- 1Department of Applied Biology and Chemical Technology, The Hong Kong Polytechnic University, Hong Kong, Hong Kong SAR, China
- 2State Key Laboratory of Chemical Biology and Drug Discovery, The Hong Kong Polytechnic University, Hong Kong, Hong Kong SAR, China
Hepatocellular carcinoma (HCC) is one of the most prevalent malignancies worldwide and has a poor prognosis. Although immune checkpoint inhibitors have entered a new era of HCC treatment, their response rates are modest, which can be attributed to the immunosuppressive tumor microenvironment within HCC tumors. Accumulating evidence has shown that tumor growth is fueled by cancer stem cells (CSCs), which contribute to therapeutic resistance to the above treatments. Given that CSCs can regulate cellular and physical factors within the tumor niche by secreting various soluble factors in a paracrine manner, there have been increasing efforts toward understanding the roles of CSC-derived secretory factors in creating an immunosuppressive tumor microenvironment. In this review, we provide an update on how these secretory factors, including growth factors, cytokines, chemokines, and exosomes, contribute to the immunosuppressive TME, which leads to immune resistance. In addition, we present current therapeutic strategies targeting CSC-derived secretory factors and describe future perspectives. In summary, a better understanding of CSC biology in the TME provides a rational therapeutic basis for combination therapy with ICIs for effective HCC treatment.
1 Introduction to hepatocellular carcinoma
Cancer has long been a major public health concern, with consistently increasing incidence and mortality rates worldwide. According to GLOBOCAN estimation data for 2020, a total of 19 million new cancer cases and 10 million cancer-related deaths are predicted to occur globally (1). Primary liver cancer is the sixth most diagnosed cancer and the third leading cause of cancer-related deaths worldwide according to GLOBOCAN 2020 evaluations (1). Liver cancer has been established as one of the key contributors to the global cancer burden, and hepatocellular carcinoma (HCC) is one of the most commonly diagnosed histological subtypes of liver malignancy, accounting for approximately 80% of all liver cancer cases worldwide (2). Despite the significance of HCC cases in transitioning countries with low and medium resources, the tumorigenicity of HCC is significantly related to various risk factors and underlying liver disorders, including chronic hepatitis B virus (HBV) and hepatitis C virus (HCV) infections, nonalcoholic fatty liver disease (NAFLD), nonalcoholic steatohepatitis (NASH), compulsive alcohol abuse, smoking, aflatoxin exposure, metabolic syndrome, diabetes, and obesity. Approximately 75% of documented HCC cases are induced by HBV and HCV, rendering such infections crucial global risk factors for HCC (2). Furthermore, the contributions of both NAFLD and NASH to HCC progression are discernible in the evidence of common occurrences worldwide. Accumulating evidence has also demonstrated the causal linkage of risk factors, such as excessive alcohol and tobacco consumption, as well as underlying metabolic disorders, including obesity and diabetes, with an increased risk of HCC, including its precursors, NAFLD and NASH (3–6). HCC is a complex and heterogeneous malignancy known to have a poor prognosis, with the life prospects of individuals varying according to disease progression. More than 50% of HCC patients are diagnosed at an advanced stage of HCC development (7, 8). Individuals with advanced-stage HCC progression have an overall median survival rate of less than a year (8, 9), whereas those with early-stage HCC have a five-year median survival rate of approximately 70% with the corresponding treatment options (10).
2 Therapeutic options for hepatocellular carcinoma patients
The severity of HCC is acknowledged in both clinical and research settings, where various treatment modalities for this malignancy continue to be developed. The HCC staging systems, among which the Barcelona Clinical Liver Cancer (BCLC) is one of the renowned systems to be adopted, facilitate the selection of therapeutic options.
Liver transplantation is a highly effective treatment option for patients with early-stage HCC (BCLC 0/A) because it can remove tumor lesions and replace a damaged liver to prevent HCC recurrence (11–13). However, this treatment is recommended only for early-stage patients. Hepatic resection and ablation are other options that provide safe and effective outcomes for early-stage patients. Hepatic resection offers greater clinical benefits in terms of overall survival and tumor control but at a higher cost and with more adverse side effects than ablation techniques (14–16). Chemotherapeutic-based treatments, such as transarterial chemoembolization (TACE), are typically used for intermediate-stage HCC patients (BCLC B) with locally unresectable tumors.
The administration of tyrosine kinase inhibitors (TKIs) as a systemic therapy method is widely adopted to treat patients with HCC in both the intermediate and advanced stages (BCLC B/C). Sorafenib, an FDA-approved oral multikinase inhibitor, is capable of impeding HCC proliferation through the inhibition of numerous cell surface and downstream kinases that are involved in angiogenesis and tumor proliferation, including vascular endothelial growth factor receptor (VEGFR) 1–3, platelet-derived growth factor receptor (PDGFR) β, FMS-like tyrosine kinase-3 (FLT3), and c-KIT (17, 18). Additionally, the inhibition of Raf kinases, including B-Raf and C-Raf, which are implicated in the Ras/Raf/MEK/ERK signaling pathway, also inhibits HCC development. Similarly, another multikinase inhibitor, lenvatinib, was synthesized. This drug is a compelling inhibitor of fibroblast growth factor receptor (FGFR) 1–4, VEGFR 1–3, PDGFR-α, RET, and KIT. Unlike sorafenib, lenvatinib is capable of impeding the fibroblast growth factor (FGF) signaling pathway, which provides a basis for its antitumor activity (19–22).
Despite numerous therapeutic options, the clinical benefits for patients with HCC remain limited owing to adverse side effects, recurrence risk, and therapeutic resistance. TKIs such as sorafenib and lenvatinib are significant for advanced-stage HCC. However, primary and acquired resistance are major obstacles, and kinase inhibitors have become ineffective over time. Genetic diversity contributes to primary resistance, whereas acquired resistance can occur during treatment. A substantial body of research has identified crosstalk between multiple signaling pathways, including the PI3K/AKT, JAK/STAT, hypoxia-inducible, and epithelial–mesenchymal transition (EMT) pathways, as one of the primary sources of acquired resistance to sorafenib in HCC patients, along with other pathways (23–26). Similarly, some studies have shown that the signaling pathways that participate in acquired resistance to lenvatinib in HCC patients include the EGFR/PAK2/ERK5, PI3K/AKT, and MAPK/ERK pathway (27, 28). Ultimately, a wide range of additional molecular mechanisms contribute to the complex nature of therapeutic resistance in HCC patients.
Combination therapy that incorporates immunotherapies, including immune checkpoint inhibitors (ICIs) and chimeric antigen receptors (CARs), with conventional treatments, such as TKIs, locoregional and radical treatments, and neoadjuvant therapy, is ongoing. Immunotherapy, as a multifaceted treatment approach, has shown promising results in improving the treatment efficacy and clinical benefits for patients with liver cancer, especially HCC. However, investigating the molecular mechanisms leading to therapeutic resistance in patients with HCC is crucial for developing of effective treatments that provide substantial and consistent clinical benefits, especially for those in the advanced stages of the disease.
3 General concept of cancer stem cells
Tumor-initiating cells (TICs) or cancer stem cells (CSCs) are a subpopulation of malignant cells within the tumor bulk that can self-renew, differentiate, and form distinct tumor cell populations, leading to tumorigenesis, and are known to be involved in the development of therapeutic resistance in cancer patients. These cells are highly resistant to conventional chemotherapeutic therapies, rendering them resistant to apoptosis, able to survive and repopulate the tumor bulk with restricted proliferative potential, and metastasize to give rise to new tumors (29, 30). The intricate biological characteristics and mechanisms of these conspicuous cells further drive tumor aggressiveness and survival, which include but are not limited to metabolic alterations (31–33), redox stress tolerance (34, 35), prompt restoration of damaged DNA (36, 37), EMT signaling (38), hyperactivity and overexpression of ATP-binding cassette (ABC) transporters for drug efflux (39, 40), and the development of multidrug resistance (MDR) (40, 41).
The detection of CSCs is feasible due to the presence of intracellular and extracellular molecules that serve as markers, and these markers are also used for predictive, diagnostic, and therapeutic purposes. The adoption of combinations of markers may help to accurately isolate, enrich, and identify CSCs, as certain markers are shared by all types of malignant and nonmalignant cells (42). Transcription factors such as SOX2, OCT4, and NANOG, as well as surface markers such as CD24, CD34, CD44, CD90, CD123, and CD133, are commonly used to help distinguish CSC populations (43–45). For years, the presence of liver CSCs has been continuously investigated as one of the prominent mechanisms behind inefficient treatment outcomes and tumor relapse in liver cancer patients. Owing to extensive research performed throughout the years, numerous markers for isolating and characterizing CSCs in liver cancer have been discovered. Through evident xenotransplantation and fluorescence-activated cell sorting (FACS) experimental outputs, markers of CSCs in HCC can be classified into intracellular markers, such as cytokeratin 19 (CK19) and surface markers, including CD13, CD24, CD44, CD47, CD90, CD133, and epithelial cellular adhesion molecule (EpCAM) (46). These reported markers have been evaluated with regard to CSCs in HCC, which is known to be associated with poor patient survival and aggressive tumor development. However, the purpose of biomarkers is widely applied to characterize or label the CSC phenotype of various malignancies; for example, CD133 can be used as a CSC marker for liver, breast, lung, pancreatic, and prostate cancers (29). The combinatory use of several intracellular and extracellular markers has served as the foundation for the identification and isolation of CSC populations.
CK19 is a known intracellular marker for premature hepatoblasts, hepatic progenitor cells, and cholangiocytes, where it can serve as a biomarker for CSCs in HCC and is involved in the SMAD/transforming growth factor (TGF)-β signaling pathway (46, 47). Previous research has shown that CK19+ cells in HCC are associated with metastasis, drug resistance, poor prognosis, tumor differentiation, and tumor relapse (47–50). A research group reported that CK19 may be a predictive biomarker for tumor relapse following surgery and adjuvant therapy in patients with HBV-induced HCC (51). A similar study examined the association between the benefits of adjuvant TACE and CK19 expression and reported that CK19+ HCC patients with an elevated risk of tumor relapse may benefit from TACE in terms of recurrence-free survival (52). Moreover, it has been suggested that HCC patients with CK19+ cells could be treated with regorafenib, a dual-targeted VEGFR2-TIE2 TKI, for individualized therapeutic purposes (53).
The expression of CSC surface markers, including CD13, CD24, CD44, CD47, CD90, and CD133, is reported to be related to self-renewal, metastasis, proliferation, angiogenesis, poor prognosis, tumor recurrence, and drug resistance in HCC. These markers are also functional markers which regulate a number of signaling pathways (46, 54, 55). Recent studies have shown novel insights based on discoveries related to HCC cell surface markers, which are summarized in Table 1. Research groups have shown that CD13 expression is correlated with chemoresistance induction and sorafenib resistance through the p38/Hsp27/CREB/ATG7 and HDAC5/LSD1/NF-κB pathways, respectively (56, 57). Moreover, the suppression of CD13 expression by ubenimex, a CD13 inhibitor, may reverse chemoresistance in HCC, suggesting that combination therapy involving chemotherapeutic agents and ubenimex may be another treatment option to counter therapeutic resistance (58). Additionally, recent research demonstrated a novel method to impede CD24 expression through the use of RNAi technology that attenuated Salmonella and harnessed its ability to bind to oxaliplatin chemotherapeutic drugs (59). The application of this novel approach led to a decrease in stemness properties and an increase in T-cell infiltration, which increased antitumor immune responses. Moreover, a discovery revealed that CD24-related sorafenib resistance in patients with HCC is associated with the activation of autophagy, and the overexpression of CD24 leads to increased production of PP2A and promotes the downregulation of the mTOR/AKT pathway, which sustains autophagy (60). CD44 promotes HCC progression, metastasis, migration, and invasion through YAP (61), a downstream regulator of the Hippo pathway, the C-X-C motif chemokine receptor (CXCR) 4/Wnt/β-catenin pathway (62), and the CXCR4/AKT/ERK pathway (63). The obstruction of CD47 expression in a murine model has been shown to promote antitumor immunity through the CD103+ dendritic cell (DC)/natural killer (NK) cell axis (64). Moreover, a novel approach that incorporates a bispecific antibody that targets glypican-3 (GPC3) and CD47 has been shown to outperform combination therapy with anti-GPC3 and anti-CD47 in a xenograft HCC model (65). The involvement of CD90 in the biological functions of HCC remained unknown until a recent study revealed that CD90 participates in migrative, viability, and sphere-forming capabilities in HCC, indicating that CD90 may serve as a candidate for diagnostic and therapeutic approaches (66). Furthermore, a research group has targeted CD90 expression via miR-125a/b, which impedes tumor-associated macrophages (TAMs) mediated by CSCs in HCC (67). Recently, several research groups have made innovations in therapeutic strategies targeting CD133 for tumor development and preservation. Novel applications of therapeutic compounds, including chromenopyrimidinone (CPO), oxytetracycline, a CD133-specific aptamer conjugated with doxorubicin, and actinomycin D (ActD), were found to suppress the expression of CD133, which leads to a positive regulation of the stemness of HCC cells (68–71).
