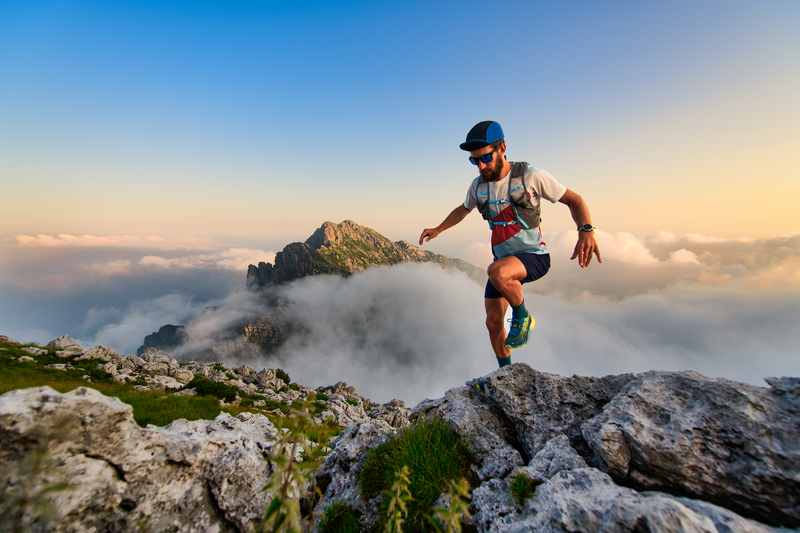
94% of researchers rate our articles as excellent or good
Learn more about the work of our research integrity team to safeguard the quality of each article we publish.
Find out more
PERSPECTIVE article
Front. Immunol. , 24 June 2024
Sec. Cytokines and Soluble Mediators in Immunity
Volume 15 - 2024 | https://doi.org/10.3389/fimmu.2024.1406886
This article is part of the Research Topic SOCS: Regulation of the Immune System at a Whole New Level View all 10 articles
Protein-protein interactions (PPIs) play critical roles in a wide range of biological processes including the dysregulation of cellular pathways leading to the loss of cell function, which in turn leads to diseases. The dysfunction of several signaling pathways is linked to the insurgence of pathological processes such as inflammation, cancer development and neurodegeneration. Thus, there is an urgent need for novel chemical modulators of dysregulated PPIs to drive progress in targeted therapies. Several PPIs have been targeted by bioactive compounds, and, often, to properly cover interacting protein regions and improve the biological activities of modulators, a particular focus concerns the employment of macrocycles as proteomimetics. Indeed, for their physicochemical properties, they occupy an intermediate space between small organic molecules and macromolecular proteins and are prominent in the drug discovery process. Peptide macrocycles can modulate fundamental biological mechanisms and here we will focus on peptidomimetics active on the Janus kinase/signal transducers and activators of transcription (JAK-STAT) pathways.
The JAK-STAT pathway is pivotal in transmitting signals initiated by cytokines, including interleukins (ILs), during immune responses, inflammation, and cancer development (1, 2). Physiologically, cytokine stimulation leads to JAK-mediated phosphorylation of specific tyrosine residues on STAT proteins, located in SH2 domains. Phosphorylated STATs dimerize, translocate into the nucleus, and regulate gene expression, tuning inflammatory and immune-related genes (3, 4). The dysregulation of this pathway can occur through mutations in upstream oncogenes, cytokine receptors, JAK, or STAT proteins and are commonly associated with cancer progression: its selective upregulation in response to cytokines drives immune responses, inflammation, and carcinogenesis (5, 6). Hence, JAK-STAT is a key target for therapeutics (7) and understanding the endogenous regulatory mechanism of this pathway is essential for designing new drugs (8).
Small molecules inhibitors of JAK proteins (JAKi) have been FDA-approved as: tofacitinib (Pfizer) for nail psoriasis (9), ruxolitinib (Novartis) for primary myelofibrosis (PMF) (10), fedratinib (Celgene) for myelofibrosis (11), filgitinib (Galapagos) for rheumatoid arthritis (RA) (12), upadacitinib (AbbVie), baricitinib (Eli Lilly) and abrocitinib (Pfizer) for atopic dermatitis (AD) (13). Many studies concerning these drugs highlighted their off-target effects, particularly in patients defined as ‘at risk’ (i.e. ≥65 years), affected by cardiovascular problems, smokers or at high risk to develop cancer (14). To limit side effects, several attempts are made in the routes of administration and to potentiate their local effects. For example, JAKi for the treatment of asthma are inhaled (15) others for AD have been formulated to act topically, as delgocitinib (approved in Japan), which demonstrated more effective in the treatment of AD in pediatrics (16).
In addition, a regulatory feedback of this pathway includes the expression of Suppressor Of Cytokine Signaling (SOCS) proteins (17) which share a common mechanism of action (MOA) consisting in inhibiting JAK-STAT by competing with SH2 of STATs for the binding to JAKs, through their own SH2. In addition with this general MOA, SOCS1 and SOCS3 members contain a Kinase Inhibitor Region (KIR), which directly inhibits the kinase activity of JAKs acting as pseudo-substrate toward the kinase catalytic site (18). In addition these two proteins differently interact with JAK proteins: indeed from crystal structure studies while SOCS1 forms a binary complex with JAK1 (19), SOCS3 interacts simultaneously with JAK2 and the glycoprotein 130 (gp130) (20).
The downregulation of SOCS1 or SOCS3 has been observed in several diseases: SOCS1, for its role in interferons (IFNs) (21, 22) and interleukins (ILs) (23–25) signaling, in involved in inflammatory diseases as rheumatoid arthritis and psoriasis (26, 27) and atherosclerosis (28); while SOCS3 is involved in tumour development and its deficiency, relevant in triple-negative breast cancer (TNBC), is associated with a worse prognosis (29).
