loVo cells via nuclear translocation of FOXO3 and ROS level elevation. Oxid Med Cell Longev. (2021) 2021:6647107. doi: 10.1155/2021/6647107
194. Nam SY, Ahn SJ, Jang YR, Chun YS, Park HK, Choi SJ, et al. A critical review of abdominopelvic computed tomography for the detection of asymptomatic metastasis in new and recurrent breast cancers. Asia Pac J Clin Oncol. (2022) 18:363–70. doi: 10.1111/ajco.13600
195. Ngoc LTN, Moon JY, Lee YC. Beneficial effects of opuntia humifusa (Korean cheonnyuncho) on human health based on antioxidant properties: systematic review and meta-analysis. Antioxidants (Basel). (2023) 12. doi: 10.3390/antiox12010174
196. Nguyen Cao TG, Kang JH, You JY, Kang HC, Rhee WJ, Ko YT, et al. Safe and targeted sonodynamic cancer therapy using biocompatible exosome-based nanosonosensitizers. ACS Appl Mater Interfaces. (2021) 13:25575–88. doi: 10.1021/acsami.0c22883
197. Oh S, Son M, Byun K-A, Jang JT, Choi CH, Son KH, et al. Attenuating effects of dieckol on high-fat diet-induced nonalcoholic fatty liver disease by decreasing the NLRP3 inflammasome and pyroptosis. Mar Drugs. (2021) 19. doi: 10.3390/md19060318
198. Oh S, Yang J, Park C, Son K, Byun K. Dieckol attenuated glucocorticoid-induced muscle atrophy by decreasing NLRP3 inflammasome and pyroptosis. Int J Mol Sci. (2021) 22. doi: 10.3390/ijms22158057
199. Park DW, Kim SH, Park JH. Distribution and characterization of prophages in Lactobacillus plantarum derived from kimchi. Food Microbiol. (2022) 102:103913. doi: 10.1016/j.fm.2021.103913
200. Park EY, Yi M, Kim HS, Kim H. A decision tree model for breast reconstruction of women with breast cancer: A mixed method approach. Int J Environ Res Public Health. (2021) 18. doi: 10.3390/ijerph18073579
201. Rai HM, Yoo J. A comprehensive analysis of recent advancements in cancer detection using machine learning and deep learning models for improved diagnostics. J Cancer Res Clin Oncol. (2023) 149:14365–408. doi: 10.1007/s00432-023-05216-w
202. Shin JW, Kim SH, Yoon JY. PTEN downregulation induces apoptosis and cell cycle arrest in uterine cervical cancer cells. Exp Ther Med. (2021) 22:1100. doi: 10.3892/etm.2021.10534
203. Son J, Lee SY. Emetine exerts anticancer effects in U2OS human osteosarcoma cells via activation of p38 and inhibition of ERK, JNK, and beta-catenin signaling pathways. J Biochem Mol Toxicol. (2021) 35:e22868. doi: 10.1002/jbt.22868
204. Batsukh S, Oh S, Rheu K, Lee B-J, Park C-H, Son KH, et al. Rice germ ameliorated chronic unpredictable mild stress-induced depressive-like behavior by reducing neuroinflammation. Nutrients. (2022) 14. doi: 10.3390/nu14245382
205. Byun K-A, Oh S, Batsukh S, Kim MJ, Lee JH, Park HJ, et al. The extracellular matrix vitalizer RA(TM) increased skin elasticity by modulating mitochondrial function in aged animal skin. Antioxidants (Basel). (2023) 12. doi: 10.3390/antiox12030694
206. Byun KA, Oh S, Yang JY, Lee SY, Son KH, Byun K. Ecklonia cava extracts decrease hypertension-related vascular calcification by modulating PGC-1alpha and SOD2. BioMed Pharmacother. (2022) 153:113283. doi: 10.1016/j.biopha.2022.113283
207. Cha HS, Lee HK, Park SH, Nam MJ. Acetylshikonin induces apoptosis of human osteosarcoma U2OS cells by triggering ROS-dependent multiple signal pathways. Toxicol In Vitro. (2023) 86:105521. doi: 10.1016/j.tiv.2022.105521
208. Choi SH, Lee YW, Kim HS, Kim SH, Lee E-H, Park EY, et al. Development and effects of a post-traumatic growth program for patients with breast cancer. Eur J Oncol Nurs. (2022) 57:102100. doi: 10.1016/j.ejon.2022.102100