Aside from the expression of CSC markers in HCC, several intrinsic regulatory molecules have also been reported to play a role in tumor initiation and self-renewal capabilities in HCC (46). These molecules include long noncoding RNAs (lncRNAs), microRNAs (miRNAs), epigenetic regulators, kinases and phosphatases, metabolic regulators, transcription factors, and secretory molecules. Generally, these elements congregate into common renowned signaling pathways, including the MAPK, JAK/STAT, IL-6/STAT3, TGF-β, NF-κB, JNK, Sonic Hedgehog (SHH), Wnt/β-catenin, and Notch pathways. Ultimately, these signaling pathways are associated with the HCC tumor microenvironment (TME), which regulates CSCs, along with other elements, including cancer-associated fibroblasts (CAFs) and TAMs (54, 55). As reciprocal interactions between CSCs and their niches allow the formation of an immunosuppressive microenvironment, the preceding intrinsic regulators of CSC properties may also contribute. Several lncRNAs and miRNAs can exert immunosuppressive influence to facilitate HCC progression, such as lncMALAT1 (72), lncHULC (73), lncHOTAIR (74), lncLINC01132 (75), lncPVT1 (76), lncLINC00662 (77), lncβ-Catm (78), miR-23a-3p (79), and miR-146a-5p (80). Dysregulated expression of epigenetic regulators, including DNMT3α (81), KDM1A (82, 83), YTHDF2 (84), and RALYL (85), as well as kinases and phosphatases such as IRAK1 (86) and SHP2 (87), may also contribute to the formation of an immunosuppressive TME in HCC. As an intricate malignancy, HCC is characterized by metabolic reprogramming, in which glycolytic and lipid metabolism aberrations can contribute to an immunosuppressive TME. The implicated metabolic regulators include XOR (88), SCD1 (89), and ACLY (90). Finally, transcription factors involved in regulating cancer stemness in HCC, such as SALL4 (80) and FOXM1 (91), are known to exert immunosuppressive effects on the TME of HCC.
4 Concept of secretome and secretory factors within the TME
The secretome can be defined as a biological factor, either soluble or insoluble, that is secreted or released into the extracellular space by cells, tissues, organs, and organisms. The secretome consists of growth and coagulation factors, chemokines, cytokines, hormones, glycoproteins, miRNAs, and enzymes (92, 93). These molecular or biological factors are secreted through mechanisms involving constitutive and regulated secretory organelles at any given time. In healthy cells, a subtly regulated secretome is crucial for the maintenance of physiological tissue homeostasis and corresponds to a large proportion of synthesized proteins in secretory tissues, along with their associated receptors, which operate as the key mechanism in cell-to-tissue communication (92, 94). The dynamic composition of the secretome is usually based on the cell type and stimulus from the microenvironment, where it possesses therapeutic benefits that are valuable for repairing impaired tissue (94, 95). Numerous biologically active molecules within the secretome exert therapeutic benefits, ranging from anti-inflammatory and immunomodulation (e.g., interleukin (IL)-6, IL-8, hepatocyte growth factor (HGF), prostaglandin E2 (PGE2), TGF-β), antifibrotic (e.g., VEGF, HGF, TIMP-1, TIMP-4, FGF-2), antiapoptotic (VEGF, HGF, TGF-β, stanniocalcin (STC)-1, FGF) and proangiogenic effects (e.g., VEGF, HGF, FGF-2, IL-6, IL-8, TGF-β, monocyte chemoattractant protein (MCP)-1) (95). Similar to healthy tissues, the tumor secretome is composed of an abundant number of elements, including growth factors (e.g., TGF-β, VEGF, HGF), immunosuppressive cytokines (e.g., IL-4, IL-10, CCL22, CCL28, CXCL12, CCL5), and other components, such as enzymes [e.g., indoleamine 2,3-dioxygenase (IDO), cyclooxygenase-2 (COX-2)], glycoproteins [e.g., galectin (GAL)1, GAL3, alpha-fetoprotein (AFP)] and EVs (e.g., tumor-derived EVs carrying PGE2, TGF-β, adenosine, IDO, GALs) (92–94). These factors are important intermediaries of cell-to-cell interactions, where augmented patterns of secretion are associated with the regulation of cancer hallmarks such as metastasis, drug resistance, tumor development, and angiogenesis. There are various pathways and mechanisms that are implicated in maintaining the tumor secretome, including genetic mutations (e.g., c-Myc, p53, PTEN), the regulation of miRNAs, and the influence of the cellular microenvironment (e.g., hypoxic conditions) (96).
Normal adult stem cell tissues are primarily located within or adjacent to an in vivo microenvironment known as a “niche,” which comprises an assortment of cell types ranging from immune, endothelial, perivascular, and fibroblastic cells, along with the presence of extracellular matrix (ECM) components, growth factors, and cytokines. The interplay of components that exist in the niche maintains the stemness of stem cells, and the niche itself also facilitates the reception of extrinsic signals to induce stem cell performance (92). Similarly, within the TME, CSCs dwell and adapt to structurally distinct niches with components that favor CSC phenotypic properties of self-renewal and differentiation, as well as their metastatic potential and therapeutic resistance. CSCs may contribute to the secretion of various factors, either directly or by other means of crosstalk, recruitment, and stimulation of various cellular components, such as CAFs, MSCs, and immune cells. Ultimately, these secretory factors are components of the CSC niche. The reciprocal interactions between CSCs and such components contribute to the regulation of cancer hallmarks and alter the immune regulatory system, leading to the formation of an immunosuppressive TME. Previous studies have revealed that in HCC, the interplay of TME components with cancerous cells can induce liver fibrosis and promote HCC progression. Therefore, understanding how CSC-related secretory factors influence and help shape an immunosuppressive TME in HCC may ultimately benefit the development of effective and safe treatment options for HCC. Several studies have provided evidence related to immunosuppressive factors, ranging from cytokines, growth factors, and exosomes, which are secreted and/or mediated by CSCs and other components within the TME (Figure 1).
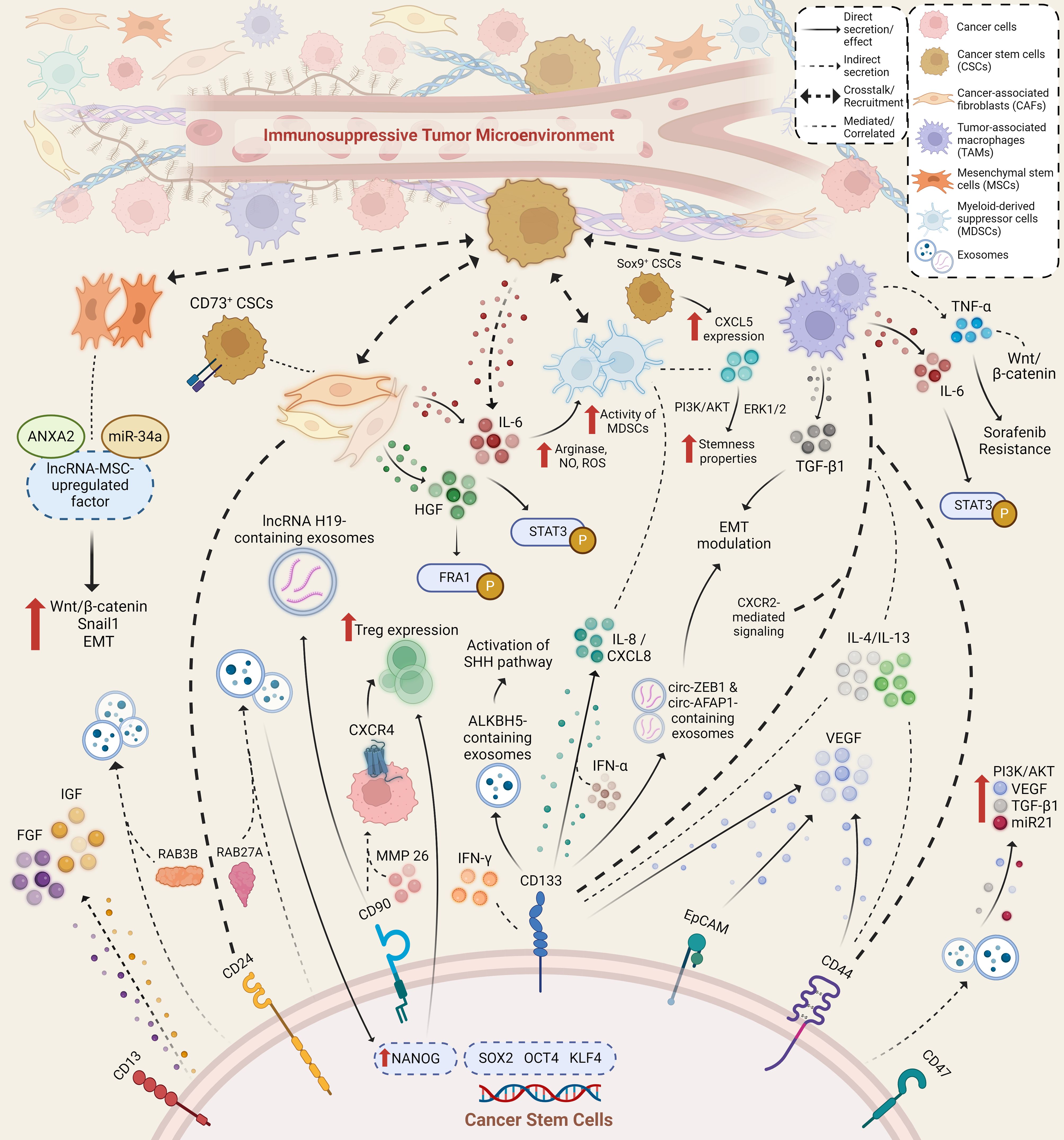
Figure 1 Immunosuppressive secretory factors that are secreted and/or mediated by cancer stem cells and other stromal and immune cells within the TME of HCC. Various cell types secrete and mediate the secretion of various secretory factors within the TME through direct and indirect method, where possible crosstalk and recruitment amongst secretory factors and cell types occur. Aside from exerting immunosuppressive capabilities, secretory factors also lead to an increase in stemness properties and hepatocarcinogenesis through activated signaling pathways and altered expression of various factors.
5 Effects of CSC-associated cytokines in shaping an immunosuppressive TME
Cytokines are a superfamily of proteins that are mainly produced by leukocytes during phases of naturally acquired immunity and have the ability to modulate inflammatory responses and the immune system. Cytokines are also involved in the positive and negative regulation of cellular activities, including growth, apoptosis, differentiation, and migration. These small glycoproteins are also secreted from different sources and may not regulate nonimmune-related events in various tissues. In the context of cancer, the function and mode of action of cytokines have been extensively studied in an attempt to exploit their antitumorigenic abilities for immunotherapeutic advancements. Cytokines normally operate by alerting the immune system to tissue injury and infection, although constant stimulation of cytokine production and secretion by immune cells may result in long-lasting inflammatory conditions and tumor development (97). Malignant cells are capable of secreting cytokines during cancer development to establish an immunosuppressive TME, where these cytokines act synergistically with other cell types to inhibit antitumorigenic responses and stimulate protumorigenic signals. Cytokines can be categorized as proinflammatory, anti-inflammatory, or immunosuppressive cytokines, the latter of which can impede the synthesis and control-related cellular activities of proinflammatory cytokines. However, owing to the pleiotropic actions of several cytokines, which promote an array of functional reactions from various cell subtypes, they are not categorized as either pro- or anti-inflammatory cytokines (98). The impact of cytokines on the development of cancer is influenced by several factors, including the concentration of cytokines, the tumor microenvironment (TME), the expression of receptors, and the balance between pro- and antitumorigenic cytokines. Different categories of cytokines, such as interleukins, chemokines, interferons (IFNs), and tumor necrosis factor (TNF), are considered in this discussion.
5.1 Interleukins
Interleukins, a subtype of cytokines, play an essential role in the activation and differentiation of immune cells and regulate growth and activation during immune responses and inflammation. In cancer, ILs play a role in fostering a conducive TME that promotes tumor development, self-renewal, survival, and immunosurveillance modulation. HCC is a malignancy closely associated with inflammation; however, the systemic interplay between ILs and HCC is still not well defined. Moreover, ILs play an essential role in facilitating crosstalk between CSCs and TME components. Certain ILs, such as IL-1, IL-6, and IL-8, are known to regulate the growth of CSC populations in various malignancies through bidirectional communication (99, 100).
The secretion of IL-6 by HCC cells with a chemoresistant phenotype, allegedly CSCs, has been reported to increase the development and activity of immunosuppressive MDSCs, in which high levels of immunosuppressive factors, namely, arginase, nitric oxide (NO), and reactive oxygen species (ROS), were observed (101). This finding highlights the pleiotropic nature of IL-6, which may contribute to the formation of an immunosuppressive TME. Furthermore, it was reported that CD133+ CSCs of HCC cells favorably secrete IL-8, which affects surrounding cells within the CSC niche, resulting in angiogenic effects (102). It was discovered that IL-8 is likely secreted by liver CSCs, as a report demonstrated a positive correlation between the CSC enrichment ratio and NANOG expression with IL-8 secretion (103). The inhibition of IL-8 repressed the properties of HCC CSCs and greatly improved cell sensitivity to sorafenib treatment (104). Although there is a lack of convincing evidence that IL-8 acts as an immunosuppressive cytokine, this chemoattractant may be capable of conditioning the TME to become immunosuppressive through the recruitment of myeloid-derived suppressor cells (MDSCs) to the TME. Studies have also revealed that IL-4 and IL-13 may contribute to the creation of an immunosuppressive TME, as they recruit and activate TAMs and MDSCs. A study, which used cell line models that originated from the AKT1/NRAS-induced HCC mouse model, revealed that IL-4/IL-13 signaling plays a crucial role in cancer development and that these cells exhibit strong CSC and CD44+ and CD133+ signatures (104). There is a lack of evidence that CSCs in HCC are involved in the secretion of both IL-4 and IL-13, although some studies have shown that CSCs in other malignancies can secrete these immunosuppressive cytokines (105).