Recently, resurgence has taken place in developing proteomimetics for therapeutic intervention: from the design of peptides mimicking the functions of proteins involved in diseases to the improvements of their drug-like features thanks to innovative synthetic and formulative platforms (30, 31). Proteomimetics, often in macrocyclic format, occupy an intermediate space between small organic molecules and macromolecules and combine significant binding affinities and selectivity, synthetic accessibility, low immunoreactivity and toxicities (32–34). Macrocyclizations render peptides more stable with increased membrane permeability, and stability in cellular environments (32, 35, 36).
Few but important examples of bioactive proteomimetics have been reported for several proteins of the JAK-STAT network paving the way to novel therapeutics (37).
IL-6 is a pro-inflammatory cytokine capable to activate several JAKs. Its dysregulation is associated with autoinflammatory and autoimmune diseases sepsis, irritable bowel syndrome (IBS), atherosclerosis, thrombosis, rheumatoid arthritis, and type 1 diabetes (38–40); hence the identification of inhibitors represents an interesting strategy to regulate its pathway (8). In this context, the θ-defensins are natural 18-mer macrocyclic peptides found in certain primates, such as rhesus macaques, able to regulate the production of cytokines, including IL-6, against various microbes (41, 42). Structurally, θ-defensins consist of a pair of antiparallel β-sheets linked by three disulfide bonds arranged as a ladder along the sheets to form an extremely stable structure (Figure 1A). The Rhesus θ-defensin-1 (RTD-1) suppresses the release of pro-inflammatory cytokines, as TNF-α and IL-6 (43). The RTD-1 isoform regulates the release of soluble tumor necrosis factor (sTNF) by inhibiting TNF-α-converting enzyme (TACE), which is a zinc metalloprotease responsible for cytokine production through proteolysis or “shedding” (43, 44). Aberrant TACE activity leads to increased TNF-α levels in inflammatory diseases and cancer progression (45–47). The macrocyclic structure of RTD is crucial for TACE inhibition, conversely, its structural modifications, as the absence of lactam bond head-to-tail RTD-1 (Figure 1B), cause the loss of inhibition (43). Hence one potential application could be the development of synthetic macrocyclic analogues of θ-defensin to block IL-6 production and limit TNF-dependent pathways in inflammatory diseases.
Figure 1 Upper panel: mimetics of θ-defensins (A) RTD-1, (B) acyclic RTD-1. Lower panel: mimetics of IL-17 SynEp (C) LF1, (D) LF2 mimicking IL17F and (E) LA3 mimicking IL17A.
Within IL-17 family, there are six homodimers (A-F) and one heterodimer, A/F, known to interact with five receptors (RA-E) (48): in detail, IL-17A and F bind to the heterodimeric receptor complex formed by IL-17RA and RC (49). Different IL-17 proteins are linked to distinct biological activities i.e. IL-17A is associated with chronic obstructive pulmonary disease (COPD), while IL-17F in psoriasis and rheumatoid arthritis (50, 51).
To target these cytokines for therapeutic intervention selective drugs are required (52) and macrocyclic peptides mimicking IL-17A and F, have been reported. By using the in situ click screening method, unique epitopes were identified: Phe40-Ser70 for IL-17F and Ile27- Lys61 for IL-17A (53). From these, synthetic epitopes (SynEps) bearing clickable N-terminal tail were designed and analyzed and two of them, SynEp1 and SynEp2, resulted active against IL-17F while SynEp3 against IL-17A. Synthetic variants of SynEp compounds were generated through combinatorial approaches and screened obtaining macrocyclic binders highly specific (Figures 1C–E).
Activators of JAK/STAT can be used as antiviral: type I interferons (IFN-α/β) are known to inhibit viral infection (54, 55), but interferons-based therapies have several side effects hence the need for new antiviral drugs (56). In patients with end-stage dilated cardiomyopathy (DCM), gp130 and JAK-STAT signaling are altered (57), studies conducted in left ventricular (LV) myocardia pointed out reduced levels of JAK2 phosphorylation and gp130 (57). The restoration of JAK/STAT in DCM is critical to prevent cardiomyocytes apoptosis and stimulates the expression of cardioprotective genes such as superoxide dismutase and vascular endothelial growth factor (58–61). Erythropoietin (Epo) and Thrombopoietin (Tpo) proteins activate JAK-STAT similarly to other cytokines through the binding to the corresponding receptors, EpoR and TpoR (Figure 2A) (62). In this way, Epo regulates bone marrow erythropoiesis and Tpo platelet production (63, 64) hence, the development of Epo and Tpo mimetic peptides (EMP and TMP) is valuable in diseases as erythrocyte and platelet disorders (65).
Figure 2 Upper panel: activation of cytokine receptors by (A) their endogenous ligands and (B) STaMPtide mimetics; Lower panel: different cyclization of peptide sequences: (C) amide, (D) hydrocarbon and (E) disulphide and (F) by introduction of non-native scaffolds.
In general, an interesting approach to obtain cytokines mimetics is based on the design of Single-chain Tandem Macrocyclic Peptides (STaMPtides) which are constituted by two disulfide-cyclic peptides linked by peptide linkers (usually (Pro-Ala)n) (Figure 2B). Interestingly STaMPtides mimicking Epo and Tpo have been developed (66): two moieties of a mimetic of Epo, named EMP35 (67), were linked through 8- or 22-mer–Pro-Ala (PA) linkers, respectively. Both STaMPtides were able to activate the cascade EpoR/JAK2 and to induce the phosphorylation of JAK2 protein acting as strong mimetics of Epo (66). A similar approach was followed to design TMP-based STaMPtides: a mimetic of Tpo (68) was dimerized with an 8-mer PA linker to form TMP-PA8. Its activity was compared with that of recombinant human Tpo (rhTpo) using a phosphokinase array, in both cases the analysis of cell lysates revealed the activation of JAK2 protein (66).