209. Jang D, Lee E, Lee S, Kwon Y, Kang KS. System-level investigation of anti-obesity effects and the potential pathways of Cordyceps militaris in ovariectomized rats. BMC Complement Med Ther. (2022) 22:132. doi: 10.1186/s12906-022-03608-y
210. Ji MJ, Son KH, Hong JH. Addition of oh8dG to Cardioplegia Attenuated Myocardial Oxidative Injury through the Inhibition of Sodium Bicarbonate Cotransporter Activity. Antioxidants (Basel). (2022) 11. doi: 10.3390/antiox11091641
211. Jin ML, Yang L, Jeong KW. SETD1A-SOX2 axis is involved in tamoxifen resistance in estrogen receptor alpha-positive breast cancer cells. Theranostics. (2022) 12:5761–75. doi: 10.7150/thno.72599
212. Kang M, Chun YS, Park HK, Cho EK, Jung J, Kim Y. Subsequent pregnancy and long-term safety after breast cancer: a retrospective analysis of Korean health insurance data. Ann Surg Treat Res. (2022) 102:73–82. doi: 10.4174/astr.2022.102.2.73
213. Kim D, Ban KY, Lee GH, Jun HS. Lysophosphatidic acid induces podocyte pyroptosis in diabetic nephropathy by an increase of egr1 expression via downregulation of ezH2. Int J Mol Sci. (2023) 24. doi: 10.3390/ijms24129968
214. Kim EK, Kim Y, Yang JY, Jang HH. Prx1 regulates thapsigargin-mediated UPR activation and apoptosis. Genes (Basel). (2022) 13. doi: 10.3390/genes13112033
215. Kim H, Jung J, Jung H, et al. Comparison of jaw mode and field width for left-breast cancer using tomoDirect three-dimensional conformal radiation therapy: A phantom study. Healthcare (Basel). (2022) 10. doi: 10.3390/healthcare10122431
216. Kim J, Paik Y, Park S. Cancer screening program delivered by community health workers for chinese married immigrant women in korea. Int J Environ Res Public Health. (2022) 19. doi: 10.3390/ijerph19116655
217. Kim J, Ahn S, Nam S, Kim Y, Chun Y, Park H, et al. Breast pseudoaneurysm in a woman after core biopsy: intravascular glue embolization. J Med Ultrasound. (2023) 31:147–9. doi: 10.4103/jmu.jmu_168_21
218. Kim KS, Choi YJ, Jang DS, Lee S. 2-O-beta-d-glucopyranosyl-4,6-dihydroxybenzaldehyde isolated from morus alba (Mulberry) fruits suppresses damage by regulating oxidative and inflammatory responses in TNF-alpha-induced human dermal fibroblasts. Int J Mol Sci. (2022) 23. doi: 10.3390/ijms232314802
219. Kim O-H, Kang G-H, Hur J, Lee J, Jung Y, Hong I-S, et al. Externalized phosphatidylinositides on apoptotic cells are eat-me signals recognized by CD14. Cell Death Differ. (2022) 29:1423–32. doi: 10.1038/s41418-022-00931-2
220. Lee SY, Hwang HJ, Ku B, Lee DW. Cell proliferation receptor-enhanced 3D high-throughput screening model for optimized drug efficacy evaluation in breast cancer cells. Anal Chem. (2022) 94:11838–47. doi: 10.1021/acs.analchem.2c02222
221. Lim HM, Park SH. Regulation of reactive oxygen species by phytochemicals for the management of cancer and diabetes. Crit Rev Food Sci Nutr. (2023) 63:5911–36. doi: 10.1080/10408398.2022.2025574
222. Longevity O. Retracted: acetylshikonin, A novel CYP2J2 inhibitor, induces apoptosis in RCC cells via FOXO3 activation and ROS elevation. Oxid Med Cell Longev. (2024) 2024:9846960. doi: 10.1155/2024/9846960
223. Nam KH, Lee JH, Chung YS, Chun YS, Park HK, Kim YY. The efficacy of oxidized regenerated cellulose (SurgiGuard(R)) in breast cancer patients who undergo total mastectomy with node surgery: A prospective randomized study in 94 patients. PloS One. (2022) 17:e0267694. doi: 10.1371/journal.pone.0267694[
224. Nguyen Cao TG, Kang JH, Kim W, Lim J, Kang SJ, You JY, et al. Engineered extracellular vesicle-based sonotheranostics for dual stimuli-sensitive drug release and photoacoustic imaging-guided chemo-sonodynamic cancer therapy. Theranostics. (2022) 12:1247–66. doi: 10.7150/thno.65516