5.2 Chemokines
Chemokines are secreted proteins that play essential roles in organ development and immune responses by binding to their corresponding receptors. Chemokines are involved in the coordination and recruitment of immune cells from and toward tissues, as well as in modulating interactions among immune cells. In the TME, chemokines regulate the growth, invasiveness, and stemness of tumor cells, and, simultaneously with their production by tumor cells, chemokines attract leukocytes, modulate neurogenesis and fibrogenesis, and induce the formation of blood vessels (106). Nevertheless, how chemokines operate within the TME to modulate several elements of immune cell initiation and phenotypic distinction remains unclear. The expression of numerous types of chemokines and their receptors has been characterized and studied in HCC cells (107).
From a previously described study, elevated expressions of chemokines, including CXCL2, CXCL3, CXCL15, CXCL17, CXCR2, and CXCR4, within the TME of established cell lines models that derived from AKT1/NRAS-induced HCC mouse model has also been reported (104). Studies have shown that the CXCR4/CXCL12 axis has an essential biological function and contributes to tumor metastasis and angiogenesis in various malignancies, including HCC (108). Because CSCs can promote metastasis and tumor recurrence, the CXCR4/CXCL12 axis may indirectly influence the properties of CSCs. After a series of subcutaneous implantations involving NOD/SCID mice, it was revealed that circulating HCC cells in the presence of CXCR4 along with CD90 and matrix metalloproteinase (MMP) 26 exhibit the CSC properties of tumor formation and metastatic potential (109, 110). The immunosuppressive effect of CXCR4 on the TME is known to be due to its high expression in a subset of immunosuppressive regulatory T cells (Tregs). It has also been shown that the inhibition of CXCR4 results in a reversal of Treg suppression, hence enhancing antitumor immune responses (111). CXCL5 expression is induced by Sox9+ HCC CSCs, which triggers HCC migration, invasion, and proliferation through the PI3K-AKT and ERK1/2 signaling pathways (112). CXCL5, a well-known chemokine, is assumed to contribute to the establishment of an immunosuppressive TME and tumor stimulation through the recruitment and activation of MDSCs (113). A recent study revealed that CD133+ HCC CSCs secrete high levels of CXCL8, which is preferentially induced by IFN-α and leads to the induction of immunosuppressive effects (114).
5.3 Interferons
IFNs are a family of pleiotropic cytokines that possess antitumor, antiviral, and immunoregulatory effects and are generally categorized into three groups, namely, alpha, beta, and gamma, corresponding to their cellular source. Type 1 IFNs include IFN-α and IFN-β, which are commonly secreted by virus-infected cells, and type 2 IFNs include IFN-γ, which mainly originates from immune cells, such as macrophages, NK cells, and T cells. In the context of cancer, IFN-γ has a dual role as an antitumorigenic factor and protumorigenic factor, where it can promote immune evasion and the establishment of an immunosuppressive TME (115). In HCC, a positive association between CD133+ CSCs and IFN-γ was established, where CD133+ CSCs were able to selectively resist IFN-γ-mediated apoptosis and autophagy (116). In a recent study, IFN-γ was also found to increase the CSC ratio and properties in HCC cells, as well as increase CSC resistance to sorafenib treatment through ferroptosis inhibition (117). Moreover, sensitization to sorafenib-mediated ferroptosis by PD-L1 knockdown led to the inhibition of IFN-γ-related CSCs in HCC (117). These findings demonstrate the ability of IFN-γ to promote an immunosuppressive TME in HCC.
5.4 Tumor necrosis factors
Tumor necrosis factors are versatile cytokines that exist in two forms, TNF-alpha (TNF-α) and TNF-beta (TNF-β), which are involved in various cellular processes, including immune modulation, inflammatory responses, and cell death. TNF-α is a widely studied cytokine due to its crucial role in inflammation-associated tumorigenesis, during which its release primarily involves a variety of immune cells, such as macrophages, dendritic cells (DCs), and T cells. Like other cytokines, TNF-α exhibits pleiotropic effects, which can have both antitumor and protumorigenic effects, as observed in HCC. TNF-α is implicated in hepatocarcinogenesis and HCC tumor relapse by activating hepatic progenitor cells (118) but also contributes to sorafenib resistance through the induction of EMT (119). To date, there is no research indicating the direct secretion of TNF-α by CSCs in the HCC TME; however, the crosstalk between CSCs and TAMs may cause TNF-α release.
6 CSC-mediated immunosuppressive growth factors
As secreted biologically active elements, growth factors act on specific cell receptors that successively transmit their growth signals to other intracellular molecules, eventually resulting in modified gene expression. The tumorigenic role of growth factors in various cancers, including HCC, continues to be explored, and these factors are known to stimulate major signaling pathways involved in promoting tumor development, angiogenesis, and therapeutic resistance. Both TGF-β and vascular endothelial growth factor (VEGF) are major growth factors with immune-suppression capabilities that can regulate the TME in HCC. Robust evidence has demonstrated the hepatocarcinogenic role of TGF-β, which is secreted by HCC cells in general; however, there is no direct evidence indicating that HCC CSCs are responsible for TGF-β release (120). In contrast, VEGF is likely secreted by CSC-like HCC cells, which exhibit high expression of the stemness markers CD44, CD133, and EpCAM following sublethal heat treatment to mimic incomplete radiofrequency ablation treatment (121). In the same study, VEGFR-1 promoted the stemness of treated HCC cells, which exhibited enhanced metastatic and migratory potential (121). Moreover, activation of insulin-like growth factor (IGF) and FGF signaling cascades was correlated with the development of CSC-enriched sorafenib-resistant HCC cells (122). It has been demonstrated that members of the IGFs and FGFs can potentially possess immunosuppressive capabilities, despite having contextual proinflammatory effects (123–126), which in this case may contribute to the formation of an immunosuppressive TME in HCC.
7 Immunosuppressive role of CSC-derived exosomes
A subset of extracellular vesicles (EVs), known as exosomes, are nanoscale particles that are actively released by various cell types, such as epithelial cells, immune cells, neuronal cells, and tumor cells. Biomolecular components of exosomes, namely, lipids, proteins, nucleic acids, and metabolites, are transferred between cells either locally or in distant microenvironments. During cancer development, this process mediates cell-to-cell communication, which is correlated with cancer hallmarks (127). Correspondingly, exosomes that originate from CSCs are secreted by malignant cells and act as mediators to permit communication with the TME. The biological features of these exosomes allow them to participate in cancer progression, proliferation, angiogenesis, metastasis, chemoresistance, and recurrence, suggesting that cancer-derived exosomes are potential biomarkers for various malignancies (127, 128). Moreover, these exosomes can modulate and alter the establishment of an immunosuppressive TME, facilitating the exchange of information from CSCs and promoting immune evasion mechanisms.
As a member of the small GTPase superfamily and Rab family, RAB27A is known to regulate the discharge of exosomes from cells. It was demonstrated that HCC cells with high metastatic capability can release exosomes that are reliant on RAB27A, which ultimately trigger EMT in low metastatic cells (129). RAB27A-mediated exosome secretion from liver CSCs cells leads to an increase in NANOG expression and resistance to regorafenib (129). An elevated signature of NANOG may increase the expansion of Tregs, which contributes to the formation of an immunosuppressive TME (130) and facilitates immune escape, particularly from NK cells (131). In another study, exosomes derived from CD133+ CSCs that contained ALKBH5 were found to be related to SOX4 and to activate the SHH signaling pathway, possibly leading to an immunosuppressive TME in HCC (132). An extensive in vivo study involving an HCC albino rat model revealed that CSC-derived exosomes enhanced tumor metastasis and growth through elevated expression of immunosuppressive factors, including TGF-β1, VEGF, miR-21, and the PI3K pathway (133). RAB3B-induced exosome secretion by HCC cells exhibiting stemness properties may indirectly contribute to the formation of an immunosuppressive TME, as indicated by a positive correlation with the expression of apolipoprotein E, which bears immune suppression capabilities. Further elucidation is required to validate such association (134).
Moreover, researchers have also revealed the role of exosomal biomolecules that may drive the secretion of CSC-derived exosomes within the TME. CD90+ liver CSCs secrete exosomes that contain lncRNA H19, which regulates endothelial cells and induces angiogenic properties that influence the TME (135). Although unrelated to HCC, lncRNA H19 could have an immunosuppressive effect on the TME of gastric cancer cells (136). CD133+ liver CSCs were found to secrete exosomes containing circ-ZEB1 and circ-AFAP1, which may be involved in the crosstalk between non-CSCs and CSCs of HCC and can modulate EMT activity (137). In HCC, EMT is known to participate in the establishment of an immunosuppressive TME, elevated tumor heterogeneity, drug resistance, and metastasis.
Several studies have reported that the direct secretion of various secretory factors from liver CSCs facilitates the formation of an immunosuppressive TME. However, some findings were correlated with the influence of TME components on the stemness properties of HCC cells, which has also been described. The complexity of HCC and the dynamic nature of the secretion of factors by CSCs pose additional challenges for further elucidation of the secretion of factors by CSCs within the HCC TME. For instance, IL-10 is a well-known immunosuppressive cytokine that is commonly produced by various immune cells, but direct evidence demonstrating the ability of CSCs in HCC to secrete IL-10 has not been found. Instead, immunosuppressive factors may be released by CSC-associated TME components.
8 Crosstalk and recruitment of TME components by CSC-derived secretory factors
Accumulating evidence suggests that, in addition to the secretion of factors by CSCs into their surroundings, CSCs are also involved in the recruitment, activation, and crosstalk of various cellular components within the CSC niche to modulate the immunosuppressive TME. Such components may include but are not limited to CAFs, undifferentiated mesenchymal stem cells (MSCs), and immune cells, such as TAMs and MDSCs. These cellular components serve as key players in the TME to preserve tumor growth; maintain CSCs, angiogenesis, migration, and metastasis; regulate inflammatory processes; and produce ECM components (138).
8.1 Cancer-associated fibroblasts
The development of CAFs in the TME originates from fibroblast modifications via crosstalk with malignant cells and other stromal cells, which ultimately promotes regional tumor invasion, progression, and dedifferentiation through the influence of elements such as chemokines, growth factors, and ECM-modifying metalloproteases. These highly inflamed cells play a substantial role in HCC progression, as the majority of patients with HCC have cirrhosis and a large number of stimulated fibroblasts. The crosstalk between CD24+ HCC CSCs and CAFs results in the paracrine secretion of HGF/c-Met and IL-6/IL-6R, which results in an enhancement of stemness properties through the phosphorylation of STAT3 (139). In this study, the pleiotropic nature of HGF and IL-6 was shown to be involved in the activation of STAT3 signaling, which may facilitate the formation of an immunosuppressive HCC TME. Similarly, another study demonstrated that the crosstalk of CAFs with CSCs led to the significant secretion of CAF-derived HGF and FRA1 activation (140). The secretion of HGF, which induces the activation of FRA1, might have an indirect facilitative influence on creating an immunosuppressive TME in HCC. Moreover, another study revealed the possible synergistic effect of CAFs with CD73+ CSCs, which promotes HCC tumorigenesis and chemoresistance through paracrine secretion of HGF from CAFs (141).
8.2 Mesenchymal stem cells
As one of the precursors of CAFs, undifferentiated MSCs are associated with the progression of malignancies and metastatic events, as well as an increase in the abundance and tumorigenic potential of CSCs. In HCC, accumulating research has revealed the controversial role of MSCs in the dual characteristics of tumor induction and suppression via positive regulation of the immune system. Recently, MSCs of diverse origins have been shown to be correlated with HCC progression and metastasis. Crosstalk between CSCs and HCC-associated MSCs may involve a novel lncRNA-MSC-upregulated factor, which was found to be highly expressed and associated with HCC tumorigenicity (142). Implicitly, lncRNA-MSC-upregulated factors, linked with ANXA2 and miR-34a, resulted in the activation and upregulation of immunosuppressive signaling pathways, namely, Wnt/β-catenin, Snail1, and EMT, which may facilitate the formation of an immunosuppressive TME in HCC (142).
8.3 Tumor-associated macrophages
In general, macrophages can polarize into two phenotypes, proinflammatory M1 and anti-inflammatory M2 macrophages, in which TAMs express M2 properties, leading to immunosuppression and protumorigenic capabilities. TAMs are immune cells within the tumor stroma that induce cancer progression and support the CSC niche. These well-defined cells are recruited by CSCs within the HCC tumor bulk via chemokine secretion. The crosstalk mechanism between TAMs and CSCs was shown to be related to the immunosuppressive EMT process induced by TAM-derived TGF-β1 (143). Moreover, M2 TAMs were found to be related to CD44+/CD133+ sorafenib-resistant HCC cells, which correlated with potential immunosuppressive CXCR2 signaling (144). Crosstalk between CD133-expressing HCC cells and TAMs could lead to the promotion of stemness properties, EMT activity, and the secretion of immunosuppressive TNFα, which are correlated with the Wnt/β-catenin pathway (145). In addition, two studies revealed that the interaction of TAMs with HCC CSCs and various markers led to the secretion of IL-6 by TAMs and subsequently activated STAT3 signaling (101, 146).