An opposite therapeutic approach consists in engineering inhibitors of JAK-STAT pathway assuming as structural template natural endogenous regulators as SOCS proteins and hence the design and optimization of SOCS mimetics (69).
Concerning SOCS1, many studies demonstrated that the linear peptide covering the KIR domain inhibits/reduces: i) the activation of STAT by cytokines Th1 and 17 in leukocytes, ii) the activation and migration of vascular cells and macrophages in vitro (70), iii) the expression of cytokines with pro-inflammatory properties in atherosclerotic plaques (71), iv) the renal inflammation, oxidative stress and fibrosis (72), v) the chronic intraocular inflammatory disease (as uveitis) (73) (equine recurrent uveitis (ERU) (74, 75), vi) the abdominal aortic aneurysm (AAA) (76), and vii) the severity of skin lesions, autoantibody production and kidney disease in lupus-associated pathologies (77). In detail it demonstrated a protective role in glomerular changes in MsPGN rat models by reducing macrophage infiltration and inhibiting macrophage polarizing to the M1 phenotype (78). A SOCS1-KIR linear peptidomimetic, named PS5, was developed in our research group (79): in keratinocytes and explants of type-1 skin disorders demonstrated greater efficacy with respect to KIR (80)reduced the migration and proliferation (“wound healing”) of VSMCs with important antioxidant properties in vitro and in vivo (28). More recently, a lactame macrocyclization led to novel compounds named internal cyclic PS5 analogues (icPS5 and icPS5(Nal1), which bears the substitution Phe/Nal1, 1-Naphthyl-L-alanine) which inhibited JAK-mediated tyrosine phosphorylation of STAT1 and reduced cytokine-induced proinflammatory gene expression, oxidative stress generation and cell migration: in this context the Nal1 containing cycle exhibited long-time anti-migratory effects which are very important to limit plaque formation (81). More recently within icPS5 sequence, SAR investigations were carried out by performing crucial amino acids substitutions and/or modifications affecting the ring size: these studies confirmed the feasibility of this class of SOCS1 peptidomimetics, as specific inhibitors of JAK2 (82).
On the other hand, our research group was the first to develop SOCS3 peptidomimetics following a structure-based approach quite similar to that of SOCS1: a long peptide, called KIRESS, exhibited a good affinity for JAK2 and an efficient suppression of IL-22 signaling in keratinocytes, in athymic nude mice with squamous cell carcinoma (SCC) (83) as well as in primary tumour growth and pulmonary metastasis in triple negative breast cancer (TNBC) models (84). Similarly, into primary cultured cells, KIRESS reduced the Neural stem cells (NSCs) proliferation via blocking the cell cycle progression from the G0/G1 to S phase and attenuated astrocytic differentiation (85). In parallel, to explore different SOCS3 protein regions involved in JAK2 recognition, several chimeric peptides connecting non-contiguous protein regions, with a strongly aromatic fragment, were investigated: the derived mimetic, named KIRCONG chim, revealed able to recognize JAK2 exhibiting a low micromolar value of dissociation constant with good anti-inflammatory properties in VSMC and RAW264.7 macrophages (86). Its further development was limited by poor water solubility which has been recently overcame by the introduction of polyethylene glycol (PEG) moiety as spacer instead of the two β-Alanines of KIRCONG chim impressively suppressed NV (87).
With the aim to improve drug-like features of KIRCONG chim, we also investigated in the recent past (88) and currently (unpublished data) novel cyclic analogues bearing different chemical linkages among SOCS3 regions. In detail, head-to-tail macrocycles of KIRCONG chim endowed with, amide (Figure 2C), hydrocarbon (Figure 2D) and disulphide (Figure 2E) bonds demonstrated reduced affinity toward JAK2 and very limited water solubility (88). We are currently applying the so-called CLIPS (Chemical Linkage of Peptides on Scaffolds) strategy (89) in different local stretches of the KIRCONG chim sequence (Figure 2F). CLIPS is a versatile strategy and involves the cyclization of linear peptides via reaction of thiol-functionalities of the cysteines with a small rigid entity (unpublished data), but despite the easiness of cyclization, determines a reduction of water solubility with respect to its linear counterpart. Hence a fine tuning among affinity, stability and aqueous solubility should be taken into account in the development of novel SOCS mimetics.
The proteomimetic approaches have predominantly focused on the folding features of protein interacting regions at PPIs interfaces, sometimes evolving toward unnatural structures with unprecedented features, as helical foldamers (90). More recently, proteomimetics have been developed for their use as biomimetic agents, selective binders or catalysts with promising applications in chemical, biological, medical, and material fields. In this context the number of biocompatible reactions used for the construction of proteomimetics is continuously in growth, as well as computational design algorithms (91). The cyclization of small molecules, peptides and macromolecules is a fundamental strategy to design precise 3D shapes tailored to chemical function and a major challenge, in current drug development efforts, is the generation of macrocycles targeting PPIs. To address this issue many, innovative design and synthetic strategies are in development including combinatorial, to diversify macrocyclic scaffolds (33) and screening formats (36). The JAK/STAT signaling pathway is characterized by extensive crosstalk of its components and is an important case study: it is endowed with many PPIs where an individual protein engages specific interactions (69). Both the activation and inhibition of JAK/STAT by external agents, in different pathological contexts, demonstrated therapeutic values. Herein we reported several examples to illustrate the importance of proteomimetic approach to selectively regulate this immune response signaling: in it mimetics of cytokines, acting at different pathway levels, amplify signaling cascades, leading to robust cellular responses in cell growth and differentiation regeneration and tissue repair. Conversely mimetics of negative regulators as SOCS1 and 3 are currently demonstrating growing therapeutic interest in inflammatory-related disorders and cancer, respectively.
