- 1Department of General Surgery (Breast and Thyroid Surgery), Shaoxing People’s Hospital; Shaoxing Hospital, Zhejiang University School of Medicine, Zhejiang, China
- 2Department of Gynecology, The Second Affiliated Hospital of Zhejiang University School of Medicine, Hangzhou, China
- 3Department of Oncology, The Second Affiliated Hospital of Anhui Medical University, Hefei, Anhui, China
- 4Department of Oncology, Anhui Medical University, Hefei, Anhui, China
- 5School of Medicine, Shaoxing University, Zhejiang, China
- 6Department of General Surgery (Breast and Thyroid Surgery), Shaoxing People’s Hospital, Shaoxing Hospital, Zhejiang University School of Medicine, Shaoxing, Zhejiang, China
- 7Key Laboratory of Cancer Prevention and Intervention, Ministry of Education, Hangzhou, Zhejiang, China
The tumor microenvironment is closely linked to the initiation, promotion, and progression of solid tumors. Among its constitutions, immunologic cells emerge as critical players, facilitating immune evasion and tumor progression. Apart from their indirect impact on anti-tumor immunity, immunocytes directly influence neoplastic cells, either bolstering or impeding tumor advancement. However, current therapeutic modalities aimed at alleviating immunosuppression from regulatory cells on effector immune cell populations may not consistently yield satisfactory results in various solid tumors, such as breast carcinoma, colorectal cancer, etc. Therefore, this review outlines and summarizes the direct, dualistic effects of immunocytes such as T cells, innate lymphoid cells, B cells, eosinophils, and tumor-associated macrophages on tumor cells within the tumor microenvironment. The review also delves into the underlying mechanisms involved and presents the outcomes of clinical trials based on these direct effects, aiming to propose innovative and efficacious therapeutic strategies for addressing solid tumors.
1 Introduction
The tumor microenvironment (TME), consisting of immunocytes, stromal cells, extracellular matrix (ECM), and blood and lymphatic vascular networks, forms a complex immunomodulatory network (1, 2). In recent years, attention has been focused on understanding how immune cells, stromal cells, and cytokines regulate tumor cell proliferation, growth, metastasis, and invasion within the TME (3–5). Rather than functioning in isolation, these components of the TME synergistically interact to form an integrated entity (1). What’s more, disruptions in any part of this network may significantly impact overall tumor behavior. Therefore, a comprehensive grasp of the intricate dynamics inherent in the TME is imperative for forming efficacious cancer therapies.
Even more noteworthy is that immune cells, as a critical component in the TME, significantly contribute to maintaining human health. They play a pivotal role in recognizing, targeting, and eliminating mutated cells within the body (6, 7). This function is not only achieved through indirect pathways, such as adjusting the functionality and differentiation of other cells through the secretion of cytokines, but also through directly influencing the survival and subsequent progression of tumor cells (8). In contrast to the complexities inherent in indirect actions and the multifaceted interplay of reciprocal regulations, immunocytes’ direct cytotoxic effects offer a clear and unequivocal avenue for tumor treatment (9, 10). These direct interactions usually remain unaffected by intermediate multi-step modulations, resulting in potent cytotoxicity or significant direct promoting effects (11). However, this dual nature complicates immune therapy, closely tying it to the current challenge of achieving effective treatment for certain malignancy (12).
Therefore, we discuss the direct interplay between various immunocytes and neoplastic cells, coupled with an ensuing discourse on related treatments and clinical applications, alongside the extant obstacles, which may be beneficial for further research.
2 Direct cytotoxic effects of immune cells and their counteractions
2.1 T cells
2.1.1 CD4+ T cells
The differentiation process of CD4+ T cells is governed by multiple factors, including antigen-specific stimulation, T cell receptors(TCR), cytokines, and transcription factors (13). Initially, upon detection of “non-self” or foreign substances by the immune system, antigens are presented to CD4+ T cells via the TCR, initiating the differentiation process (14). The type of antigen presenting cell(APC) determines the antigen type, while its affinity and quantity influence the nature and strength of TCR signaling, collectively regulating the activation and differentiation of CD4+ T cells in conjunction with co-stimulatory molecules (13, 14).
Subsequently, cytokines secreted by antigen-presenting cells and differentiated CD4+ T cells play crucial roles in differentiation. For instance, interleukin-12(IL-12) and interferon-gamma(IFN-γ) promote type 1helper T(Th1) cell differentiation (15, 16), IL-4 induces Th2 cell differentiation (17), IL-4 and transforming growth factor beta(TGF-β) enhance Th9 cell differentiation (18, 19), and IL-6 and TGF-β drive Th17 cell differentiation (20). These cytokines activate distinct signaling pathways, guiding the formation of specific T cell subgroups (13).
Finally, under the regulation of specific cytokine signals and cellular environments, master transcription factors contribute to shaping and maintaining the balance and diversity of the immune system by activating specific gene expression patterns (16). Each T cell subset is governed by lineage-specific master transcription factors, such as T-bet, GATA binding protein 3(GATA3), interferon regulatory factor 4(IRF4), and retinoid-related orphan receptor gamma t(RORγt), which control the expression of subset-specific genes, thereby determining the direction of cell differentiation (18, 21–24). Therefore, the variegated landscape of the tumor microenvironment impels T cells toward distinct subtypes, underscoring the critical importance of the types and functional states of T cell subtypes in shaping the immune response to tumors (13).
2.1.1.1 Th1 cells
Historically, CD4+ T cells have been construed as orchestrators of immune responses, activating and recruiting other immune cells by producing their distinctive cytokines (25). In contrast, CD8+ T cells are intricately associated with the direct elimination of target cells (26). Nevertheless, recent years have witnessed an in-depth exploration of the intricacies of CD4+ T cell functionality, particularly those cells exhibiting antigen-specific cytotoxic activity, denoted as CD4+ cytotoxic T lymphocytes (CTLs) (27).
These CD4+ T lymphocytes have been demonstrated to elicit cytotoxic effects on tumor cells by directly releasing granule enzymes (28–31). Additionally, they have been validated to implement cytotoxic responses in solid tumors such as melanoma and lymphoma through mediation of the factor associated suicide/factor associated suicide ligand(Fas/FasL) and tumor necrosis associated apoptosis-inducing ligand(TRAIL) pathways (32–34). While in the initial phases, CD4+ CTLs were erroneously classified within the Th1 cell subset (25). However, lamentably, there is a dearth of conclusive evidence substantiating the assertion that Th1 cells can induce direct cytotoxicity against tumor cells through the three pathways above.
However, Th1 cells efficaciously manifest their anticancer prowess through the secretion of IFN-γ (Figure 1). Primarily, the IFN-γ orchestrates a reduction in the envelopment of peripheral tumor cells, facilitating the aberrant genesis of vasculature and the regularization of vascular architecture, thereby impeding the proliferation of tumor vasculature (35). These actions possess the potential to perturb the oxygenic and nutritive milieu within the TME, ultimately precipitating the demise of neoplastic cells (36). Additionally, the impact of IFN-γ extends through the orchestrated proteasomal degradation of the human epidermal growth factor receptor 2(HER2) membrane receptor, mediated by the E3 ubiquitin ligase cullin-5, inducing the senescence of tumor cells in breast cancer (37). According to the latest pancreatic cancer study, the collaboration of Th1 cell-derived IFN-γ with tumor necrosis factor(TNF) triggers a state of enduring growth arrest in the G1/G0 phase, activates p16 the inhibitor of cyclin-dependent kinase 4a(p16INK4a), and instigates downstream hypophosphorylation of the Rb protein at serine residues, thereby effectuating the senescence of β-pancreatic cancer cells (38) (Figure 1).
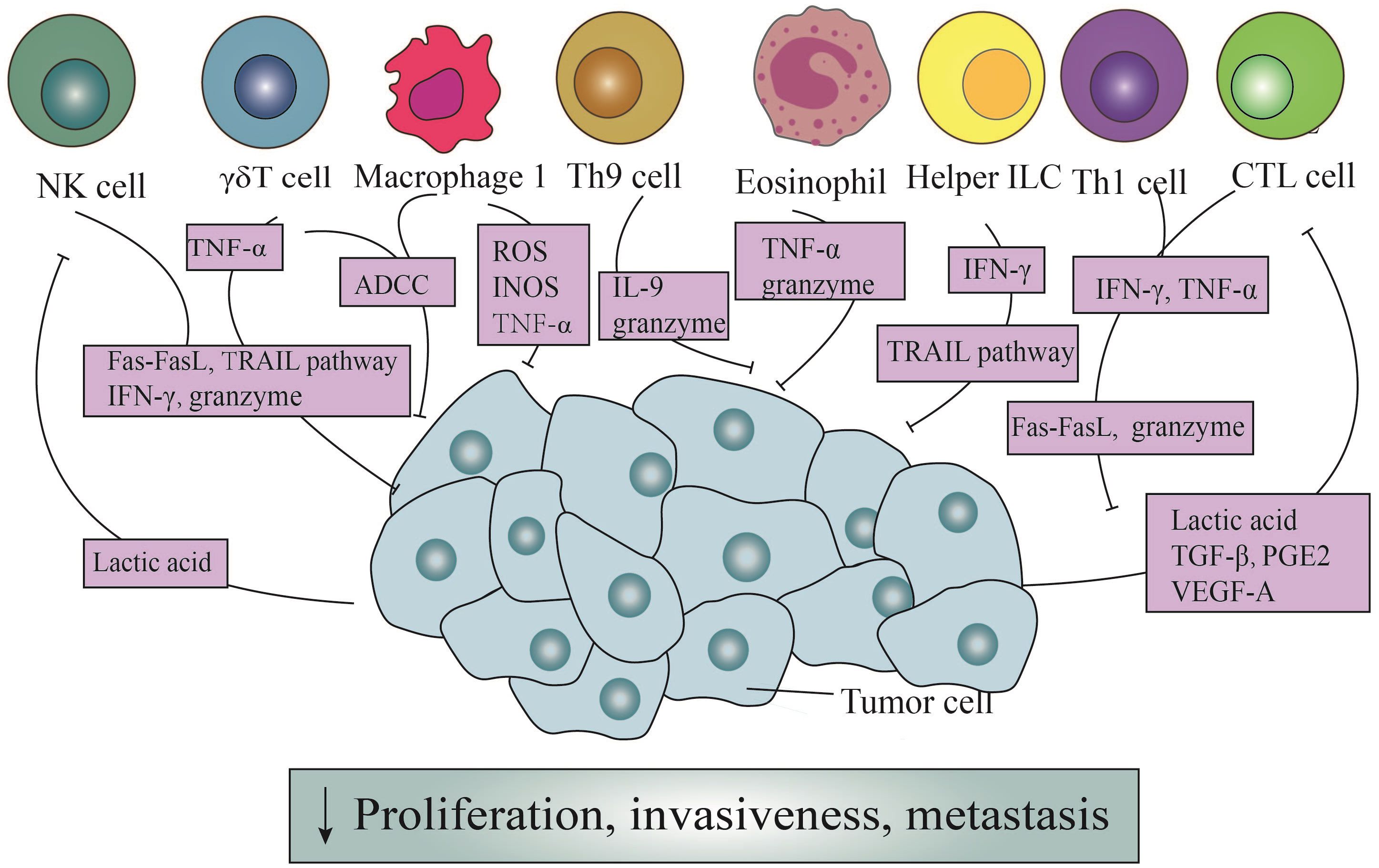
Figure 1 The direct antitumor action of immune cells and the counteraction of tumor cells. Through the Fas/Fasl pathway, ADDC pathway, and TRAIL pathway, immune cells exert direct cytotoxic effects on tumor cells. Simultaneously, they can release granule enzymes, IFN-γ, TNF-α, ROS, INOS, and other mediators to generate cytotoxicity. In addition, Th9 cells induce apoptosis in tumor cells by releasing IL-9. It is noteworthy that tumor cells, in turn, enhance the cytotoxicity of NK cells and CTL cells through secretion lactic acid, TGF-β, PGE2, and VEGF-A.
2.1.1.2 Th9 cells
In the presence of IL-4 and TGF-β1, naïve CD4+ T cells exhibit the capacity to differentiate into a distinct subset known as Th9 cells (39). These cells possess the ability to generate IL-9, a cytokine initially proposed to be involved in promoting tumorigenesis (39). However, subsequent investigations have revealed its anti-tumor effects. Purwar et al. pioneered the demonstration of Th9 cells’ efficacy in suppressing melanoma growth upon injection into murine hosts, outperforming the effects of Th1, Th2, and Th17 cells (40). The heightened efficiency in inducing tumor cell apoptosis was intricately associated with the elevated expression of granzyme B, the blockade of which markedly mitigated the cytotoxic effects (23, 40) (Figure 1). Further elucidation of Th9 cells unveils their proficiency in eradicating advanced tumors. Studies emphasize Eomesodermin as a principal regulatory factor governing the expression of cytotoxic enzymes. Augmentation of Eomesodermin coincides with an increase in the gene expression of the cytotoxic enzyme repertoire within Th9 cells (41).
In addition, IL-9 is critical for suppressing tumor growth (42) (Figure 1). In murine melanoma experiments, the increase in both the quantity of Th9 cells and IL-9 significantly reduces the tumor growth rate, despite in vitro studies demonstrating a close association with their indirect effects (43). Another study centered around HTB-72 and SK-Mel-5 melanoma cells has established a link between the anti-proliferative effects of IL-9 and the heightened expression of p21 (44). Besides, a discernibly elevated count of apoptotic cells following IL-9 treatment has been observed compared to when contrasted with the control group, further validating their conclusion (44).
2.1.2 CD8+ T cells
Under antigen stimulation, naïve CD8+ T cells generate effector and memory T cells, with the effector CD8+ T cells referred to as CD8+ CTLs (13). CTLs assume a pivotal role in the vigilant immune surveillance against neoplastic entities, recognizing cell surface antigens on tumor cells through the discerning receptors of the TCR (44, 45). The primary mechanisms through which CTLs coordinate their anti-tumor capabilities involve the granzyme/perforin pathway and cytotoxicity mediated by Fas receptors (46). The granzyme/perforin cascade involves the liberation of granules containing granzymes and perforin, thereby directly instigating apoptotic cascades within targeted cellular domains (47–49). Usually, perforin serves as the conduit for ingressing granzymes into tumor cells, thereby facilitating the demise of targeted cells (50). Therefore, the absence or impairment of perforin may diminish the tumor-suppressive efficacy of CTLs (47). In the latest literature, it has been discovered that endosomal sorting complexes required for transport can repair the plasma membrane pores caused by perforin. This rehabilitative action restores membrane integrity, effectively preventing the invasion of granzymes (51) (Figure 1).