8.4 Myeloid-derived suppressor cells
In addition to TAMs, which are crucial immunomodulators of the TME, MDSCs play a prominent role in inhibiting T-cell infiltration into tumor sites. These premature marrow-derived cell populations contribute to HCC progression through either modulation of T-cell responses or independent action. MDSCs exert their immunosuppressive function by suppressing T-cell immune responses through the production of NO, ROS, arginase 1, IL-10, and TGF-β (147–149). The stimulation of immune suppressor cells, such as regulatory T-cells, through differentiation and expansion can further impede T-cell activity (148, 149). In terms of HCC, MDSCs can impede the cytotoxic function of NK cells against HCC cells and diminish the production of IFN-γ through membrane-bound TGF-β1 (148). MDSCs are strongly associated with therapeutic resistance to ICIs. In terms of HCC, a study revealed that MDSCs might be implicated in anti-PD-L1 resistance, where HCC patient-derived T-cells were found to be exhausted and that PD-L1+ MDSCs are involved in the suppression of T-cell function (150). An additional study revealed that MDSC-induced CCRK/EZH2/NF-κB/IL-6 signaling in HCC facilitates dampened antitumor T-cell activity and leads to immune evasion (151). As CCRK depletion resulted in a decrease in the immunosuppressive effect of MDSCs and led to an increase in anti-PD-L1 efficacy, this may suggest the influence of MDSCs on anti-PD-L1 resistance in HCC (151). To date, there is no related evidence indicating that the recruitment and crosstalk between MDSCs and CSCs in HCC leads to the secretion of immunosuppressive secretory factors. However, several studies have mentioned the possibility that factors within the TME that participate in MDSC recruitment may drive tumorigenesis. These factors include IL-1α (152), HIF-mediated IL-1β (153), HIF-mediated CCL26 (154), CCL2 (155), CXCL1 (155), CD36+ CAFs (156), CCL5 (157), HMGB1 (158), and GM-CSF (159).
9 Therapeutic implications of targeting secretory factors within the immunosuppressive TME of HCC
The complex interplay between tumor cells, CSCs, cellular elements, and immune cells within the TME presents a challenge for therapeutic intervention. CSCs exert intricate therapeutic resistance mechanisms, which leads to difficulties in overcoming the immunosuppressive TME of HCC. The development of innovative approaches to counter the immunosuppressive TME, which mostly involves the inhibition of allegedly implicated secretory factors, is imperative. While most of these inhibitors or molecules have already been assessed by researchers in the preclinical stage, some are undergoing clinical trials (Table 2).
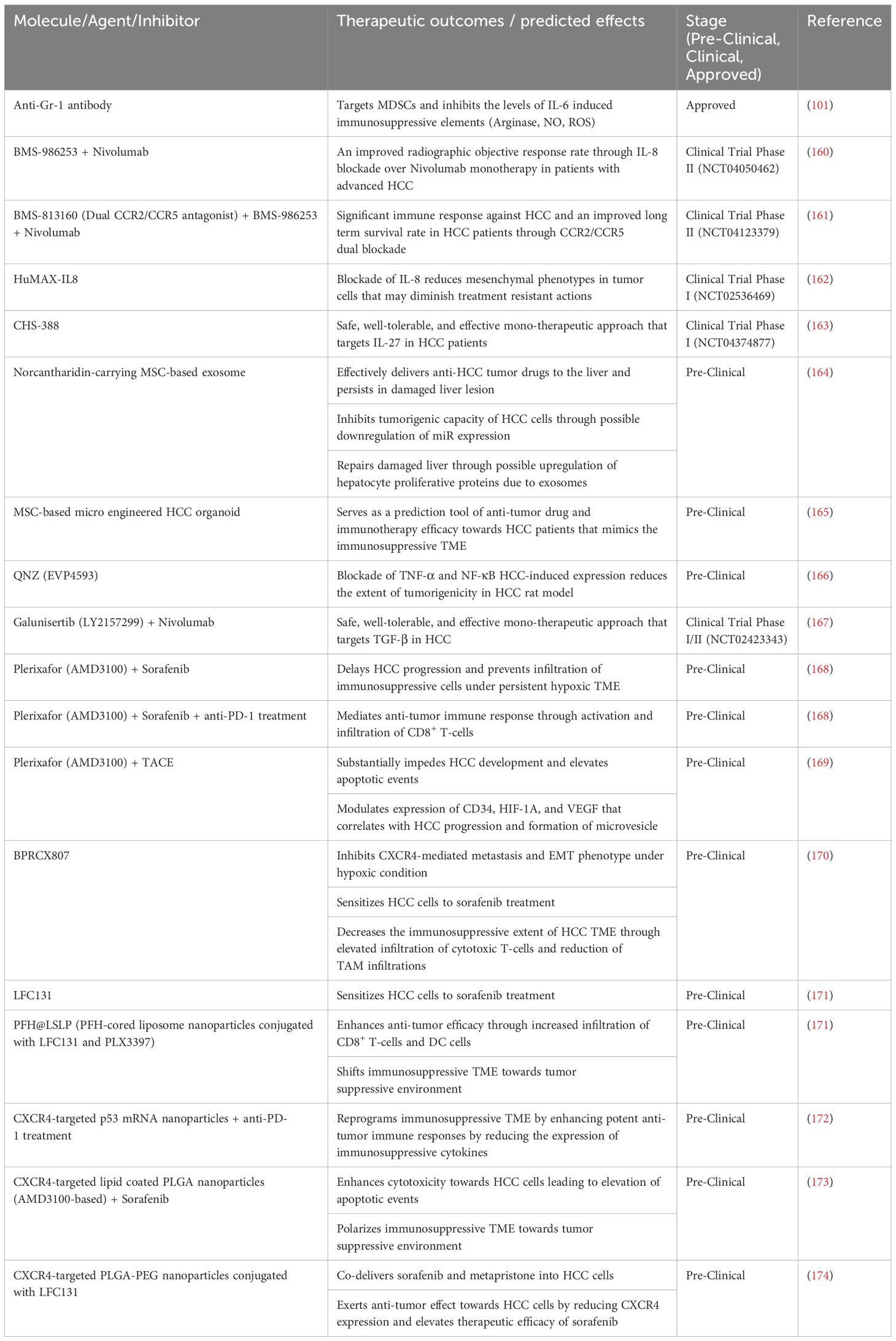
Table 2 Therapeutic strategies that target immunosuppressive secretory factors within the TME of HCC.
An established IL-6 antagonist known as an anti-Gr-1 antibody targets immunosuppressive MDSCs, resulting in a decrease in IL-6 levels and related immunosuppressive elements, such as arginase, NO, and ROS (101). This approach leads to the depletion of MDSCs and enhances chemotherapeutic efficacy and response. A humanized IL-8 antagonist known as BMS-986253 is currently in a phase II clinical trial (NCT04050462) in combination with nivolumab, an anti-PD-1 antibody (160). This combination therapy approach aims to improve the objective response rate of patients with advanced HCC compared with that of patients undergoing nivolumab monotherapy. Similarly, combination therapy involving BMS-986253, nivolumab, and BMS-813160 is currently in a phase II clinical trial (NCT04123379) (161). BMS-813160 is a dual CCR2/CCR5 antagonist in which these targets are known to be immunosuppressive. A substantial positive immune response and long-term survival benefit for HCC patients are expected from this clinical trial. BMS-986253 or HuMAX-IL8 alone has been tested in a phase I clinical trial (NCT02536469), with the expectation that blockade of IL-8 can degrade mesenchymal phenotypes in solid tumor cells and diminish treatment resistance (162). However, this clinical trial does not focus on HCC specifically but rather on all forms of solid tumors. Moreover, CHS-388, formerly known as SRF-388, an antagonist of the immunosuppressive agent IL-27, is being tested in a phase I clinical trial (NCT04374877) focused on developing a safe, well-tolerated, and effective monotherapeutic approach for targeting IL-27 in HCC patients (163).
MSC-based therapeutic approaches have also been documented in preclinical trials. One study investigated the delivery of norcantharidin, a potential anticancer drug in HCC patients, which is mediated by MSC-derived exosomes (164). This study demonstrated the effective delivery of anti-HCC tumor drugs to the liver, where they persist in damaged liver lesions and inhibit the tumorigenic capabilities of HCC cells through possible downregulation of miR expression (164). Moreover, this delivery system repairs the damaged liver through possible upregulation of hepatocyte proliferative proteins due to exosomes (164). In another study, norcantharidin was found to impede IL-6-induced EMT, leading to suppression of the JAK/STAT/TWIST signaling pathway (175). Interestingly, a research group successfully devised a microengineered organoid-on-a-chip through a coculture system of MSCs and peripheral blood mononuclear cells (PBMCs) in combination with CAFs and patient-derived organoids (PDOs) that can mimic the immunosuppressive TME (165). Such an innovation provides a platform for researchers to predict the outcomes of patients treated with immunotherapeutic modalities for HCC.
QNZ (EVP4593) is a relatively novel antagonist that inhibits HCC-induced TNF-α and NF-κB expression, leading to increased survival, reduced liver nodules, and an improved hepatocyte structure in the HCC rat model (166). Moreover, galunisertib (LY2157299) is undergoing a phase I/II clinical trial (NCT02423343) in conjunction with nivolumab (167). This clinical trial investigated the clinical efficacy and safety of the combination of galunisertib and nivolumab in patients with recurrent or refractory HCC. Galunisertib is an antagonist of the immunosuppressive agent TGF-β, and preliminary results have shown that galunisertib induces a vigorous antitumor immune response and mediates the breach of T cells toward the midpoint of tumors (176).
Plerixafor (AMD3100) is a potential CXCR4 antagonist that continues to be studied. AMD3100 in combination with sorafenib was found to delay the progression of HCC and impede the infiltration of immunosuppressive cells under persistent hypoxic conditions (168). Moreover, the same study incorporated AMD3100, sorafenib, and anti-PD-1 treatment, which mediated the antitumor immune response through the activation and infiltration of CD8+ T cells (168). TACE is still used for treating HCC patients, and a research group investigated the effects of combination therapy of AMD3100 with TACE in an HCC rat model. The results indicated a substantial hindrance to HCC development and an increase in apoptotic events, in which such a therapeutic approach can modulate the expression of CD34, HIF-1α, and VEGF, which is correlated with HCC progression and the formation of microvesicles (169). Moreover, another potent CXCR4 inhibitor known as BPRCX807 was found to inhibit CXCR4-mediated metastasis and the EMT phenotype under hypoxic conditions, sensitize HCC cells to sorafenib treatment, and decrease the extent of immunosuppression in the HCC TME through increased infiltration of cytotoxic T cells and reduced infiltration of TAMs (170). Similarly, LFC131, a CXCR4 peptide-based inhibitor, was also found to sensitize HCC cells to sorafenib treatment (171).
The utilization of a nanoparticle-based treatment approach was found to facilitate the targeting of a specific protein target, namely CXCR4. PFH@LSLP, which is a PFH-cored liposome nanoparticle conjugated with LFC131 and PLX3397, was shown to enhance antitumor efficacy through increased infiltration of CD8+ T cells and DCs while also shifting the immunosuppressive TME toward a tumor-suppressing environment (171). Similar results were also exhibited in a study that focused on CXCR4-targeted p53 mRNA nanoparticles in combination with anti-PD-1 treatment (172). Aside from the modified TME state from being immunosuppressive, this therapeutic approach elevates the expression of MHC-I and reduces the expression of immunosuppressive cytokines (172). AMD3100-based lipid-coated Poly (lactic-co-glycolic acid) (PLGA) nanoparticles in combination with sorafenib, which was developed by a research group, has been shown to enhance the cytotoxicity toward HCC, leading to elevated apoptotic events and polarizing the immunosuppressive TME toward a tumor-suppressive environment (173). In addition, CXCR4-targeted PLGA-PEG nanoparticles conjugated with LFC131 allow the codelivery of sorafenib and metapristone into HCC cells, which leads to antitumor effects through a decrease in CXCR4 expression and an increase in the efficacy of sorafenib treatment (174).
10 Conclusion
Liver CSCs have been shown to contribute significantly to therapeutic resistance and tumor relapse in HCC patients, suggesting that prevention strategies and the development of innovative treatment approaches are important. Additionally, therapeutic resistance becomes even more complex with the contribution of secretory factors derived from liver CSCs, which leads to the establishment of an immunosuppressive TME that debilitates effective antitumor immune responses. As described in this review, CSCs may participate in the secretion of immunosuppressive factors, such as cytokines, growth factors, and exosomes, which collectively make up anatomically distinct CSC niches within the TME in HCC. Although there is a lack of evidence and research on the secretion of immunosuppressive factors by CSCs, these conspicuous cells can attract and participate in crosstalk with diverse cellular components within the TME that ultimately secrete various factors. To address the immunosuppressive state of the TME, therapeutic approaches targeting these secretory factors are crucial. An in-depth analysis of these approaches is needed to provide a better understanding of how to effectively reduce the immunosuppressive state of the TME in patients with HCC while still providing beneficial, safe, and well-tolerated ICI treatment options.