The original contributions presented in the study are included in the article/supplementary material. Further inquiries can be directed to the corresponding author.
AC: Conceptualization, Writing – review & editing. SL: Conceptualization, Writing – original draft. DM: Conceptualization, Writing – original draft, Funding acquisition, Writing – review & editing.
The author(s) declare financial support was received for the research, authorship, and/or publication of this article. This work was supported by Associazione Italiana per la Ricerca sul Cancro (AIRC) grant IG 2022, Rif. 27378 (DM).
The authors declare that the research was conducted in the absence of any commercial or financial relationships that could be construed as a potential conflict of interest.
All claims expressed in this article are solely those of the authors and do not necessarily represent those of their affiliated organizations, or those of the publisher, the editors and the reviewers. Any product that may be evaluated in this article, or claim that may be made by its manufacturer, is not guaranteed or endorsed by the publisher.
1. Bousoik E, Montazeri Aliabadi H. "Do we know jack" About JAK? A closer look at JAK/STAT signaling pathway. Front Oncol. (2018) 8:287. doi: 10.3389/fonc.2018.00287
2. Loh CY, Arya A, Naema AF, Wong WF, Sethi G, Looi CY. Signal transducer and activator of transcription (STATs) proteins in cancer and inflammation: functions and therapeutic implication. Front Oncol. (2019) 9:48. doi: 10.3389/fonc.2019.00048
3. O'Shea JJ, Schwartz DM, Villarino AV, Gadina M, McInnes IB, Laurence A. The JAK-STAT pathway: impact on human disease and therapeutic intervention. Annu Rev Med. (2015) 66:311–28. doi: 10.1146/annurev-med-051113-024537
4. Durham GA, Williams JJL, Nasim MT, Palmer TM. Targeting SOCS proteins to control JAK-STAT signalling in disease. Trends Pharmacol Sci. (2019) 40:298–308. doi: 10.1016/j.tips.2019.03.001
5. Li X, Zhang B, Yu K, Bao Z, Zhang W, Bai Y. Identifying cancer specific signaling pathways based on the dysregulation between genes. Comput Biol Chem. (2021) 95:107586. doi: 10.1016/j.compbiolchem.2021.107586
6. Xin P, Xu X, Deng C, Liu S, Wang Y, Zhou X, et al. The role of JAK/STAT signaling pathway and its inhibitors in diseases. Int Immunopharmacol. (2020) 80:106210. doi: 10.1016/j.intimp.2020.106210
7. Sabaawy HE, Ryan BM, Khiabanian H, Pine SR. JAK/STAT of all trades: linking inflammation with cancer development, tumor progression and therapy resistance. Carcinogenesis. (2021) 42:1411–9. doi: 10.1093/carcin/bgab075
8. Shawky AM, Almalki FA, Abdalla AN, Abdelazeem AH, Gouda AM. A comprehensive overview of globally approved JAK inhibitors. Pharmaceutics. (2022) 14:1001. doi: 10.3390/pharmaceutics14051001
9. Merola JF, Elewski B, Tatulych S, Lan S, Tallman A, Kaur M. Efficacy of tofacitinib for the treatment of nail psoriasis: two 52-week, randomized, controlled phase 3 studies in patients with moderate-to-severe plaque psoriasis. J Of Am Acad Of Dermatol. (2017) 77:79–87.e1. doi: 10.1016/j.jaad.2017.01.053
10. Greenfield G, McPherson S, Mills K, McMullin MF. The ruxolitinib effect: understanding how molecular pathogenesis and epigenetic dysregulation impact therapeutic efficacy in myeloproliferative neoplasms. J Trans Med. (2018) 16:360. doi: 10.1186/s12967-018-1729-7
12. Becciolini A, Di Donato E, Santilli D, Lucchini G, Mozzani F, Riva M, et al. Filgotinib as rheumatoid arthritis therapy. Drugs Today (Barcelona Spain. (2021) 1998) 57:543–50. doi: 10.1358/dot.2021.57.9.3317241
13. Wan H, Jia H, Xia T, Zhang D. Comparative efficacy and safety of abrocitinib, baricitinib, and upadacitinib for moderate-to-severe atopic dermatitis: A network meta-analysis. Dermatologic Ther. (2022) 35:e15636. doi: 10.1111/dth.15636
14. Ytterberg SR, Bhatt DL, Mikuls TR, Koch GG, Fleischmann R, Rivas JL, et al. Cardiovascular and cancer risk with tofacitinib in rheumatoid arthritis. New Engl J Med. (2022) 386:316–26. doi: 10.1056/NEJMoa2109927
15. Nilsson M, Rhedin M, Hendrickx R, Berglund S, Piras A, Blomgran P, et al. Characterization of selective and potent JAK1 inhibitors intended for the inhaled treatment of asthma. Drug design Dev Ther. (2023 )2022(16):2901–17. doi: 10.2147/DDDT.S354291
16. Nakagawa H, Nemoto O, Igarashi A, Saeki H, Kabashima K, Oda M, et al. Delgocitinib ointment in pediatric patients with atopic dermatitis: A phase 3, randomized, double-blind, vehicle-controlled study and a subsequent open-label, long-term study. J Am Acad Dermatol. (2021) 85:854–62. doi: 10.1016/j.jaad.2021.06.014