In instances where the integrity of the granzyme/perforin pathway is compromised, there emerges a heightened prominence of Fas-mediated processes (46) (Figure 1). FasL triggers apoptosis through the intricate activation of caspases (52). Nonetheless, noteworthy observations posit that FasL expressed by exosomes might exert divergent effects, potentially fostering tumor invasion instead of inducing apoptotic signals (53).
Furthermore, CTLs also possess the capability to modulate the metabolic dynamics of neoplastic cells through the secretion of cytokines (Figure 1). Notably, factors such as IFN-γ, originating from CTLs, downregulate certain components of the glutamate-cystine antiporter system, subsequently influencing lipid metabolism within the tumor cell milieu and promoting tumor cells’ apoptosis (54, 55). Another secreted product, TNF-α, despite its potential derivation from various immune cells, undeniably plays a role in inducing the rupture of tumor blood vessels, promoting cell infiltration, and maintaining an ischemic state in tumors (56) (Figure 1). However, under typical circumstances, the contact of individual CTLs with tumor cells fails to eliminate the tumor cells effectively. And it is only through sequential interactions with multiple CTLs that elimination occurs (57).
In addition, tumor cells heavily impact the function of CTLs (Figure 1). Tumor-derived lactic acid efficaciously reduces the activity of monocarboxylate transporter -1, which weakens cellular metabolism and diminishes the cytotoxicity of IFN-γ, granzymes, and perforin in CTLs (58). Moreover, the secretion of TGF-β by tumor cells directly impedes the immune activity of CTLs by inducing the upregulation of miR-23a and simultaneous downregulation of B-lymphocyte-induced maturation protein 1(Blimp-1) (59). Notably, Blimp-1, as a pivotal transcriptional repressor, plays a fundamental role in the differentiation and memory response of effector CD8+ T cells (60). Consequently, this mechanism undermines the immune function mediated by CTLs. Tumor cells also induce the expression of FasL in endothelial cells via vascular endothelial growth factor-A (VEGF-A), IL-10, and prostaglandin E2 (PGE2), thereby eliciting specific cytotoxic effects in effector T cells (61).
2.1.3 Gammadelta T cells (γδT cells)
γδT cells and αβT cells are the two main types of T cells within the adaptive immune system. γδT cells have T-cell receptors composed of γ and δ chains and recognize a broader range of antigens, while αβT cells bear T-cell receptors made of α and β chains and primarily respond to peptide antigens presented by major histocompatibility complex (MHC) molecules (62).
Despite being a minority among peripheral blood cells, γδT cells assume a pivotal role in the detection and eradication of tumor cells (63). In a previous study focused on squamous cell carcinoma of the head and neck (SCCHN), it was observed CD56+ γδT cells, isolated from peripheral blood mononuclear cells (PBMCs) expanded under the stimulation of isopentenyl pyrophosphate (IPP) and IL-2, could effectively destroy SCCHN cell lines in a dose-dependent manner, in contrast to CD56- γδT cells (64). What’s more, the cytotoxicity of γδT cells underwent a notable suppression following treatment with concanamycin A (CMA), an inhibitor of the granzyme/perforin pathway (64), which concurrently functions as a downregulator (65). Additionally, γδT cells also exhibit a lytic effect on MCF-7 breast tumor cells. Subsequent research revealed that MCF-7 tumor cells were surrounded by a substantial number of γδT cells, forming a tight conjugate, and were subsequently eliminated within a span of ten seconds. Furthermore, γδT cells were empirically demonstrated to possess the capability to lyse autologous primary tumor kidney cells, a phenomenon alleviated upon the application of CMA (66). From the above, it can be deduced that the perforin/granzyme pathway occupies an irreplaceable position in the cytotoxic activity of γδT cells (67) (Figure 1).
Antibody-dependent cell-mediated cytotoxicity (ADCC) constitutes another crucial mechanism (68) (Figure 1). Classified by their maturation levels, γδT cells categorize into four functionally distinct subpopulations: naïve γδT cells, central memory γδT cells, effector memory γδT cells, and terminally differentiated effector memory γδT cells (69). The latter two subpopulations express CD16, a surface receptor that efficiently facilitates tumor cell killing, even in the absence of antibody engagement (68, 70). Vγ9Vδ2 T cells, isolated from PBMCs of healthy donors, undergo activation, leading to the expression of CD16, a phenomenon not observed in their unstimulated counterparts (71). Furthermore, when TCR-activated Vγ9Vδ2 T cells are cross-linked to plastic wells with anti-CD16 monoclonal antibodies, substantial TNF-α production occurs, a response mitigated by the addition of soluble anti-CD16 monoclonal antibodies (71).
The cytotoxic activity of γδT cells is also ascribed to the expression of TRAIL and FasL, which bind to corresponding receptors on tumor cells (72, 73) (Figure 1). TRAIL’s interaction with different receptors produces varied outcomes: knockdown of TRAIL-Receptor 4(TRAIL-R4) in Colo357 and MDA-MB-231 cells significantly reduces sensitivity to γδT cell-induced cytotoxicity, whereas TRAIL-R4 knock-in HeLa cells show reinforced cytotoxicity (74). Furthermore, serum TRAIL levels hold clinical significance, as evidenced in a study involving eighteen patients with refractory prostate cancer, where higher serum TRAIL levels at nine months correlated with improved clinical outcomes (75). Additionally, the upregulation of Fas on the surface of osteosarcoma cells effectively increases the cytotoxicity of γδT cells (76).
Finally, γδT cells serves as potent producers of IFN-γ and TNF-α, exerting anti-tumor effects through various mechanisms, including the inhibition of tumor vascular growth (77) (Figure 1). Blocking TNF-α or its receptor significantly diminishes cytotoxicity, while knocking down miR-125b-5p could increase the secretion of IFN-γ and TNF-α, thereby enhancing anti-tumor effects (78, 79). Studies focusing on solid tumors, particularly breast cancer, nasopharyngeal carcinoma, and melanoma, have demonstrated a positive correlation between the production of TNF-α by peripheral γδT cells and their contribution to tumor defense (77).
2.2 Innate lymphoid cells (ILCs)
2.2.1 NK cells
NK cells have consistently been acknowledged as effector cells proficient in lysing tumor cells or viruses, albeit with a non-specific targeting of cells. Upon recognizing target cells, NK cells exhibit directed movement of their abundant granules toward the binding site of target cells with the assistance of dynein motors (80, 81). The aggregation of these granules enhances efficiency in secretion while reducing the killing of surrounding cells. However, the cytotoxic impact of granules is contingent upon the presence of perforin. Mouse experiments have demonstrated that defective perforin leads to diminished cellular cytotoxicity, expedited tumor growth, and heightened metastasis, underscoring the crucial role of perforin in this process (82–84) (Figure 1). Currently, it is possible to induce the expression granzymes and perforin genes to augment the cytotoxic effects of NK cells.
Termed as “serial killers”, NK cells frequently shift towards cell destruction contingent upon FasL and TRAIL once their reservoirs of granzymes and perforin are depleted (85, 86) (Figure 1). Subsequent investigations have revealed NK cells deficient in perforin, previously considered lacking cytotoxicity, effectively eliminate MHC class I-deficient tumor cells due to the upregulation of FasL (87). FasL, in turn, interacts with the CD95 receptor on target cells, thereby initiating the apoptotic signaling cascade intrinsic to target cells (88). Intriguingly, the cleaved soluble form of FasL proves to be devoid of cytotoxic efficacy. Furthermore, NK cells harvested from the murine hepatic milieu distinctly express TRAIL, with their cytotoxicity potential markedly attenuated upon the introduction of anti-TRAIL monoclonal antibodies (85, 89).
Besides, IFN-γ secreted by NK cells has been demonstrated independently to exert anti-tumor functions, irrespective of perforin (Figure 1). Its collective influence plays a crucial role in governing the initiation, proliferation, and metastasis of tumors (90). Furthermore, while the specific anti-tumor mechanism of IFN-γ in particular tumors remains incompletely understood, its capabilities to inhibit tumor angiogenesis and modulate the sensitivity of tumor cells have long been reported (89).
In contrast, neoplastic cells may indeed serve as a crucial force driving the anti-tumor effects innitiated by NK cells (Figure 1). In melanoma, lactate derived from tumor cells significantly reduces the quantity and activation of NK cells. This is accomplished by suppressing the upregulation of the nuclear factor of activated T cells (NFAT) with NK cells, leading to a noticeable reduction in IFN-γ production and a simultaneous alleviation of the cytotoxic impact on tumors (91). In another study, it was revealed that lactate derived from tumor cells also directly diminishes the expression of perforin and granzyme, thereby impeding their cellular lytic functionality (92).
2.2.2 Helper ILCs
ILCs earn their name due to their absence of adaptive antigen receptors. In addition to NK cells, other subsets include ILC1s, ILC2s, ILC3s, and lymphoid tissue inducer cells (LTi) (93). They predominantly inhabit tissues and maintain close associations with the extracellular matrix (93). Typically, within the tumor microenvironment, ILC1s release significant levels of IFN-γ. This cytokine acts on tumor cells, inducing the upregulation of MHC-I and MHC-II, thereby directly stimulating tumor cell apoptosis and pyroptosis (93). Moreover, both ILC1s and ILC3s possess the ability to directly eliminate tumor cells by expressing TRAIL, thereby imbuing these cells with the potential of anti-tumor effector cells (94) (Figure 1).
2.3 M1-type macrophages(M1 macrophages)
In the TME, a subset of infiltrating macrophages, referred to as tumor-associated macrophages (TAMs), exhibits the capacity to differentiate into two distinct polarization states: M1 macrophages and M2-type (M2) macrophages (95). The identification of new markers such as C-X-C motif chemokine ligand 9(CXCL9) and (secreted phosphoprotein 1)SPP1 challenges the conventional M1/M2 classification paradigm (96). CXCL9, produced by macrophages, plays a pivotal role in immune cell activation and signaling involved in inflammatory responses, thereby enhancing anti-tumor capabilities (97, 98). Conversely, SPP1 expressed in macrophages can boost the expression of interferon-gamma and interleukin-12, influencing macrophage polarization, migration, and cytokine profile (98). The CXCL9:SPP1 expression ratio holds greater clinical significance (98). These newfound markers present a nuanced perspective on the potential range of macrophage activation states, offering fresh insights and avenues for the advancement of targeted immunotherapy strategies. In the following discussion, we chose to describe the more traditional and extensively studied M1/M2 classical polarization.
M1 macrophages possess potent antimicrobial and anti-tumor activities, releasing cytotoxic molecules such as reactive oxygen species (ROS) and nitric oxide synthases (INOS), gradually causing damage to tumor cells (99–101) (Figure 1). In murine animal experiments, it has been demonstrated that M1 macrophages secrete these factors that delay the growth of ovarian cancer tumors (102). However, others argue that TAMs release nitric oxide (NO) and reactive oxygen intermediates (ROI), causing DNA damage and genetic instability in the initial stages, categorizing them as tumor-promoting factors (103). Another rapid method of cell destruction involves ADCC, as clearly shown by the vitamin D-dependent release of antimicrobial peptide cathelicidin. This peptide effectively targets the mitochondria of malignant cells, culminating in the demise of high-grade B-cell lymphoma entities (104). As previously found, TNF-α at the tumor site is primarily derived from M1 macrophages and tumor cells (105) (Figure 1). Early research demonstrated that exogenous TNF-α could promote the destruction of tumor vasculature, thereby indirectly leading to the necrosis of tumor cells (106). Subsequent studies showed that high levels of exogenous TNF-α administration may act directly on malignant cells by inducing apoptosis, although the specific mechanisms of this process are not yet fully understood (56).
2.4 Eosinophils
Despite their lower presence in the peripheral bloodstream compared to T cells or B cells, eosinophils are selectively recruited to the tumor microenvironment by chemotactic agents, such as high mobility group box one protein (HMGB1) (107). Subsequently, these granulocytes release a spectrum of mediators, causing a direct cytotoxic impact on tumor cells. The identified mediators encompass major basic protein (MBP), eosinophil cationic protein (ECP), and eosinophil peroxidase (EPX), all capable of inducing tumor cell lysis in vitro (108, 109) (Figure 1). Furthermore, murine experiments focusing on colorectal cancer and lymphoma have revealed that the cytotoxic mediators wielded by eosinophils predominantly involve granule enzymes A and B (110–112).
In addition, in the presence of IL-5, eosinophils exhibit a significantly enhanced cytotoxic potency, coinciding with a noticeable deceleration in murine tumor growth (113). Moreover, when induced by lipopolysaccharide (LPS), eosinophils demonstrate the ability to directly undermine murine hepatic cancer cells via the release of TNF-α (114) (Figure 1). However, this phenomenon, can be effectively impeded by the administration of anti-TNF-α antibodies (115).
3 Direct tumor-promoting effects of immune cells and their counteractions
3.1 T cells
3.1.1 CD4+ T cells
3.1.1.1 Th2 cells
The role of Th2 cells in allergic diseases has been extensively investigated, but their specific implications in tumor immunity remain elusive (116). Notably, several studies have highlighted a close association between Th2 cells in the TME and the progression and metastasis of breast, cervical, colorectal, and lung cancers (117). IL-4, a pivotal factor in Th2 cell polarization and a primary secretion of Th2 cells, is proposed as a potential mechanism for its direct impact on tumors (Figure 2). Firstly, in colorectal cancer, IL-4 induces the expression of epithelial-mesenchymal transition(EMT)-promoting proteins through signal transducer and activator of transcription 6(STAT6)-dependent transcription, thereby prompting EMT in colon cancer cells (118). Secondly, IL-4 stimulates the proliferation of pancreatic cancer cells by activating phosphorylation in mitogen-activated protein kinases(MAPK), Akt-1, STAT3, and insulin receptors (119). In vitro experiments have additionally demonstrated that IL-4 promotes the expression of anti-apoptotic genes in various human cancers (120).