225. Noh H, Ahn S, Nam S, Jang Y, Chun Y, Park H, et al. Comparison of diagnostic performance and confidence between contrast-enhanced computed tomography scan and non-contrast-enhanced computed tomography plus abdomen ultrasound for hepatic metastasis in patients with breast cancer. J Med Ultrasound. (2022) 30:116–24. doi: 10.4103/JMU.JMU_58_21
226. Vulli A, Srinivasu PN, Sashank MSK, Shafi J, Choi J, Ijaz MF. Fine-tuned denseNet-169 for breast cancer metastasis prediction using fastAI and 1-cycle policy. Sensors (Basel). (2022) 22. doi: 10.3390/s22082988
227. Yadav N. Pyrimethamine induces phototoxicity in human keratinocytes via lysosomal and mitochondrial dependent signaling pathways under environmental UVA and UVB exposure. Toxicology. (2022) 479:153320. doi: 10.1016/j.tox.2022.153320
228. Yadav N, Tripathi AK, Parveen A, Parveen S, Banerjee M. PLGA-quercetin nano-formulation inhibits cancer progression via mitochondrial dependent caspase-3,7 and independent foxO1 activation with concomitant PI3K/AKT suppression. Pharmaceutics. (2022) 14. doi: 10.3390/pharmaceutics14071326
229. Bae KR, So WY, Jang S. Effects of a post-traumatic growth program on young korean breast cancer survivors. Healthcare (Basel). (2023) 11. doi: 10.3390/healthcare11010140
230. Bhakar S, Sinwar D, Pradhan N, Dhaka VS, Cherrez-Ojeda I, Parveen A, et al. Computational intelligence-based disease severity identification: A review of multidisciplinary domains. Diagnostics (Basel). (2023) 13. doi: 10.3390/diagnostics13071212
231. Choi Y, Park S, Lee S, Shin H-E, Kwon S, Choi J-K, et al. Cremastranone-derived homoisoflavanes suppress the growth of breast cancer cells via cell cycle arrest and caspase-independent cell death. Biomol Ther (Seoul). (2023) 31:526–35. doi: 10.4062/biomolther.2023.057
232. Choi YE, Sung K, Dong KS, Shin HB, Kim HJ, Lim YK. The effect of respiratory motion in breast intensity-modulated radiation therapy: 3D-printed dynamic phantom study. Anticancer Res. (2023) 43:4425–33. doi: 10.21873/anticanres.16638
233. De A, Roychowdhury P, Bhuyan NR, Ko YT, Singh SK, Dua K, et al. Folic acid functionalized diallyl trisulfide-solid lipid nanoparticles for targeting triple negative breast cancer. Molecules. (2023) 28. doi: 10.3390/molecules28031393
234. De A, Wadhwani A, Sauraj, Roychowdhury P, Kang JH, Ko YT, et al. WZB117 decorated metformin-carboxymethyl chitosan nanoparticles for targeting breast cancer metabolism. Polymers (Basel). (2023) 15. doi: 10.3390/polym15040976
235. He MT, Nguyen QN, Cho EJ, Kim SH, Park S, Park JY, et al. Aloe-emodin isolated from rheum undulatum L. Regulates cell cycle distribution and cellular senescence in human prostate cancer LNCaP cells. J Diet Suppl. (2024) 21:389–407. doi: 10.1080/19390211.2023.2284985
236. Jeong KY, Park SY, Park MH, Kim HM. Suppressing src-mediated EGFR signaling by sustained calcium supply targeting triple-negative breast cancer. Int J Mol Sci. (2023) 24. doi: 10.3390/ijms241713291
237. Jun HR, Kang HJ, Ju SH, Kim JE, Jeon SY, Ku B, et al. High-throughput organo-on-pillar (high-TOP) array system for three-dimensional ex vivo drug testing. Biomaterials. (2023) 296:122087. doi: 10.1016/j.biomaterials.2023.122087
238. Kim Y, Park EY, Lee H. The effect of myofascial release in patients with breast cancer-related lymphedema: a cross-over randomized controlled trial. Eur J Phys Rehabil Med. (2023) 59:85–93. doi: 10.23736/S1973-9087.22.07698-5
239. Lee D, Hong JH. Modulation of lysosomal cl(-) mediates migration and apoptosis through the TRPML1 as a lysosomal cl(-) sensor. Cells. (2023) 12. doi: 10.3390/cells12141835