17. Philips RL, Wang Y, Cheon H, Kanno Y, Gadina M, Sartorelli V, et al. The JAK-STAT pathway at 30: Much learned, much more to do. Cell. (2022) 185:3857–76. doi: 10.1016/j.cell.2022.09.023
18. Hilton DJ, Richardson RT, Alexander WS, Viney EM, Willson TA, Sprigg NS, et al. Twenty proteins containing a C-terminal SOCS box form five structural classes. Proc Natl Acad Sci U.S.A. (1998) 95:114–9. doi: 10.1073/pnas.95.1.114
19. Liau NPD, Laktyushin A, Lucet IS, Murphy JM, Yao S, Whitlock E, et al. The molecular basis of JAK/STAT inhibition by SOCS1. Nat Commun. (2018) 9:1558. doi: 10.1038/s41467-018-04013-1
20. Kershaw NJ, Murphy JM, Liau NP, Varghese LN, Laktyushin A, Whitlock EL, et al. SOCS3 binds specific receptor-JAK complexes to control cytokine signaling by direct kinase inhibition. Nat Struct Mol Biol. (2013) 20:469–76. doi: 10.1038/nsmb.2519
21. Alexander WS, Starr R, Fenner JE, Scott CL, Handman E, Sprigg NS, et al. SOCS1 is a critical inhibitor of interferon gamma signaling and prevents the potentially fatal neonatal actions of this cytokine. Cell. (1999) 98:597–608. doi: 10.1016/S0092-8674(00)80047-1
22. Fenner JE, Starr R, Cornish AL, Zhang JG, Metcalf D, Schreiber RD, et al. Suppressor of cytokine signaling 1 regulates the immune response to infection by a unique inhibition of type I interferon activity. Nat Immunol. (2006) 7:33–9. doi: 10.1038/ni1287
23. Davey GM, Starr R, Cornish AL, Burghardt JT, Alexander WS, Carbone FR, et al. SOCS-1 regulates IL-15-driven homeostatic proliferation of antigen-naive CD8 T cells, limiting their autoimmune potential. J Exp Med. (2005) 202:1099–108. doi: 10.1084/jem.20050003
24. Eyles JL, Metcalf D, Grusby MJ, Hilton DJ, Starr R. Negative regulation of interleukin-12 signaling by suppressor of cytokine signaling-1. J Biol Chem. (2002) 277:43735–40. doi: 10.1074/jbc.M208586200
25. Tamiya T, Kashiwagi I, Takahashi R, Yasukawa H, Yoshimura A. Suppressors of cytokine signaling (SOCS) proteins and JAK/STAT pathways regulation of T-cell inflammation by SOCS1 and SOCS3. Arterioscl Throm Vas. (2011) 31:980–5. doi: 10.1161/ATVBAHA.110.207464
26. Sharma J, Larkin J. 3rd, therapeutic implication of SOCS1 modulation in the treatment of autoimmunity and cancer. Front Pharmacol. (2019) 10:324. doi: 10.3389/fphar.2019.00324
27. Lamana A, Villares R, Seoane IV, Andres N, Lucas P, Emery P, et al. Identification of a human SOCS1 polymorphism that predicts rheumatoid arthritis severity. Front Immunol. (2020) 11:1336. doi: 10.3389/fimmu.2020.01336
28. La Manna S, Lopez-Sanz L, Bernal S, Jimenez-Castilla L, Prieto I, Morelli G, et al. Antioxidant effects of PS5, a peptidomimetic of suppressor of cytokine signaling 1, in experimental atherosclerosis. Antioxidants (Basel). (2020) 9:754. doi: 10.3390/antiox9080754
29. Kim G, Ouzounova M, Quraishi AA, Davis A, Tawakkol N, Clouthier SG, et al. SOCS3-mediated regulation of inflammatory cytokines in PTEN and p53 inactivated triple negative breast cancer model. Oncogene. (2015) 34:671–80. doi: 10.1038/onc.2014.4
30. Recio C, Maione F, Iqbal AJ, Mascolo N, De Feo V. The potential therapeutic application of peptides and peptidomimetics in cardiovascular disease. Front Pharmacol. (2017) 7:526. doi: 10.3389/fphar.2016.00526
31. Scognamiglio PL, Di Natale C, Perretta G, Marasco D. From peptides to small molecules: an intriguing but intricated way to new drugs. Curr Med Chem. (2013) 20:3803–17. doi: 10.2174/09298673113209990184
32. Frost JR, Scully CC, Yudin AK. Oxadiazole grafts in peptide macrocycles. Nat Chem. (2016) 8:1105–11. doi: 10.1038/nchem.2636
33. Habeshian S, Merz ML, Sangouard G, Mothukuri GK, Schuttel M, Bognar Z, et al. Synthesis and direct assay of large macrocycle diversities by combinatorial late-stage modification at picomole scale. Nat Commun. (2022) 13:3823. doi: 10.1038/s41467-022-31428-8
34. Bechtler C, Lamers C. Macrocyclization strategies for cyclic peptides and peptidomimetics. RSC Medicinal Chem. (2021) 12:1325–51. doi: 10.1039/D1MD00083G
35. Muttenthaler M, King GF, Adams DJ, Alewood PF. Trends in peptide drug discovery. Nat Rev Drug Discovery. (2021) 20:309–25. doi: 10.1038/s41573-020-00135-8
36. Merz ML, Habeshian S, Li B, David JGL, Nielsen AL, Ji X, et al. De novo development of small cyclic peptides that are orally bioavailable. Nat Chem Biol. (2023) 20(5):624–33. doi: 10.1038/s41589-023-01496-y
37. Abdulkadir S, Li C, Jiang W, Zhao X, Sang P, Wei L, et al. Modulating angiogenesis by proteomimetics of vascular endothelial growth factor. J Am Chem Soc. (2021) 144:270–81. doi: 10.1021/jacs.1c09571