Author contributions
MK: Writing – review & editing, Writing – original draft. TK-W: Writing – review & editing, Supervision, Funding acquisition.
Funding
The author(s) declare financial support was received for the research, authorship, and/or publication of this article. This work was supported by RGC General Research Fund (15104023) and Research Impact Fund (R7022-20 and R5008-22F). This funding provides the financial support for the publication fee.
Conflict of interest
The authors declare that the research was conducted in the absence of any commercial or financial relationships that could be construed as a potential conflict of interest.
Publisher’s note
All claims expressed in this article are solely those of the authors and do not necessarily represent those of their affiliated organizations, or those of the publisher, the editors and the reviewers. Any product that may be evaluated in this article, or claim that may be made by its manufacturer, is not guaranteed or endorsed by the publisher.
Glossary
References
1. Sung H, Ferlay J, Siegel RL, Laversanne M, Soerjomataram I, Jemal A, et al. Global Cancer Statistics 2020: GLOBOCAN estimates of incidence and mortality worldwide for 36 cancers in 185 countries. CA: A Cancer J Clin. (2021) 71:209–49. doi: 10.3322/caac.21660
2. Janevska D, Chaloska-Ivanova V, Janevski V. Hepatocellular carcinoma: risk factors, diagnosis and treatment. Open Access Macedonian J Med Sci. (2015) 3:732–6. doi: 10.3889/oamjms.2015.111
3. Liu Z, Song C, Suo C, Hong F, Zhang T, Jin L, et al. Alcohol consumption and hepatocellular carcinoma: novel insights from a prospective cohort study and nonlinear Mendelian randomization analysis. BMC Med. (2022) 20:413. doi: 10.1186/s12916–022-02622–8
4. Petrick JL, Campbell PT, Koshiol J, Thistle JE, Andreotti G, Freeman LEB, et al. Tobacco, alcohol use and risk of hepatocellular carcinoma and intrahepatic cholangiocarcinoma: The Liver Cancer Pooling Project. Br J Cancer. (2018) 118:1005–12. doi: 10.1038/s41416-018-0007-z
5. Siegel AB, Zhu AX. Metabolic syndrome and hepatocellular carcinoma: Two growing epidemics with a potential link. Cancer. (2009) 115:5651–61. doi: 10.1002/cncr.24687
6. Paradis V, Zalinski S, Chelbi E, Guedj N, Degos F, Vilgrain V, et al. Hepatocellular carcinomas in patients with metabolic syndrome often develop without significant liver fibrosis: A pathological analysis. Hepatology. (2009) 49:851–9. doi: 10.1002/hep.22734
7. Ronot M, Bouattour M, Wassermann J, Bruno O, Dreyer C, Larroque B, et al. Alternative Response Criteria (CHOI, European Association for the Study of the Liver, and Modified Response Evaluation Criteria in solid Tumors [RECIST]) versus RECIST 1.1 in patients with advanced hepatocellular carcinoma treated with Sorafenib. Oncologist. (2014) 19:394–402. doi: 10.1634/theoncologist.2013–0114
8. Singal AG, Nehra M, Adams-Huet B, Yopp AC, Tiro JA, Marrero JA, et al. Detection of hepatocellular carcinoma at advanced stages among patients in the HALT-C trial: Where did surveillance fail? Am J Gastroenterology. (2013) 108:425–32. doi: 10.1038/ajg.2012.449
9. Llovet JM, Bustamante J, Castells A, Vilana R, Del Carmen Ayuso M, Sala M, et al. Natural history of untreated nonsurgical hepatocellular carcinoma: Rationale for the design and evaluation of therapeutic trials. Hepatology. (1999) 29:62–7. doi: 10.1002/hep.510290145
10. Yang JD. Detect or not to detect very early stage hepatocellular carcinoma? The western perspective. Clin Mol Hepatology. (2019) 25:335–43. doi: 10.3350/cmh.2019.0010
11. Reig M, Forner A, Rimola J, Ferrer-Fàbrega J, Burrel M, García-Criado Á, et al. BCLC strategy for prognosis prediction and treatment recommendation: The 2022 update. J Hepatology. (2022) 76:681–93. doi: 10.1016/j.jhep.2021.11.018
12. Santopaolo F, Lenci I, Mihalj M, Manzia TM, Baiocchi L. Liver transplantation for hepatocellular carcinoma: Where do we stand? World J Gastroenterology. (2019) 25:2591–602. doi: 10.3748/wjg.v25.i21.2591
13. Sapisochín G, Bruix J. Liver transplantation for hepatocellular carcinoma: outcomes and novel surgical approaches. Nat Rev Gastroenterol Hepatology. (2017) 14:203–17. doi: 10.1038/nrgastro.2016.193
14. Lee DH, Kim JW, Lee JM, Kim JM, Lee MW, Rhim H, et al. Laparoscopic liver resection versus percutaneous radiofrequency ablation for small single nodular hepatocellular carcinoma: Comparison of treatment outcomes. Liver Cancer. (2021) 10:25–37. doi: 10.1159/000510909
15. Li J, Liu X, Cui H, Xie X. Radiofrequency ablation vs. surgical resection for resectable hepatocellular carcinoma: A systematic review and meta-analysis. Mol Clin Oncol. (2019) 12:15–22. doi: 10.3892/mco.2019.1941
16. Wei C, Chau GY, Chen PH, Liu CA, Huang YH, Huo TI, et al. A comparison of prognoses between surgical resection and radiofrequency ablation therapy for patients with hepatocellular carcinoma and esophagogastric varices. Sci Rep. (2020) 10:17259. doi: 10.1038/s41598-020-74424-y
17. Bruix J, Raoul J, Sherman M, Mazzaferro V, Bolondi L, Craxì A, et al. Efficacy and safety of sorafenib in patients with advanced hepatocellular carcinoma: Subanalyses of a phase III trial. J Hepatology. (2012) 57:821–9. doi: 10.1016/j.jhep.2012.06.014
18. Tang W, Chen Z, Zhang W, Cheng Y, Zhang B, Wu F, et al. The mechanisms of sorafenib resistance in hepatocellular carcinoma: theoretical basis and therapeutic aspects. Signal Transduction Targeted Ther. (2020) 5:87. doi: 10.1038/s41392-020-0187-x
19. Uehara Y, Ikeda S, Kim K, Lim H, Adashek JJ, Persha HE, et al. Targeting the FGF/FGFR axis and its co-alteration allies. ESMO Open. (2022) 7:100647. doi: 10.1016/j.esmoop.2022.100647
20. Muraishi N, Matsuyama Y, Akuta N, Shindoh J, Matsumura M, Okubo S, et al. The Impact of lenvatinib on tumor blood vessel shrinkage of hepatocellular carcinoma during treatment: An imaging-based analysis. Oncology. (2022) 101:134–44. doi: 10.1159/000526976
21. Hegde A, Andreev-Drakhlin AY, Roszik J, Huang L, Liu S, Hess KR, et al. Responsiveness to immune checkpoint inhibitors versus other systemic therapies in RET-aberrant Malignancies. ESMO Open. (2020) 5:e000799. doi: 10.1136/esmoopen-2020–000799
22. Wang Y, Yang X, Wang D, Long J, Zhou J, Lu Z, et al. Lenvatinib beyond first-Line therapy in patients with advanced biliary tract carcinoma. Front Oncol. (2022) 12:785535. doi: 10.3389/fonc.2022.785535
23. Murakami M, Zhao S, Zhao Y, Chowdhury N, Yu W, Nishijima K, et al. Evaluation of changes in the tumor microenvironment after sorafenib therapy by sequential histology and 18F-fluoromisonidazole hypoxia imaging in renal cell carcinoma. Int J Oncol. (2012) 41:1593–600. doi: 10.3892/ijo.2012.1624
24. Liang Y, Zheng T, Song R, Wang J, Yin D, Wang L, et al. Hypoxia-mediated sorafenib resistance can be overcome by EF24 through Von Hippel-Lindau tumor suppressor-dependent HIF-1α inhibition in hepatocellular carcinoma. Hepatology. (2013) 57:1847–57. doi: 10.1002/hep.26224
25. Nagai T, Arao T, Furuta K, Sakai K, Kudo K, Kaneda H, et al. Sorafenib inhibits the hepatocyte growth Factor–Mediated epithelial mesenchymal transition in hepatocellular carcinoma. Mol Cancer Ther. (2011) 10:169–77. doi: 10.1158/1535–7163.mct-10–0544
26. Van Malenstein H, Dekervel J, Verslype C, Van Cutsem É, Windmolders P, Nevens F, et al. Long-term exposure to sorafenib of liver cancer cells induces resistance with epithelial-to-mesenchymal transition, increased invasion and risk of rebound growth. Cancer Letters. (2013) 329:74–83. doi: 10.1016/j.canlet.2012.10.021
27. Jin H, Shi Y, Lv Y, Yuan S, Ramirez C, Lieftink C, et al. EGFR activation limits the response of liver cancer to lenvatinib. Nature. (2021) 595:730–4. doi: 10.1038/s41586–021-03741–7
28. Lu Y, Shen H, Huang W, He S, Chen J, Zhang D, et al. Genome-scale CRISPR-Cas9 knockout screening in hepatocellular carcinoma with lenvatinib resistance. Cell Death Discovery. (2021) 7:359. doi: 10.1038/s41420-021-00747-y
29. Phi LT, Sari IN, Yang Y, Lee S, Jun N, Kim KS, et al. Cancer Stem Cells (CSCs) in drug resistance and their therapeutic implications in cancer treatment. Stem Cells Int. (2018) 2018:5416923. doi: 10.1155/2018/5416923
30. Ayob AZ, Ramasamy TS. Cancer stem cells as key drivers of tumour progression. J Biomed Science. (2018) 25:20. doi: 10.1186/s12929–018-0426–4
31. Emmink BL, Verheem A, Van Houdt WJ, Steller EJ, Govaert KM, Pham TV, et al. The secretome of colon cancer stem cells contains drug-metabolizing enzymes. J Proteomics. (2013) 91:84–96. doi: 10.1016/j.jprot.2013.06.027
32. Yadav UP, Singh T, Kumar P, Sharma P, Kaur H, Sharma SN, et al. Metabolic adaptations in cancer stem cells. Front Oncol. (2020) 10:1010. doi: 10.3389/fonc.2020.01010
33. Zhu X, Chen H, Gao C, Zhang X, Jiang J, Zhang Y, et al. Energy metabolism in cancer stem cells. World J Stem Cells. (2020) 12:448–61. doi: 10.4252/wjsc.v12.i6.448
34. Ishimoto T, Nagano O, Yae T, Tamada M, Motohara T, Oshima H. CD44 variant regulates redox status in cancer cells by stabilizing the xCT subunit of system xc– and thereby promotes tumor growth. Cancer Cell. (2011) 19:387–400. doi: 10.1016/j.ccr.2011.01.038
35. Son YW, Cheon MG, Kim Y, Jang HH. Prx2 links ROS homeostasis to stemness of cancer stem cells. Free Radical Biol Med. (2019) 134:260–7. doi: 10.1016/j.freeradbiomed.2019.01.001
36. Vitale I, Manic G, De Maria R, Kroemer G, Galluzzi L. DNA damage in stem cells. Mol Cell. (2017) 66:306–19. doi: 10.1016/j.molcel.2017.04.006
37. Skvortsov S, Debbage P, Lukáš P, Skvortsova I. Crosstalk between DNA repair and cancer stem cell (CSC) associated intracellular pathways. Semin Cancer Biol. (2015) 31:36–42. doi: 10.1016/j.semcancer.2014.06.002
38. Paul R, Dorsey JF, Fan Y. Cell plasticity, senescence, and quiescence in cancer stem cells: Biological and therapeutic implications. Pharmacol Ther. (2022) 231:107985. doi: 10.1016/j.pharmthera.2021.107985
39. Dean M. ABC transporters, drug resistance, and cancer stem cells. J Mammary Gland Biol Neoplasia. (2009) 14:3–9. doi: 10.1007/s10911–009-9109–9
40. García-Mayea Y, Mir C, Masson F, Paciucci R, Lleonart ME. Insights into new mechanisms and models of cancer stem cell multidrug resistance. Semin Cancer Biol. (2020) 60:166–80. doi: 10.1016/j.semcancer.2019.07.022
41. Erin N, Grahovac J, Brozović A, Efferth T. Tumor microenvironment and epithelial mesenchymal transition as targets to overcome tumor multidrug resistance. Drug Resistance Updates. (2020) 53:100715. doi: 10.1016/j.drup.2020.100715
42. Wang Z, Zöller M. Exosomes, metastases, and the miracle of cancer stem cell markers. Cancer Metastasis Rev. (2019) 38:259–95. doi: 10.1007/s10555–019-09793–6