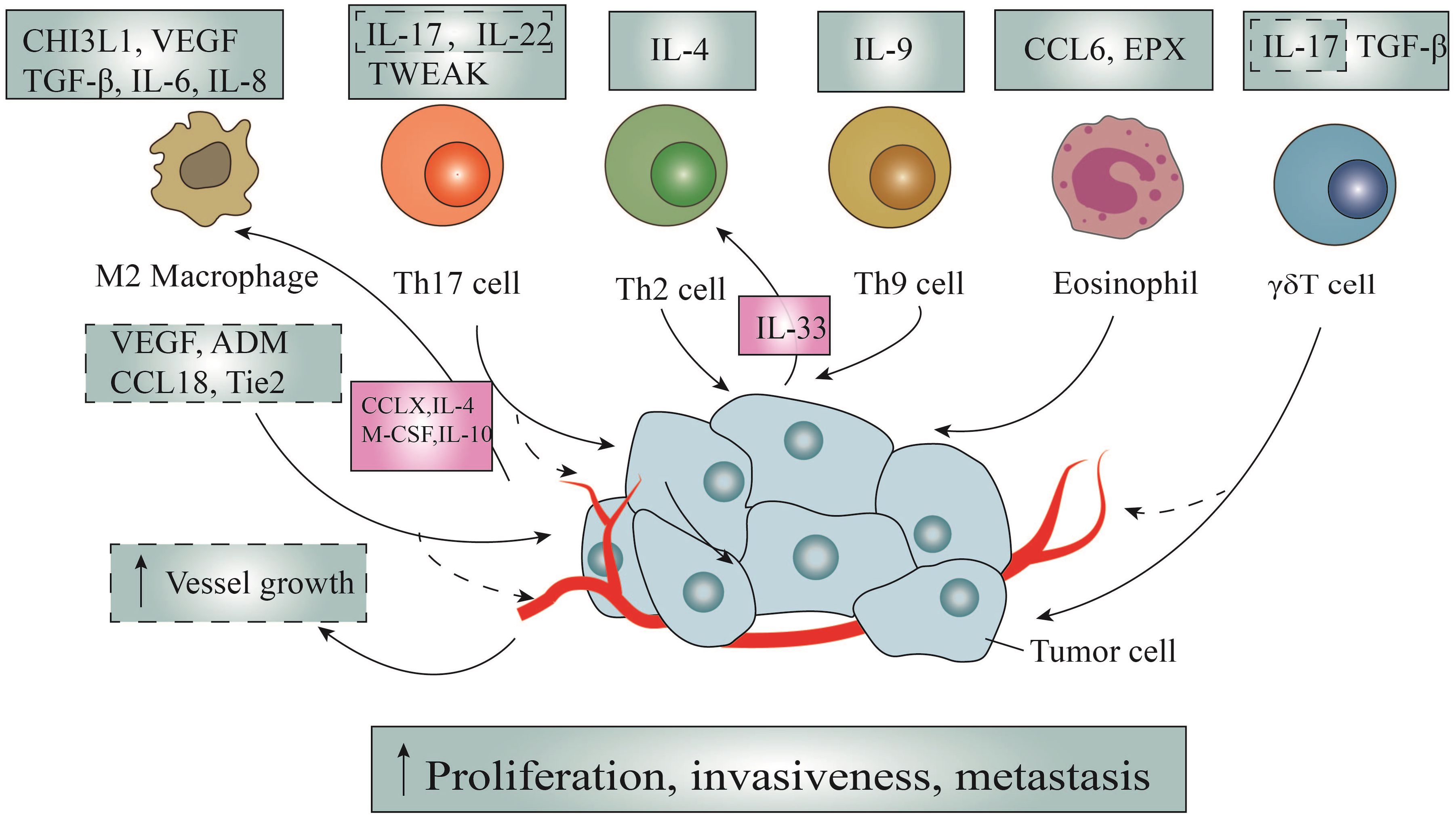
Figure 2 The direct tumor-promoting action of immune cells and the counteraction of tumor cells. Through the secretion of multiple chemokines, cytokines and other effector molecules such as IL-4, interleukin-5, and so on, immunocytes promote tumor cells through the following ways: promoting the proliferation of tumor cells, promoting the migration and metastasis of tumor cells and promoting tumor angiogenesis. It is worth noting that tumor cells can in turn promote the activation and recruitment of macrophages and Th2 cells via secreting CCLX, IL-33, IL-4, IL-10, and M-CSF, thus promoting the formation of loops.
Besides, recent research has also highlighted the interplay between tumor-infiltrating Th2 cells and tumor cells, where tumor fungal elements activate signaling pathways in cancer cells, promoting the secretion of IL-33, which is essential for the recruitment and activation of Th2 cells (121) (Figure 2). Conversely, the genetic deletion of IL-33 or antifungal therapy results in the regression of stable pancreatic ductal adenocarcinoma (PDAC), underscoring the tumor-promoting nature of Th2 cells. Despite this, concrete evidence substantiating the tumor-promoting effects of Th2 cells remains limited (121).
3.1.1.2 Th9 cells
Tumor-infiltrating Th9 lymphocytes release the characteristic cytokine IL-9, which has been implicated in various immune and inflammatory diseases, including parasitic infections, allergies, and lymphoma (122). However, the precise and consistent role of IL-9 in tumor immunity remains enigmatic and subject to controversy (Figure 2). According to existing literature, IL-9 binds to heterodimer receptors, activating the Janus kinase(JAK)-STAT, insulin receptor substrates(IRS), and MAPK signaling pathways, thereby directly stimulating tumor cell proliferation (123). Additionally, investigations have indicated that overexpression of IL-9 leads to amplified proliferation of colonic epithelial cells, attributed to the upregulation of c-MYC and cyclin D1 expression (124).
EMT, a pivotal mechanism underlying tumor metastasis, involves profound phenotypic alterations such as cytoskeletal reorganization, detachment from the extracellular matrix, and loss of polarity (125). Salazar et al. conducted a study encompassing lymphocyte co-cultures, in vivo mouse models, and human lung cancer tissues (126). The study revealed that tumor-infiltrating Th9 cells induce EMT and migration, and metastatic expansion of lung cancer. Similarly, others disclosed that IL-9 exerts notable influence on increasing the expression of C-C chemokine ligand 20 (CCL20) in hepatocellular carcinoma cells, thereby eliciting EMT changes through STAT3 phosphorylation (127).
3.1.1.3 Th17 cells
Named after their hallmark product, IL-17A, Th17 cells are considered a major component among infiltrating tumor lymphocytes, concurrently secreting IL-17F, IL-21, IL-22, and IL-2 (128). First, IL-17, originating from Th17 cells, serves as a stimulant for tumor cell proliferation across diverse pathways (129) (Figure 2). For instance, B-cell acute lymphoblastic leukemia relies on Akt and STAT3 pathways, colorectal cancer involves STAT3 and NF-κB pathways, and ovarian cancer stem cells necessitate the engagement of NF-κB and MAPK pathways (130, 131). In a recent investigation, it has been unveiled that the secretion of tumor necrosis factor-like weak inducer of apoptosis(TWEAK) by Th17 cells triggers epithelial-mesenchymal transition, consequently fostering liver metastasis in colorectal cancer (132) (Figure 2).
Moreover, within the domain of lung cancer research, the interaction between Th17/Treg cells and their impact on non-squamous non-small cell lung cancer (NSCLC) biology has garnered attention. These emphasize that Th17 cells not only induce EMT in lung cancer cells, but also augment migration and dissemination, correlating with lymphatic vessel density (126). Subsequent investigations have provided evidence linking IL-17 and IL-22 to increased invasiveness and metastasis of lung cancer cells (Figure 2). Furthermore, these studies have demonstrated resistance to combined MEK inhibitor and anti-PD-L1 therapies in KRAS/p53 mutant lung cancers (133). In the context of non-small cell lung cancer, IL-17A actively promotes migration and invasion through the STAT3/NF-κB/Notch1 signaling pathway (134). Despite the substantial roles of IL-17 and IL-22 in inducing angiogenesis, facilitating EMT, and expressing matrix metalloproteinases (MMPs) to promote tumor growth and tumor metastasis, there is currently a dearth of literature specifying the specific sources of these two cytokines (135–139).
3.1.2 γδT cells
While traditionally recognized for their potent anti-tumor effects, γδT lymphocytes also possess the potential to accelerate the progression and invasive tendencies of solid tumors (140). Nonetheless, compelling evidence suggests that γδT cells may expedite the development and invasion of solid tumors (141). At the core of their tumorigenic impact is the pivotal mediator IL-17, a molecule that not only drives neoplastic cell proliferation through intricate IL-6/STAT3 and NF-κB signaling cascades but also triggers metastasis by inducing the secretion of VEGF and MMP (142). Furthermore, under specific circumstances, epithelial Vδ1 T cells have been observed to secrete notable quantities of TGF-β, initiating the transformation of epithelial cells into mesenchymal cells and thereby amplifying the invasiveness of malignancies (143) (Figure 2).
3.2 M2 macrophages
In contrast to the anti-tumor effects associated with M1 macrophages discussed earlier, M2 macrophages are typically considered closely associated with promoting tumor metastasis (Figure 2). Notably, macrophage-colony stimulating factor(CSF-1), primarily sourced from macrophages, has been found to be correlated with poor prognosis in breast cancer, ovarian cancer, endometrial cancer, lung cancer, and prostate cancer, though the detailed underlying mechanisms remain unclear (144, 145). M2 macrophages promote metastasis by producing MMPs and tissue proteases, which degrade the extracellular matrix, allowing invasive tumor cells to migrate into surrounding tissues and the vascular system (145). Secondly, M2 macrophages can promote lymph node metastasis of tumor cells by enhancing the functionality of lymphatic vessels. Additionally, M2 macrophages play roles in inducing the formation of tip cells in lymphatic endothelial cells (LECs) and the proliferation of lymphocytes through the secretion of VEGF-C and the expression of podoplanin (146).
The promotion of tumor metastasis by M2 macrophages is closely associated with the formation of new tumor blood vessel as well (147, 148) (Figure 2). The MMP9 produced by these macrophages typically facilitate the release of VEGF from the extracellular reservoir, thereby increasing the bioavailability of VEGF (147). Although TAM infiltration is predominantly associated with extensive angiogenesis via VEGF signaling pathway, studies have shown that disrupting the VEGFA allele effectively impacts vascular sprouting without affecting the recruitment of macrophages and angiogenesis. Further research has demonstrated that this is closely associated with TAM-derived adrenomedullin (ADM) and C-C motif ligand 18(CCL18) (149, 150). Respectively ADM promotes angiogenesis and melanoma growth via the paracrine effect, mediated by the endothelial nitric oxide synthase signaling pathway, and CCL18 promotes human umbilical vein endothelial cell migration and tube formation via PITPNM3 (149, 150). Additionally, the expression of the Tie2 receptor by these macrophages is a known receptor for angiopoietin, playing a crucial role in angiogenesis. Additionally, the expression of the Tie2 receptor by these macrophages is a known receptor for angiopoietin, playing a crucial role in angiogenesis (151).
The EMT is a process in which epithelial cells gradually lose their epithelial characteristics and acquire a mesenchymal phenotype, playing a crucial role in tumor cell metastasis. Macrophages exhibit high infiltration in the tumor microenvironment, secreting a series of inflammatory and cytokine factors to promote EMT and enhance the stemness of cancer cells (152) (Figure 2). For instance, IL-6 derived from M2 macrophages has been found to downregulate the epithelial marker E-cadherin and upregulate the mesenchymal marker vimentin in cancer cells (145). Additionally, M2 macrophages can also secrete TGF-β to induce Sox9 expression in lung cancer cells through the c-Jun/Smad3 pathway, thereby inducing EMT and enhancing lung cancer cell migration (145). IL-8 also has the ability to induce EMT by activating the JAK2/STAT3/Snail pathway (153). Moreover, TAMs regulate breast cancer stem cell phenotype and promote tumor growth via the EGFR/Stat3/Sox-2 signaling pathway (154).
Several other cytokines derived from M2 macrophages also play vital roles, as follows (Figure 2). For instance, IL-6 has been shown to activate cancer stem cells, facilitating cancer growth and metastasis by promoting anti-apoptotic pathways through STAT3 phosphorylation (155, 156). As we all know, Chitinase 3-like protein -1 (CHI3L1), as a glycoprotein, assumes a pivotal role in governing various aspects of tumor cell behavior, including growth, proliferation, invasion, metastasis, angiogenesis, and activation (157). Correspondingly, CHI3L1, derived from M2 macrophages in mice, facilitates the metastasis of gastric cancer and breast cancer through the IL-13 receptor (158).
Surprisingly, tumor cells often react against TAMs in a way that amplifies their facilitation (Figure 2). TAMs originate from peripheral monocytes, recruited into tumors by several growth factors, particularly those produced by matrix and tumor cells (159). Macrophages’ polarization is regulated by various microenvironmental signals from tumor cells, such as IL-4 and IL-10, which serve the same purpose (160). In addition to macrophage colony-stimulating factor (M-CSF) and tumor-derived factors such as chemokines CCL2, CCL3, CCL4, and CCL5, which serve as macrophage chemoattractants, CCL2 is extensively expressed in various human tumors (161). For example, cancer cells produce CCL2 to recruit inflammatory CC chemokine receptor 2(CCR2) monocytes from blood to metastatic sites, where they differentiate into related macrophages and promote tumor cell extravasation under the influence of VEGF (162).
3.3 Eosinophils
Initially, eosinophils were commonly associated with specific inflammatory issues, particularly allergies and parasitic infections (114). However, as our knowledge grows regarding how inflammatory factors play a role in starting and advancing tumor cells, there has been a recent reevaluation of the role of eosinophils in this process. Preliminary studies have confirmed MMP9’s role in extracellular matrix degradation (163). Still, we are not completely sure about how this contributes to tumor cell invasion and spreading. Likewise, in situations with inflammation, MBP has been seen to make blood vessel cells multiply and boost the growth effects of VEGF (164). However, we are still working to confirm its similar role in the tumor environment (164).
Moving forward, substantial progress has been achieved in investigating eosinophils in solid tumors. According to Vasilios and his team, EPX from eosinophils has been observed to encourage tumor spreading in a mouse breast cancer model using the 4T1 strain (165). Furthermore, eosinophils have been strongly linked to speeding up the movement and spread of tumor cells in melanoma, credited to the release of a substance called CCL6 (166) (Figure 2).