38. Hirano T. Interleukin 6 in autoimmune and inflammatory diseases: a personal memoir. Proc Jpn Acad Ser B Phys Biol Sci. (2010) 86:717–30. doi: 10.2183/pjab.86.717
39. Villar-Fincheira P, Sanhueza-Olivares F, Norambuena-Soto I, Cancino-Arenas N, Hernandez-Vargas F, Troncoso R, et al. Role of interleukin-6 in vascular health and disease. Front Mol Biosci. (2021) 8:641734. doi: 10.3389/fmolb.2021.641734
40. Rehman K, Akash MSH, Liaqat A, Kamal S, Qadir MI, Rasul A. Role of interleukin-6 in development of insulin resistance and type 2 diabetes mellitus. Crit Rev Eukaryot Gene Expr. (2017) 27:229–36. doi: 10.1615/CritRevEukaryotGeneExpr.v27.i3
41. Lehrer RI, Cole AM, Selsted ME. theta-Defensins: cyclic peptides with endless potential. J Biol Chem. (2012) 287:27014–9. doi: 10.1074/jbc.R112.346098
42. Selsted ME. Theta-defensins: cyclic antimicrobial peptides produced by binary ligation of truncated alpha-defensins. Curr Protein Pept Sci. (2004) 5:365–71. doi: 10.2174/1389203043379459
43. Schaal JB, Maretzky T, Tran DQ, Tran PA, Tongaonkar P, Blobel CP, et al. Macrocyclic theta-defensins suppress tumor necrosis factor-alpha (TNF-alpha) shedding by inhibition of TNF-alpha-converting enzyme. J Biol Chem. (2018) 293:2725–34. doi: 10.1074/jbc.RA117.000793
44. Gooz M. ADAM-17: the enzyme that does it all. Crit Rev Biochem Mol Biol. (2010) 45:146–69. doi: 10.3109/10409231003628015
45. Issuree PD, Maretzky T, McIlwain DR, Monette S, Qing X, Lang PA, et al. iRHOM2 is a critical pathogenic mediator of inflammatory arthritis. J Clin Invest. (2013) 123:928–32. doi: 10.1172/JCI66168
46. Chalaris A, Adam N, Sina C, Rosenstiel P, Lehmann-Koch J, Schirmacher P, et al. Critical role of the disintegrin metalloprotease ADAM17 for intestinal inflammation and regeneration in mice. J Exp Med. (2010) 207:1617–24. doi: 10.1084/jem.20092366
47. Murphy G. The ADAMs: signalling scissors in the tumour microenvironment. Nat Rev Cancer. (2008) 8:929–41. doi: 10.1038/nrc2459
48. Kolls JK, Linden A. Interleukin-17 family members and inflammation. Immunity. (2004) 21:467–76. doi: 10.1016/j.immuni.2004.08.018
49. Hu Y, Ota N, Peng I, Refino CJ, Danilenko DM, Caplazi P, et al. IL-17RC is required for IL-17A- and IL-17F-dependent signaling and the pathogenesis of experimental autoimmune encephalomyelitis. J Immunol. (2010) 184:4307–16. doi: 10.4049/jimmunol.0903614
50. Miossec P, Kolls JK. Targeting IL-17 and TH17 cells in chronic inflammation. Nat Rev Drug Discovery. (2012) 11:763–76. doi: 10.1038/nrd3794
51. Eustace A, Smyth LJC, Mitchell L, Williamson K, Plumb J, Singh D. Identification of cells expressing IL-17A and IL-17F in the lungs of patients with COPD. Chest. (2011) 139:1089–100. doi: 10.1378/chest.10-0779
52. Donnelly RP, Young HA, Rosenberg AS. An overview of cytokines and cytokine antagonists as therapeutic agents. Ann N Y Acad Sci. (2009) 1182:1–13. doi: 10.1111/j.1749-6632.2009.05382.x
53. Lai BT, Wilson JA, Malette Loredo J, Pitram SM, LaBerge NA, Heath JR, et al. Epitope-targeted macrocyclic peptide ligand with picomolar cooperative binding to interleukin-17F. Chemistry. (2018) 24:3760–7. doi: 10.1002/chem.201704752
54. Grandvaux N, tenOever BR, Servant MJ, Hiscott J. The interferon antiviral response: from viral invasion to evasion. Curr Opin Infect Dis. (2002) 15:259–67. doi: 10.1097/00001432-200206000-00008
55. Ivashkiv LB, Donlin LT. Regulation of type I interferon responses. Nat Rev Immunol. (2014) 14:36–49. doi: 10.1038/nri3581
56. Tai ZF, Zhang GL, Wang F. Identification of small molecule activators of the janus kinase/signal transducer and activator of transcription pathway using a cell-based screen. Biol Pharm Bull. (2012) 35:65–71. doi: 10.1248/bpb.35.65
57. Podewski EK, Hilfiker-Kleiner D, Hilfiker A, Morawietz H, Lichtenberg A, Wollert KC, et al. Alterations in Janus kinase (JAK)-signal transducers and activators of transcription (STAT) signaling in patients with end-stage dilated cardiomyopathy. Circulation. (2003) 107:798–802. doi: 10.1161/01.CIR.0000057545.82749.FF
58. Funamoto M, Fujio Y, Kunisada K, Negoro S, Tone E, Osugi T, et al. Signal transducer and activator of transcription 3 is required for glycoprotein 130-mediated induction of vascular endothelial growth factor in cardiac myocytes. J Biol Chem. (2000) 275:10561–6. doi: 10.1074/jbc.275.14.10561
59. Negoro S, Kunisada K, Fujio Y, Funamoto M, Darville MI, Eizirik DL, et al. Activation of signal transducer and activator of transcription 3 protects cardiomyocytes from hypoxia/reoxygenation-induced oxidative stress through the upregulation of manganese superoxide dismutase. Circulation. (2001) 104:979–81. doi: 10.1161/hc3401.095947