43. Takahashi K, Yamanaka S. Induction of pluripotent stem cells from mouse embryonic and adult fibroblast cultures by defined factors. Cell. (2006) 126:663–76. doi: 10.1016/j.cell.2006.07.024
44. Okita K, Ichisaka T, Yamanaka S. Generation of germline-competent induced pluripotent stem cells. Nature. (2007) 448:313–7. doi: 10.1038/nature05934
45. Zhang H, Wang ZZ. Mechanisms that mediate stem cell self-renewal and differentiation. J Cell Biochem. (2008) 103:709–18. doi: 10.1002/jcb.21460
46. Lee TK, Guan XY, Ma S. Cancer stem cells in hepatocellular carcinoma — from origin to clinical implications. Nat Rev Gastroenterol Hepatology. (2022) 19:26–44. doi: 10.1038/s41575–021-00508–3
47. Yoneda N, Sato Y, Kitao A, Ikeda H, Sawada-Kitamura S, Miyakoshi M, et al. Epidermal growth factor induces cytokeratin 19 expression accompanied by increased growth abilities in human hepatocellular carcinoma. Lab Invest. (2011) 91:262–72. doi: 10.1038/labinvest.2010.161
48. Uenishi T, Kubo S, Yamamoto T, Shuto T, Ogawa M, Tanaka H, et al. Cytokeratin 19 expression in hepatocellular carcinoma predicts early postoperative recurrence. Cancer Science. (2003) 94:851–7. doi: 10.1111/j.1349-7006.2003.tb01366.x
49. Lee JI, Lee JW, Kim JM, Kim JK, Chung HJ, Kim YS. Prognosis of hepatocellular carcinoma expressing cytokeratin 19: Comparison with other liver cancers. World J Gastroenterology. (2012) 18:4751. doi: 10.3748/wjg.v18.i34.4751
50. Takano M, Shimada K, Fujii T, Morita K, Takeda M, Nakajima Y, et al. Keratin 19 as a key molecule in progression of human hepatocellular carcinomas through invasion and angiogenesis. BMC Cancer. (2016) 16:903. doi: 10.1186/s12885-016-2949-y
51. Shuyao W, Bao M, Ma F, Ha X. CK19 predicts recurrence and prognosis of HBV positive HCC. J Gastrointestinal Surgery. (2021) 26:341–51. doi: 10.1007/s11605-021-05107-w
52. Wu M, Zhong J, Chen K, Luo C, Zhang J, Zhou Y, et al. Association of CK19 expression with the efficacy of adjuvant transarterial chemoembolization after hepatic resection in hepatocellular carcinoma patients at high risk of recurrence. J Clin Trans Res. (2022) 8:71–9. doi: 10.18053/jctres.08.202201.009
53. Zhuo J, Lu D, Lin Z, Yang X, Yang M, Wang J, et al. The distinct responsiveness of cytokeratin 19-positive hepatocellular carcinoma to regorafenib. Cell Death Disease. (2021) 12:1084. doi: 10.1038/s41419–021-04320–4
54. Wang N, Wang S, Li M, Hu B, Liu L, Yang S, et al. Cancer stem cells in hepatocellular carcinoma: an overview and promising therapeutic strategies. Ther Adv Med Oncol. (2018) 10:175883591881628. doi: 10.1177/1758835918816287
55. Wu Y, Zhang J, Zhang X, Zhou H, Liu G, Li Q. Cancer stem cells: a potential breakthrough in HCC-Targeted therapy. Front Pharmacol. (2020) 11:198. doi: 10.3389/fphar.2020.00198
56. Zhao Y, Wu H, Xing X, Ma Y, Ji S, Xu X, et al. CD13 induces autophagy to promote hepatocellular carcinoma cell chemoresistance through the P38/HSP27/CREB/ATG7 pathway. J Pharmacol Exp Ther. (2020) 374:512–20. doi: 10.1124/jpet.120.265637
57. Hu B, Xu Y, Li Y, Huang J, Cheng J, Guo W, et al. CD13 promotes hepatocellular carcinogenesis and sorafenib resistance by activating HDAC5-LSD1-NF-κB oncogenic signaling. Clin Trans Med. (2020) 10:e233. doi: 10.1002/ctm2.233
58. Ji S, Ma Y, Xing X, Ge B, Li Y, Xu X, et al. Suppression of CD13 enhances the cytotoxic effect of chemotherapeutic drugs in hepatocellular carcinoma cells. Front Pharmacol. (2021) 12:660377. doi: 10.3389/fphar.2021.660377
59. Li B, Zhao T, Shao M, Cai J, Chen S, Chen X, et al. Attenuated Salmonella carrying siRNA-CD24 improved the effect of oxaliplatin on HCC. Int Immunopharmacology. (2023) 124:111025. doi: 10.1016/j.intimp.2023.111025
60. Lu S, Yao Y, Xu G, Zhou C, Zhang Y, Sun J, et al. CD24 regulates sorafenib resistance via activating autophagy in hepatocellular carcinoma. Cell Death Disease. (2018) 9:646. doi: 10.1038/s41419-018-0681-z
61. Zhang J, He X, Wan Y, Zhang H, Tang T, Zhang M, et al. CD44 promotes hepatocellular carcinoma progression via upregulation of YAP. Exp Hematol Oncol. (2021) 10:54. doi: 10.1186/s40164-021-00247-w
62. Song X, Ding F, Luo W, Tao J, Yang K, Li Q, et al. Knockdown of CD44 inhibits proliferation, migration, and invasiveness in hepatocellular carcinoma cells by modulating CXCR4/Wnt/β-Catenin Axis. Acta Biochim Polonica. (2023) 70:117–22. doi: 10.18388/abp.2020_6319
63. Xie P, Yan J, Wu M, Li H, Chen Z, Yu M, et al. CD44 potentiates hepatocellular carcinoma migration and extrahepatic metastases via the AKT/ERK signaling CXCR4 axis. Ann Trans Med. (2022) 10:689. doi: 10.21037/atm-22–2482
64. Wang S, Wu Q, Chen T, Su R, Pan C, Qian J, et al. Blocking CD47 promotes antitumour immunity through CD103+ dendritic cell–NK cell axis in murine hepatocellular carcinoma model. J Hepatology. (2022) 77:467–78. doi: 10.1016/j.jhep.2022.03.011
65. Du K, Li Y, Liu J, Chen W, Wei Z, Luo Y. A bispecific antibody targeting GPC3 and CD47 induced enhanced antitumor efficacy against dual antigen-expressing HCC. Mol Ther. (2021) 29:1572–84. doi: 10.1016/j.ymthe.2021.01.006
66. Zhang K, Che S, Su Z, Zheng S, Zhang H, Yang S, et al. CD90 promotes cell migration, viability and sphere-forming ability of hepatocellular carcinoma cells. Int J Mol Med. (2018) 41:946–54. doi: 10.3892/ijmm.2017.3314
67. Wang Y, Wang B, Xiao S, Yang L, Chen Q. miR-125a/b inhibits tumor-associated macrophages mediated in cancer stem cells of hepatocellular carcinoma by targeting CD90. J Cell Biochem. (2018) 120:3046–55. doi: 10.1002/jcb.27436
68. Song Y, Kim S, Lee H, No JH, Ryu HC, Kim J, et al. Chromenopyrimidinone controls stemness and Malignancy by suppressing CD133 expression in hepatocellular carcinoma. Cancers. (2020) 12:1193. doi: 10.3390/cancers12051193
69. Song Y, Kim I, Choi I, Kim S, Seo HR. Oxytetracycline have the therapeutic efficiency in CD133+ HCC population through suppression CD133 expression by decreasing of protein stability of CD133. Sci Rep. (2018) 8:16100. doi: 10.1038/s41598–018-34301–1
70. Zhou G, Bae SDW, Nguyen R, Huo X, Han S, Zhang Z, et al. An aptamer-based drug delivery agent (CD133-apt-Dox) selectively and effectively kills liver cancer stem-like cells. Cancer Letters. (2021) 501:124–32. doi: 10.1016/j.canlet.2020.12.022
71. Song Y, Park I, Kim J, Seo HR. Actinomycin D inhibits the expression of the cystine/glutamate transporter xCT via attenuation of CD133 synthesis in CD133+ HCC. Chemico-Biological Interactions. (2019) 309:108713. doi: 10.1016/j.cbi.2019.06.026
72. Hou ZH, Xu XW, Fu XY, Zhou LD, Liu SP, Tan DM. Long non-coding RNA MALAT1 promotes angiogenesis and immunosuppressive properties of HCC cells by sponging miR-140. Am J Physiol Cell Physiol. (2020) 318:C649–63. doi: 10.1152/ajpcell.00510.2018
73. Wang X, Mo X, Yang Z, Zhao C. Qntrolling the LncRNA HULC-Tregs-PD-1 axis inhibits immune escape in the tumor microenvironment. Heliyon. (2024) 10:e28386. doi: 10.1016/j.heliyon.2024.e28386
74. Fujisaka Y, Iwata T, Tamai K, Nakamura M, Mochizuki M, Shibuya R, et al. Long non-coding RNA HOTAIR up-regulates chemokine (C-C motif) ligand 2 and promotes proliferation of macrophages and myeloid-derived suppressor cells in hepatocellular carcinoma cell lines. Oncol Letters. (2018) 15:509–14. doi: 10.3892/ol.2017.7322
75. Zhang J, Pan T, Zhou W, Zhang Y, Xu G, Xu Q, et al. Long noncoding RNA LINC01132 enhances immunosuppression and therapy resistance via NRF1/DPP4 axis in hepatocellular carcinoma. J Exp Clin Cancer Res. (2022) 41:270. doi: 10.1186/s13046-022-02478-z
76. Guo J, Hao C, Wang C, Li L. Long noncoding RNA PVT1 modulates hepatocellular carcinoma cell proliferation and apoptosis by recruiting EZH2. Cancer Cell Int. (2018) 18:98. doi: 10.1186/s12935–018-0582–3
77. Tian X, Wu Y, Yang Y, Wang J, Niu M, Gao S, et al. Long noncoding RNA LINC00662 promotes M2 macrophage polarization and hepatocellular carcinoma progression via activating Wnt/β-catenin signaling. Mol Oncol. (2020) 14:462–83. doi: 10.1002/1878–0261.12606
78. Zhu P, Wang Y, Huang G, Ye B, Liu B, Wu J, et al. lnc-β-Catm elicits EZH2-dependent β-catenin stabilization and sustains liver CSC self-renewal. Nat Struct Mol Biol. (2016) 23:631–9. doi: 10.1038/nsmb.3235
79. Liu J, Fan L, Yu H, Zhang J, He Y, Feng D, et al. Endoplasmic reticulum stress causes liver cancer cells to release exosomal miR-23a-3p and up-regulate programmed death ligand 1 expression in macrophages. Hepatology. (2019) 70:241–58. doi: 10.1002/hep.30607
80. Yin C, Han Q, Xu D, Zheng B, Zhao X, Zhang J. SALL4-mediated upregulation of exosomal miR-146a-5p drives T-cell exhaustion by M2 tumor-associated macrophages in HCC. Oncoimmunology. (2019) 8:1601479. doi: 10.1080/2162402X.2019.1601479
81. Zhao Z, Wu Q, Cheng J, Qiu X, Zhang J, Fan H. Depletion of DNMT3A suppressed cell proliferation and restored PTEN in hepatocellular carcinoma cell. J Biomedicine Biotechnol. (2020) 2010:737535. doi: 10.1155/2010/737535
82. Wang Y, Cao K. KDM1A promotes immunosuppression in hepatocellular carcinoma by regulating PD-L1 through demethylating MEF2D. J Immunol Res. (2021) 2021:9965099. doi: 10.1155/2021/9965099
83. Huang M, Chen C, Geng J, Han D, Wang T, Xie T, et al. Targeting KDM1A attenuates Wnt/β-catenin signaling pathway to eliminate sorafenib-resistant stem-like cells in hepatocellular carcinoma. Cancer Letters. (2017) 398:12–21. doi: 10.1016/j.canlet.2017.03.038
84. Wen J, Xue L, Wei Y, Liang J, Jia W, Yong T, et al. YTHDF2 is a therapeutic target for HCC by suppressing immune evasion and angiogenesis through ETV5/PD-L1/VEGFA axis. Advanced Sci (Weinheim Baden-Wurttemberg Germany). (2024) 11:e202307242. doi: 10.1002/advs.202307242
85. Wang X, Wang J, Tsui YM, Shi C, Wang Y, Zhang X, et al. RALYL increases hepatocellular carcinoma stemness by sustaining the mRNA stability of TGF-β2. Nat Commun. (2021) 12:1518. doi: 10.1038/s41467–021-21828–7
86. Chen W, Hu M, Wei T, Liu Y, Tan T, Zhang C, et al. IL-1 receptor-associated kinase 1 participates in the modulation of the NLRP3 inflammasome by tumor-associated macrophages in hepatocellular carcinoma. J Gastrointestinal Oncol. (2022) 13:1317–29. doi: 10.21037/jgo-22–471
87. Wei Q, Luo SB, He G. Mechanism study of tyrosine phosphatase shp-1 in inhibiting hepatocellular carcinoma progression by regulating the SHP2/GM-CSF pathway in TAMs. Sci Rep. (2024) 14:9128. doi: 10.1038/s41598-024-59725-w
88. Lu Y, Sun Q, Guan Q, Zhang Z, He Q, He J, et al. The XOR-IDH3α axis controls macrophage polarization in hepatocellular carcinoma. J Hepatology. (2023) 79:1172–84. doi: 10.1016/j.jhep.2023.06.022