4 Therapeutic strategies according to the mechanisms of direct effects of immunocytes on cancer cells
Despite atechnological advances, immunotherapy and targeted therapy remain key cancer treatments. Common immune checkpoints, exemplified by programmed death 1(PD-1), upon binding with programmed cell death-ligand 1(PDL-1), are typically expressed on the surface of tumor cells, orchestrating the inhibition of T cell proliferation and activation, thereby facilitating the evasion of tumor cells from immune surveillance (167). A parallel player in this regulatory milieu is cytotoxic T lymphocyte-associated antigen-4(CTLA-4), predominantly curtailing T cell activation and proliferation through competitive interference with the engagement of CD28 and co-stimulatory molecules CD80/86 (168). Additionally, lymphocyte activation gene-3(LAG-3) and T-cell immunoglobulin and mucin-domain containing-3(TIM-3) serve as pivotal suppressors of T cell activation and functionality by respectively engaging with MHC-II molecules and the ligand Galectin-9 (169). These two are typically not expressed on tumor cells, but are mainly expressed on T cells. Presently, PD-1 inhibitors such as Pembrolizumab and Nivolumab, along with PDL-1 inhibitors like Atezolizumab and Avelumab, as well as the CTLA-4 inhibitor Ipilimumab, stand as stalwarts in clinical intervention (170). Meanwhile, agents targeting LAG-3, TIM-3, among others, represented by BMS-986016 and MBG453, traverse the clinical research terrain, poised to offer therapeutic avenues for diverse malignancies in the forthcoming era. Nevertheless, notwithstanding the therapeutic promise, the response rate to immune checkpoint therapy remains modest, with resistance posing a formidable challenge (171, 172). Since current methods primarily enhance indirect anti-tumor effects, there is a pressing need to understand and explore direct anti-tumor therapies for comprehensive.
In this review, we focus mainly on the direct anti-tumor mechanisms of immune cells from three perspectives: amplifying the ADCC effect, triggering the secretion of granzymes and perforin, and modulating the Fas/FasL pathway (Table 1). Firstly, monoclonal antibodies such as Rituximab(anti CD20) and Trastuzumab(anti-HER2) have shown effectiveness via the ADCC pathway (173). Noteworthy studies involving mice lacking the Fcγ chain have revealed increased ADCC-mediated cytotoxicity in the absence of Fc gammaRIIB, while optimal antibody binding minimizes inhibitory effects via Fc gammaRIIB (174). Other antibodies, such as Cetuximab(anti-EGFR), Pertuzumab(anti-HER2), and bispecific antibodies-Catumaxomab, have opened new avenues in clinical research for gastrointestinal and breast cancer treatments (175). In addition, clinical drugs like Anktiva and Nemvaleukin alfa (ALKS 4230) stimulate the secretion of cytokines, such as IL-2, IL-21, and IL-15, to enhance the ADCC effect of NK cells (176). In the most recent study, researchers have devised a high-affinity, non-cleavable CD16 variant. Upon fusion with the NK cell activation domain, this novel construct robustly augments anti-tumor cell activity via the ADCC pathway (177). This approach primarily focuses on NK cells, which is less common in research involving other immune cells (178, 179).
Chimeric antigen receptor-modified T(CAR-T) technology enhances the release of perforin and granzymes, transforming CTLs, Th cells, NK cells, and other cells into powerful weapons for eliminating tumor cells (180). Additionally, the NKp30 receptor serves as another specific receptor for CAR-T technology, triggering the secretion of granzymes and perforin upon when binding to B7-H6l (181). However, there is a lack of developed antibodies or small ligands targeting NKp30. Despite various studies demonstrating the presence of perforin and granzyme B in T cells from CAR patients, resulting the cleavage of fibronectin extra domain B-positive cells and the induction of apoptosis, effective therapeutic interventions are still pending (158, 182, 183). In addition, blocking immune checkpoints can alleviate the suppression of the expression of perforin and granzymes, enhancing cytotoxicity (184). Furthermore, in in vitro experiments, it has been demonstrated that the use of PD-1 blockade drugs can effectively boost the cytotoxicity of γδ T cells (185). Furthermore, in vitro, assays revealed that either Bacillus Calmette-Guéri or Zoledronate treatment of bladder tumor cells induced granzymes (186). Ongoing experiments are focused on investigating fluorescent biosensors, allowing for a more specific and sensitive assessment of granzyme B activity (187).
Compared to the involvement of granzyme and perforin, the Fas/FasL pathway and secreted cytokines, as another potent anti-tumor target, can significantly and directly enhance the tumor-killing efficacy (188). Traditional chemotherapy drugs like Doxorubicin and Methotrexate induce DNA damage in immune cells, leading to the expression of FasL on their surface to bolster the effectiveness of the immune system (189, 190). Undoubtedly, the application of antibodies, such as R-125224, is undeniable in this context. Moreover, FasL gene therapy is also actively under development, though its practical implementation remains contentious. Common delivery methods encompass adenovirus delivery, FasL-engineered cell delivery, and attenuated bacterial delivery (191). Despite, IFN-γ being an FDA-approved drug for treating chronic granulomatous disease and osteopetrosis, its approval for malignancy treatment is currently pending (192).
Given the evolving landscape of direct tumor-modulating mechanisms of immune cells, strategies to curtail tumor cell proliferation and inhibit blood vessel growth have garnered exploration (Table 1). Despite their crucial role in regulating tumor cell proliferation, differentiation, and apoptosis (193), the precise mechanisms and long-term consequences of STAT3 and STAT5 remain relatively unknown (194). Among the few inhibitors targeting the SH2 domain of STAT3 and interacting with STAT5, OPB-31121 has shown anti-tumor activity in leukemia, with ongoing phase I/II clinical trials assessing efficacy against solid tumors and hematopoietic cancers (195).
The prominence of EMT in tumor progression has galvanized extensive research into approaches for tumor treatment (196, 197) (Table 1). In a recent investigation, Soundararajan et al. embarked on exploring the potential of combining EMT therapy to overcome resistance to immunotherapy, presenting a promising strategy for enhancing treatment outcomes (198, 199). Targeting upstream pathways of EMT can significantly inhibit tumor growth, with TGF-β signaling being the most prominent inducer of EMT (200). Extensive research has focused on evaluating the effectiveness of TGF-β inhibitors, such as LY2157299, as potent anti-EMT compounds in ongoing clinical trials (201, 202). Similarly, targeting upstream transcription factors of EMT has been proposed as a feasible therapeutic alternative for invasive cancers (203–206). Furthermore, another treatment option for EMT-dependent cancers is targeting the stromal cells, with an exciting approach being to target the stromal cells themselves by inhibiting stroma-specific proteins with monoclonal antibodies (207). This has been validated in a mouse model of breast cancer (208). However, the current therapeutic approaches for EMT programs remain rudimentary, suggesting an exciting avenue for future developments in highly effective therapies to manage high-grade tumor malignancies.
In addition to the aforementioned factors, MMPs are other major mediators for metastasis and invasion of tumor cells in the tumor microenvironment (209) (Table 1). Though attempts to develop drugs targeting MMPs were made twenty-five years ago, phase III clinical trials evaluating small molecule metalloproteinase inhibitors (MPIs) yielded disappointing, failing to improve survival rates for cancer patients. The limited efficacy of MPIs for palliative care has been widely recognized (201). Currently, MMP inhibitory monoclonal antibodies are considered promising MMP-targeted therapies, as they offer higher target selectivity and better pharmacokinetic properties compared to small molecule drugs (210). Inhibitory monoclonal antibodies targeting individual MMP-9 and MMP-14 have been developed and demonstrated anti-tumor activity in preclinical models of breast cancer, which could become a promising area of research in the future (211–214).
Ultimately, interfering with tumor vasculature has emerged as a promising strategy to inhibit tumor growth (202, 215, 216) (Table 1). Notably, Bevacizumab(anti-VEGF), an FDA-approved drug for previously untreated metastatic colorectal cancer, has demonstrated remarkable effects, extending its application to diverse malignant tumors, including NSCLC, renal cell carcinoma, ovarian cancer, and cervical cancer (215, 217). Another fusion protein capable of effectively targeting angiogenesis by inhibiting VEGF-A, VEGF-B, and placental growth factor is Ziv-aflibercept, which has also been brought to market. It is worth noting that, compared to bevacizumab, it exhibits a higher binding affinity to VEGF-A (218, 219). Additionally, Ramucirumab is a human IgG1 monoclonal antibody that acts as an inhibitor of VEGFR2 (220). It works by binding to and inhibiting the activation of VEGFR2, thereby suppressing the signaling pathways mediated by VEGF (220). other drugs like Aflibercept are currently under development, showing promising potential in inhibiting tumor vasculature (221).
5 Conclusions
Despite significant progress in cancer treatment, the ongoing existence of malignant tumors highlights persistent challenges such as immune suppression, evasion, and tolerance. Given the pivotal role of immune cells within the TME, this review comprehensively delves into the direct, intricate, and bidirectional impacts they exert on tumor cells. These dynamic interactions unveil a complex pattern, wherein distinct immune cell cohorts may paradoxically propel tumor progression or incite robust antitumor responses across varied tumor microenvironments. Precision interventions aimed at enhancing immune cell cytotoxicity or diminishing their tumor-promoting effects show promise in overcoming the challenges presented by the dual nature of immune cells and the intricate landscape of indirect immune regulation.
However, in the overall scheme, the efficacy of tumor treatment is closely related to the immune environment of tumor patients, going beyond just describing the direct interactions between immune cells and tumor cells as outlined in this paper. The indirect influences of immune cells, including the regulation of T cells and fibroblasts, need to be considered. Additionally, the emergence of novel immune cell markers may indicate the emergence of diverse subgroups of immune cells with various functionalities and contributions to tumor biology. These new insights challenge traditional paradigms of immune polarization, emphasizing the importance of a detailed understanding of immune cell heterogeneity in oncology and highlighting the complex composition of immune cell biology. Therefore, exploring the intricately complex components of the tumor microenvironment, understanding their specific, direct mechanisms of action, can yield valuable insights into slowing tumor progression, controlling drug resistance, and more.
Author contributions
ZY: Writing – original draft, Writing – review & editing. PC: Writing – original draft, Writing – review & editing. QH: Writing – original draft, Writing – review & editing. JH: Writing – original draft. LH: Conceptualization, Writing – review & editing. GH: Conceptualization, Funding acquisition, Resources, Writing – review & editing.
Funding
The author(s) declare financial support was received for the research, authorship, and/or publication of this article. This work was supported by Zhejiang Provincial Natural Science Foundation of China (Grant No. LY23H160002, GMH) and the National Natural Science Foundation of China (Grant No. 82173080, GMH). This work was partly granted from High-level Talent Training Project in Health of Zhejiang Province (GMH).
Acknowledgments
We thank all the members of the departments who helped in this study.
Conflict of interest
The authors declare that the research was conducted in the absence of any commercial or financial relationships that could be construed as a potential conflict of interest.
Publisher’s note
All claims expressed in this article are solely those of the authors and do not necessarily represent those of their affiliated organizations, or those of the publisher, the editors and the reviewers. Any product that may be evaluated in this article, or claim that may be made by its manufacturer, is not guaranteed or endorsed by the publisher.