240. Lee SY, Hwang HJ, Ku B, Lee DW. Correction to cell proliferation receptor-enhanced 3D high-throughput screening model for optimized drug efficacy evaluation in breast cancer cells. Anal Chem. (2023) 95:6476. doi: 10.1021/acs.analchem.3c00706
241. Lee SY, Koo IS, Hwang HJ, Lee DW. In Vitro three-dimensional (3D) cell culture tools for spheroid and organoid models. SLAS Discovery. (2023) 28:119–37. doi: 10.1016/j.slasd.2023.03.006
242. Lee Y, Moon S, Seok JY, Lee JH, Nam S, Chung YS. Characterization of the genomic alterations in poorly differentiated thyroid cancer. Sci Rep. (2023) 13:19154. doi: 10.1038/s41598-023-46466-5
243. Na K, Oh BC, Jung Y. Multifaceted role of CD14 in innate immunity and tissue homeostasis. Cytokine Growth Factor Rev. (2023) 74:100–7. doi: 10.1016/j.cytogfr.2023.08.008
244. Park E, Choi H, Truong CS, Jun HS. The inhibition of autophagy and pyroptosis by an ethanol extract of nelumbo nucifera leaf contributes to the amelioration of dexamethasone-induced muscle atrophy. Nutrients. (2023) 15. doi: 10.3390/nu15040804
245. Park EY, Song MK, Baek SY. Analysis of perceptions, preferences, and participation intention of urban forest healing program among cancer survivors. Int J Environ Res Public Health. (2023) 20. doi: 10.3390/ijerph20021604
246. Sharma H, Kim DY, Shim KH, Sharma N, An SSA. Multi-Targeting Neuroprotective Effects of Syzygium aromaticum Bud Extracts and Their Key Phytocompounds against Neurodegenerative Diseases. Int J Mol Sci. (2023) 24. doi: 10.3390/ijms24098148
247. Varaganti P, Buddolla V, Lakshmi BA, Kim YJ. Recent advances in using folate receptor 1 (FOLR1) for cancer diagnosis and treatment, with an emphasis on cancers that affect women. Life Sci. (2023) 326:121802. doi: 10.1016/j.lfs.2023.121802
248. Darlami O, Pun R, Ahn SH, Kim SH, Shin D. Macrocyclization strategy for improving candidate profiles in medicinal chemistry. Eur J Med Chem. (2024) 272:116501. doi: 10.1016/j.ejmech.2024.116501
249. Lee D, Lee SY, Ra M-J, Jung S-M, Yu J-N, Kang KS, et al. Cancer therapeutic potential of hovetrichoside C from Jatropha podagrica on apoptosis of MDA-MB-231 human breast cancer cells. Food Chem Toxicol. 2024:114794. doi: 10.1016/j.fct.2024.114794
Keywords: triple negative breast cancer, immunotherapy, tumor microenvironment, drug resistance, nanomedicine
Citation: Singh DD, Haque S, Kim Y, Han I and Yadav DK (2024) Remodeling of tumour microenvironment: strategies to overcome therapeutic resistance and innovate immunoengineering in triple-negative breast cancer. Front. Immunol. 15:1455211. doi: 10.3389/fimmu.2024.1455211
Received: 26 June 2024; Accepted: 31 October 2024;
Published: 10 December 2024.
Edited by:
Umberto Malapelle, University of Naples Federico II, ItalyReviewed by:
Qingxin Mu, University of Washington, United StatesSugandh Kumar, University of California, San Francisco, United States
Nilesh Kumar Sharma, Dr. D. Y. Patil Biotechnology & Bioinformatics Institute, India
Giacomo Miserocchi, Scientific Institute of Romagna for the Study and Treatment of Tumors (IRCCS), Italy
Copyright © 2024 Singh, Haque, Kim, Han and Yadav. This is an open-access article distributed under the terms of the Creative Commons Attribution License (CC BY). The use, distribution or reproduction in other forums is permitted, provided the original author(s) and the copyright owner(s) are credited and that the original publication in this journal is cited, in accordance with accepted academic practice. No use, distribution or reproduction is permitted which does not comply with these terms.
*Correspondence: Dharmendra Kumar Yadav, ZGhhcm1lbmRyYTMwb2N0QGdtYWlsLmNvbQ==; Ihn Han, aGFuaWhuQGt3LmFjLmty