89. Liu HH, Xu Y, Li CJ, Hsu SJ, Lin XH, Zhang R, et al. An SCD1-dependent mechanoresponsive pathway promotes HCC invasion and metastasis through lipid metabolic reprogramming. Mol Ther. (2022) 30:2554–67. doi: 10.1016/j.ymthe.2022.03.015
90. Xiang W, Lv H, Xing F, Sun X, Ma Y, Wu L, et al. Inhibition of ACLY overcomes cancer immunotherapy resistance via polyunsaturated fatty acids peroxidation and cGAS-STING activation. Sci Adv. (2023) 9:eadi2465. doi: 10.1126/sciadv.adi2465
91. Chen X, Tang Y, Wu D, Li R, Lin Z, Zhou X, et al. From imaging to clinical outcome: dual-region CT radiomics predicting FOXM1 expression and prognosis in hepatocellular carcinoma. Front Oncol. (2023) 13:1278467. doi: 10.3389/fonc.2023.1278467
92. Madden EC, Gorman AM, Logue SE, Samali A. Tumour cell secretome in chemoresistance and tumour recurrence. Trends Cancer. (2020) 6:489–505. doi: 10.1016/j.trecan.2020.02.020
93. Paltridge JL, Belle L, Khew-Goodall Y. The secretome in cancer progression. Biochim Et Biophys Acta (BBA) - Proteins Proteomics. (2013) 1834:2233–41. doi: 10.1016/j.bbapap.2013.03.014
94. Ritchie S, Reed DA, Pereira BA, Timpson P. The cancer cell secretome drives cooperative manipulation of the tumour microenvironment to accelerate tumourigenesis. Faculty Rev. (2021) 10:4. doi: 10.12703/r/10–4
95. Daneshmandi L, Shah S, Jafari T, Bhattacharjee M, Momah D, Saveh-Shemshaki N, et al. Emergence of the stem cell secretome in regenerative engineering. Trends Biotechnol. (2020) 38:1373–84. doi: 10.1016/j.tibtech.2020.04.013
96. Karagiannis GS, Pavlou M, Diamandis EP. Cancer secretomics reveal pathophysiological pathways in cancer molecular oncology. Mol Oncol. (2010) 4:496–510. doi: 10.1016/j.molonc.2010.09.001
97. Kartikasari AER, Huertas CS, Mitchell A, Plebanski M. Tumor-Induced inflammatory cytokines and the emerging diagnostic devices for cancer detection and prognosis. Front Oncol. (2021) 11:692142. doi: 10.3389/fonc.2021.692142
98. Budhu A, Wang XW. The role of cytokines in hepatocellular carcinoma. J Leukocyte Biol. (2006) 80:1197–213. doi: 10.1189/jlb.0506297
99. De Andrés JL, Griñán-Lisón C, Jiménez G, Marchal JA. Cancer stem cell secretome in the tumor microenvironment: a key point for an effective personalized cancer treatment. J Hematol Oncol. (2020) 13:136. doi: 10.1186/s13045–020-00966–3
100. Korkaya H, Liu S, Wicha MS. Regulation of cancer stem cells by cytokine networks: Attacking cancer’s inflammatory roots. Clin Cancer Res. (2011) 17:6125–9. doi: 10.1158/1078–0432.ccr-10–2743
101. Xu M, Zhao Z, Song J, Lan X, Lu S, Chen M, et al. Interactions between interleukin-6 and myeloid-derived suppressor cells drive the chemoresistant phenotype of hepatocellular cancer. Exp Cell Res. (2017) 351:142–9. doi: 10.1016/j.yexcr.2017.01.008
102. Tang KH, Ma S, Lee T, Chan YP, Kwan PS, Tong M, et al. CD133+ liver tumor-initiating cells promote tumor angiogenesis, growth, and self-renewal through neurotensin/interleukin-8/CXCL1 signaling. Hepatology. (2012) 55:807–20. doi: 10.1002/hep.24739
103. Kahraman D, Kahraman T, Cetin-Atalay R. Targeting PI3K/AKT/MTOR pathway identifies differential expression and functional role of IL8 in liver cancer stem cell enrichment. Mol Cancer Ther. (2019) 18:2146–57. doi: 10.1158/1535–7163.mct-19–0004
104. Cheng Y, Ko Y, Ku H, Huang C, Yao Y, Liao Y, et al. Novel paired cell lines for the study of lipid metabolism and cancer stemness of hepatocellular carcinoma. Front Cell Dev Biol. (2022) 10:821224. doi: 10.3389/fcell.2022.821224
105. Allavena P, Digifico E, Belgiovine C. Macrophages and cancer stem cells: a malevolent alliance. Mol Med. (2021) 27:121. doi: 10.1186/s10020–021-00383–3
106. Li H, Wu M, Zhao X. Role of chemokine systems in cancer and inflammatory diseases. MedComm. (2022) 3:e147. doi: 10.1002/mco2.147
107. Xue D, Zheng Y, Wen J, Han J, Tuo H, Liu Y, et al. Role of chemokines in hepatocellular carcinoma (Review). Oncol Rep. (2020) 45:809–23. doi: 10.3892/or.2020.7906
108. Jeng K, Jeng C, Jeng W, Chang C, Sheen I. Role of C-X-C chemokine ligand 12/C-X-C chemokine receptor 4 in the progression of hepatocellular carcinoma. Oncol Letters. (2017) 14:1905–10. doi: 10.3892/ol.2017.6396
109. Zhu L, Zhang W, Wang J, Liu R. Evidence of CD90+CXCR4+ cells as circulating tumor stem cells in hepatocellular carcinoma. Tumor Biol. (2015) 36:5353–60. doi: 10.1007/s13277–015-3196–6
110. Chang Y, Wang Z, Xu X, Wei X, Huang X. Circulating hepatocellular carcinoma cells are characterized by CXCR4 and MMP26. Cell Physiol Biochem. (2015) 36:2393–402. doi: 10.1159/000430201
111. Fortunato O, Belisario DC, Compagno M, Giovinazzo F, Bracci C, Pastorino U, et al. CXCR4 inhibition counteracts immunosuppressive properties of metastatic NSCLC stem cells. Front Immunol. (2020) 11:2168. doi: 10.3389/fimmu.2020.02168
112. Ren Z, Chen Y, Shi L, Shao F, Ge YSJ, Zhang J, et al. Sox9/CXCL5 axis facilitates tumour cell growth and invasion in hepatocellular carcinoma. FEBS J. (2022) 289:3535–49. doi: 10.1111/febs.16357
113. Deng J, Jiang R, Meng E, Wu H. CXCL5: A coachman to drive cancer progression. Front Oncol. (2022) 12:944494. doi: 10.3389/fonc.2022.944494
114. Ma Y, Lu Z, Zhao C, Zhang Y, Xu X, Wang X, et al. Interferon-α induces differentiation of cancer stem cells and immunosuppression in hepatocellular carcinoma by upregulating CXCL8 secretion. Cytokine. (2024) 177:156555. doi: 10.1016/j.cyto.2024.156555
115. Mojić M, Takeda K, Hayakawa Y. The dark side of IFN-γ: its role in promoting cancer immunoevasion. Int J Mol Sci. (2017) 19:89. doi: 10.3390/ijms19010089
116. Li J, Chen JN, Zeng T, He F, Chen SP, Ma S, et al. CD133+ liver cancer stem cells resist interferon-gamma-induced autophagy. BMC Cancer. (2016) 16:15. doi: 10.1186/s12885–016-2050–6
117. Li T, Huang HY, Qian B, Wang WH, Yuan Q, Zhang HY, et al. Interventing mitochondrial PD-L1 suppressed IFN-γ-induced cancer stemness in hepatocellular carcinoma by sensitizing sorafenib-induced ferroptosis. Free Radical Biol Med. (2024) 212:360–74. doi: 10.1016/j.freeradbiomed.2023.12.034
118. Jing Y, Sun K, Liu W, Sheng D, Zhao S, Gao L, et al. Tumor necrosis factor-α promotes hepatocellular carcinogenesis through the activation of hepatic progenitor cells. Cancer Letters. (2018) 434:22–32. doi: 10.1016/j.canlet.2018.07.001
119. Tan W, Luo X, Li W, Zhong J, Cao J, Zhu S, et al. TNF-α is a potential therapeutic target to overcome sorafenib resistance in hepatocellular carcinoma. EBioMedicine. (2019) 40:446–56. doi: 10.1016/j.ebiom.2018.12.047
120. Álvarez-Mercado AI, Caballeria-Casals A, Rojano-Alfonso C, Chávez-Reyes J, Micó-Carnero M, Sánchez-González A, et al. Insights into growth factors in liver carcinogenesis and regeneration: An ongoing debate on minimizing cancer recurrence after liver resection. Biomedicines. (2021) 9:1158. doi: 10.3390/biomedicines9091158
121. Tan L, Chen S, Wei G, Li Y, Liao J, Jin H, et al. Sublethal heat treatment of hepatocellular carcinoma promotes intrahepatic metastasis and stemness in a VEGFR1-dependent manner. Cancer Letters. (2019) 460:29–40. doi: 10.1016/j.canlet.2019.05.041
122. Tovar V, Cornellà H, Moeini A, Vidal SJ, Hoshida Y, Sia D, et al. Tumour initiating cells and IGF/FGF signalling contribute to sorafenib resistance in hepatocellular carcinoma. Gut. (2015) 66:530–40. doi: 10.1136/gutjnl-2015–309501
123. Bilbao D, Luciani L, Jóhannesson B, Piszczek A, Rosenthal N. Insulin-like growth factor-1 stimulates regulatory T cells and suppresses autoimmune disease. EMBO Mol Med. (2014) 6:1423–35. doi: 10.15252/emmm.201303376
124. Salminen A, Kaarniranta K, Kauppinen A. Insulin/IGF-1 signaling promotes immunosuppression via the STAT3 pathway: impact on the aging process and age-related diseases. Inflammation Res. (2021) 70:1043–61. doi: 10.1007/s00011–021-01498–3
125. Mossahebi-Mohammadi M, Quan M, Zhang JS, Li X. FGF signaling pathway: a key regulator of stem cell pluripotency. Front Cell Dev Biol. (2020) 8:79. doi: 10.3389/fcell.2020.00079
126. Hu C, Qiao W, Li X, Ning Z, Liu J, Dalangood S, et al. Tumor-secreted FGF21 acts as an immune suppressor by rewiring cholesterol metabolism of CD8+T cells. Cell Metab. (2024) 36:630–47. doi: 10.1016/j.cmet.2024.01.005
127. Tai YL, Chen KC, Hsieh JT, Shen TL. Exosomes in cancer development and clinical applications. Cancer Science. (2018) 109:2364–74. doi: 10.1111/cas.13697
128. Li X, Li X, Zhang B, He B. The role of Cancer Stem Cell-Derived exosomes in cancer progression. Stem Cells Int. (2022) 2022:9133658. doi: 10.1155/2022/9133658
129. Huang H, Hou J, Liu K, Liu Q, Shen L, Liu B, et al. RAB27A-dependent release of exosomes by liver cancer stem cells induces Nanog expression in their differentiated progenies and confers regorafenib resistance. J Gastroenterol Hepatology. (2021) 36:3429–37. doi: 10.1111/jgh.15619
130. Gawlik-Rzemieniewska N, Bednarek I. The role of NANOG transcriptional factor in the development of Malignant phenotype of cancer cells. Cancer Biol Ther. (2016) 17:1–10. doi: 10.1080/15384047.2015.1121348
131. Saga K, Park J, Nimura K, Kawamura N, Ishibashi A, Nonomura N, et al. NANOG helps cancer cells escape NK cell attack by downregulating ICAM1 during tumorigenesis. J Exp Clin Cancer Res. (2019) 38:416. doi: 10.1186/s13046-019-1429-z
132. Yang Q, Liang Y, Shi Y, Shang J, Huang X. The ALKBH5/SOX4 axis promotes liver cancer stem cell properties via activating the SHH signaling pathway. J Cancer Res Clin Oncol. (2023) 149:15499–510. doi: 10.1007/s00432–023-05309–6
133. Alzahrani F, El-Magd MA, Abdelfattah-Hassan A, Saleh AA, Saadeldin IM, El-Shetry ES, et al. Potential effect of exosomes derived from cancer stem cells and MSCs on progression of DEN-induced HCC in rats. Stem Cells Int. (2018) 2018:1–17. doi: 10.1155/2018/8058979
134. Tsunedomi R, Yoshimura K, Kimura Y, Nishiyama M, Fujiwara N, Matsukuma S, et al. Elevated expression of RAB3B plays important roles in chemoresistance and metastatic potential of hepatoma cells. BMC Cancer. (2022) 22:260. doi: 10.1186/s12885–022-09370–1
135. Conigliaro A, Costa V, Lo Dico A, Saieva L, Buccheri S, Dieli F, et al. CD90+ liver cancer cells modulate endothelial cell phenotype through the release of exosomes containing H19 lncRNA. Mol Cancer. (2015) 14:155. doi: 10.1186/s12943-015-0426-x
136. Sun L, Li J, Yan W, Yao Z, Wang R, Zhou X, et al. H19 promotes aerobic glycolysis, proliferation, and immune escape of gastric cancer cells through the microRNA-519d-3p/lactate dehydrogenase A axis. Cancer Science. (2021) 112:2245–59. doi: 10.1111/cas.14896