Glossary
FAS: Factor associated suicide
FASL: Factor associated suicide ligand
TRAIL: Tumor necrosis associated apoptosis-inducing ligand
HER2: Human epidermal growth factor receptor 2
TNF: Tumor necrosis factor
INK4a: The inhibitor of cyclin-dependent kinase 4a
Blimp-1: B-lymphocyte-induced maturation protein 1
VEGF-A: Vascular endothelial growth factor-A
PGE2: Prostaglandin E2
MHC: Major histocompatibility complex
PBMCs: Peripheral blood mononuclear
IPP: Isopentenyl pyrophosphate
SCCHN: Squamous cell carcinoma of the head and neck
CMA: Concanamycin A
ADCC: Antibody-dependent cell-mediated cytotoxicity
ILCs: Innate lymphoid cells
NFAT: Nuclear factor of activated T cells
LTi: Lymphoid tissue inducer cells
TAMs: Tumor-associated macrophages
M1 macrophages: M1-type macrophages
M2 macrophages: M2-type macrophages
ROS: Reactive oxygen species
iNOS: Nitric oxide synthases
NO: Nitric oxide
ROI: Reactive oxygen intermediates
HMGB1: High mobility group box one protein
MBP: Major basic protein
ECP: Eosinophil cationic protein
EPX: Eosinophil peroxidase
LPS: Lipopolysaccharide
EMT: Epithelial-mesenchymal transition
STAT6: Signal Transducer and Activator of Transcription 6
MAPK: Mitogen-activated protein kinases
PDAC: Pancreatic ductal adenocarcinoma
IRS: Insulin receptor substrates
JAK: Janus kinase
CCL20: C-C chemokine ligand 20
NSCLC: Non-squamous non-small cell lung cancer
MMPs: Expressing matrix metalloproteinases
CSF-1: Macrophage-colony stimulating factor
LECs: Lymphatic endothelial cells
ADM: TAM-derived adrenomedullin
CHI3L1: Chitinase 3-like protein -1
M-CSF: Macrophage colony-stimulating factor
CCR2: CC chemokine receptor 2
PD-1: Programmed death 1
CTLA-4: Cytotoxic T lymphocyte-associated antigen-4
PDL-1: Programmed cell death-ligand 1
LAG-3: Lymphocyte activation gene-3
TIM-3: T-cell immunoglobulin and mucin-domain containing-3
CAR-T: Chimeric antigen receptor-modified T
MPIs: Metalloproteinase inhibitors
CXCL9: C-X-C motif chemokine ligand 9
SPP1: secreted phosphoprotein 1
TWEAK: tumor necrosis factor-like weak inducer of apoptosis
References
1. Hinshaw DC, Shevde LA. The tumor microenvironment innately modulates cancer progression. Cancer Res. (2019) 79:4557–66. doi: 10.1158/0008-5472.CAN-18-3962
2. Bejarano L, Jordao MJC, Joyce JA. Therapeutic targeting of the tumor microenvironment. Cancer Discovery. (2021) 11:933–59. doi: 10.1158/2159-8290.CD-20-1808
3. Czajka-Francuz P, Cison-Jurek S, Czajka A, Kozaczka M, Wojnar J, Chudek J, et al. Systemic interleukins’ Profile in early and advanced colorectal cancer. Int J Mol Sci. (2021) 23:124. doi: 10.3390/ijms23010124
4. Mao X, Xu J, Wang W, Liang C, Hua J, Liu J, et al. Crosstalk between cancer-associated fibroblasts and immune cells in the tumor microenvironment: new findings and future perspectives. Mol Cancer. (2021) 20:131. doi: 10.1186/s12943-021-01428-1
5. Wculek SK, Cueto FJ, Mujal AM, Melero I, Krummel MF, Sancho D. Dendritic cells in cancer immunology and immunotherapy. Nat Rev Immunol. (2020) 20:7–24. doi: 10.1038/s41577-019-0210-z
6. Kim HJ, Cantor H. CD4 T-cell subsets and tumor immunity: the helpful and the not-so-helpful. Cancer Immunol Res. (2014) 2:91–8. doi: 10.1158/2326-6066.CIR-13-0216
7. Gajewski TF, Schreiber H, Fu YX. Innate and adaptive immune cells in the tumor microenvironment. Nat Immunol. (2013) 14:1014–22. doi: 10.1038/ni.2703
8. Lei X, Lei Y, Li JK, Du WX, Li RG, Yang J, et al. Immune cells within the tumor microenvironment: Biological functions and roles in cancer immunotherapy. Cancer Lett. (2020) 470:126–33. doi: 10.1016/j.canlet.2019.11.009
9. Upadhyay R, Boiarsky JA, Pantsulaia G, Svensson-Arvelund J, Lin MJ, Wroblewska A, et al. A critical role for fas-mediated off-target tumor killing in T-cell immunotherapy. Cancer Discovery. (2021) 11:599–613. doi: 10.1158/2159-8290.CD-20-0756
10. Beck RJ, Slagter M, Beltman JB. Contact-dependent killing by cytotoxic T lymphocytes is insufficient for EL4 tumor regression in vivo. Cancer Res. (2019) 79:3406–16. doi: 10.1158/0008-5472.CAN-18-3147
11. Li Y, Li G, Zhang J, Wu X, Chen X. The dual roles of human gammadelta T cells: anti-tumor or tumor-promoting. Front Immunol. (2020) 11:619954. doi: 10.3389/fimmu.2020.619954
12. Kyrysyuk O, Wucherpfennig KW. Designing cancer immunotherapies that engage T cells and NK cells. Annu Rev Immunol. (2023) 41:17–38. doi: 10.1146/annurev-immunol-101921-044122
13. Sun L, Su Y, Jiao A, Wang X, Zhang B. T cells in health and disease. Signal Transduct Target Ther. (2023) 8:235. doi: 10.1038/s41392-023-01471-y
14. Taniuchi I. CD4 helper and CD8 cytotoxic T cell differentiation. Annu Rev Immunol. (2018) 36:579–601. doi: 10.1146/annurev-immunol-042617-053411
15. Meitei HT, Lal G. T cell receptor signaling in the differentiation and plasticity of CD4+ T cells. Cytokine Growth Factor Rev. (2023) 69:14–27. doi: 10.1016/j.cytogfr.2022.08.001
16. Saravia J, Chapman NM, Chi H. Helper T cell differentiation. Cell Mol Immunol. (2019) 16:634–43. doi: 10.1038/s41423-019-0220-6
17. Walker JA, McKenzie ANJ. TH2 cell development and function. Nat Rev Immunol. (2017) 18:121–33. doi: 10.1038/nri.2017.118
18. Koch S, Sopel N, Finotto S. Th9 and other IL-9-producing cells in allergic asthma. Semin Immunopathology. (2016) 39:55–68. doi: 10.1007/s00281-016-0601-1
19. Staudt V, Bothur E, Klein M, Lingnau K, Reuter S, Grebe N, et al. Interferon-regulatory factor 4 is essential for the developmental program of T helper 9 cells. Immunity. (2010) 33:192–202. doi: 10.1016/j.immuni.2010.07.014
20. Campe J, Ullrich E. T helper cell lineage-defining transcription factors: potent targets for specific GVHD therapy? Front Immunol. (2022) 12:806529. doi: 10.3389/fimmu.2021.806529
21. Zhu J. T helper cell differentiation, heterogeneity, and plasticity. Cold Spring Harbor Perspect Biol. (2018) 10:a030338. doi: 10.1101/cshperspect.a030338
22. Spinner CA, Lazarevic V. Transcriptional regulation of adaptive and innate lymphoid lineage specification. Immunol Rev. (2020) 300:65–81. doi: 10.1111/imr.12935
23. Chen T, Guo J, Cai Z, Li B, Sun L, Shen Y, et al. Th9 cell differentiation and its dual effects in tumor development. Front Immunol. (2020) 11:1026. doi: 10.3389/fimmu.2020.01026
24. Korn T, Bettelli E, Oukka M, Kuchroo VK. IL-17 and th17 cells. Annu Rev Immunol. (2009) 27:485–517. doi: 10.1146/annurev.immunol.021908.132710
25. Preglej T, Ellmeier W. CD4(+) cytotoxic T cells - phenotype, function and transcriptional networks controlling their differentiation pathways. Immunol Lett. (2022) 247:27–42. doi: 10.1016/j.imlet.2022.05.001
26. Reina-Campos M, Scharping NE, Goldrath AW. CD8(+) T cell metabolism in infection and cancer. Nat Rev Immunol. (2021) 21:718–38. doi: 10.1038/s41577-021-00537-8
27. Borst J, Ahrends T, Babala N, Melief CJM, Kastenmuller W. CD4(+) T cell help in cancer immunology and immunotherapy. Nat Rev Immunol. (2018) 18:635–47. doi: 10.1038/s41577-018-0044-0
28. Cachot A, Bilous M, Liu YC, Li X, Saillard M, Cenerenti M, et al. Tumor-specific cytolytic CD4 T cells mediate immunity against human cancer. Sci Adv. (2021) 7:eabe3348. doi: 10.1126/sciadv.abe3348
29. Preglej T, Ellmeier W. CD4+ Cytotoxic T cells – phenotype, function and transcriptional networks controlling their differentiation pathways. Immunol Lett. (2022) 247:27–42. doi: 10.1016/j.imlet.2022.05.001
30. Echchakir H, Bagot M, Dorothée G, Martinvalet D, Le Gouvello S, Boumsell L, et al. Cutaneous T cell lymphoma reactive CD4+ cytotoxic T lymphocyte clones display a Th1 cytokine profile and use a fas-independent pathway for specific tumor cell lysis. J Invest Dermatol. (2000) 115:74–80. doi: 10.1046/j.1523-1747.2000.00995.x
31. Takeuchi A, Saito T. CD4 CTL, a cytotoxic subset of CD4+ T cells, their differentiation and function. Front Immunol. (2017) 8:194. doi: 10.3389/fimmu.2017.00194
32. Schattner EJ, Mascarenhas J, Bishop J, Yoo DH, Chadburn A, Crow MK, et al. CD4+ T-cell induction of Fas-mediated apoptosis in Burkitt’s lymphoma B cells. Blood. (1996) 88:1375–82. doi: 10.1182/blood.V88.4.1375.bloodjournal8841375
33. Thomas WD, Hersey P. TNF-related apoptosis-inducing ligand (TRAIL) induces apoptosis in Fas ligand-resistant melanoma cells and mediates CD4 T cell killing of target cells. J Immunol. (1998) 161:2195–200. doi: 10.4049/jimmunol.161.5.2195
34. Peng S, Lin A, Jiang A, Zhang C, Zhang J, Cheng Q, et al. CTLs heterogeneity and plasticity: implications for cancer immunotherapy. Mol Cancer. (2024) 23:58. doi: 10.1186/s12943-024-01972-6
35. Lee WS, Yang H, Chon HJ, Kim C. Combination of anti-angiogenic therapy and immune checkpoint blockade normalizes vascular-immune crosstalk to potentiate cancer immunity. Exp Mol Med. (2020) 52:1475–85. doi: 10.1038/s12276-020-00500-y
36. Jorgovanovic D, Song M, Wang L, Zhang Y. Roles of IFN-γ in tumor progression and regression: a review. biomark Res. (2020) 8:49. doi: 10.1186/s40364-020-00228-x
37. Jia Y, Kodumudi KN, Ramamoorthi G, Basu A, Snyder C, Wiener D, et al. Th1 cytokine interferon gamma improves response in HER2 breast cancer by modulating the ubiquitin proteasomal pathway. Mol Ther. (2021) 29:1541–56. doi: 10.1016/j.ymthe.2020.12.037
38. Braumüller H, Wieder T, Brenner E, Aßmann S, Hahn M, Alkhaled M, et al. T-helper-1-cell cytokines drive cancer into senescence. Nature. (2013) 494:361–5. doi: 10.1038/nature11824
39. He Y, Dong L, Cao Y, Bi Y, Liu G. IL-9 and th9 cells in tumor immunity. Adv Exp Med Biol. (2020) 1240:35–46. doi: 10.1007/978-3-030-38315-2_3
40. Purwar R, Schlapbach C, Xiao S, Kang HS, Elyaman W, Jiang X, et al. Robust tumor immunity to melanoma mediated by interleukin-9-producing T cells. Nat Med. (2012) 18:1248–53. doi: 10.1038/nm.2856
41. Lu Y, Wang Q, Xue G, Bi E, Ma X, Wang A, et al. Th9 cells represent a unique subset of CD4(+) T cells endowed with the ability to eradicate advanced tumors. Cancer Cell. (2018) 33:1048–1060.e7. doi: 10.1016/j.ccell.2018.05.004
42. Rivera Vargas T, Humblin E, Végran F, Ghiringhelli F, Apetoh L. T(H)9 cells in anti-tumor immunity. Semin Immunopathol. (2017) 39:39–46. doi: 10.1007/s00281-016-0599-4
43. Schanz O, Cornez I, Yajnanarayana SP, David FS, Peer S, Gruber T, et al. Tumor rejection in Cblb(-/-) mice depends on IL-9 and Th9 cells. J Immunother Cancer. (2021) 9:e002889. doi: 10.1136/jitc-2021-002889
44. Fang Y, Chen X, Bai Q, Qin C, Mohamud AO, Zhu Z, et al. IL-9 inhibits HTB-72 melanoma cell growth through upregulation of p21 and TRAIL. J Surg Oncol. (2015) 111:969–74. doi: 10.1002/jso.23930
45. Sykulev Y. Factors contributing to the potency of CD8+ T cells. Trends Immunol. (2023) 44:693–700. doi: 10.1016/j.it.2023.07.005
46. Al Subeh ZY, Poschel DB, Redd PS, Klement JD, Merting AD, Yang D, et al. Lipid nanoparticle delivery of fas plasmid restores fas expression to suppress melanoma growth in vivo. ACS Nano. (2022) 16:12695–710. doi: 10.1021/acsnano.2c04420
47. Barry M, Bleackley RC. Cytotoxic T lymphocytes: all roads lead to death. Nat Rev Immunol. (2002) 2:401–9. doi: 10.1038/nri819
48. Voskoboinik I, Whisstock JC, Trapani JA. Perforin and granzymes: function, dysfunction and human pathology. Nat Rev Immunol. (2015) 15:388–400. doi: 10.1038/nri3839
49. Zophel D, Angenendt A, Kaschek L, Ravichandran K, Hof C, Janku S, et al. Faster cytotoxicity with age: Increased perforin and granzyme levels in cytotoxic CD8(+) T cells boost cancer cell elimination. Aging Cell. (2022) 21:e13668. doi: 10.1111/acel.13668
50. Lopez JA, Susanto O, Jenkins MR, Lukoyanova N, Sutton VR, Law RH, et al. Perforin forms transient pores on the target cell plasma membrane to facilitate rapid access of granzymes during killer cell attack. Blood. (2013) 121:2659–68. doi: 10.1182/blood-2012-07-446146
51. Ritter AT, Shtengel G, Xu CS, Weigel A, Hoffman DP, Freeman M, et al. ESCRT-mediated membrane repair protects tumor-derived cells against T cell attack. Science. (2022) 376:377–82. doi: 10.1126/science.abl3855
52. Golstein P, Griffiths GM. An early history of T cell-mediated cytotoxicity. Nat Rev Immunol. (2018) 18:527–35. doi: 10.1038/s41577-018-0009-3
53. Cai Z, Yang F, Yu L, Yu Z, Jiang L, Wang Q, et al. Activated T cell exosomes promote tumor invasion via Fas signaling pathway. J Immunol. (2012) 188:5954–61. doi: 10.4049/jimmunol.1103466
54. Wang W, Green M, Choi JE, Gijón M, Kennedy PD, Johnson JK, et al. CD8(+) T cells regulate tumour ferroptosis during cancer immunotherapy. Nature. (2019) 569:270–4. doi: 10.1038/s41586-019-1170-y
55. Liao P, Wang W, Wang W, Kryczek I, Li X, Bian Y, et al. CD8(+) T cells and fatty acids orchestrate tumor ferroptosis and immunity via ACSL4. Cancer Cell. (2022) 40:365–78. doi: 10.1016/j.ccell.2022.02.003
56. Balkwill F. Tumour necrosis factor and cancer. Nat Rev Cancer. (2009) 9:361–71. doi: 10.1038/nrc2628
57. Weigelin B, Friedl P. T cell-mediated additive cytotoxicity - death by multiple bullets. Trends Cancer. (2022) 8:980–7. doi: 10.1016/j.trecan.2022.07.007
58. Fischer K, Hoffmann P, Voelkl S, Meidenbauer N, Ammer J, Edinger M, et al. Inhibitory effect of tumor cell-derived lactic acid on human T cells. Blood. (2007) 109:3812–9. doi: 10.1182/blood-2006-07-035972
59. Lin R, Chen L, Chen G, Hu C, Jiang S, Sevilla J, et al. Targeting miR-23a in CD8+ cytotoxic T lymphocytes prevents tumor-dependent immunosuppression. J Clin Invest. (2014) 124:5352–67. doi: 10.1172/JCI76561
60. Kallies A, Xin A, Belz GT, Nutt SL. Blimp-1 transcription factor is required for the differentiation of effector CD8(+) T cells and memory responses. Immunity. (2009) 31:283–95. doi: 10.1016/j.immuni.2009.06.021
61. Motz GT, Santoro SP, Wang LP, Garrabrant T, Lastra RR, Hagemann IS, et al. Tumor endothelium FasL establishes a selective immune barrier promoting tolerance in tumors. Nat Med. (2014) 20:607–15. doi: 10.1038/nm.3541
62. Muro R, Takayanagi H, Nitta T. T cell receptor signaling for γδT cell development. Inflammation Regener. (2019) 39:6. doi: 10.1186/s41232-019-0095-z
63. Wang X, Zhang Y, Chung Y, Tu CR, Zhang W, Mu X, et al. Tumor vaccine based on extracellular vesicles derived from gammadelta-T cells exerts dual antitumor activities. J Extracell Vesicles. (2023) 12:e12360. doi: 10.1002/jev2.12360
64. Alexander AA, Maniar A, Cummings JS, Hebbeler AM, Schulze DH, Gastman BR, et al. Isopentenyl pyrophosphate-activated CD56+ {gamma}{delta} T lymphocytes display potent antitumor activity toward human squamous cell carcinoma. Clin Cancer Res. (2008) 14:4232–40. doi: 10.1158/1078-0432.CCR-07-4912
65. Dhar S, Chiplunkar SV. Lysis of aminobisphosphonate-sensitized MCF-7 breast tumor cells by Vγ9Vδ2 T cells. Cancer Immun. (2010) 10:10.