60. Sheng Z, Knowlton K, Chen J, Hoshijima M, Brown JH, Chien KR. Cardiotrophin 1 (CT-1) inhibition of cardiac myocyte apoptosis via a mitogen-activated protein kinase-dependent pathway. Divergence from downstream CT-1 signals for myocardial cell hypertrophy. J Biol Chem. (1997) 272:5783–91. doi: 10.1074/jbc.272.9.5783
61. Hirota H, Chen J, Betz UA, Rajewsky K, Gu Y, Ross J Jr., et al. Loss of a gp130 cardiac muscle cell survival pathway is a critical event in the onset of heart failure during biomechanical stress. Cell. (1999) 97:189–98. doi: 10.1016/S0092-8674(00)80729-1
62. Won HH, Park I, Lee E, Kim JW, Lee D. Comparative analysis of the JAK/STAT signaling through erythropoietin receptor and thrombopoietin receptor using a systems approach. BMC Bioinf. (2009) 10 Suppl 1:S53. doi: 10.1186/1471-2105-10-S1-S53
63. Suresh S, Rajvanshi PK, Noguchi CT. The many facets of erythropoietin physiologic and metabolic response. Front Physiol. (2019) 10:1534. doi: 10.3389/fphys.2019.01534
64. Kuter DJ. The biology of thrombopoietin and thrombopoietin receptor agonists. Int J Hematol. (2013) 98:10–23. doi: 10.1007/s12185-013-1382-0
65. Gutti U, Pasupuleti SR, Sahu I, Kotipalli A, Undi RB, Kandi R, et al. Erythropoietin and thrombopoietin mimetics: Natural alternatives to erythrocyte and platelet disorders. Crit Rev Oncol Hematol. (2016) 108:175–86. doi: 10.1016/j.critrevonc.2016.11.002
66. Ito K, Matsuda Y, Mine A, Shikida N, Takahashi K, Miyairi K, et al. Single-chain tandem macrocyclic peptides as a scaffold for growth factor and cytokine mimetics. Commun Biol. (2022) 5:56. doi: 10.1038/s42003-022-03015-6
67. Johnson DL, Farrell FX, Barbone FP, McMahon FJ, Tullai J, Kroon D, et al. Amino-terminal dimerization of an erythropoietin mimetic peptide results in increased erythropoietic activity. Chem Biol. (1997) 4:939–50. doi: 10.1016/S1074-5521(97)90302-1
68. Dower WJ, Cwirla SE, Balasubramanian P, Schatz PJ, Baccanari DP, Barrett RW. Peptide agonists of the thrombopoietin receptor. Stem Cells. (1998) 16:21–9. doi: 10.1002/stem.v16:1+
69. Hu X, Li J, Fu M, Zhao X, Wang W. The JAK/STAT signaling pathway: from bench to clinic. Signal transduction targeted Ther. (2021) 6:402. doi: 10.1038/s41392-021-00791-1
70. Ahmed CM, Larkin J 3rd, Johnson HM. SOCS1 mimetics and antagonists: A complementary approach to positive and negative regulation of immune function. Front Immunol. (2015) 6:183. doi: 10.3389/fimmu.2015.00183
71. Recio C, Oguiza A, Lazaro I, Mallavia B, Egido J, Gomez-Guerrero C. Suppressor of cytokine signaling 1-derived peptide inhibits Janus kinase/signal transducers and activators of transcription pathway and improves inflammation and atherosclerosis in diabetic mice. Arterioscler Thromb Vasc Biol. (2014) 34:1953–60. doi: 10.1161/ATVBAHA.114.304144
72. Lopez-Sanz L, Bernal S, Recio C, Lazaro I, Oguiza A, Melgar A, et al. SOCS1-targeted therapy ameliorates renal and vascular oxidative stress in diabetes via STAT1 and PI3K inhibition. Lab Invest. (2018) 98:1276–90. doi: 10.1038/s41374-018-0043-6
73. He C, Yu CR, Mattapallil MJ, Sun L, Larkin Iii J, Egwuagu CE. SOCS1 mimetic peptide suppresses chronic intraocular inflammatory disease (Uveitis). Mediators Inflammation. (2016) 2016:2939370. doi: 10.1155/2016/2939370
74. Larkin J, Polk T, Sharma J, Bae S, Barr O, Jones A, et al. Mitigation of Equine Recurrent Uveitis through topical Suppressor of Cytokine signaling-1 (SOCS1) mimetic peptide: Open Label Safety and Efficacy Pilot study. Am Assoc Immnol. (2020) 204(Suppl 1):92.43. doi: 10.4049/jimmunol.204.Supp.92.43
75. Plummer CE, Polk T, Sharma J, Bae SS, Barr O, Jones A, et al. Open label safety and efficacy pilot to study mitigation of equine recurrent uveitis through topical suppressor of cytokine signaling-1 mimetic peptide. Sci Rep. (2022) 12:7177. doi: 10.1038/s41598-022-11338-x
76. Bernal S, Lopez-Sanz L, Jimenez-Castilla L, Prieto I, Melgar A, La Manna S, et al. Protective effect of suppressor of cytokine signalling 1-based therapy in experimental abdominal aortic aneurysm. Br J Pharmacol. (2020) 178(3):564–81. doi: 10.1111/bph.15330
77. Sharma J, Collins T, Lam B, Cain A, Roach TR, Cornaby C, et al. SOCS1 mimetics attenuate autoimmune pathology in a murine model of lupus. Am Assoc Immnol. (2021) 206(Suppl 1):66.02. doi: 10.4049/jimmunol.206.Supp.66.02
78. Zhao Y, Peng F, He J, Qu Y, Ni H, Wu L, et al. SOCS1 peptidomimetic alleviates glomerular inflammation in msPGN by inhibiting macrophage M1 polarization. Inflammation. (2023) 46:2402–14. doi: 10.1007/s10753-023-01886-3