137. Han T, Chen L, Li K, Hu Q, Zhang Y, Xuan Y, et al. Significant CircRNAs in liver cancer stem cell exosomes: mediator of Malignant propagation in liver cancer? Mol Cancer. (2023) 22:197. doi: 10.1186/s12943-023-01891-y
138. Ferraro F, Celso CL, Scadden D. Adult stem Cels and their niches. Adv Exp Med Biol. (2010) 695:155–68. doi: 10.1007/978–1-4419–7037-4_11
139. Li Y, Wang R, Xiong S, Wang X, Zhao Z, Bai S, et al. Cancer-associated fibroblasts promote the stemness of CD24+ liver cells via paracrine signaling. J Mol Med. (2018) 97:243–55. doi: 10.1007/s00109–018-1731–9
140. Lau EY, Lo J, Cheng BY, Ma MK, Lee JM, Ng JK, et al. Cancer-associated fibroblasts regulate tumor-initiating cell plasticity in hepatocellular carcinoma through c-Met/FRA1/HEY1 signaling. Cell Rep. (2016) 15:1175–89. doi: 10.1016/j.celrep.2016.04.019
141. Peng H, Xue R, Zheng J, Qiu J, Wang J, Yan W, et al. Cancer-associated fibroblasts enhance the chemoresistance of CD73+ hepatocellular carcinoma cancer cells via HGF-Met-ERK1/2 pathway. Ann Trans Med. (2020) 8:856. doi: 10.21037/atm-20–1038
142. Yan X, Zhang D, Wu W, Wu S, Qian J, Hao Y, et al. Mesenchymal stem cells promote hepatocarcinogenesis via lncRNA–MUF interaction with ANXA2 and miR-34a. Cancer Res. (2017) 77:6704–16. doi: 10.1158/0008–5472.can-17–1915
143. Fan Q, Jing Y, Yu G, Kou X, Ye F, Gao L, et al. Tumor-associated macrophages promote cancer stem cell-like properties via transforming growth factor-beta1-induced epithelial–mesenchymal transition in hepatocellular carcinoma. Cancer Letters. (2014) 352:160–8. doi: 10.1016/j.canlet.2014.05.008
144. Wang HC, Haung LY, Wang CJ, Chao YJ, Hou YC, Yen CJ, et al. Tumor-associated macrophages promote resistance of hepatocellular carcinoma cells against sorafenib by activating CXCR2 signaling. J Biomed Science. (2022) 29:99. doi: 10.1186/s12929–022-00881–4
145. Chen Y, Wen H, Zhou C, Su Q, Lin Y, Xie Y, et al. TNF-α derived from M2 tumor-associated macrophages promotes epithelial-mesenchymal transition and cancer stemness through the Wnt/β-catenin pathway in SMMC-7721 hepatocellular carcinoma cells. Exp Cell Res. (2019) 378:41–50. doi: 10.1016/j.yexcr.2019.03.005
146. Wan S, Zhao E, Kryczek I, Vatan L, Sadovskaya A, Ludema G, et al. Tumor-Associated macrophages produce interleukin 6 and signal via STAT3 to promote expansion of human hepatocellular carcinoma stem cells. Gastroenterology. (2014) 147:1393–404. doi: 10.1053/j.gastro.2014.08.039
147. Lu J, Luo Y, Rao D, Wang T, Lei Z, Chen X, et al. Myeloid-derived suppressor cells in cancer: therapeutic targets to overcome tumor immune evasion. Exp Hematol Oncol. (2024) 13:39. doi: 10.1186/s40164–024-00505–7
148. Zhang C, Sui Y, Liu S, Yang M. The role of myeloid-derived suppressor cells in liver disease. Biomedicines. (2024) 12:299. doi: 10.3390/biomedicines12020299
149. Gabrilovich DI, Nagaraj S. Myeloid-derived suppressor cells as regulators of the immune system. Nat Rev Immunol. (2009) 9:162–74. doi: 10.1038/nri2506
150. Iwata T, Kondo Y, Kimura O, Morosawa T, Fujisaka Y, Umetsu T, et al. PD-L1+MDSCs are increased in HCC patients and induced by soluble factor in the tumor microenvironment. Sci Rep. (2016) 6:39296. doi: 10.1038/srep39296
151. Zhou J, Liu M, Sun H, Feng Y, Xu L, Chan AWH, et al. Hepatoma-intrinsic CCRK inhibition diminishes myeloid-derived suppressor cell immunosuppression and enhances immune-checkpoint blockade efficacy. Gut. (2018) 67:931–44. doi: 10.1136/gutjnl-2017–314032
152. Lin D, Mei Y, Lei L, Hanafi ZB, Jin Z, Liu Y, et al. Immune suppressive function of IL-1α release in the tumor microenvironment regulated by calpain 1. OncoImmunology. (2022) 11:2088467. doi: 10.1080/2162402X.2022.2088467
153. He Q, Liu M, Huang W, Chen X, Zhang B, Zhang T, et al. IL-1β-induced elevation of solute carrier family 7 member 11 promotes hepatocellular carcinoma metastasis through up-regulating programmed death ligand 1 and colony-stimulating factor 1. Hepatology. (2021) 74:3174–93. doi: 10.1002/hep.32062
154. Chiu DKC, Tse APW, Xu IMJ, Cui JD, Lai RKH, Li LL, et al. Hypoxia inducible factor HIF-1 promotes myeloid-derived suppressor cells accumulation through ENTPD2/CD39L1 in hepatocellular carcinoma. Nat Commun. (2017) 8:517. doi: 10.1038/s41467–017-00530–7
155. Ma C, Zhang Q, Greten TF. MDSCs in liver cancer: a critical tumor-promoting player and a potential therapeutic target. Cell Immunol. (2021) 361:104295. doi: 10.1016/j.cellimm.2021.104295
156. Zhu GQ, Tang Z, Huang R, Qu WF, Fang Y, Yang R, et al. CD36+ cancer-associated fibroblasts provide immunosuppressive microenvironment for hepatocellular carcinoma via secretion of macrophage migration inhibitory factor. Cell Discovery. (2023) 9:25. doi: 10.1038/s41421-023-00529-z
157. Zhang F, Hu K, Liu W, Quan B, Li M, Lu S, et al. Oxaliplatin-resistant hepatocellular carcinoma drives immune evasion through PD-L1 up-regulation and PMN-singular recruitment. Cell Mol Gastroenterol Hepatology. (2023) 15:573–91. doi: 10.1016/j.jcmgh.2022.12.002
158. Jin S, Yang Z, Hao X, Tang W, Ma W, Zong H. Roles of HMGB1 in regulating myeloid-derived suppressor cells in the tumor microenvironment. biomark Res. (2020) 8:2021. doi: 10.1186/s40364–020-00201–8
159. Kapanadze T, Gamrekelashvili J, Ma C, Chan C, Zhao F, Hewitt S, et al. Regulation of accumulation and function of myeloid derived suppressor cells in different murine models of hepatocellular carcinoma. J Hepatology. (2013) 59:1007–13. doi: 10.1016/j.jhep.2013.06.010
160. US National Library of Medicine. Nivolumab combined with BMS-986253 in HCC patients. ClinicalTrials.gov. Bethesda, Maryland. (2019). Available at: https://www.clinicaltrials.gov/study/NCT04050462.
161. US National Library of Medicine. Neoadjuvant nivolumab with CCR2/5-inhibitor or anti-IL-8) for non-small cell lung cancer (NSCLC) or hepatocellular carcinoma (HCC). ClinicalTrials.gov. Bethesda, Maryland. (2020). Available at: https://www.clinicaltrials.gov/study/NCT04123379.
162. US National Library of Medicine. HuMax-IL8 (interleukin8) in patients with advanced Malignant solid tumors. ClinicalTrials.gov. Bethesda, Maryland. (2015). Available at: https://www.clinicaltrials.gov/study/NCT02536469.
163. US National Library of Medicine. Study of CHS-388 (formerly known as SRF388) in patients with advanced solid tumors. ClinicalTrials.gov (2020). Available at: https://www.clinicaltrials.gov/study/NCT04374877.
164. Liang L, Zhao L, Wang Y, Wang Y. Treatment for hepatocellular carcinoma is enhanced when norcantharidin is encapsulated in exosomes derived from bone marrow mesenchymal stem cells. Mol Pharmaceutics. (2021) 18:1003–13. doi: 10.1021/acs.molpharmaceut.0c00976
165. Zou Z, Lin Z, Wu C, Tan J, Zhang J, Peng Y, et al. Micro-engineered organoid-on-a-chip based on mesenchymal stromal cells to predict immunotherapy responses of HCC patients. Advanced Science. (2023) 10:e2302640. doi: 10.1002/advs.202302640
166. Al-Gayyar MM, Alattar A, Alshaman R, Hamdan AM. QNZ alleviated hepatocellular carcinoma by targeting inflammatory pathways in a rat model. Cytokine. (2021) 148:155710. doi: 10.1016/j.cyto.2021.155710
167. US National Library of Medicine. A Study of Galunisertib (LY2157299) in Combination with nivolumab in advanced refractory solid tumors and in recurrent or refractory NSCLC, or hepatocellular carcinoma. ClinicalTrials.gov. Bethesda, Maryland. (2015). Available at: https://www.clinicaltrials.gov/study/NCT02423343.
168. Chen Y, Ramjiawan RR, Reiberger T, Ng MR, Hato T, Huang Y, et al. CXCR4 inhibition in tumor microenvironment facilitates anti-programmed death receptor-1 immunotherapy in sorafenib-treated hepatocellular carcinoma in mice. Hepatology. (2015) 61:1591–602. doi: 10.1002/hep.27665
169. Lei H, Huang B, Cai J, Li C, Shang C, Liao Z, et al. CXCR4 antagonist AMD3100 enhances therapeutic efficacy of transcatheter arterial chemoembolization in rats with hepatocellular carcinoma. Kaohsiung J Med Sci. (2022) 38:781–9. doi: 10.1002/kjm2.12540
170. Song J, Chang C, Wu C, Dinh TK, Jan J, Huang K, et al. A highly selective and potent CXCR4 antagonist for hepatocellular carcinoma treatment. Proc Natl Acad Sci United States America. (2021) 118:e2015433118. doi: 10.1073/pnas.2015433118
171. Wang Y, Wang Z, Jia F, Xu Q, Shu Z, Deng J, et al. CXCR4-guided liposomes regulating hypoxic and immunosuppressive microenvironment for sorafenib-resistant tumor treatment. Bioactive Materials. (2022) 17:147–61. doi: 10.1016/j.bioactmat.2022.01.003
172. Xiao Y, Chen J, Zhou H, Zeng X, Ruan Z, Pu Z, et al. Combining p53 mRNA nanotherapy with immune checkpoint blockade reprograms the immune microenvironment for effective cancer therapy. Nat Commun. (2022) 13:758. doi: 10.1038/s41467–022-28279–8
173. Gao D, Lin T, Sung Y, Liu YC, Chiang W, Chang C, et al. CXCR4-targeted lipid-coated PLGA nanoparticles deliver sorafenib and overcome acquired drug resistance in liver cancer. Biomaterials. (2015) 67:194–203. doi: 10.1016/j.biomaterials.2015.07.035
174. Zheng N, Liu W, Li B, Nie H, Liu J, Cheng Y, et al. Co-delivery of sorafenib and metapristone encapsulated by CXCR4-targeted PLGA-PEG nanoparticles overcomes hepatocellular carcinoma resistance to sorafenib. J Exp Clin Cancer Res. (2019) 38:232. doi: 10.1186/s13046-019-1216-x
175. Gao Y, Li W, Liu R, Guo Q, Li J, Bao Y, et al. Norcantharidin inhibits IL-6-induced epithelial-mesenchymal transition via the JAK2/STAT3/TWIST signaling pathway in hepatocellular carcinoma cells. Oncol Rep. (2017) 38:1224–32. doi: 10.3892/or.2017.5775
Keywords: cancer stem cell (CSC), hepatocellular carcinoma, immunosuppression, secretory factors, tumor microenvironment
Citation: Muliawan GK and Lee TK-W (2024) The roles of cancer stem cell-derived secretory factors in shaping the immunosuppressive tumor microenvironment in hepatocellular carcinoma. Front. Immunol. 15:1400112. doi: 10.3389/fimmu.2024.1400112
Received: 13 March 2024; Accepted: 15 May 2024;
Published: 29 May 2024.
Edited by:
Rosanna Paciucci, Vall d’Hebron University Hospital, SpainReviewed by:
Zhenbo Tu, Beth Israel Deaconess Medical Center, United StatesSaptak Banerjee, Chittaranjan National Cancer Institute (CNCI), India
Copyright © 2024 Muliawan and Lee. This is an open-access article distributed under the terms of the Creative Commons Attribution License (CC BY). The use, distribution or reproduction in other forums is permitted, provided the original author(s) and the copyright owner(s) are credited and that the original publication in this journal is cited, in accordance with accepted academic practice. No use, distribution or reproduction is permitted which does not comply with these terms.
*Correspondence: Terence Kin-Wah Lee, terence.kw.lee@polyu.edu.hk