66. Viey E, Fromont G, Escudier B, Morel Y, Da Rocha S, Chouaib S, et al. Phosphostim-activated gamma delta T cells kill autologous metastatic renal cell carcinoma. J Immunol. (2005) 174:1338–47. doi: 10.4049/jimmunol.174.3.1338
67. Sandoz PA, Kuhnigk K, Szabo EK, Thunberg S, Erikson E, Sandström N, et al. Modulation of lytic molecules restrain serial killing in γδ T lymphocytes. Nat Commun. (2023) 14:6035. doi: 10.1038/s41467-023-41634-7
68. Jalali S, Stankovic S, Westall GP, Reading PC, Sullivan LC, Brooks AG. Examining the impact of immunosuppressive drugs on antibody-dependent cellular cytotoxicity (ADCC) of human peripheral blood natural killer (NK) cells and gamma delta (gammadelta) T cells. Transpl Immunol. (2024) 82:101962. doi: 10.1016/j.trim.2023.101962
69. Fattori S, Gorvel L, Granjeaud S, Rochigneux P, Rouviere MS, Ben Amara A, et al. Quantification of immune variables from liquid biopsy in breast cancer patients links vdelta2(+) gammadelta T cell alterations with lymph node invasion. Cancers (Basel). (2021) 13:441. doi: 10.3390/cancers13030441
70. Mandelboim O, Malik P, Davis DM, Jo CH, Boyson JE, Strominger JL. Human CD16 as a lysis receptor mediating direct natural killer cell cytotoxicity. Proc Natl Acad Sci U.S.A. (1999) 96:5640–4. doi: 10.1073/pnas.96.10.5640
71. Lafont V, Liautard J, Liautard JP, Favero J. Production of TNF-alpha by human V gamma 9V delta 2 T cells via engagement of Fc gamma RIIIA, the low affinity type 3 receptor for the Fc portion of IgG, expressed upon TCR activation by nonpeptidic antigen. J Immunol. (2001) 166:7190–9. doi: 10.4049/jimmunol.166.12.7190
72. Lee D, Rosenthal CJ, Penn NE, Dunn ZS, Zhou Y, Yang L. Human γδ T cell subsets and their clinical applications for cancer immunotherapy. Cancers (Basel). (2022) 14:3005. doi: 10.3390/cancers14123005
73. Wang X, Zhang Y, Mu X, Tu CR, Chung Y, Tsao SW, et al. Exosomes derived from gammadelta-T cells synergize with radiotherapy and preserve antitumor activities against nasopharyngeal carcinoma in immunosuppressive microenvironment. J Immunother Cancer. (2022) 10:e003832. doi: 10.1136/jitc-2021-003832
74. Tawfik D, Groth C, Gundlach JP, Peipp M, Kabelitz D, Becker T, et al. TRAIL-receptor 4 modulates gammadelta T cell-cytotoxicity toward cancer cells. Front Immunol. (2019) 10:2044. doi: 10.3389/fimmu.2019.02044
75. Dieli F, Vermijlen D, Fulfaro F, Caccamo N, Meraviglia S, Cicero G, et al. Targeting human {gamma}delta} T cells with zoledronate and interleukin-2 for immunotherapy of hormone-refractory prostate cancer. Cancer Res. (2007) 67:7450–7. doi: 10.1158/0008-5472.CAN-07-0199
76. Li Z, Xu Q, Peng H, Cheng R, Sun Z, Ye Z. IFN-γ enhances HOS and U2OS cell lines susceptibility to γδ T cell-mediated killing through the Fas/Fas ligand pathway. Int Immunopharmacol. (2011) 11:496–503. doi: 10.1016/j.intimp.2011.01.001
77. Ramstead AG, Jutila MA. Complex role of γδ T-cell-derived cytokines and growth factors in cancer. J Interferon Cytokine Res. (2012) 32:563–9. doi: 10.1089/jir.2012.0073
78. Zhu Y, Zhang S, Li Z, Wang H, Li Z, Hu Y, et al. miR-125b-5p and miR-99a-5p downregulate human γδ T-cell activation and cytotoxicity. Cell Mol Immunol. (2019) 16:112–25. doi: 10.1038/cmi.2017.164
79. Li H, Luo K, Pauza CD. TNF-alpha is a positive regulatory factor for human Vgamma2 Vdelta2 T cells. J Immunol. (2008) 181:7131–7. doi: 10.4049/jimmunol.181.10.7131
80. Mace EM, Dongre P, Hsu HT, Sinha P, James AM, Mann SS, et al. Cell biological steps and checkpoints in accessing NK cell cytotoxicity. Immunol Cell Biol. (2014) 92:245–55. doi: 10.1038/icb.2013.96
81. Vivier E, Rebuffet L, Narni-Mancinelli E, Cornen S, Igarashi RY, Fantin VR. Natural killer cell therapies. Nature. (2024) 626:727–36. doi: 10.1038/s41586-023-06945-1
82. Smyth MJ, Thia KY, Street SE, MacGregor D, Godfrey DI, Trapani JA. Perforin-mediated cytotoxicity is critical for surveillance of spontaneous lymphoma. J Exp Med. (2000) 192:755–60. doi: 10.1084/jem.192.5.755
83. van den Broek ME, Kägi D, Ossendorp F, Toes R, Vamvakas S, Lutz WK, et al. Decreased tumor surveillance in perforin-deficient mice. J Exp Med. (1996) 184:1781–90. doi: 10.1084/jem.184.5.1781
84. Smyth MJ, Thia KY, Cretney E, Kelly JM, Snook MB, Forbes CA, et al. Perforin is a major contributor to NK cell control of tumor metastasis. J Immunol. (1999) 162:6658–62. doi: 10.4049/jimmunol.162.11.6658
85. Takeda K, Hayakawa Y, Smyth MJ, Kayagaki N, Yamaguchi N, Kakuta S, et al. Involvement of tumor necrosis factor-related apoptosis-inducing ligand in surveillance of tumor metastasis by liver natural killer cells. Nat Med. (2001) 7:94–100. doi: 10.1038/83416
86. Parseh B, Khosravi A, Fazel A, Ai J, Ebrahimi-Barough S, Verdi J, et al. 3-dimensional model to study apoptosis induction of activated natural killer cells conditioned medium using patient-derived colorectal cancer organoids. Front Cell Dev Biol. (2022) 10:895284. doi: 10.3389/fcell.2022.895284
87. Wallin RP, Screpanti V, Michaëlsson J, Grandien A, Ljunggren HG. Regulation of perforin-independent NK cell-mediated cytotoxicity. Eur J Immunol. (2003) 33:2727–35. doi: 10.1002/eji.200324070
88. Pang Z, Wang Z, Li F, Feng C, Mu X. Current progress of CAR-NK therapy in cancer treatment. Cancers (Basel). (2022) 14:4318. doi: 10.3390/cancers14174318
89. Gocher AM, Workman CJ, Vignali DAA. Interferon-gamma: teammate or opponent in the tumour microenvironment? Nat Rev Immunol. (2022) 22:158–72. doi: 10.1038/s41577-021-00566-3
90. Street SE, Cretney E, Smyth MJ. Perforin and interferon-gamma activities independently control tumor initiation, growth, and metastasis. Blood. (2001) 97:192–7. doi: 10.1182/blood.V97.1.192
91. Brand A, Singer K, Koehl GE, Kolitzus M, Schoenhammer G, Thiel A, et al. LDHA-associated lactic acid production blunts tumor immunosurveillance by T and NK cells. Cell Metab. (2016) 24:657–71. doi: 10.1016/j.cmet.2016.08.011
92. Husain Z, Huang Y, Seth P, Sukhatme VP. Tumor-derived lactate modifies antitumor immune response: effect on myeloid-derived suppressor cells and NK cells. J Immunol. (2013) 191:1486–95. doi: 10.4049/jimmunol.1202702
93. Ruf B, Greten TF, Korangy F. Innate lymphoid cells and innate-like T cells in cancer - at the crossroads of innate and adaptive immunity. Nat Rev Cancer. (2023) 23:351–71. doi: 10.1038/s41568-023-00562-w
94. Siegler JJ, Correia MP, Hofman T, Prager I, Birgin E, Rahbari NN, et al. Human ILC3 exert TRAIL-mediated cytotoxicity towards cancer cells. Front Immunol. (2022) 13:742571. doi: 10.3389/fimmu.2022.742571
95. Wu K, Lin K, Li X, Yuan X, Xu P, Ni P, et al. Redefining tumor-associated macrophage subpopulations and functions in the tumor microenvironment. Front Immunol. (2020) 11:1731. doi: 10.3389/fimmu.2020.01731
96. Bill R, Wirapati P, Messemaker M, Roh W, Zitti B, Duval F, et al. CXCL9:SPP1 macrophage polarity identifies a network of cellular programs that control human cancers. Science. (2023) 381:515–24. doi: 10.1126/science.ade2292
97. Wu J, Shen Y, Zeng G, Liang Y, Liao G. SPP1+ TAM subpopulations in tumor microenvironment promote intravasation and metastasis of head and neck squamous cell carcinoma. Cancer Gene Ther. (2023) 31:311–21. doi: 10.1038/s41417-023-00704-0
98. Su X, Liang C, Chen R, Duan S. Deciphering tumor microenvironment: CXCL9 and SPP1 as crucial determinants of tumor-associated macrophage polarity and prognostic indicators. Mol Cancer. (2024) 23:13. doi: 10.1186/s12943-023-01931-7
99. Pan Y, Yu Y, Wang X, Zhang T. Tumor-associated macrophages in tumor immunity. Front Immunol. (2020) 11:583084. doi: 10.3389/fimmu.2020.583084
100. Bernsmeier C, van der Merwe S, Perianin A. Innate immune cells in cirrhosis. J Hepatol. (2020) 73:186–201. doi: 10.1016/j.jhep.2020.03.027
101. Paul S, Chhatar S, Mishra A, Lal G. Natural killer T cell activation increases iNOS(+)CD206<(>-<)> M1 macrophage and controls the growth of solid tumor. J Immunother Cancer. (2019) 7:208. doi: 10.1186/s40425-019-0697-7
102. Kulshrestha A, Katara GK, Ibrahim SA, Riehl VE, Schneiderman S, Bilal M, et al. In vivo anti-V-ATPase antibody treatment delays ovarian tumor growth by increasing antitumor immune responses. Mol Oncol. (2020) 14:2436–54. doi: 10.1002/1878-0261.12782
103. Mantovani A, Allavena P, Marchesi F, Garlanda C. Macrophages as tools and targets in cancer therapy. Nat Rev Drug Discovery. (2022) 21:799–820. doi: 10.1038/s41573-022-00520-5
104. Bruns H, Buttner M, Fabri M, Mougiakakos D, Bittenbring JT, Hoffmann MH, et al. Vitamin D-dependent induction of cathelicidin in human macrophages results in cytotoxicity against high-grade B cell lymphoma. Sci Transl Med. (2015) 7:282ra47. doi: 10.1126/scitranslmed.aaa3230
105. Cruceriu D, Baldasici O, Balacescu O, Berindan-Neagoe I. The dual role of tumor necrosis factor-alpha (TNF-alpha) in breast cancer: molecular insights and therapeutic approaches. Cell Oncol (Dordr). (2020) 43:1–18. doi: 10.1007/s13402-019-00489-1
106. Seynhaeve AL, Hoving S, Schipper D, Vermeulen CE, de Wiel-Ambagtsheer G, van Tiel ST, et al. Tumor necrosis factor alpha mediates homogeneous distribution of liposomes in murine melanoma that contributes to a better tumor response. Cancer Res. (2007) 67:9455–62. doi: 10.1158/0008-5472.CAN-07-1599
107. Jackson DJ, Akuthota P, Roufosse F. Eosinophils and eosinophilic immune dysfunction in health and disease. Eur Respir Rev. (2022) 31:210150. doi: 10.1183/16000617.0150-2021
108. Varricchi G, Galdiero MR, Loffredo S, Lucarini V, Marone G, Mattei F, et al. Eosinophils: The unsung heroes in cancer? Oncoimmunology. (2018) 7:e1393134. doi: 10.1080/2162402X.2017.1393134
109. Andreone S, Spadaro F, Buccione C, Mancini J, Tinari A, Sestili P, et al. IL-33 promotes CD11b/CD18-mediated adhesion of eosinophils to cancer cells and synapse-polarized degranulation leading to tumor cell killing. Cancers (Basel). (2019) 11:1664. doi: 10.3390/cancers11111664
110. Legrand F, Driss V, Delbeke M, Loiseau S, Hermann E, Dombrowicz D, et al. Human eosinophils exert TNF-α and granzyme A-mediated tumoricidal activity toward colon carcinoma cells. J Immunol. (2010) 185:7443–51. doi: 10.4049/jimmunol.1000446
111. Costain DJ, Guha AK, Liwski RS, Lee TD. Murine hypodense eosinophils induce tumour cell apoptosis by a granzyme B-dependent mechanism. Cancer Immunol Immunother. (2001) 50:293–9. doi: 10.1007/PL00006690
112. Shang Q, Yu X, Sun Q, Li H, Sun C, Liu L. Polysaccharides regulate Th1/Th2 balance: A new strategy for tumor immunotherapy. BioMed Pharmacother. (2024) 170:115976. doi: 10.1016/j.biopha.2023.115976
113. Simson L, Ellyard JI, Dent LA, Matthaei KI, Rothenberg ME, Foster PS, et al. Regulation of carcinogenesis by IL-5 and CCL11: a potential role for eosinophils in tumor immune surveillance. J Immunol. (2007) 178:4222–9. doi: 10.4049/jimmunol.178.7.4222
114. Ghaffari S, Rezaei N. Eosinophils in the tumor microenvironment: implications for cancer immunotherapy. J Transl Med. (2023) 21:551. doi: 10.1186/s12967-023-04418-7
115. Kataoka S, Konishi Y, Nishio Y, Fujikawa-Adachi K, Tominaga A. Antitumor activity of eosinophils activated by IL-5 and eotaxin against hepatocellular carcinoma. DNA Cell Biol. (2004) 23:549–60. doi: 10.1089/1044549041939214
116. Jacenik D, Karagiannidis I, Beswick EJ. Th2 cells inhibit growth of colon and pancreas cancers by promoting anti-tumorigenic responses from macrophages and eosinophils. Br J Cancer. (2023) 128:387–97. doi: 10.1038/s41416-022-02056-2
117. Schreiber S, Hammers CM, Kaasch AJ, Schraven B, Dudeck A, Kahlfuss S. Metabolic interdependency of th2 cell-mediated type 2 immunity and the tumor microenvironment. Front Immunol. (2021) 12:632581. doi: 10.3389/fimmu.2021.632581