79. Doti N, Scognamiglio PL, Madonna S, Scarponi C, Ruvo M, Perretta G, et al. New mimetic peptides of the kinase-inhibitory region (KIR) of SOCS1 through focused peptide libraries. Biochem J. (2012) 443:231–40. doi: 10.1042/BJ20111647
80. Madonna S, Scarponi C, Doti N, Carbone T, Cavani A, Scognamiglio PL, et al. Therapeutical potential of a peptide mimicking the SOCS1 kinase inhibitory region in skin immune responses. Eur J Immunol. (2013) 43:1883–95. doi: 10.1002/eji.201343370
81. La Manna S, Lopez-Sanz L, Bernal S, Fortuna S, Mercurio FA, Leone M, et al. Cyclic mimetics of kinase-inhibitory region of Suppressors of Cytokine Signaling 1: Progress toward novel anti-inflammatory therapeutics. Eur J Med Chem. (2021) 221:113547. doi: 10.1016/j.ejmech.2021.113547
82. La Manna S, Fortuna S, Leone M, Mercurio FA, Di Donato I, Bellavita R, et al. Ad-hoc modifications of cyclic mimetics of SOCS1 protein: Structural and functional insights. Eur J Medicinal Chem. (2022) 243:114781. doi: 10.1016/j.ejmech.2022.114781
83. Madonna S, Scarponi C, Morelli M, Sestito R, Scognamiglio PL, Marasco D, et al. SOCS3 inhibits the pathological effects of IL-22 in non-melanoma skin tumor-derived keratinocytes. Oncotarget. (2017) 8:24652–67. doi: 10.18632/oncotarget.v8i15
84. La Manna S, Lee E, Ouzounova M, Di Natale C, Novellino E, Merlino A, et al. Mimetics of suppressor of cytokine signaling 3: Novel potential therapeutics in triple breast cancer. Int J Cancer. (2018) 143:2177–86. doi: 10.1002/ijc.31594
85. An J, Tan RL, Hu XX, Cai ZL, Sun MQ, Ge Q, et al. Kinase inhibit region of SOCS3 attenuates IL6-induced proliferation and astrocytic differentiation of neural stem cells via cross talk between signaling pathways. CNS Neurosci Ther. (2023) 29:168–80. doi: 10.1111/cns.13992
86. La Manna S, Lopez-Sanz L, Mercurio FA, Fortuna S, Leone M, Gomez-Guerrero C, et al. Chimeric peptidomimetics of SOCS 3 able to interact with JAK2 as anti-inflammatory compounds. ACS medicinal Chem Lett. (2020) 11:615–23. doi: 10.1021/acsmedchemlett.9b00664
87. Wang T, Kaneko S, Kriukov E, Alvarez D, Lam E, Wang Y, et al. SOCS3 regulates pathological retinal angiogenesis through modulating SPP1 expression in microglia and macrophages. Mol Ther. (2024) 32(5):1425–44. doi: 10.1016/j.ymthe.2024.03.025
88. La Manna S, Leone M, Mercurio FA, Florio D, Marasco D. Structure-activity relationship investigations of novel constrained chimeric peptidomimetics of SOCS3 protein targeting JAK2. Pharmaceuticals. (2022) 15:458. doi: 10.3390/ph15040458
89. Streefkerk DE, Schmidt M, Ippel JH, Hackeng TM, Nuijens T, Timmerman P, et al. Synthesis of constrained tetracyclic peptides by consecutive CEPS, CLIPS, and oxime ligation. Organic Lett. (2019) 21:2095–100. doi: 10.1021/acs.orglett.9b00378
90. Sang P, Cai J. Unnatural helical peptidic foldamers as protein segment mimics. Chem Soc Rev. (2023) 52(15):4843–77. doi: 10.1039/D2CS00395C
Keywords: jak-stat, peptidomimetics, macrocycles, inflammation, cytokines
Citation: Cugudda A, La Manna S and Marasco D (2024) Are peptidomimetics the compounds of choice for developing new modulators of the JAK-STAT pathway? Front. Immunol. 15:1406886. doi: 10.3389/fimmu.2024.1406886
Received: 25 March 2024; Accepted: 12 June 2024;
Published: 24 June 2024.
Edited by:
Flavia Bazzoni, University of Verona, ItalyReviewed by:
Mark D. Turner, Nottingham Trent University, United KingdomCopyright © 2024 Cugudda, La Manna and Marasco. This is an open-access article distributed under the terms of the Creative Commons Attribution License (CC BY). The use, distribution or reproduction in other forums is permitted, provided the original author(s) and the copyright owner(s) are credited and that the original publication in this journal is cited, in accordance with accepted academic practice. No use, distribution or reproduction is permitted which does not comply with these terms.
*Correspondence: Daniela Marasco, daniela.marasco@unina.it
Disclaimer: All claims expressed in this article are solely those of the authors and do not necessarily represent those of their affiliated organizations, or those of the publisher, the editors and the reviewers. Any product that may be evaluated in this article or claim that may be made by its manufacturer is not guaranteed or endorsed by the publisher.
Research integrity at Frontiers
Learn more about the work of our research integrity team to safeguard the quality of each article we publish.