118. Chen J, Gong C, Mao H, Li Z, Fang Z, Chen Q, et al. E2F1/SP3/STAT6 axis is required for IL-4-induced epithelial-mesenchymal transition of colorectal cancer cells. Int J Oncol. (2018) 53:567–78. doi: 10.3892/ijo
119. Prokopchuk O, Liu Y, Henne-Bruns D, Kornmann M. Interleukin-4 enhances proliferation of human pancreatic cancer cells: evidence for autocrine and paracrine actions. Br J Cancer. (2005) 92:921–8. doi: 10.1038/sj.bjc.6602416
120. Traub B, Sun L, Ma Y, Xu P, Lemke J, Paschke S, et al. Endogenously expressed IL-4Ralpha promotes the Malignant phenotype of human pancreatic cancer in vitro and in vivo. Int J Mol Sci. (2017) 18:716. doi: 10.3390/ijms18040716
121. Alam A, Levanduski E, Denz P, Villavicencio HS, Bhatta M, Alhorebi L, et al. Fungal mycobiome drives IL-33 secretion and type 2 immunity in pancreatic cancer. Cancer Cell. (2022) 40:153–167 e11. doi: 10.1016/j.ccell.2022.01.003
122. Roostaee A, Yaghobi R, Afshari A, Jafarinia M. Regulatory role of T helper 9/interleukin-9: Transplantation view. Heliyon. (2024) 10:e26359. doi: 10.1016/j.heliyon.2024.e26359
123. Lv X, Wang X. The role of interleukin-9 in lymphoma. Leuk Lymphoma. (2013) 54:1367–72. doi: 10.3109/10428194.2012.745072
124. Tian L, Li Y, Chang R, Zhang P, Zhang J, Huo L. Lentiviral vector-mediated IL-9 overexpression stimulates cell proliferation by targeting c-myc and cyclin D1 in colitis-associated cancer. Oncol Lett. (2019) 17:175–82. doi: 10.3892/ol.2018.9567
125. Fontana R, Mestre-Farrera A, Yang J. Update on epithelial-mesenchymal plasticity in cancer progression. Annu Rev Pathol. (2024) 19:133–56. doi: 10.1146/annurev-pathmechdis-051222-122423
126. Salazar Y, Zheng X, Brunn D, Raifer H, Picard F, Zhang Y, et al. Microenvironmental Th9 and Th17 lymphocytes induce metastatic spreading in lung cancer. J Clin Invest. (2020) 130:3560–75. doi: 10.1172/JCI124037
127. Tan H, Wang S, Zhao L. A tumour-promoting role of Th9 cells in hepatocellular carcinoma through CCL20 and STAT3 pathways. Clin Exp Pharmacol Physiol. (2017) 44:213–21. doi: 10.1111/1440-1681.12689
128. Kono M. New insights into the metabolism of Th17 cells. Immunol Med. (2023) 46:15–24. doi: 10.1080/25785826.2022.2140503
129. Pan Y, Yang W, Tang B, Wang X, Zhang Q, Li W, et al. The protective and pathogenic role of Th17 cell plasticity and function in the tumor microenvironment. Front Immunol. (2023) 14:1192303. doi: 10.3389/fimmu.2023.1192303
130. Bi L, Wu J, Ye A, Wu J, Yu K, Zhang S, et al. Increased Th17 cells and IL-17A exist in patients with B cell acute lymphoblastic leukemia and promote proliferation and resistance to daunorubicin through activation of Akt signaling. J Transl Med. (2016) 14:132. doi: 10.1186/s12967-016-0894-9
131. De Simone V, Franzè E, Ronchetti G, Colantoni A, Fantini MC, Di Fusco D, et al. Th17-type cytokines, IL-6 and TNF-α synergistically activate STAT3 and NF-kB to promote colorectal cancer cell growth. Oncogene. (2015) 34:3493–503. doi: 10.1038/onc.2014.286
132. Liu X, Wang X, Yang Q, Luo L, Liu Z, Ren X, et al. Th17 cells secrete TWEAK to trigger epithelial-mesenchymal transition and promote colorectal cancer liver metastasis. Cancer Res. (2024) 84:1352–71. doi: 10.1158/0008-5472.CAN-23-2123
133. Peng DH, Rodriguez BL, Diao L, Gaudreau PO, Padhye A, Konen JM, et al. Th17 cells contribute to combination MEK inhibitor and anti-PD-L1 therapy resistance in KRAS/p53 mutant lung cancers. Nat Commun. (2021) 12:2606. doi: 10.1038/s41467-021-22875-w
134. Wang R, Yang L, Zhang C, Wang R, Zhang Z, He Q, et al. Th17 cell-derived IL-17A promoted tumor progression via STAT3/NF-κB/Notch1 signaling in non-small cell lung cancer. Oncoimmunology. (2018) 7:e1461303. doi: 10.1080/2162402X.2018.1461303
135. Numasaki M, Fukushi J, Ono M, Narula SK, Zavodny PJ, Kudo T, et al. Interleukin-17 promotes angiogenesis and tumor growth. Blood. (2003) 101:2620–7. doi: 10.1182/blood-2002-05-1461
136. Chung AS, Wu X, Zhuang G, Ngu H, Kasman I, Zhang J, et al. An interleukin-17-mediated paracrine network promotes tumor resistance to anti-angiogenic therapy. Nat Med. (2013) 19:1114–23. doi: 10.1038/nm.3291
137. Protopsaltis NJ, Liang W, Nudleman E, Ferrara N. Interleukin-22 promotes tumor angiogenesis. Angiogenesis. (2019) 22:311–23. doi: 10.1007/s10456-018-9658-x
138. Kessenbrock K, Plaks V, Werb Z. Matrix metalloproteinases: regulators of the tumor microenvironment. Cell. (2010) 141:52–67. doi: 10.1016/j.cell.2010.03.015
139. Li J, Lau GK, Chen L, Dong SS, Lan HY, Huang XR, et al. Interleukin 17A promotes hepatocellular carcinoma metastasis via NF-kB induced matrix metalloproteinases 2 and 9 expression. PloS One. (2011) 6:e21816. doi: 10.1371/journal.pone.0021816
140. Hovav AH, Wilensky A. The role of the epithelial sentinels, Langerhans cells and gammadeltaT cells, in oral squamous cell carcinoma. Periodontol 2000. (2024), 1–8. doi: 10.1111/prd.12544
141. Zhu R, Yan Q, Wang Y, Wang K. Biological characteristics of gammadeltaT cells and application in tumor immunotherapy. Front Genet. (2022) 13:1077419. doi: 10.3389/fgene.2022.1077419
142. Li Y, Li G, Zhang J, Wu X, Chen X. The dual roles of human γδ T cells: anti-tumor or tumor-promoting. Front Immunol. (2020) 11:619954. doi: 10.3389/fimmu.2020.619954
143. Kühl AA, Pawlowski NN, Grollich K, Blessenohl M, Westermann J, Zeitz M, et al. Human peripheral gammadelta T cells possess regulatory potential. Immunology. (2009) 128:580–8. doi: 10.1111/j.1365-2567.2009.03162.x
144. Lin EY, Pollard JW. Macrophages: modulators of breast cancer progression. Novartis Found Symp. (2004) 256:158–68.
145. Zhou Y, Qian M, Li J, Ruan L, Wang Y, Cai C, et al. The role of tumor-associated macrophages in lung cancer: From mechanism to small molecule therapy. BioMed Pharmacother. (2024) 170:116014. doi: 10.1016/j.biopha.2023.116014
146. Cao R, Ji H, Yang Y, Cao Y. Collaborative effects between the TNFalpha-TNFR1-macrophage axis and the VEGF-C-VEGFR3 signaling in lymphangiogenesis and metastasis. Oncoimmunology. (2015) 4:e989777. doi: 10.4161/2162402X.2014.989777
147. Murdoch C, Muthana M, Coffelt SB, Lewis CE. The role of myeloid cells in the promotion of tumour angiogenesis. Nat Rev Cancer. (2008) 8:618–31. doi: 10.1038/nrc2444
148. Ojalvo LS, King W, Cox D, Pollard JW. High-density gene expression analysis of tumor-associated macrophages from mouse mammary tumors. Am J Pathol. (2009) 174:1048–64. doi: 10.2353/ajpath.2009.080676
149. Chen P, Huang Y, Bong R, Ding Y, Song N, Wang X, et al. Tumor-associated macrophages promote angiogenesis and melanoma growth via adrenomedullin in a paracrine and autocrine manner. Clin Cancer Res. (2011) 17:7230–9. doi: 10.1158/1078-0432.CCR-11-1354
150. Lin L, Chen YS, Yao YD, Chen JQ, Chen JN, Huang SY, et al. CCL18 from tumor-associated macrophages promotes angiogenesis in breast cancer. Oncotarget. (2015) 6:34758–73. doi: 10.18632/oncotarget.v6i33
151. Jakab M, Rostalski T, Lee KH, Mogler C, Augustin HG. Tie2 receptor in tumor-infiltrating macrophages is dispensable for tumor angiogenesis and tumor relapse after chemotherapy. Cancer Res. (2022) 82:1353–64. doi: 10.1158/0008-5472.CAN-21-3181
152. Chen Y, Tan W, Wang C. Tumor-associated macrophage-derived cytokines enhance cancer stem-like characteristics through epithelial-mesenchymal transition. Onco Targets Ther. (2018) 11:3817–26. doi: 10.2147/OTT
153. Fu XT, Dai Z, Song K, Zhang ZJ, Zhou ZJ, Zhou SL, et al. Macrophage-secreted IL-8 induces epithelial-mesenchymal transition in hepatocellular carcinoma cells by activating the JAK2/STAT3/Snail pathway. Int J Oncol. (2015) 46:587–96. doi: 10.3892/ijo.2014.2761
154. Yang J, Liao D, Chen C, Liu Y, Chuang TH, Xiang R, et al. Tumor-associated macrophages regulate murine breast cancer stem cells through a novel paracrine EGFR/Stat3/Sox-2 signaling pathway. Stem Cells. (2013) 31:248–58. doi: 10.1002/stem.1281
155. Zhou N, Zhang Y, Zhang X, Lei Z, Hu R, Li H, et al. Exposure of tumor-associated macrophages to apoptotic MCF-7 cells promotes breast cancer growth and metastasis. Int J Mol Sci. (2015) 16:11966–82. doi: 10.3390/ijms160611966
156. Leu CM, Wong FH, Chang C, Huang SF, Hu CP. Interleukin-6 acts as an antiapoptotic factor in human esophageal carcinoma cells through the activation of both STAT3 and mitogen-activated protein kinase pathways. Oncogene. (2003) 22:7809–18. doi: 10.1038/sj.onc.1207084
157. Zhao T, Su Z, Li Y, Zhang X, You Q. Chitinase-3 like-protein-1 function and its role in diseases. Signal Transduct Target Ther. (2020) 5:201. doi: 10.1038/s41392-020-00303-7
158. Blache U, Weiss R, Boldt A, Kapinsky M, Blaudszun AR, Quaiser A, et al. Advanced flow cytometry assays for immune monitoring of CAR-T cell applications. Front Immunol. (2021) 12:658314. doi: 10.3389/fimmu.2021.658314
159. Lahmar Q, Keirsse J, Laoui D, Movahedi K, Van Overmeire E, Van Ginderachter JA. Tissue-resident versus monocyte-derived macrophages in the tumor microenvironment. Biochim Biophys Acta. (2016) 1865:23–34. doi: 10.1016/j.bbcan.2015.06.009
160. Cheng Y, Zhu Y, Xu J, Yang M, Chen P, Xu W, et al. PKN2 in colon cancer cells inhibits M2 phenotype polarization of tumor-associated macrophages via regulating DUSP6-Erk1/2 pathway. Mol Cancer. (2018) 17:13. doi: 10.1186/s12943-017-0747-z
161. Conti I, Rollins BJ. CCL2 (monocyte chemoattractant protein-1) and cancer. Semin Cancer Biol. (2004) 14:149–54. doi: 10.1016/j.semcancer.2003.10.009
162. Qian BZ, Li J, Zhang H, Kitamura T, Zhang J, Campion LR, et al. CCL2 recruits inflammatory monocytes to facilitate breast-tumour metastasis. Nature. (2011) 475:222–5. doi: 10.1038/nature10138
163. Schwingshackl A, Duszyk M, Brown N, Moqbel R. Human eosinophils release matrix metalloproteinase-9 on stimulation with TNF-alpha. J Allergy Clin Immunol. (1999) 104:983–9. doi: 10.1016/S0091-6749(99)70079-5
164. Puxeddu I, Berkman N, Nissim Ben Efraim AH, Davies DE, Ribatti D, Gleich GJ, et al. The role of eosinophil major basic protein in angiogenesis. Allergy. (2009) 64:368–74. doi: 10.1111/j.1398-9995.2008.01822.x
165. Panagopoulos V, Leach DA, Zinonos I, Ponomarev V, Licari G, Liapis V, et al. Inflammatory peroxidases promote breast cancer progression in mice via regulation of the tumour microenvironment. Int J Oncol. (2017) 50:1191–200. doi: 10.3892/ijo.2017.3883
166. Li F, Du X, Lan F, Li N, Zhang C, Zhu C, et al. Eosinophilic inflammation promotes CCL6-dependent metastatic tumor growth. Sci Adv. (2021) 7:eabb5943. doi: 10.1126/sciadv.abb5943
167. Groeger S, Meyle J. The role of programmed death receptor (PD-)1/PD-ligand (L)1 in periodontitis and cancer. Periodontol. (2024) 2000:1–20. doi: 10.1111/prd.12548
168. Burke KP, Chaudhri A, Freeman GJ, Sharpe AH. The B7:CD28 family and friends: Unraveling coinhibitory interactions. Immunity. (2024) 57:223–44. doi: 10.1016/j.immuni.2024.01.013
169. Qin S, Xu L, Yi M, Yu S, Wu K, Luo S. Novel immune checkpoint targets: moving beyond PD-1 and CTLA-4. Mol Cancer. (2019) 18:155. doi: 10.1186/s12943-019-1091-2
170. Bagchi S, Yuan R, Engleman EG. Immune checkpoint inhibitors for the treatment of cancer: clinical impact and mechanisms of response and resistance. Annu Rev Pathol. (2021) 16:223–49. doi: 10.1146/annurev-pathol-042020-042741
171. Galle P, Finn RS, Mitchell CR, Ndirangu K, Ramji Z, Redhead GS, et al. Treatment-emergent antidrug antibodies related to PD-1, PD-L1, or CTLA-4 inhibitors across tumor types: a systematic review. J Immunother Cancer. (2024) 12:e008266. doi: 10.1136/jitc-2023-008266
172. Okazaki T, Honjo T. PD-1 and PD-1 ligands: from discovery to clinical application. Int Immunol. (2007) 19:813–24. doi: 10.1093/intimm/dxm057
173. Musolino A, Gradishar WJ, Rugo HS, Nordstrom JL, Rock EP, Arnaldez F, et al. Role of Fcγ receptors in HER2-targeted breast cancer therapy. J Immunother Cancer. (2022) 10:e003171. doi: 10.1136/jitc-2021-003171
174. Clynes RA, Towers TL, Presta LG, Ravetch JV. Inhibitory Fc receptors modulate in vivo cytotoxicity against tumor targets. Nat Med. (2000) 6:443–6. doi: 10.1038/74704
175. Seimetz D, Lindhofer H, Bokemeyer C. Development and approval of the trifunctional antibody catumaxomab (anti-EpCAM x anti-CD3) as a targeted cancer immunotherapy. Cancer Treat Rev. (2010) 36:458–67. doi: 10.1016/j.ctrv.2010.03.001
176. Maskalenko NA, Zhigarev D, Campbell KS. Harnessing natural killer cells for cancer immunotherapy: dispatching the first responders. Nat Rev Drug Discovery. (2022) 21:559–77. doi: 10.1038/s41573-022-00413-7
177. Meng F, Zhang S, Xie J, Zhou Y, Wu Q, Lu B, et al. Leveraging CD16 fusion receptors to remodel the immune response for enhancing anti-tumor immunotherapy in iPSC-derived NK cells. J Hematol Oncol. (2023) 16:62. doi: 10.1186/s13045-023-01455-z
178. Desroys du Roure P, Lajoie L, Mallavialle A, Alcaraz LB, Mansouri H, Fenou L, et al. A novel Fc-engineered cathepsin D-targeting antibody enhances ADCC, triggers tumor-infiltrating NK cell recruitment, and improves treatment with paclitaxel and enzalutamide in triple-negative breast cancer. J Immunother Cancer. (2024) 12:e007135. doi: 10.1136/jitc-2023-007135
179. Zhang B, Shi J, Shi X, Xu X, Gao L, Li S, et al. Development and evaluation of a human CD47/HER2 bispecific antibody for Trastuzumab-resistant breast cancer immunotherapy. Drug Resist Update. (2024) 74:101068. doi: 10.1016/j.drup.2024.101068
180. Sterner RC, Sterner RM. CAR-T cell therapy: current limitations and potential strategies. Blood Cancer J. (2021) 11:69. doi: 10.1038/s41408-021-00459-7
181. Pinheiro PF, Justino GC, Marques MM. NKp30 - A prospective target for new cancer immunotherapy strategies. Br J Pharmacol. (2020) 177:4563–80. doi: 10.1111/bph.15222
182. Zhang Z, Liu C, Yang Z, Yin H. CAR-T-cell therapy for solid tumors positive for fibronectin extra domain B. Cells. (2022) 11:2863. doi: 10.3390/cells11182863
183. Czaplicka A, Lachota M, Paczek L, Zagozdzon R, Kaleta B. Chimeric antigen receptor T cell therapy for pancreatic cancer: A review of current evidence. Cells. (2024) 13:101. doi: 10.3390/cells13010101
184. Gao Z, Bai Y, Lin A, Jiang A, Zhou C, Cheng Q, et al. Gamma delta T-cell-based immune checkpoint therapy: attractive candidate for antitumor treatment. Mol Cancer. (2023) 22:31. doi: 10.1186/s12943-023-01722-0
185. Castella B, Foglietta M, Sciancalepore P, Rigoni M, Coscia M, Griggio V, et al. Anergic bone marrow Vgamma9Vdelta2 T cells as early and long-lasting markers of PD-1-targetable microenvironment-induced immune suppression in human myeloma. Oncoimmunology. (2015) 4:e1047580. doi: 10.1080/2162402X.2015.1047580
186. Nguyen S, Chevalier MF, Benmerzoug S, Cesson V, Schneider AK, Rodrigues-Dias SC, et al. Vdelta2 T cells are associated with favorable clinical outcomes in patients with bladder cancer and their tumor reactivity can be boosted by BCG and zoledronate treatments. J Immunother Cancer. (2022) 10:e004880. doi: 10.1136/jitc-2022-004880
187. Bednar C, Kubel S, Cordsmeier A, Scholz B, Menschikowski H, Ensser A. A genetically encoded dark-to-bright biosensor for visualisation of granzyme-mediated cytotoxicity. Int J Mol Sci. (2023) 24:13589. doi: 10.3390/ijms241713589
188. Benmebarek MR, Karches CH, Cadilha BL, Lesch S, Endres S, Kobold S. Killing mechanisms of chimeric antigen receptor (CAR) T cells. Int J Mol Sci. (2019) 20:1283. doi: 10.3390/ijms20061283
189. Poulaki V, Mitsiades CS, Mitsiades N. The role of Fas and FasL as mediators of anticancer chemotherapy. Drug Resist Update. (2001) 4:233–42. doi: 10.1054/drup.2001.0210
190. Risso V, Lafont E, Le Gallo M. Therapeutic approaches targeting CD95L/CD95 signaling in cancer and autoimmune diseases. Cell Death Dis. (2022) 13:248. doi: 10.1038/s41419-022-04688-x
191. Villa-Morales M, Fernández-Piqueras J. Targeting the Fas/FasL signaling pathway in cancer therapy. Expert Opin Ther Targets. (2012) 16:85–101. doi: 10.1517/14728222.2011.628937
192. Bekisz J, Sato Y, Johnson C, Husain SR, Puri RK, Zoon KC. Immunomodulatory effects of interferons in Malignancies. J Interferon Cytokine Res. (2013) 33:154–61. doi: 10.1089/jir.2012.0167
193. Verhoeven Y, Tilborghs S, Jacobs J, De Waele J, Quatannens D, Deben C, et al. The potential and controversy of targeting STAT family members in cancer. Semin Cancer Biol. (2020) 60:41–56. doi: 10.1016/j.semcancer.2019.10.002
194. Wang W, Lopez McDonald MC, Hariprasad R, Hamilton T, Frank DA. Oncogenic STAT transcription factors as targets for cancer therapy: innovative strategies and clinical translation. Cancers (Basel). (2024) 16:1387. doi: 10.3390/cancers16071387
195. Hayakawa F, Sugimoto K, Harada Y, Hashimoto N, Ohi N, Kurahashi S, et al. A novel STAT inhibitor, OPB-31121, has a significant antitumor effect on leukemia with STAT-addictive oncokinases. Blood Cancer J. (2013) 3:e166. doi: 10.1038/bcj.2013.63
196. Shibue T, Weinberg RA. EMT, CSCs, and drug resistance: the mechanistic link and clinical implications. Nat Rev Clin Oncol. (2017) 14:611–29. doi: 10.1038/nrclinonc.2017.44
197. Ye X, Weinberg RA. Epithelial-mesenchymal plasticity: A central regulator of cancer progression. Trends Cell Biol. (2015) 25:675–86. doi: 10.1016/j.tcb.2015.07.012
198. Soundararajan R, Fradette JJ, Konen JM, Moulder S, Zhang X, Gibbons DL, et al. Targeting the interplay between epithelial-to-mesenchymal-transition and the immune system for effective immunotherapy. Cancers (Basel). (2019) 11:714. doi: 10.3390/cancers11050714
199. Huang Y, Hong W, Wei X. The molecular mechanisms and therapeutic strategies of EMT in tumor progression and metastasis. J Hematol Oncol. (2022) 15:129. doi: 10.1186/s13045-022-01347-8
200. Imodoye SO, Adedokun KA. EMT-induced immune evasion: connecting the dots from mechanisms to therapy. Clin Exp Med. (2023) 23:4265–87. doi: 10.1007/s10238-023-01229-4
201. Herbertz S, Sawyer JS, Stauber AJ, Gueorguieva I, Driscoll KE, Estrem ST, et al. Clinical development of galunisertib (LY2157299 monohydrate), a small molecule inhibitor of transforming growth factor-beta signaling pathway. Drug Des Devel Ther. (2015) 9:4479–99. doi: 10.2147/DDDT
202. Bu MT, Chandrasekhar P, Ding L, Hugo W. The roles of TGF-beta and VEGF pathways in the suppression of antitumor immunity in melanoma and other solid tumors. Pharmacol Ther. (2022) 240:108211. doi: 10.1016/j.pharmthera.2022.108211
203. Fang Y, Liao G, Yu B. LSD1/KDM1A inhibitors in clinical trials: advances and prospects. J Hematol Oncol. (2019) 12:129. doi: 10.1186/s13045-019-0811-9
204. Wang M, Liu X, Guo J, Weng X, Jiang G, Wang Z, et al. Inhibition of LSD1 by Pargyline inhibited process of EMT and delayed progression of prostate cancer in vivo. Biochem Biophys Res Commun. (2015) 467:310–5. doi: 10.1016/j.bbrc.2015.09.164
205. Duan YC, Ma YC, Qin WP, Ding LN, Zheng YC, Zhu YL, et al. Design and synthesis of tranylcypromine derivatives as novel LSD1/HDACs dual inhibitors for cancer treatment. Eur J Med Chem. (2017) 140:392–402. doi: 10.1016/j.ejmech.2017.09.038
206. Xu Y, Guo B, Liu X, Tao K. miR-34a inhibits melanoma growth by targeting ZEB1. Aging (Albany NY). (2021) 13:15538–47. doi: 10.18632/aging.v13i11
207. Pattabiraman DR, Bierie B, Kober KI, Thiru P, Krall JA, Zill C, et al. Activation of PKA leads to mesenchymal-to-epithelial transition and loss of tumor-initiating ability. Science. (2016) 351:aad3680. doi: 10.1126/science.aad3680
208. Dongre A, Rashidian M, Eaton EN, Reinhardt F, Thiru P, Zagorulya M, et al. Direct and indirect regulators of epithelial-mesenchymal transition-mediated immunosuppression in breast carcinomas. Cancer Discovery. (2021) 11:1286–305. doi: 10.1158/2159-8290.CD-20-0603
209. Wang Q, Wang K, Tan X, Li Z, Wang H. Immunomodulatory role of metalloproteases in cancers: Current progress and future trends. Front Immunol. (2022) 13:1064033. doi: 10.3389/fimmu.2022.1064033
210. Kwon MJ. Matrix metalloproteinases as therapeutic targets in breast cancer. Front Oncol. (2022) 12:1108695. doi: 10.3389/fonc.2022.1108695
211. Fischer T, Riedl R. Inhibitory antibodies designed for matrix metalloproteinase modulation. Molecules. (2019) 24:2265. doi: 10.3390/molecules24122265
212. Owyong M, Chou J, van den Bijgaart RJ, Kong N, Efe G, Maynard C, et al. MMP9 modulates the metastatic cascade and immune landscape for breast cancer anti-metastatic therapy. Life Sci Alliance. (2019) 2:e201800226. doi: 10.26508/lsa.201800226
213. Ager EI, Kozin SV, Kirkpatrick ND, Seano G, Kodack DP, Askoxylakis V, et al. Blockade of MMP14 activity in murine breast carcinomas: implications for macrophages, vessels, and radiotherapy. J Natl Cancer Inst. (2015) 107:djv017. doi: 10.1093/jnci/djv017
214. Sabeh F, Shimizu-Hirota R, Weiss SJ. Protease-dependent versus -independent cancer cell invasion programs: three-dimensional amoeboid movement revisited. J Cell Biol. (2009) 185:11–9. doi: 10.1083/jcb.200807195
215. Choi HY, Chang JE. Targeted therapy for cancers: from ongoing clinical trials to FDA-approved drugs. Int J Mol Sci. (2023) 24:13218. doi: 10.3390/ijms241713618
216. Perez-Gutierrez L, Ferrara N. Biology and therapeutic targeting of vascular endothelial growth factor A. Nat Rev Mol Cell Biol. (2023) 24:816–34. doi: 10.1038/s41580-023-00631-w
217. Garcia J, Hurwitz HI, Sandler AB, Miles D, Coleman RL, Deurloo R, et al. Bevacizumab (Avastin(R)) in cancer treatment: A review of 15 years of clinical experience and future outlook. Cancer Treat Rev. (2020) 86:102017. doi: 10.1016/j.ctrv.2020.102017
218. Tang PA, Cohen SJ, Kollmannsberger C, Bjarnason G, Virik K, MacKenzie MJ, et al. Phase II clinical and pharmacokinetic study of aflibercept in patients with previously treated metastatic colorectal cancer. Clin Cancer Res. (2012) 18:6023–31. doi: 10.1158/1078-0432.CCR-11-3252
219. Van Cutsem E, Tabernero J, Lakomy R, Prenen H, Prausova J, Macarulla T, et al. Addition of aflibercept to fluorouracil, leucovorin, and irinotecan improves survival in a phase III randomized trial in patients with metastatic colorectal cancer previously treated with an oxaliplatin-based regimen. J Clin Oncol. (2012) 30:3499–506. doi: 10.1200/JCO.2012.42.8201
220. Ko J, Hyung S, Heo YJ, Jung S, Kim ST, Park SH, et al. Patient-derived tumor spheroid-induced angiogenesis preclinical platform for exploring therapeutic vulnerabilities in cancer. Biomaterials. (2024) 306:122504. doi: 10.1016/j.biomaterials.2024.122504
Keywords: immunocytes, cancer cells, direct and dual effect, tumor microenvironment, solid tumor
Citation: Ye Z, Cheng P, Huang Q, Hu J, Huang L and Hu G (2024) Immunocytes interact directly with cancer cells in the tumor microenvironment: one coin with two sides and future perspectives. Front. Immunol. 15:1388176. doi: 10.3389/fimmu.2024.1388176
Received: 19 February 2024; Accepted: 07 May 2024;
Published: 22 May 2024.
Edited by:
Jun Zhang, Kumamoto University, JapanReviewed by:
Utpreksha Vaish, University of Alabama at Birmingham, United StatesRoberto Rangel, University of Texas MD Anderson Cancer Center, United States
Chuan Lan, Affiliated Hospital of North Sichuan Medical College, China
Copyright © 2024 Ye, Cheng, Huang, Hu, Huang and Hu. This is an open-access article distributed under the terms of the Creative Commons Attribution License (CC BY). The use, distribution or reproduction in other forums is permitted, provided the original author(s) and the copyright owner(s) are credited and that the original publication in this journal is cited, in accordance with accepted academic practice. No use, distribution or reproduction is permitted which does not comply with these terms.
*Correspondence: Guoming Hu, aGdtcGxqQDEyNi5jb20=; Liming Huang, c2hhb3hpbmdobG1AMTI2LmNvbQ==
†These authors share the first authorship